DOI:
10.1039/D4SC01046A
(Edge Article)
Chem. Sci., 2024,
15, 8873-8879
Insulated π-conjugated 2,2′-bipyridine transition-metal complexes: enhanced photoproperties in luminescence and catalysis†
Received
14th February 2024
, Accepted 3rd May 2024
First published on 7th May 2024
Abstract
2,2′-Bipyridine has been identified as a privileged ligand scaffold for photofunctional transition metal complexes. We herein report on the synthesis and photoproperties of an insulated π-conjugated 2,2′-bipyridine with a linked rotaxane structure consisting of permethylated α-cyclodextrin (PM α-CD) and oligo(p-phenylene ethynylene). The insulated π-conjugated 2,2′-bipyridine exhibited enhanced ligand performance in the solid-state emitting biscyclometalated Ir complexes and visible-light-driven Ni catalysts owing to π-extension and remote steric effects based on the linked rotaxane structure.
Introduction
2,2′-Bipyridine ligand constructs π-conjugated systems containing metal atoms through N,N′-chelating coordination,1 and is therefore widely used in photofunctional transition-metal complexes including luminescent materials,2 photosensitizers,3 and photocatalysts.4 To effectively utilize visible light, π-extension of 2,2′-bipyridine has been adopted as a reliable method to reduce HOMO–LUMO gaps.5 However, open metal coordination environments based on the planarity of 2,2′-bipyridine ligands facilitate intermolecular interactions, including π–π and metal–metal interactions, as well as additional ligation to coordinatively unsaturated metal centers (Fig. 1a),6 resulting in the decreased performance of photofunctional transition-metal complexes. Spatial isolation of π-conjugated molecules based on steric protection effectively circumvents these problems.
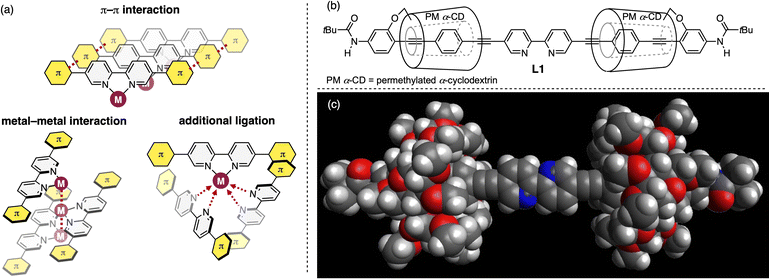 |
| Fig. 1 (a) Conceptual diagrams illustrating issues encountered in π-extended 2,2′-bipyridine ligands. Red dotted lines show π–π and metal–metal interactions, and additional ligation to the metal center. (b) Structure of insulated π-conjugated 2,2′-bipyridine L1 with the linked rotaxane structure end-capped by pivaloyl groups. (c) Optimized molecular structure of L1 calculated using the B3LYP/6-31G(d,p) level. | |
In this context, three-dimensional insulation of π-conjugated molecules by nonconductive macrocycles is a promising strategy owing to its high degree of steric protection.7 We previously reported on an insulated π-conjugated polymer containing 2,2′-bipyridine moieties that exhibits significant solid-state luminescence with typical metal coordination.8,9 The sandwich-structured 2,2′-bipyridine with linked rotaxanes consisting of permethylated α-cyclodextrin (PM α-CD) and oligo(p-phenylene ethynylene) suppresses the interaction between π-conjugated chains while allowing metal ions to easily access the coordination site. We thus designed an insulated π-conjugated 2,2′-bipyridine L1 with a linked rotaxane structure end-capped by pivaloyl groups as a ligand for photofunctional transition-metal complexes (Fig. 1b).10 This paper describes the synthesis and characterization of L1, and its high ligand performance in solid-state emitting biscyclometalated Ir complexes and visible-light-driven Ni catalysts. Experimental and computational studies indicate that the enhanced performance is attributable to π-extension and remote steric effects resulting from the linked rotaxane structure.
Results and discussion
Synthesis of insulated π-conjugated 2,2′-bipyridine
Fig. 1c shows the density functional theory (DFT)-optimized model structure of L1. Two PM α-CDs are located outside the carbon–carbon triple bond directly connected to the 2,2′-bipyridine core, covering the diphenylacetylene units extensively. Therefore, a size-limited but molecular accessible space is around the coordinating N atom (ca. 11–12 Å).
The synthesis of L1 is illustrated in Scheme 1. The Pd-catalyzed Sonogashira coupling reaction between the PM α-CD-tethered terminal alkyne 1 and 2-bromo-5-iodopyridine produced bis(phenylene ethynylene)-conjugated pyridine 2. To construct the 2,2′-bipyridine structure, sequential stannylation and Stille coupling of 2 were conducted using Bu3Sn–SnBu3 in the presence of [Pd(PPh3)4], affording 3. Following the reduction of the NO2 group with Na2S2O4, heating NH2-substituted 2,2′-bipyridine 4 in a mixed solvent of H2O and MeOH (1
:
1) induced the self-inclusion of phenylene ethynylene with PM α-CD driven by hydrophilic–hydrophobic interactions.11 Subsequent pivaloyl end capping of the terminal NH2 group in 5 furnished L1 as an air- and moisture-stable yellow-brown solid. The insulated structure of L1 was confirmed by the nuclear Overhauser effect (NOE) between the inner PM α-CD and phenylene protons in 1H–1H ROESY NMR spectra.12 The steric hindrance of the pivaloyl group inhibits PM α-CD dethreading on nonpolar solvents, such as CH2Cl2 and toluene, favoring the uninsulated structure. L2 prepared from 4via direct pivaloylation in CH2Cl2 and L3 with PM α-CDs removed served as uncovered references.
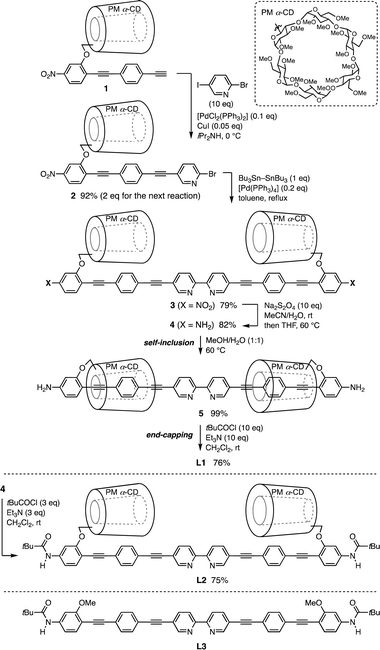 |
| Scheme 1 Synthesis and structures of L1–L3. | |
Luminescent biscyclometalated Ir complexes
Our previous studies on the utility of linked rotaxane structures in luminescent materials9a,c,d,e prompted us to apply L1 to phosphorescent biscyclometalated Ir complexes.13 The newly synthesized Ir complex [Ir(ppy)2(L1)]PF6 (ppy = 2-phenylpyridine, Fig. 2a) exhibits significant red-shift of absorption and emission peaks compared to the parent complex [Ir(ppy)2(bpy)]PF6 (bpy = 2,2′-bipyridine) (Fig. 2b and c), similar to uncovered π-extended biscyclometalated Ir complexes reported by Tatay14a and Schanze.14b Notably, unlike its uncovered counterpart [Ir(ppy)2(L3)]PF6, [Ir(ppy)2(L1)]PF6 demonstrated a higher emission quantum yield (QY) in the solid state than in the solution state (Fig. 2d). The linked rotaxane structure effectively suppresses intermolecular π–π interactions, even in the solid state where molecular aggregation is prominent (Fig. 2e). Time-dependent density functional theory (TD-DFT) calculations of [Ir(ppy)2(L3)]+ showed that the electronic transitions of [Ir(ppy)2(L1)]PF6 around 413 nm have an intraligand charge transfer (ILCT) character from the phenylene-ethynylenes to the 2,2′-bipyridine moiety, mixed with some metal-to-ligand charge transfer (MLCT) character from the Ir(III) center to the 2,2′-bipyridine moiety and ligand-to-ligand charge transfer (LLCT) character from the ppy ligand to the 2,2′-bipyridine moiety (Fig. 2f).14–17
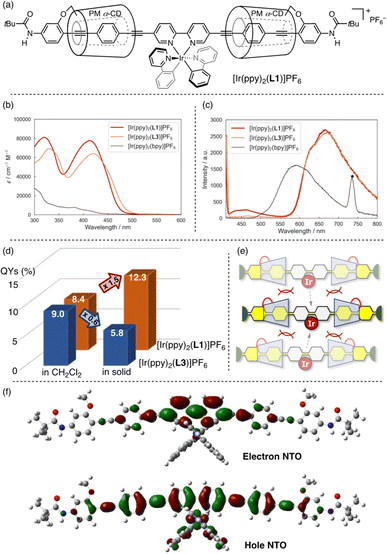 |
| Fig. 2 (a) Molecular structure of [Ir(ppy)2(L1)]PF6. (b) Absorption spectra of [Ir(ppy)2(L1)]PF6, [Ir(ppy)2(L3)]PF6, and [Ir(ppy)2(bpy)]PF6 (in CH2Cl2, 1 × 10−5 M). (c) Emission spectra of [Ir(ppy)2(L1)]PF6, [Ir(ppy)2(L3)]PF6, and [Ir(ppy)2(bpy)]PF6 (in CH2Cl2, 1 × 10−6 M, under a N2 atmosphere, excited at 405, 405, 365 nm, respectively). The asterisk indicates the second order of excitation wavelength. (d) Solution (1 × 10−6 M in CH2Cl2) and solid-state luminescence quantum yields (QYs) of [Ir(ppy)2(L1)]PF6 and [Ir(ppy)2(L3)]PF6 (excited at 405 nm under a N2 atmosphere). (e) Schematic of the aggregation suppression of [Ir(ppy)2(L1)]PF6. (f) Natural transition orbitals (NTOs) of [Ir(ppy)2(L3)]+ calculated using the CAM-B3LYP/6-31+G(d,p) (for C, H, N, O) and LANL2DZ (for Ir) levels with the PCM (CH2Cl2) solvation model. | |
Visible-light-driven Ni catalysts
The preferred ILCT nature of the excited Ir-L1 system prompted us to investigate the applicability of L1 as a ligand for visible-light-driven Ni-catalyzed cross-coupling reactions,18 classified as metallaphotoredox catalysis,19 merging transition metal catalysis with photocatalysis. Pieber et al. proposed ILCT-induced Ni-catalyzed carbon-heteroatom coupling using π-extended 2,2′-bipyridine sandwiched between two N-carbazolyl groups,20 generating catalytically active Ni(I) species through single-electron reduction of bench-stable Ni(II)-bipyridine complexes under visible-light irradiation without exogenous photocatalysts.21–23 Li et al. reported similar photocatalyst-free metallaphotoredox systems based on 2-(pyridin-2-yl)quinoline-based N,N′-chelating ligands.24
TD-DFT calculations of the model Ni complex NiCl2(L3) with an uncovered phenylene-ethynylene structure show that the 375 nm band (onset ca. 450 nm) obtained in the experimental spectrum of the Ni(II)-L1 complex in situ prepared from NiCl2·DME (DME: 1,2-dimethoxyethane) and L1 in DMF (N,N-dimethylformamide) is attributed to ILCT transitions from the phenylene-ethynylene to 2,2′-bipyridine moieties (Fig. 3a). The natural transition orbital (NTO) distribution of NiCl2(L3) was similar to that of the carbazole-bipyridine Ni(II) complex reported by Pieber et al. (Fig. 3b),20 implying that Ni(I) species could be formed from the Ni(II)-L1 system via ILCT transitions during the catalytic reaction.
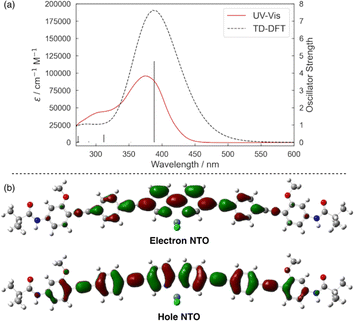 |
| Fig. 3 (a) Experimental (red solid line) and calculated (black dashed line) UV/Visible spectra of NiCl2·DME/L1 (1 : 1, in DMF) and NiCl2(L3), respectively. A vertical line is assigned to an intraligand charge transfer (ILCT) transition. (b) NTOs of NiCl2(L3) calculated using the CAM-UB3LYP/6-31+G(d,p) (for C, H, N, O, Cl) and LANL2DZ (for Ni) levels with the PCM (DMF) solvation model. | |
The Ni(II)-L1 system was prepared in situ from NiCl2·DME (1 mol%) and L1 (1 mol%), and applied to the C–O coupling of 4-bromobenzonitrile (6a, 0.1 mmol) and MeOH (4 mmol) in DMF (0.2 M) under 427 nm LED irradiation for 20 h in the presence of iPr2EtN as a base with cooling fans.18c To our delight, the reaction proceeded smoothly without the use of an exogenous photocatalyst, yielding 94% of 4-methoxybenzonitrile 7a (Table 1, entry 1). In contrast, no reaction was observed in the dark (entry 2). The reaction did not proceed without the nickel salt or L1 (entries 3 and 4). Using the simple parent 2,2′-bipyridine instead of L1 produced no reaction (entry 5). Therefore, a combination of the nickel salt, L1, and visible light irradiation is required.
Table 1 Visible-light-driven Ni-catalyzed C–O coupling between 6a and MeOHa
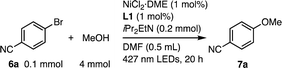
|
Entry |
Variation |
Conv. of 6ab (%) |
Yield of 7ab (%) |
Conditions: 4-bromobenzonitrile (6a, 0.1 mmol), MeOH (4 mmol), NiCl2·DME (1 mol%), L1 (1 mol%), iPr2EtN (0.2 mmol), DMF (0.5 mL), 1,3,5-trimethoxybenzene (0.1 mmol) as an internal standard, 427 nm LEDs, 20 h, with cooling fans.
Determined by 1H NMR using an internal standard. The isolated yields are shown in parentheses.
|
1 |
None |
97 |
94 (68) |
2 |
No light |
0 |
0 |
3 |
No NiCl2·DME |
0 |
0 |
4 |
No L1 |
0 |
0 |
5 |
bpy instead of L1 |
1 |
0 |
6 |
L2 instead of L1 |
29 |
27 |
7 |
L3 instead of L1 |
1 |
0 |
8 |
L1 (2 mol%) |
86 |
84 |
9 |
L2 (2 mol%) instead of L1 |
10 |
8 |
In contrast to the results obtained using L1, the uncovered counterparts L2 and L3 were less effective (27 and 0%, entries 6 and 7). This suggests that the steric effects of the linked rotaxane structure in L1 are critical. Furthermore, increasing L1 loading from 1 mol% (94%, entry 1) to 2 mol% (84%, entry 8) did not significantly reduce reaction efficiency, whereas using 2 mol% L2 resulted in almost loss of activity (8%, entry 9). Based on the optimal structure of L1 (Fig. 1c), we postulated that the linked rotaxane structure, with fixed PM α-CD positions, induced a remote steric effect that inhibited the coordination of the secondary ligand molecules to the catalytically active monochelating Ni species. This assumption is supported by our previous discovery of the mono-chelating properties of dumbbell-shaped 2,2′-bipyridines with triarylmethyl substituents at the C5 and C5′ positions.25
The Ni(II)-L1 system enabled efficient C–O coupling of several aryl halides and alcohols (Scheme 2). An electro-deficient aryl bromide, methyl 4-bromobenzoate, was an appropriate substrate, yielding 7b in 73% isolated yield. Although the reaction of 4-bromoanisole, which was electronically deactivated by the MeO group, was less effective, the use of the corresponding aryl iodide, 4-iodoanisole, with increasing the amount of MeOH and extending the reaction time (80 equiv., 48 h), allowed the coupling to produce 7c in 60% yield. The use of n-hexanol as the nucleophile also resulted in the complete consumption of 6a, yielding 7d in a reasonable yield with minor formation of the hydration product 4-hydroxybenzonitrile (7f, 28%). A secondary alcohol, cyclopentanol, was coupled with 4-iodobenzonitrile under the modified conditions (80 equiv, 48 h), yielding 7e in 50% yield.
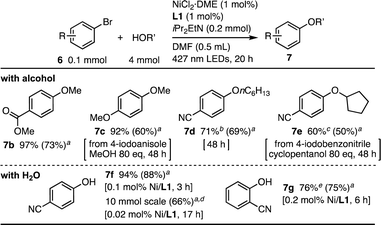 |
| Scheme 2 Substrate scope in the visible-light-driven Ni-catalyzed C–O coupling with L1. Conditions: aryl bromide (6, 0.1 mmol), HOR′ (4 mmol), NiCl2·DME (1 mol%), L1 (1 mol%), iPr2EtN (0.2 mmol), DMF (0.5 mL), 1,3,5-trimethoxybenzene as an internal standard (0.1 mmol), 427 nm LEDs, 20 h with cooling fans. Determined by 1H NMR analysis using an internal standard. a Isolated yields. b Hydration product 7f was also obtained in 28% NMR yield. c Some unreacted 4-iodobenzonitrile remained in the crude product (83% conversion). Dehaloprotonation product, benzonitrile, was also obtained in 20% NMR yield. d6a (10 mmol), H2O (40 mmol), NiCl2·DME (0.02 mol%), L1 (0.02 mol%), iPr2EtN (20 mmol), DMF (7.5 mL), 427 nm LEDs, 17 h with cooling fans. Complete conversion of 6a was observed. The dehaloprotonation product, benzonitrile, was also formed. e The homocoupling product, [1,1′-biphenyl]-2,2′-dicarbonitrile, was also obtained in 24% NMR yield. | |
H2O also contributes to C–O coupling at low catalyst loadings (Scheme 2). The reaction between 6a and H2O proceeded smoothly with the 0.1 mol% Ni-L1 catalyst, yielding 7f in 88% isolated yield. The 10 mmol-scale reaction of 6a with the 0.02 mol% Ni loading afforded 7f in 66% yield, corresponding to the turnover number (TON) of 3300. The reaction efficacy with L1 was comparable to those with other state-of-art visible-light-driven Ni catalysts.23,26 An ortho-substituted aryl bromide, 2-bromobenzonitrile, also reacted with H2O in the presence of 0.2 mol% Ni loading, yielding 7g in 75% yield, and a minor formation of the homocoupling product [1,1′-biphenyl]-2,2′-dicarbonitrile (24%).
Furthermore, L1 was applicable to Ni-catalyzed C–N coupling under visible light irradiation (Scheme 3).18b,27 With L1, the reaction between 6a and n-octylamine was successful with 1 mol% Ni loading in the presence of 1,4-diazabicyclo[2.2.2]octane (DABCO) as the base in N,N-dimethylacetamide (DMA) under 427 nm LED irradiation, yielding 8a in 86% NMR yield and a small amount of hydration product 7f (13%). In contrast, the use of L2, L3, or 2,2′-bipyridine resulted in lower efficiency, with either no reaction or only a low conversion reaction occurring, emphasizing the importance of the linked rotaxane structure.
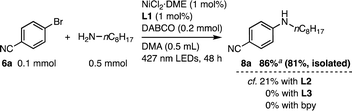 |
| Scheme 3 Visible-light-driven Ni-catalyzed C–N coupling. Conditions: 4-bromobenzonitrile (6a, 0.1 mmol), H2N-nC8H17 (0.5 mmol), NiCl2·DME (1 mol%), L1 (1 mol%), DABCO (0.2 mmol), DMA (0.5 mL), 1,3,5-trimethoxybenzene as an internal standard (0.1 mmol), 427 nm LEDs, 48 h with cooling fans. Determined by 1H NMR analysis using an internal standard. Isolated yields are shown in parentheses. a Hydration product 7f was also obtained in 13% NMR yield. | |
Conclusion
The insulated π-conjugated 2,2′-bipyridine L1 bearing a linked rotaxane structure consisting of PM α-CDs and p-phenylene ethynylenes at the C5 and C5′ positions was synthesized. L1 outperformed uncovered 2,2′-bipyridine ligands in the solid-state emitting biscyclometalated Ir complex and visible-light-driven Ni-catalyzed C–O and C–N coupling without exogenous photocatalysts owing to the π-extension and remote steric effects based on the linked rotaxane structure. The validity of ILCT processes from phenylene-ethynylene to 2,2′-bipyridine moieties via photoexcitation was demonstrated via TD-DFT calculations. This study provides new design guidelines for photofunctional transition-metal complexes and contributes to further development in the field of luminescent materials and synthetic catalysis. Further investigation of applications of the insulated π-conjugated 2,2′-bipyridines for photofunctional transition-metal complexes is ongoing in our laboratory.
Data availability
All experimental procedures and spectroscopic data can be found in the ESI.†
Author contributions
T. I. and J. T. conceived and supervised the idea. S. A. performed all experiments. All authors discussed the results and wrote the manuscript.
Conflicts of interest
The authors declare no conflict of interest.
Acknowledgements
This research was partially supported by JSPS KAKENHI Grant Number JP22K19022 (T. I.), JP22H02060 (J. T.), by JST CERST Grant Number JPMJCR19I2 (J. T.), by NEDO Grant Number JPNP21016 (J. T.), by Toshiaki Ogasawara Memorial Foundation (J. T.), and by The Yamada Science Foundation (J. T.). We thank Dr Hiromichi V. Miyagishi (Hokkaido University) for fruitful discussion on the early work.
Notes and references
- For selected reviews on 2,2′-bipyridine, see:
(a) C. Kaes, A. Katz and M. W. Hosseini, Chem. Rev., 2000, 10, 3553–3590 CrossRef PubMed;
(b) E. C. Constable and C. E. Housecroft, Molecules, 2019, 24, 3951 CrossRef CAS PubMed.
- M. D. Ward, C. M. White, F. Barigelletti, N. Armaroli, G. Calogero and L. Flamigni, Coord. Chem. Rev., 1998, 171, 481–488 CrossRef CAS.
-
(a) M. A. Munegowda, A. Manalac, M. Weersink, S. A. McFarland and L. Lilge, Coord. Chem. Rev., 2022, 470, 214712 CrossRef PubMed;
(b)
T. S. Teets and Y. Wu, Organometallic Photosensitizers, In Comprehensive Organometallic Chemistry IV, ed. G. Parkin, K. Meyer and D. O'hare, Elsevier, Oxford, UK, 2022, pp. 284–338 Search PubMed.
- K. Teegardin, J. I. Day, J. Chan and J. Weaver, Org. Process Res. Dev., 2016, 20, 1156–1163 CrossRef CAS PubMed.
- H. S. Joshi, R. Jamshidi and Y. Tor, Angew. Chem., Int. Ed., 1999, 38, 2721–2725 CrossRef PubMed.
-
(a) H. L.-K. Fu, C. Po, H. He, S. Y.-L. Leung, K. S. Wong and V. W.-W. Yam, Chem.–Eur. J., 2016, 22, 11826–11836 CrossRef CAS PubMed;
(b) P. V. Petrović, G. V. Janjić and S. D. Zarić, Cryst. Growth Des., 2014, 14, 3880–3889 CrossRef;
(c) R. Palmans, D. B. MacQueen, C. G. Pierpont and A. J. Frank, J. Am. Chem. Soc., 1996, 118, 12647–12653 CrossRef CAS.
-
(a) M. J. Frampton and H. L. Anderson, Angew. Chem., Int. Ed., 2007, 46, 1028–1064 CrossRef CAS PubMed;
(b) H. Masai and J. Terao, Bull. Chem. Soc. Jpn., 2019, 92, 529–539 CrossRef CAS;
(c) C. Pan, C. Zhao, M. Takeuchi and K. Sugiyasu, Chem.–Asian J., 2015, 10, 1820–1835 CrossRef CAS PubMed.
- T. Hosomi, H. Masai, T. Fujihara, Y. Tsuji and J. Terao, Angew. Chem., Int. Ed., 2016, 55, 13427–13431 CrossRef CAS PubMed.
- For selected examples on the linked rotaxane-base materials reported by our groups, see:
(a) T. Kaneko, G. M. Russell, Y. Kawano, H. Masai and J. Terao, Angew. Chem., Int. Ed., 2023, 62, e202305374 CrossRef CAS PubMed;
(b) S.-Y. Chou, H. Masai, M. Otani, H. V. Miyagishi, G. Sakamoto, Y. Yamada, Y. Kinoshita, H. Tamiaki, T. Katase, H. Ohta, T. Kondo, A. Nakada, R. Abe, T. Tanaka, K. Uchida and J. Terao, Appl. Catal., B, 2023, 327, 122373 CrossRef CAS;
(c) G. M. Russell, T. Kaneko, S. Ishino, H. Masai and J. Terao, Adv. Funct. Mater., 2022, 32, 2205855 CrossRef CAS;
(d) H. Masai, T. Yokoyama, H. V. Miyagishi, M. Liu, Y. Tachibana, T. Fujihara, Y. Tsuji and J. Terao, Nat. Commun., 2020, 11, 408 CrossRef CAS PubMed;
(e) H. Masai, J. Terao, S. Makuta, Y. Tachibana, T. Fujihara and Y. Tsuji, J. Am. Chem. Soc., 2014, 136, 14714–14717 CrossRef CAS PubMed;
(f) H. Masai, J. Terao, S. Seki, S. Nakashima, M. Kiguchi, K. Okoshi, T. Fujihara and Y. Tsuji, J. Am. Chem. Soc., 2014, 136, 1742–1745 CrossRef CAS PubMed;
(g) J. Terao, A. Wadahama, A. Matono, T. Tada, S. Watanabe, S. Seki, T. Fujihara and Y. Tsuji, Nat. Commun., 2013, 4, 1691 CrossRef PubMed;
(h) J. Terao, Y. Tanaka, S. Tsuda, N. Kambe, M. Taniguchi, T. Kawai, A. Saeki and S. Seki, J. Am. Chem. Soc., 2009, 131, 18046–18047 CrossRef CAS PubMed;
(i) J. Terao, S. Tsuda, Y. Tanaka, K. Okoshi, T. Fujihara, Y. Tsuji and N. Kambe, J. Am. Chem. Soc., 2009, 131, 16004–16005 CrossRef CAS PubMed.
- For selected examples on cyclodextrin-based ligands on metal complexes, see:
(a) T.-A. Phan, N. Armaroli, A. S. Moncada, E. Bandini, B. Delavaux-Nicot, J.-F. Nierengarten and D. Armspach, Angew. Chem., Int. Ed., 2023, 62, e202214638 CrossRef CAS PubMed;
(b) G. Xu, S. Leloux, P. Zhang, J. M. Suárez, Y. Zhang, E. Derat, M. Ménand, O. Bistri-Aslanoff, S. Roland, T. Leyssens, O. Riant and M. Sollogoub, Angew. Chem., Int. Ed., 2020, 59, 7591–7597 CrossRef CAS PubMed;
(c) H. Kitagishi, D. Shimoji, T. Ohta, R. Kamiya, Y. Kudo, A. Onoda, T. Hayashi, J. Weiss, J. A. Wytko and K. Kano, Chem. Sci., 2018, 9, 1989–1995 RSC;
(d) P. Zhang, C. Tugny, J. M. Suárez, M. Guitet, E. Derat, N. Vanthuyne, Y. Zhang, O. Bistri, V. Mouriès-Mansuy, M. Ménand, S. Roland, L. Fensterbank and M. Sollogoub, Chem, 2017, 3, 174–191 CrossRef CAS;
(e) M. Jouffroy, D. Armspach, D. Matt, K. Osakada and D. Takeuchi, Angew. Chem., Int. Ed., 2016, 55, 8367–8370 CrossRef CAS PubMed;
(f) M. Jouffroy, R. Gramage-Doria, D. Armspach, D. Sémeril, W. Oberhauser, D. Matt and L. Toupet, Angew. Chem., Int. Ed., 2014, 53, 3937–3940 CrossRef CAS PubMed;
(g) M. Guitet, P. Zhang, F. Marcelo, C. Tugny, J. Jiménez-Barbero, O. Buriez, C. Amatore, V. Mouriès-Mansuy, J.-P. Goddard, L. Fensterbank, Y. Zhang, S. Roland, M. Ménand and M. Sollogoub, Angew. Chem., Int. Ed., 2013, 52, 7213–7218 CrossRef CAS PubMed , for a related review, see:;
(h) D. Armspach and D. Matt, C. R. Chim., 2011, 14, 135–148 CrossRef CAS.
-
(a) H. Masai, J. Terao, T. Fujihara and Y. Tsuji, Chem.–Eur. J., 2016, 22, 6624–6630 CrossRef CAS PubMed;
(b) R. Nishiyabu and K. Kano, Eur. J. Org Chem., 2004, 2004, 4985–4988 CrossRef.
-
(a) S. Menuel, N. Azaroual, D. Landy, N. Six, F. Hapiot and E. Monflier, Chem.–Eur. J., 2011, 17, 3949–3955 CrossRef CAS PubMed;
(b) T. Fujimoto, Y. Sakata and T. Kaneda, Chem. Commun., 2000, 2143–2144 RSC.
- For selected reviews on
cyclometalated Ir(III) complexes, see:
(a) H. Shi, Y. Wang, S. Lin, J. Lou and Q. Zhang, Dalton Trans., 2021, 50, 6410–6417 RSC;
(b) C. Caporale and M. Massi, Coord. Chem. Rev., 2018, 363, 71–91 CrossRef CAS;
(c) R. D. Costa, E. Ortí, H. J. Bolink, F. Monti, G. Accorsi and N. Armaroli, Angew. Chem., Int. Ed., 2012, 51, 8178–8211 CrossRef CAS PubMed;
(d) Y. Chi and P.-T. Chou, Chem. Soc. Rev., 2010, 39, 638–655 RSC;
(e) L. Flamigni, A. Barbieri, C. Sabatini, B. Ventura and F. Barigelletti, Top. Curr. Chem., 2007, 281, 143–203 CrossRef CAS;
(f) M. S. Lowry and S. Bernhard, Chem.–Eur. J., 2006, 12, 7970–7977 CrossRef CAS PubMed.
- For cyclometalated Ir(III) complexes with diimine ligands bearing phenylene-ethynylenes, see:
(a) J. Ponce, J. Aragó, I. Vayá, J. G. Magenti, S. Tatay, E. Ortí and E. Coronado, Eur. J. Inorg. Chem., 2016, 1851–1859 CrossRef CAS;
(b) K. D. Glusac, S. Jiang and K. S. Schanze, Chem. Commun., 2002, 2504–2505 RSC.
- For selected examples of luminescence transition metal complexes with π-extended diimine ligands, see:
(a) J. M. Van Raden, S. Louie, L. N. Zakharov and R. Jasti, J. Am. Chem. Soc., 2017, 139, 2936–2939 CrossRef CAS PubMed;
(b) N. Zhao, Y.-H. Wu, H.-M. Wen, X. Zhang and Z.-N. Chen, Organometallics, 2009, 28, 5603–5611 CrossRef CAS;
(c) Q. Zhao, S. Liu, M. Shi, C. Wang, M. Yu, L. Li, F. Li, T. Yi and C. Huang, Inorg. Chem., 2006, 45, 6152–6160 CrossRef CAS PubMed;
(d) A. Kokil, P. Yao and C. Weder, Macromolecules, 2005, 38, 3800–3807 CrossRef CAS;
(e) K. A. Walters, K. D. Ley, C. S. P. Cavalaheiro, S. E. Miller, D. Gosztola, M. R. Wasielewski, A. P. Bussandri, H. van Willigen and K. S. Schanze, J. Am. Chem. Soc., 2001, 123, 8329–8342 CrossRef CAS PubMed;
(f) T. Pautzsch, C. Rode, E. Klemm and J. Prakt, Chem, 1999, 341, 548–551 CAS;
(g) K. D. Ley, C. E. Whittle, M. D. Bartberger and K. S. Schanze, J. Am. Chem. Soc., 1997, 119, 3423–3424 CrossRef CAS;
(h) Z. Peng, A. R. Gharavi and L. Yu, J. Am. Chem. Soc., 1997, 119, 4622–4632 CrossRef CAS , for a related review, see:;
(i) Y. Liu, Y. Li and K. S. Schanze, J. Photochem. Photobiol., C, 2002, 3, 1–23 CrossRef CAS.
- See the ESI† for TD-DFT calculations of [Ir(ppy)2(L3)]+ (Tables S2, S3 and Fig. S43†).
- Emission quantum yields of [Ir(ppy)2(bpy)]PF6 in the solution (in CH2Cl2, 1 × 10–5 M) and solid states were 31.6 and 19.0% (excited at 365 nm under N2 atmosphere), respectively. See the ESI† for absorption-emission spectra of all biscyclometalated Ir(III) complexes in solution and solid states (Fig. S45–S47†).
- For seminal papers on dual Ni/photoredox catalyzed cross-coupling, see:
(a) E. R. Welin, C. Le, D. M. Arias-Rotondo, J. K. McCusker and D. W. C. MacMillan, Science, 2017, 355, 380–385 CrossRef CAS PubMed;
(b) E. B. Corcoran, M. T. Pirnot, S. Lin, S. D. Dreher, D. A. DiRocco, I. W. Davies, S. L. Buchwald and D. W. C. MacMillan, Science, 2016, 353, 279–283 CrossRef CAS PubMed;
(c) J. A. Terrett, J. D. Cuthbertson, V. W. Shurtleff and D. W. C. MacMillan, Nature, 2015, 524, 330–334 CrossRef CAS PubMed;
(d) Z. Zuo, D. T. Ahneman, L. Chu, J. A. Terrett, A. G. Doyle and D. W. C. MacMillan, Science, 2014, 345, 437–440 CrossRef CAS PubMed;
(e) J. C. Tellis, D. N. Primer and G. A. Molander, Science, 2014, 345, 433–436 CrossRef CAS PubMed.
- For selected reviews on metallaphotoredox catalysis, see:
(a) A. Y. Chan, I. B. Perry, N. B. Bissonnette, B. F. Buksh, G. A. Edwards, L. I. Frye, O. L. Garry, M. N. Lavagnino, B. X. Li, Y. Liang, E. Mao, A. Millet, J. V. Oakley, N. L. Reed, H. A. Sakai, C. P. Seath and D. W. C. MacMillan, Chem. Rev., 2022, 122, 1485–1542 CrossRef CAS PubMed;
(b) S. K. Kariofillis and A. G. Doyle, Acc. Chem. Res., 2021, 54, 988–1000 CrossRef CAS PubMed;
(c) C. Zhu, H. Yue, L. Chu and M. Rueping, Chem. Sci., 2020, 11, 4051–4064 RSC;
(d) J. Twilton, C. Le, P. Zhang, M. H. Shaw, R. W. Evans and D. W. C. MacMilan, Nat. Rev. Chem, 2017, 1, 0052 CrossRef CAS;
(e) L. N. Cavalcanti and G. A. Molander, Top. Curr. Chem., 2016, 374, 39 CrossRef PubMed.
- C. Cavedon, S. Gisbertz, S. Reischauer, S. Vogl, E. Sperlich, J. H. Burke, R. F. Wallick, S. Schrottke, W.-H. Hsu, L. Anghileri, Y. Pfeifer, N. Richter, C. Teutloff, H. Müller-Werkmeister, D. Cambié, P. H. Seeberger, J. Vura-Weis, R. M. van der Veen, A. Thomas and B. Pieber, Angew. Chem., Int. Ed., 2022, 61, e202211433 CrossRef CAS PubMed.
- For selected papers on studies of Ni(I) species in Ni-catalyzed cross-coupling reactions, see:
(a) N. A. Till, S. Oh, D. W. C. MacMillan and M. J. Bird, J. Am. Chem. Soc., 2021, 143, 9332–9337 CrossRef CAS PubMed;
(b) N. A. Till, L. Tian, Z. Dong, G. D. Scholes and D. W. C. MacMillan, J. Am. Chem. Soc., 2020, 142, 15830–15841 CrossRef CAS PubMed;
(c) R. Sui, Y. Qin and D. G. Nocera, Angew. Chem., Int. Ed., 2020, 59, 9527–9533 CrossRef PubMed;
(d) S. I. Ting, S. Garakyaraghi, C. M. Taliaferro, B. J. Shields, G. D. Scholes, F. N. Castellano and A. G. Doyle, J. Am. Chem. Soc., 2020, 142, 5800–5810 CrossRef CAS PubMed;
(e) R. Sun, Y. Qin, S. Ruccolo, C. Schnedermann, C. Costentin and D. G. Nocera, J. Am. Chem. Soc., 2019, 141, 89–93 CrossRef CAS PubMed;
(f) B. J. Shields, B. Kudisch, G. D. Scholes and A. G. Doyle, J. Am. Chem. Soc., 2018, 140, 3035–3039 CrossRef CAS PubMed.
- Recently, photocatalyst-free, light-induced Ni-catalyzed cross-coupling reactions with or without simple 2,2′-bipyridine-based ligands have been reported. For selected examples, see:
(a) H. Luo, G. Wang, Y. Feng, W. Zheng, L. Kong, Y. Ma, S. Matsunaga and L. Lin, Chem.–Eur. J., 2023, 29, e202202385 CrossRef CAS PubMed;
(b) L. Yang, H.-H. Lu, C.-H. Lai, G. Li, W. Zhang, R. Cao, F. Liu, C. Wang, J. Xiao and D. Xue, Angew. Chem., Int. Ed., 2020, 59, 12714–12719 CrossRef CAS PubMed;
(c) C.-H. Lim, M. Kudisch, B. Liu and G. M. Miyake, J. Am. Chem. Soc., 2018, 140, 7667–7673 CrossRef CAS PubMed ; for a related review, see:;
(d) H. Luo, Y. Feng and L. Lin, ChemCatChem, 2023, 15, e202300303 CrossRef CAS.
- Recently, heterogeneous dual Ni/photoredox catalyst systems with π-conjugated covalent organic frameworks have been reported. For selected examples, see:
(a) Y. Fan, D. W. Kang, S. Labalme and W. Lin, J. Am. Chem. Soc., 2023, 145, 25074–25079 CrossRef CAS PubMed;
(b) Y. Fan, D. W. Kang, S. Labalme, J. Li and W. Lin, Angew. Chem., Int. Ed., 2023, 62, e202218908 CrossRef CAS PubMed;
(c) A. Jati, S. Dam, S. Kumar, K. Kumar and B. Maji, Chem. Sci., 2023, 14, 8624–8634 RSC.
- J. Li, C.-Y. Huang and C.-J. Li, Chem, 2022, 8, 2419–2431 CAS.
- Y. Kim, T. Iwai, S. Fujii, K. Ueno and M. Sawamura, Chem.–Eur. J., 2021, 27, 2289–2293 CrossRef CAS PubMed.
- Y. Pan, N. Zhang, C.-H. Liu, S. Fan, S. Guo, Z.-M. Zhang and Y.-Y. Zhu, ACS Catal., 2020, 10, 11758–11767 CrossRef CAS.
- M. Kuai, Z. Jia, L. Chen, S. Gao and W. Fang, Eur. J. Org Chem., 2024, 27, e202300933 CrossRef CAS.
Footnote |
† Electronic supplementary information (ESI) available: Experimental procedures, compound characterizations, and computational studies. See DOI: https://doi.org/10.1039/d4sc01046a |
|
This journal is © The Royal Society of Chemistry 2024 |
Click here to see how this site uses Cookies. View our privacy policy here.