DOI:
10.1039/D4SC01030B
(Review Article)
Chem. Sci., 2024,
15, 6285-6313
Emerging single-atom catalysts in the detection and purification of contaminated gases
Received
14th February 2024
, Accepted 1st April 2024
First published on 2nd April 2024
Abstract
Single atom catalysts (SACs) show exceptional molecular adsorption and electron transfer capabilities owing to their remarkable atomic efficiency and tunable electronic structure, thereby providing promising solutions for diverse important processes including photocatalysis, electrocatalysis, thermal catalysis, etc. Consequently, SACs hold great potential in the detection and degradation of pollutants present in contaminated gases. Over the past few years, SACs have made remarkable achievements in the field of contaminated gas detection and purification. In this review, we first provide a concise introduction to the significance and urgency of gas detection and pollutant purification, followed by a comprehensive overview of the structural feature identification methods for SACs. Subsequently, we systematically summarize the three key properties of SACs for detecting contaminated gases and discuss the research progress made in utilizing SACs to purify polluted gases. Finally, we analyze the enhancement mechanism and advantages of SACs in polluted gas detection and purification, and propose strategies to address challenges and expedite the development of SACs in polluted gas detection and purification.
1. Introduction
The emission of pollutants such as sulfur oxides (SOx), nitrogen oxides (NOx), carbon oxides (COx), and volatile organic compounds (VOCs) from activities and industrial manufacturing processes of human beings poses a substantial risk to human health, leading to emergence of various diseases, disruption of ecological balance, and decline in biodiversity.1–4 The real-time detection and purification of contaminated gases are therefore of paramount importance and merit significant attention.5 The monitoring and purification processes both involve adsorption and activation of contaminated gas molecules, highlighting the pivotal role of catalysis in contaminated gas detection and purification. Therefore, a profound comprehension of catalytic mechanisms in gas detection and purification processes is indispensable for the development of cutting-edge catalysts.
Single atom catalysts (SACs) demonstrate maximum atom utilization efficiency with tunable electronic structure through manipulating the single atom centers, coordination atoms and/or coordination numbers.6–12 Moreover, SACs offer an ideal platform to investigate the structure–performance relationship thanks to their homogeneous isolated active centers.13–16 Most gas sensors operate based on catalytic processes, making SACs ideal for high-performance gas sensing applications.17,18 Early research showed that compared to traditional gas sensing materials, SACs could maintain high sensitivity and rapid response even at low temperatures.19–22 The efficient catalytic activity of metal single atoms and their synergistic effects with metal supports play crucial roles in this context.23–25 Over the past decades, various strategies have been employed to control emissions of contaminated gases, including absorption, condensation, biological degradation, and catalysis. Among these approaches, catalysis shows great promise in eliminating contaminated gases, advancing clean energy development, and producing high-value chemicals. Thus, it is crucial to design and develop highly efficient and chemically stable heterogeneous catalysts with adjustable structures and abundant active sites to address challenges associated with contaminated gas detection and purification.26,27 SACs have demonstrated exceptional performance in purifying contaminated gas by converting it into harmless products and even value-added fuels/chemicals.28,29 Understanding the scientific mechanisms of SACs in this field will greatly contribute to advancing their applications in gas sensing and gas purification.30,31
In this review, the characterization methods to determine the structure of SACs is first outlined. Next, the working mechanisms of gas sensors are systematically summarized, followed by a comprehensive description of research progress in gas sensing using SACs, focusing on chemical sensitization, electronic sensitization, and synergistic sensitization. Subsequently, the purification mechanism of contaminated gas is summarized, followed by a systematic overview of purifying polluted gases using SACs with a focus on photocatalysis and electrocatalysis. Last but not least, some issues in this research field are put forward and the corresponding solutions are suggested (Scheme 1).
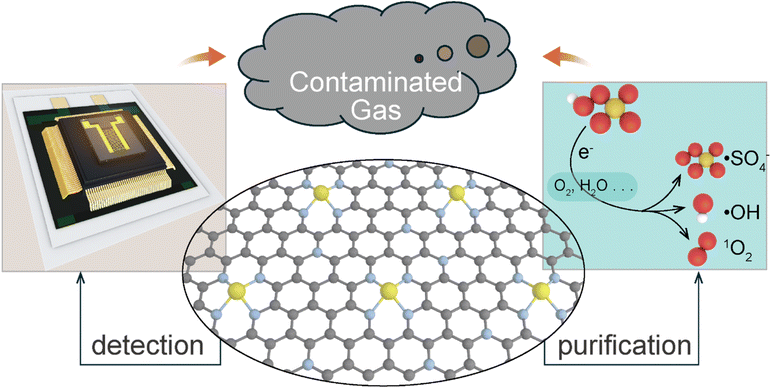 |
| Scheme 1 A comprehensive summary diagram illustrating the overview of the manuscript. | |
2. Characterization of SACs
Structural identification of SACs heavily depends on characterization techniques.32–35 Recent advancements in electron microscopy, structure-sensitive spectroscopy, and in situ/operando methodologies have led to a deep understanding of heterogeneous catalysis, including the structure of the catalyst and catalytic process.36–41Fig. 1 summarizes the commonly used techniques for characterizing structures of SACs at the atomic scale. The atomic structure of SACs can be probed by using high-resolution transmission electron microscopy (HRTEM) and scanning transmission electron microscopy (STEM). HRTEM enables direct visualization of individual atoms, revealing their sizes, shapes, and distributions. STEM, particularly when combined with energy dispersive X-ray spectroscopy (EDX), facilitates mapping of individual atomic elements on the supporting material to confirm their presence and provide information about their spatial distribution. The characterization of SACs' electronic structure primarily involves X-ray photoelectron spectroscopy (XPS), X-ray absorption spectroscopy (XAS), and infrared spectroscopy. XPS can offer insights into the oxidation states and electronic structure of individual atoms, facilitating comprehension of the interaction between single atoms and their support material as well as the impact on catalytic behavior. XAS, encompassing both X-ray absorption near edge structure (XANES) and extended X-ray absorption fine structure (EXAFS), can provide valuable information regarding the local structure and coordination environment of single atoms, thereby confirming their monatomic properties. Furthermore, infrared spectroscopy (IR), particularly when combined with CO as a probe molecule, can furnish details about adsorption sites on SACs while aiding in verifying the presence of single atoms and offering insights into their catalytic behaviors. These characterization methods, often used in combination, can provide comprehensive information about the structure, composition, and behavior of SACs. However, characterizing SACs is still challenging due to their unique nature, and ongoing research is aimed at developing more advanced characterization techniques for these materials.42–44 A comprehensive understanding of the structure of SACs is crucial for designing more efficient SACs.45–48Fig. 2 illustrates the general process for determining whether a prepared catalyst is a SAC. Firstly, X-ray diffraction (XRD) analysis is performed to verify characteristic peaks corresponding to metal compounds in the sample. Subsequently, electron microscopy techniques including scanning electron microscopy (SEM), transmission electron microscopy (TEM), and high-angle annular dark-field scanning transmission electron microscopy (HAADF-STEM) are utilized to examine whether nanoparticles, sub-nanoparticles, or nanoclusters exist in the sample. Among these electron microscopy methods, HAADF-STEM provides high-quality imaging maps enabling identification of individual metal atoms through dispersed bright spots.49–51 Finally, X-ray absorption spectroscopy (XAS) is employed to ascertain whether there exists metal–metal coordination. If all answers to these questions are “No”, it indicates that the introduced metal atoms are atomically dispersed. A comprehensive analysis of the coordination structure of atomically dispersed metal atoms can be conducted using various techniques, such as X-ray photoelectron spectroscopy (XPS) and X-ray absorption near edge structure (XANES) spectroscopy to determine the valence states of single atom centers in SACs; extended X-ray absorption fine structure (EXAFS) spectroscopy to get the coordination numbers; and X-ray emission spectroscopy (XES) together with density functional theory (DFT) calculation and spectroscopy simulation to obtain structural details.52–56
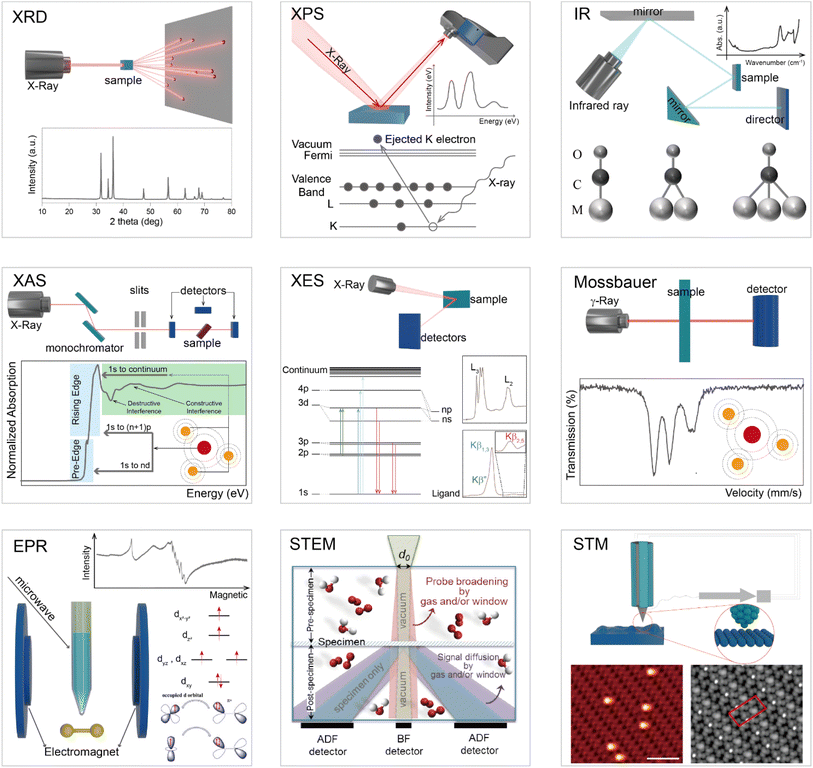 |
| Fig. 1 Schematic illustration of different characterization methods for studying SACs. | |
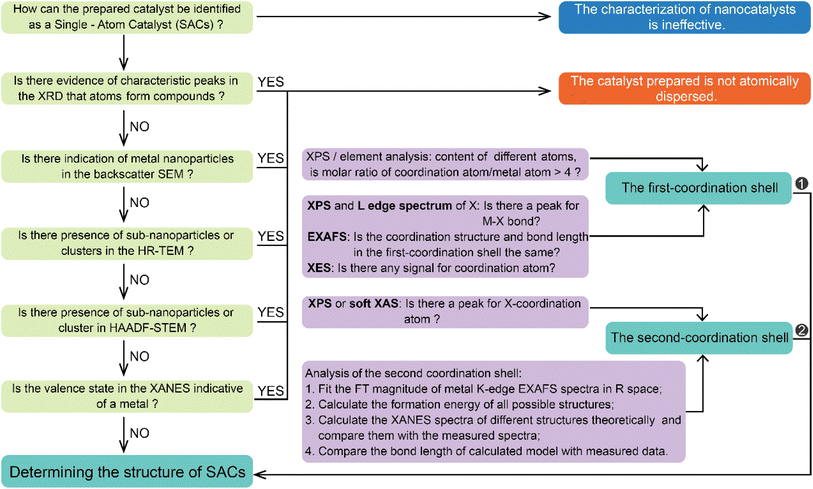 |
| Fig. 2 The well-accepted protocol for determining the structure of SACs. | |
3. Application of surface acoustic wave (SAW) in gas detection
Since 1999, Kevin Ashton's development of the Internet of Things (IoT) has garnered significant recognition from technical communities and scientific experts. Sensors, serving as the fundamental building blocks of IoT, offer substantial advantages for studying diverse domains such as environmental conditions, climate patterns, human health status, food quality assessment, and water analysis. For instance, wearable sensors capable of detecting respiration or subcutaneous tissue can be employed for medical diagnostics; gas or water quality sensors can establish robust industrial safety measures and environmental monitoring systems; paper-based sensors enable instantaneous determination of food freshness and safety levels. However, despite their pivotal role in numerous scenarios, sensors still face challenges related to insufficient sensitivity, selectivity limitations, stability issues, and reliability concerns, which hinder their ability to meet expanding demands. Sensors still encounter difficulties in detecting trace analytes at parts per billion (ppb) levels or lower concentrations while differentiating between similar compounds like methanol and ethanol. Therefore, it is imperative to develop highly sensitive sensors with strong selectivity that exhibit exceptional reliability and stability. One effective approach is to optimize the sensing material/catalyst. Precious metals such as Pt, Pd, and Ir, as well as metal oxides like ZnO, SnO2, and Co3O4 are commonly employed as catalysts due to their unoccupied d-electron orbitals. However, the limited number of unsaturated coordination atoms on the surface of particle catalysts leads to low utilization of active sites. Reducing the size of metal nanoparticles is considered as an efficient strategy for increasing the number of catalytically exposed atoms, which consequently modifies the surface atomic structure, electron configuration, and surface defects. These alterations can generate favorable geometric and electronic effects on the coordination environment and enhance catalyst activity. A typical example illustrating this concept is single-atom catalysts (SACs), where active metal sites are isolated on a carrier or stabilized by a non-metallic ligand to maximize atomic utilization efficiency and catalytic activity. The significant enhancement of catalytic activity and selectivity in electrocatalysis, thermal catalysis, photocatalysis, and enzyme catalysis by SACs has been well established. These principles have paved the way for the development of various sensing methods such as electrochemistry, chemical resistance, colorimetry, luminescence, etc. In comparison to nanoparticles, nanoclusters, and bulk catalysts, SACs offer several advantages: (1) maximizing the utilization of catalytic metals while minimizing their consumption; (2) exhibiting unexpected stability and selectivity owing to their customizable coordination environment; (3) providing a comprehensive understanding of the mechanism behind the coordination environment. Although sensing with SACs is still at an early stage of development, remarkable progress has been made since 2016 with immense potential. Therefore, this section begins by providing a concise overview of the fundamental principles underlying gas sensors, followed by a comprehensive examination of three distinct mechanisms for enhancing gas sensitivity in single-atom catalysts, drawing upon recent advancements in the field of gas sensor research.
3.1 Working mechanism of gas sensors based on SACs
Gas sensors are typically made of metal oxides or conducting polymers, which exhibit changes in electrical conductivity when exposed to different gases.57–59 The change in conductivity can be attributed to the change in electronic properties induced by adsorption/desorption of gas molecules on the surface of sensing materials.60,61 Physisorption and chemisorption are two different types of adsorption processes that occur on the surface of semiconductors in gas sensors. They play a crucial role in the sensing mechanism of these devices. The process of physical adsorption involves the adherence of gas molecules to the sensor surface through weak van der Waals forces (or London dispersion forces). These relatively weak forces allow for reversible adsorption and desorption processes at low temperatures. Importantly, no chemical bonding forms between the gas molecules and the sensor surface in physical adsorption. This non-specific process can occur for any gas molecules, and its capacity increases with increasing surface area. Additionally, physical adsorption typically results in multilayered adsorption as there is no limit to the number of layers that can be formed. The process of chemisorption involves the formation of a chemical bond between gas molecules and the sensor surface. This interaction is characterized by its increased strength and specificity compared to physical adsorption. During chemisorption, gas molecules undergo reactions with the sensor surface, resulting in the creation of new compounds. This process is typically irreversible and occurs at elevated temperatures. Chemisorption generally leads to monolayer adsorption as further adsorption becomes hindered once a monolayer is formed. In terms of gas sensors, both physisorption and chemisorption can influence their responses; chemisorption is often more desirable due to its ability to provide higher sensitivity and selectivity through specific interactions between gas molecules and the sensor surface. Several theories, such as the chemisorbed oxygen model,62,63 grain boundary barrier model,64,65 bulk resistance model,66 space-charge layer model (electron depletion layer (EDL) and hole accumulation layer (HAL)),67,68 can explain the variations in conductivity. These theories focus on electron transfer between gas molecules and semiconductor surfaces. When the electron affinity of the gas exceeds the work function of the semiconductor, electrons will be transferred from the semiconductor surface to adsorbed gas molecules, leading to the formation of adsorbed gas anions (Fig. 3a), which alters the electronic conductivity of the semiconductor.69–71 In addition to the gas molecules adsorbed on the semiconductor surface, the chemisorbed oxygen species (O2−, O−, O2−) also play a crucial role in the gas sensor, significantly influencing the surface conductivity that is closely related to the operating temperature and type of semiconductor, and therefore these factors ultimately impact the characteristics of semiconductors in gas sensing applications.60,62,72 The process of generating chemisorbed oxygen species on the semiconductor surface can be summarized by formulae (1)–(4).73–76 | O2(gas) → O2(adsorbed), (air) | (1) |
| O2(adsorbed) + e− → 2O−, (air, <150 °C) | (2) |
| O2(adsorbed) + e− → O2−, (air, 150–400 °C) | (3) |
| O2(adsorbed) + e− → O2−, (air, >400 °C) | (4) |
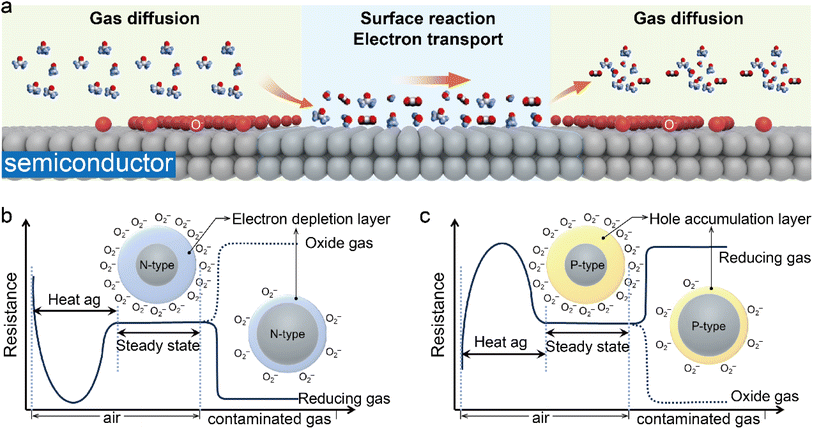 |
| Fig. 3 (a) Schematic illustration showing the three steps taking place over a semiconductor for sensing gas molecules. Schematic diagram showing the detection principle of n-type (b) and p-type (c) semiconductors for sensing gas molecules. | |
For an n-type semiconductor, the gas–surface interaction can be explained as follows (Fig. 3b): initially, upon heating to a specific temperature, oxygen in the air is adsorbed onto designated sites on the semiconductor surface. Subsequently, the adsorbed oxygen molecules capture electrons from the semiconductor conduction band, forming chemisorbed oxygen species (O2−, O−, O2−) and simultaneously creating an EDL at the semiconductor–gas interface.67,77–79 This EDL causes an increased electron transfer barrier. Reductive gases like ethanol can react with chemisorbed oxygen species to release electrons back into the semiconductor, thereby reducing the resistance.80–82 After consumption of the chemisorbed oxygen species, oxygen molecules can be re-adsorbed onto the semiconductor surface to produce new oxygen anions until the reaction reaches equilibrium. Conversely, introducing an oxidizing gas will further deplete electrons and consequently increase the resistance in the semiconductor. For a p-type semiconductor, when it comes into contact with oxygen molecules (Fig. 3c), the oxygen molecules will capture electrons from the semiconductor valence band, converting them into chemisorbed oxygen ions.83 Since the p-type semiconductor conducts current through holes, this loss of electrons leads to the formation of an HAL on the semiconductor surface and thus a reduction in the charge transfer barrier. When detecting reducing gases, these gas molecules react with adsorbed oxygen ions on the semiconductor surface, releasing electrons back into the semiconductor where they recombine with holes.84 This reduces hole concentration and diminishes the hole accumulation layer, resulting in decreased conductivity and increased resistance in the semiconductor. Conversely, when oxidizing gases are detected, the oxidizing gas molecules can capture more electrons from the semiconductor, which will increase hole concentration and further enhance the conductivity while reducing the resistance. Gas detection is achieved by monitoring changes in resistance before and after gas exposure. The resistances of semiconductor gas sensors, whether n-type or p-type, are influenced by various factors. These factors can be categorized into internal and external factors. Internal factors primarily include the following aspects. (a) Doping level: the resistance of a semiconductor is directly correlated with its doping level. In n-type semiconductors, doping introduces additional free electrons, thereby enhancing the conductivity and reducing the resistance. Similarly, in p-type semiconductors, doping generates more holes which can also enhance the conductivity and reduce the resistance. (b) Temperature: the resistance of a semiconductor is temperature dependent. With increasing temperature, there is an increase in the number of free carriers (n-type electrons or p-type holes), resulting in a decrease in resistance. External factors mainly encompass: (a) gas concentration: when the semiconductor comes into contact with target gases within a gas sensor setup, its resistance changes accordingly. For an n-type semiconductor interacting with reducing (oxidizing) gases like CO (O2), its resistance typically decreases (increases). For a p-type semiconductor, the resistance change is in the opposite direction to an n-type semiconductor. (b) Humidity: water vapor can affect the resistance of semiconductors by providing or accepting free carriers. (c) Crystal lattice structure: the surface morphology of semiconductors will impact the impurity concentration and grain boundary count; surfaces that are rougher with more grain boundaries tend to disperse carriers while increasing the impurity concentration.
In brief, the gas sensor system comprises of a sensor device, a conversion circuit, and a power supply.85–88 The gas sensor device is responsible for detecting contaminated gases, while the conversion circuit transforms the chemical signal from the gas sensor into an electrical signal. The specific operation principle involves the adsorption or reaction of contaminated gas molecules onto a gas-sensitive material, leading to alterations in the resistance/current/voltage.89–91 Thus, comprehending the sensitization mechanism of gas-sensitive materials is pivotal for investigating adsorption, chemical reaction, and desorption of oxygen molecules and polluted gases on semiconductor materials.92–94 SACs serve as an exemplary platform for exploring these sensitization processes by offering novel insights into the sensitization mechanisms at the atomic level.95 In the context of chemical sensitization, SACs exhibit unique catalytic properties in various chemical reactions due to their distinct electronic structure and unsaturated coordination environment. Additionally, SACs can provide active sites to adsorb oxygen and pollutant gas molecules, which may migrate to the surface of the sensing material through a phenomenon known as the surface spillover effect.96,97 In terms of electronic sensitization, SACs form Schottky junctions with carriers by exploiting differences in band gap and work function, thereby facilitating electron transport.98,99 Furthermore, SACs are able to react with oxygen to generate metal oxides and establish heterojunction interfaces with carrier materials. The manipulation of loss layers at these heterojunction interfaces can effectively regulate electron transport and ultimately enhance sensor performance.100 For synergistic sensitization, SACs act as activation centers for oxygen molecules by modulating their activation kinetics.101 Moreover, the activated oxygen molecules influenced by SACs can also impact gas sensitivity through an overflow effect.
3.2 Chemical sensitization of SACs
Chemical sensitization sensors for gas detection have been extensively investigated. Unlike electrochemical sensors, chemical sensitization sensor devices typically consist of a smaller number of interdigitated electrodes and metal oxide semiconductor (MOS) materials, eliminating the need for electrolyte and reference electrodes. Chemical sensitization sensors and electrochemical sensors are two commonly used gas detection systems, each with its own distinct advantages. In comparison to electrochemical sensors, chemical sensitization sensors possess the following advantages: (a) chemical sensitization sensors typically exhibit higher sensitivity and can be applied to detect extremely low concentration target gases. (b) Chemical sensitization allows for high selectivity towards specific gases by utilizing specific catalysts or active substances that only react with the target gases. This can reduce interference from other gases, a problem more frequently encountered in electrochemical sensors. (c) Chemical sensitization sensors offer superior long-term stability and are not affected by environmental conditions such as temperature and humidity, which can impact the performance of electrochemical sensors. (d) Chemical sensitization sensors usually operate without an external power support, making them more convenient and cost-effective for use in remote or hard-to-reach locations. (e) The design and manufacturing processes for chemical sensitization sensors are generally simpler. The resistance or direct current of chemical sensitization sensors is commonly measured by the galvanostatic or constant bias voltage method known as conductometry. To ensure accurate measurements, it is crucial for chemical sensitization sensors to exhibit appropriate conductivity. Insufficient conductivity will result in unmeasurable current or voltage drop. Pd single atoms (SAs) supported on TiO2 exhibit a p-type characteristic that can generate active oxygen species (such as O2−) from adsorbed oxygen, resulting in a state of high conductivity. TiO2 is a widely used n-type semiconductor. By introducing Pt onto TiO2, new energy levels can be created within the bandgap of TiO2, which can generate hole carriers – a characteristic of p-type semiconductors. This occurs due to charge carrier modification and Schottky barrier formation between Pt and TiO2, which separates electron–hole pairs and improves hole conductivity. Using p-type semiconductors in gas-sensitive applications offers advantages such as enhanced selectivity and sensitivity to certain types of gases. The catalytic activity of Pt can enhance the gas sensitive properties of the material by improving chemical reactions on its surface while also increasing oxygen vacancies that serve as electron capture sites thereby transforming TiO2 into a p-type semiconductor. When reductive analyte CO molecules adhere to the Pd SAC, they undergo oxidation and release free electrons into the p-type Pd SAC, depleting hole carriers and leading to reduced conductivity. Upon removal of gaseous CO2 molecules, oxygen molecules re-adhere and restore the high conductivity state of Pd SAC. Gas sensors based on conventional semiconductors such as ZnO, SnO2, TiO2, and In2O3 typically require high operating temperatures, resulting in increased energy consumption and limited applicability.102–105 SACs offer advantages in chemical sensitization sensing due to their abundant active sites and exceptional oxygen adsorption ability,106,107 which can enhance the catalytic spillover effect, leading to improved sensitivity and selectivity while reducing the required operating temperature.108–110 For instance, in our previous study, Pt single atoms and Pt nanoparticles were deposited onto ZnO using a dip-coating method as verified by EXAFS (Fig. 4a) and CO DRIFTS (Fig. 4b).111 The Pt1/ZnO gas sensor demonstrated a significant response to triethylamine (TEA) gas, while its responses to other gases were not prominent, indicating high selectivity of Pt1/ZnO towards TEA. Conversely, both ZnO and Pt-NP/ZnO sensors showed low responses to all gases (Fig. 4c). The supports in SACs often possess porous structures with regular patterns that have high specific surface areas, facilitating efficient molecule/ion transport and enhancing the adsorption capacity to expand the resistance range.112 Xiang et al. reported that the three-dimensional ordered mesoporous structure of SnO2 (3DOM) was significantly enhanced by incorporating Pt.113 EXAFS analysis revealed that atomically dispersed Pt predominated on the surface of 3DOM at a concentration of 0.20 wt% Pt/SnO2 (Fig. 4d). This resulted in a large number of active sites, a high Hall mobility, and a great content of reactive oxygen species, while simultaneously reducing the response energy barrier. The sensor made of 0.20 wt% Pt/SnO2 demonstrated exceptional performance with a low operating temperature of 80 °C and an impressive detection limit (0.32 ppb) for TEA (Fig. 4e). The gas sensing performance of Pt SAs could be significantly enhanced when loaded onto a three-dimensional ordered mesoporous WO3, as demonstrated by Gu and co-workers (Fig. 4f).114 Moreover, the limitations in sensitivity and selectivity commonly associated with chemical sensing materials at room temperature (RT) can be overcome by adjusting the surface coordination of SACs. The exceptional capability of Pd1/TiO2, which utilizes a solitary Pd atom to generate adsorbed O2−, along with its high efficiency and specificity for catalytic CO oxidation under ambient conditions, gives an unparalleled level of sensitivity compared to previously reported room temperature sensing materials.115 This finding is reminiscent of observations made with other SACs employed for CO conversion (Fig. 4g). Generally, when SAs are loaded onto metal oxide semiconductor (MOS), their coordination with oxygen is fixed within the lattice structure.116 This minimizes oxygen depletion during gas-sensitive reactions and allows for easy replenishment by atmospheric oxygen, leading to exceptional stability typically exhibited in SACs. Murata et al. demonstrated that Pt atoms dispersed randomly in a SnO2 lattice underwent partial reduction without losing their lattice positions under reducing gases such as 1% CH4 and 1% H2.117In situ XAFS measurements showed no formation of Pt nanoparticles even during the reaction (Fig. 4h). For MOS, introducing SAs may cause loss of complete coordination between metal and oxygen atoms and result in incomplete metal–oxygen vacancy centers, however, these vacancies can be replenished from the surrounding air to maintain high stability.118,119 The experimental phenomenon in the Pt1/WO3 system was confirmed by first-principles calculations, wherein the introduction of Pt SAs led to a reduction in Fermi level of WO3 and an enhancement of its reactive oxygen capability (Fig. 4i).120
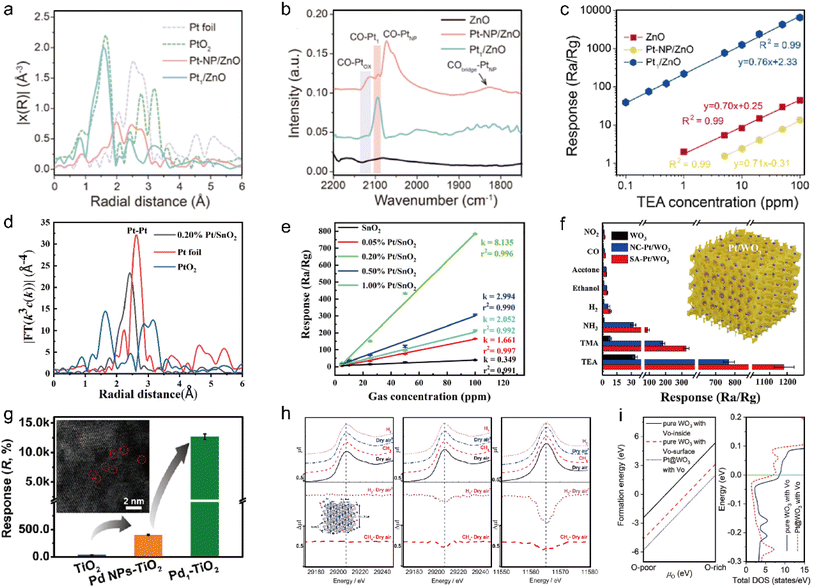 |
| Fig. 4 (a) Fourier-transformed (FT) k3-weighted EXAFS spectra of the Pt K-edge for Pt1/ZnO, Pt-NP/ZnO, PtO2, and Pt foil. (b) CO-DRIFTS of pure ZnO, Pt1/ZnO, and Pt-NP/ZnO. (c) The linear relationship between response and TEA concentration within 0.1 to 100 ppm. Reproduced with permission from ref. 111 Copyright 2023, American Chemical Society. (d) EXAFS spectra in R space for Pt foil, PtO2, and 0.2 wt% Pt/SnO2. (e) Pt/SnO2 sensor response to different TEA concentrations. Reproduced with permission from ref. 113 Copyright 2022, American Chemical Society. (f) Responses of the sensors to different gases (the concentrations of TEA, TMA and hydrogen are 50 ppm and the concentrations of other gases are 100 ppm. The operating temperature is 240 °C and the error bars represent the standard deviation (SD) of the responses for five independent determinations). Reproduced with permission from ref. 114 Copyright 2019, Elsevier. (g) Column charts of responses to 100 ppm CO over pristine TiO2, Pd NPs–TiO2, and Pd1–TiO2 nanoflowers. Inset shows an AC-HAADF-STEM image of Pd1–TiO2. Reproduced with permission from ref. 115 Copyright 2021, American Chemical Society. (h) XANES spectra of SnO2 and 7.5 at% Pt–SnO2 recorded at 703 K. Left: Sn K-edge of SnO2, center: Sn K-edge, and right: Pt L3-edge of Pt–SnO2. Dry air in the figure means air treatment after CH4 flow. Reproduced with permission from ref. 117 Copyright 2022, American Chemical Society. (i) Left: formation energies of VO in pure WO3 and Pt anchored WO3 slab as a function of the chemical potential of O atom (μo). VO-inside/surface indicates that the VO is inside/at the surface of the WO3 slab. Right: the total DOS of pure WO3 and Pt anchored WO3 slab with VO. The Fermi level is set to zero. Reproduced with permission from ref. 120 Copyright 2022, Wiley-VCH. | |
3.3 Electronic sensitization of SACs
Enhancement of gas response by tuning the concentration of charge carriers is referred to as “electronic sensitization”. The formation of a Schottky junction between surface-active centers and carriers is crucial for enhancing electron transfer in electronic sensitization.121,122 SACs possess unique properties to facilitate chemical reactions with high selectivity and efficiency. Additionally, SACs present in air can react with molecular oxygen to form metal oxides, creating heterojunctions with the carrier material.123 Heterojunctions refer to interfaces between two different materials that have distinct electronic properties.124–126 A heterojunction plays a significant role in modulating electron transport by altering the depletion layer between the two different materials. Precise control of the heterojunction can enhance sensor performance, opening possibilities for developing advanced sensing technologies capable of detecting various substances with high sensitivity and selectivity. According to a study conducted by Shin et al., Pt SA-anchored carbon nitride nanosheets were integrated into a 1D nanofiber structure containing SnO2 (Pt1-N/CSnO2).127 The Pt1-N/C site exhibited strong adsorption of O2, with an adsorption energy of −1.93 eV. Furthermore, the O–O bond length of O2 adsorbed on the Pt atom was longer compared to that on Pt bulk. These findings suggested easier adsorption and dissociation of O2 on Pt SA in comparison to that on SnO2, resulting in an increased concentration of chemisorbed oxygen species (Fig. 5a). The incorporation of Pt1-N/C into SnO2 led to a noticeable decrease of work function to 4.38 eV, indicating electron transfer from Pt1-N/C to the main body of SnO2. This observation supported the formation of the heterojunction as a second enhancement mechanism, where enhanced oxygen species adsorption occurred at the electron accumulation layer formed between SnO2 and carbon nitride (Fig. 5b). Consequently, this increased the concentration of chemisorbed oxygen on the surface of Pt1-N/C-SnO2. Gas sensing tests showed that compared to the state-of-the-art formaldehyde gas sensors (Fig. 5c), Pt1-N/C-SnO2 gas sensor exhibited superior performance. Sun et al. developed a novel type of Pt1/SnO2@SiC multi-heterojunction, which demonstrated that the incorporation of Pt SAs into SnO2 altered its electronic structure, resulting in efficient electron transfer within the formed heterojunction.128 During gas sensing, the redox reaction between ethanol gas and ionized oxygen on Pt1/SnO2 generated electrons that were transferred to the heterojunction sensing material (Fig. 5d). The presence of Pt SAs significantly enhanced the electron transfer rate. Gas sensitivity tests revealed a significant improvement in ethanol detection performance for Pt1/SnO2@SiC (Fig. 5e). To elucidate the sensing mechanism, the carrier concentration of Fe1/NC was measured by the Hall-effect measurement, and DFT calculations were performed based on an FeN4 coordination structure (Fig. 5f).129 DFT analysis showed that Fe1/NC exhibited n-type semiconductor behavior with a band gap of 0.5 eV (Fig. 5g), consistent with the result obtained from the Hall effect measurement. Like n-type MOS gas sensing materials, it was anticipated that NO2, as an oxidizing gas, would induce an increase in electrical resistance when interacting with Fe1/NC. To clarify the selective response of the Fe1/NC gas sensor towards NOx, the adsorption structures and energies of various experimentally measured gases were further calculated by DFT (Fig. 5h). Based on the above research examples, it is evident that SACs hold immense potential in optimizing gas detection performance. Firstly, SACs possess unique electronic properties that enable efficient adsorption and transfer of electrical charges.130 The distinctive electronic structure of SACs can not only enhance the catalytic activity, but also improve the selective recognition of gas molecules. Additionally, SACs also exhibit sensitivity to oxygen molecules, rendering them ideal candidates for gas detection.
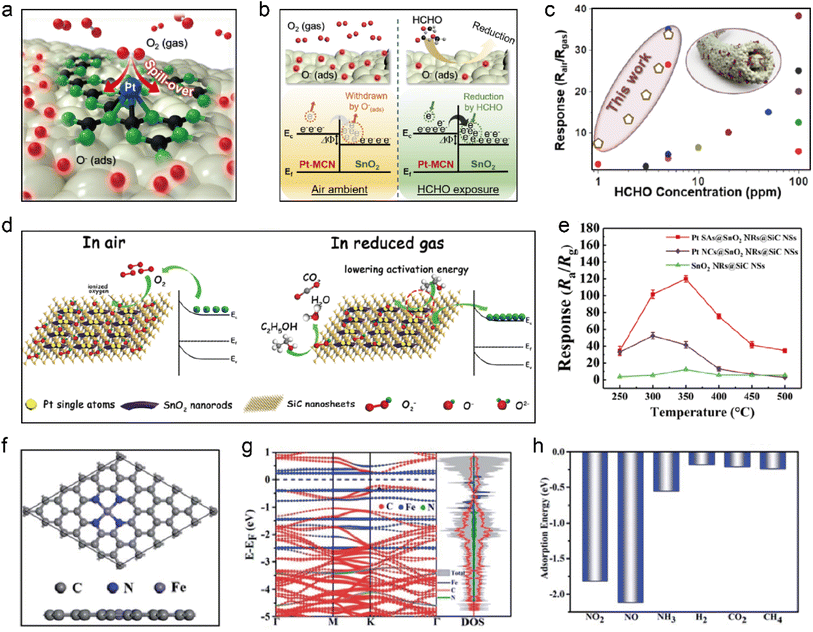 |
| Fig. 5 (a) Schematic illustration of O2(gas) dissociation over Pt SAs on Pt–MCN–SnO2 to form chemisorbed oxygen species (O(ads)−). (b) Schematic illustration showing the HCHO-sensing mechanism as well as the simplified energy band diagram of the heterojunction between MCN and SnO2. (c) Comparison of the state-of-the-art formaldehyde gas sensors with that made of Pt–MCN–SnO2. Reproduced with permission from ref. 127 Copyright 2020, American Chemical Society. (d) Schematic illustration showing the gas sensing mechanism of Pt SAs@SnO2 NRs@SiC NSs. (e) Gas sensing response toward 500 ppm ethanol versus operating temperature over Pt SAs@SnO2 NRs@SiC NSs, Pt NCs@SnO2 NRs@SiC NSs, and SnO2 NRs@SiC NSs. Reproduced with permission from ref. 128 Copyright 2020, American Chemical Society. (f) Top and side views showing the atomic configuration of FeNC in a 5 × 5 supercell. (g) Electronic band structure and density of states (DOS) for FeNC. (h) Adsorption energies of NO2, NO, NH3, H2, CO2 and CH4 on FeNC. Reproduced with permission from ref. 129 Copyright 2020, Royal Society of Chemistry. | |
3.4 Synergistic sensitization of SACs
The unique characteristics of SACs can facilitate effective interaction between gas molecules and single atomic active sites, thereby enhancing the probability of oxygen molecule adsorption and subsequent activation.131 The increased sensitivity provided by SACs allows for faster and more precise detection of trace gases, particularly important when identifying harmful pollutants or volatile organic compounds (VOCs) at low concentrations. Moreover, an excess of reactive oxygen species may accumulate on the surface of SACs, resulting in rapid oxidation and removal of the target gas from the catalyst's surface. Xue et al. achieved highly sensitive NO2 detection by dispersing Au atoms on a stepped ZnO surface (Au1/ZnO) featuring abundant unsaturated terrace defects.132 The electron transport associated with the sensing process was elucidated through theoretical studies. Fig. 6a illustrates the density of states (DOS) for ZnO, Au1–ZnO, and Au1/ZnO + NO2. Upon introducing Au SAs onto ZnO, a new band gap becomes apparent, while the band gap of NO2 widens significantly after adsorption on the ZnO surface, indicating an increase in resistance. The charge density analysis revealed that upon NO2 adsorption on Au1/ZnO, a substantial number of electrons were transferred from ZnO to NO2, consistent with the DOS analysis results. Subsequent activation and oxidation occurred when O2 was adsorbed on the Au SAs, leading to rapid detachment of NO2 from the ZnO surface. This entire process caused a significant alteration in gas sensor resistance. This example clearly demonstrates that synergistic effects between O2 adsorption at the Au SA site and NO2 adsorption on ZnO surface can enhance gas sensing performance. Furthermore, SACs based on MOS, which exhibit selective adsorption of certain gas molecules over single metal atom active sites, in contrast to vacancies on the MOS support that often generate ROS to excite and catalyze reactions, may achieve high sensing performance.133,134 For example, we demonstrated an ultra-sensitive gas sensor to detect SO2 that used atomically dispersed Ni on oxygen vacancy rich SnO2 nanorods (SAC-Ni/H-SnO2) as the sensing material.135 As shown in Fig. 6b, all tested sensors show the highest response to SO2 at 250 °C, among which, SAC-Ni/H-SnO2 (2 mol% Ni loaded H-SnO2) displays the highest gas sensitivity. Fig. 6c presents the 60 days stability test of the gas sensor. After 60 days, the SO2 response of the gas sensor made of SAC-Ni/H-SnO2, H-SnO2, SAC-Ni/SnO2 and SnO2 decreased by 4.6%, 13.4%, 15.9% and 24.5%, respectively, reflecting long-term stability of the SAC-Ni/H-SnO2 gas sensor. Based on in situ electron paramagnetic resonance (EPR) and in situ infrared (IR) spectroscopy measurements, it was revealed that the adsorbed SO2 could effectively react with the abundant superoxide free radicals on the SAC-Ni/H-SnO2 surface, resulting in a reduction of the electrical double layer thickness and sensor resistance through efficient release of trapped electrons. Hence, it can be concluded that the synergistic effect between Ni-SAC and oxygen vacancy makes SAC-Ni/H-SnO2 exhibit high sensitivity and selectivity to SO2 (Fig. 6b).
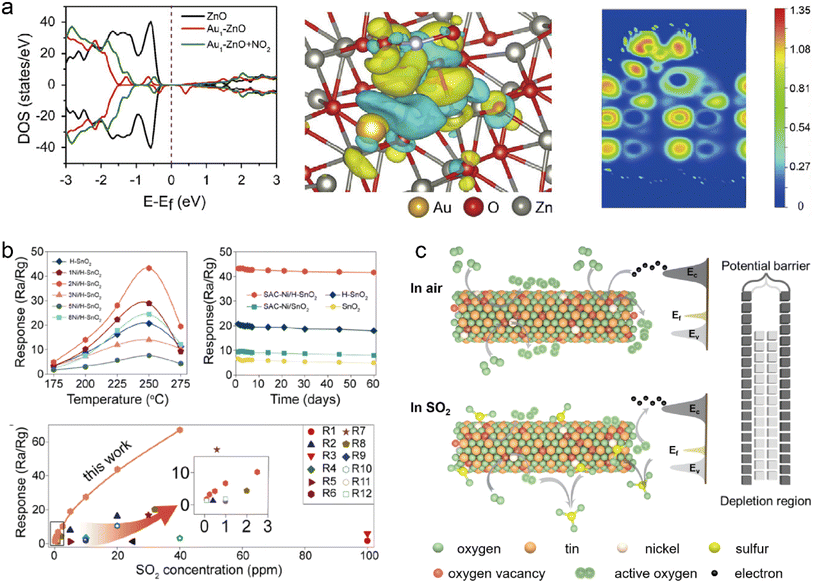 |
| Fig. 6 (a) Left: calculated DOS for ZnO, Au1–ZnO, and Au1–ZnO + NO2. Center: the charge density difference for Au1–ZnO + NO2. Right: schematic representation showing charge distribution in a two-dimensional plane. Reproduced with permission from ref. 132 Copyright 2020, Elsevier. (b) Top left: sensor's response to 20 ppm of SO2 over 0–8 mol% Ni doped SnO2 as a function of operating temperature at 40% RH. Top right: Long-term stability test at 250 °C. Bottom: comparison of state-of-the-art SO2 gas sensors with that made of SAC-Ni/H-SnO2. (c) Schematic illustration showing the gas sensing mechanism over SAC-Ni/H-SnO2. Reproduced with permission from ref. 135 Copyright 2022, Elsevier. | |
To summarize, the use of SACs can significantly enhance gas sensing performance via chemical sensitization, electronic sensitization and synergistic sensitization mechanisms, enabling gas sensors to operate at room temperature and thereby enhancing their safety, energy efficiency, and environmental friendliness.
3.5 Advantages/disadvantages of SACs for gas sensing
SACs represent a novel frontier in heterogeneous catalysis, owing to their distinctive properties and potential applications across various fields, including gas sensing. As shown in Table 1, we highlight the advantages and disadvantages of using single atom catalysts for gas sensing performance. SACs demonstrate high catalytic efficiency as each atom functions as an active site, thereby significantly enhancing the gas sensing performance. Moreover, the unique electronic and geometric structures of SACs enable exceptional selectivity towards specific gases. Additionally, SACs exhibit superior stability compared to conventional catalysts, resulting in prolonged sensor lifetimes. Furthermore, despite the potentially higher initial cost, the long-term cost-effectiveness of SACs is enhanced due to their excellent efficiency and stability. However, the preparation of SACs is complex which requires precise control over the synthesis conditions. While SACs are generally stable, they can exhibit susceptibility to aggregation under specific conditions, resulting in reduced catalytic activity and compromised gas sensing performance. The sensitivity of SACs can be influenced by various factors, such as the type of support material. The high cost associated with SACs, particularly those based on precious metals, poses a disadvantage for their widespread utilization in gas sensing applications. Additionally, limited understanding of the mechanisms underlying SACs hinders their optimization and application in the field of gas sensing.
Table 1 Advantages and disadvantages of SACs for the gas sensing performance
Advantages |
Disadvantages |
High efficiency |
Preparation complexity |
Exceptional selectivity |
Poor durability |
Superior stability |
Low sensitivity |
Cost-effective |
High cost of precious metal-based SACs |
Environmentally friendly |
Limited understanding of the SAC mechanisms |
4. Catalytic properties of SACs
With the rapid advancement of industrialization, urbanization, and global population growth, along with extensive utilization of fossil fuels and the impact of climate change, the issue of contaminated gas has become increasingly severe. Consequently, there is a growing emphasis on research aimed at discovering efficient, cost-effective and environmentally friendly technologies for mitigating contaminated gas emissions to our surrounding environment. Among the gas purification technologies that have been developed, advanced oxidation processes (AOPs) show excellent performance.27,136,137 AOPs possess the ability to break down persistent contaminants through the generation of highly reactive oxygen species (ROS), including hydroxyl radical (˙OH), sulfate radical (˙SO4−), superoxide radical (˙O2−), etc.138–140 Based on the external energy supply methods, the AOP system can be categorized into catalytic oxidation, photocatalytic oxidation, and electrocatalytic oxidation. In the following section, we will focus on the recent advancements in utilizing SACs for gas purification, elucidating the catalytic mechanisms of SACs in various AOPs, and examining the prospective opportunities and research directions for further developing SACs in the field of gas purification (Fig. 7).
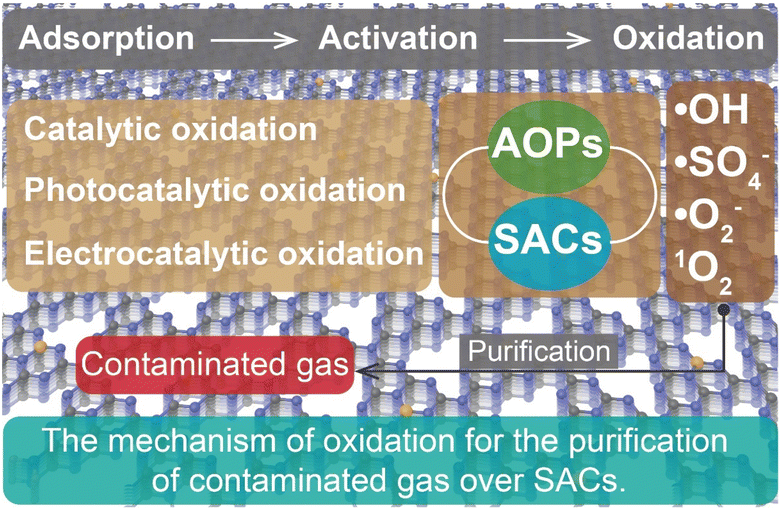 |
| Fig. 7 The catalytic mechanisms of SACs in various AOPs. | |
4.1 Catalytic oxidation reactions of contaminated gas over SACs
The oxidation reactions can effectively convert contaminated gases into non-toxic substances that are in alignment with stable elements, leading to fewer secondary pollutants compared to other treatment methods (Fig. 8a).141 However, the oxidation treatment typically requires a high reaction temperature and consumes a significant amount of energy (Fig. 8b).142,143 This dependence not only escalates the energy demand but also presents challenges in terms of equipment design and operation. To surmount these limitations, researchers have been exploring alternative approaches to mitigate the requirement for high reaction temperature while maintaining efficient catalytic oxidation. Another extensively studied strategy entails advancing catalytic reactions such as novel photocatalyses and electrocatalyses, which rely on natural sunlight or renewable electricity, rendering them clean and sustainable recycling technologies.
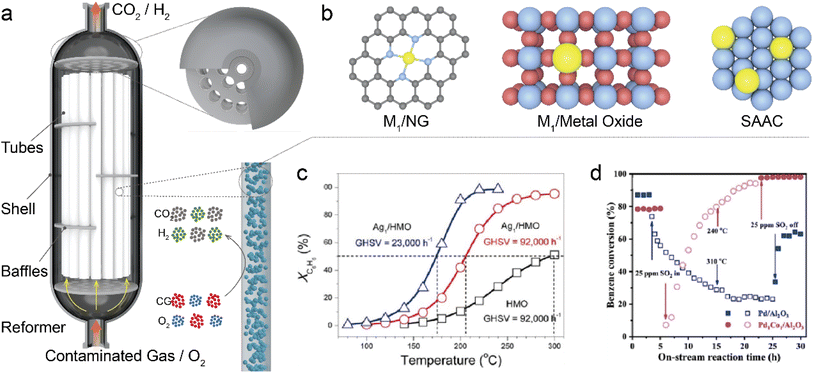 |
| Fig. 8 (a) A schematic diagram illustrating a catalytic oxidation reactor designed for purification of contaminated gas. (b) A diagram showing different types of single-atom catalysts. (c) Conversion of benzene (XC6H6) as a function of temperature over Ag1/HMO at different gas hourly space velocities (GHSVs) and XC6H6 of HMO at a GHSV of 92 000 h−1 is also given for comparison. Reaction conditions: benzene, 200 ppm; O2, 20% balanced by N2; flow rate, 100 mL min−1. Reproduced with permission from ref. 151 Copyright 2017, American Chemical Society. (d) Benzene conversion as a function of on-stream reaction time in the presence or absence of SO2 over the as-obtained samples. Reproduced with permission from ref. 158 Copyright 2021, Elsevier. | |
Recently, significant advancements have been made in the development of high-performance SACs for AOPs.144–146 These SACs demonstrate superior performance compared to nanoparticle counterparts.147–150 For instance, Ag SAs loaded on hollandite manganese oxide (Ag1/HMO) achieved complete benzene oxidation at 220 °C at a gas space velocity (GHSV) as high as 23
000 h−1 (Fig. 8c).151 The isolated Ag atoms exhibited exceptional ability in activating lattice oxygen and gaseous O2 due to their upshifted 4d orbitals. Incorporation of Ag atoms onto cryptomelane-type manganese oxide (K/Ag-OMS-40) resulted in an even higher rate of benzene conversion along with excellent stability and enhanced resistance against chlorine poisoning and moisture compared to the catalyst of 1 wt% Pd supported on Al2O3.152 This enhancement could be attributed to an increased number of Mn octahedral defects and newly formed Ag–O–Mn interaction entities that expedited charge transfer and facilitated efficient benzene conversion. The atomically dispersed noble metal atoms can also promote HCHO oxidation at low temperature. For example, introducing Au SAs onto α-MnO2 and CeO2 to form Au1/α-MnO2 and Au1/CeO2 catalysts significantly enhanced their activity and stability by facilitating the formation of oxygen vacancies, generating active oxygen species, and providing charged Au species as active sites.153,154 At 75 °C, Au1/α-MnO2 catalyst could completely decompose 500 ppm HCHO stream with a WHSV of 60 L g−1 h−1. On the other hand, among the various CeO2 morphologies tested, Au SAs on CeO2 nanorods exhibited optimal catalytic performance with achieving complete mineralization of HCHO at 85 °C. Besides Au SACs, Pt1/MnO2 catalyst could achieve full conversion of indoor-level toluene at ambient temperature thanks to the generation of surface-active oxygen species (e.g., ·OH).155 Chen et al. identified 0.47 wt% Pt1/Mn–TiO2 as an exceptional catalyst with remarkable activity for complete elimination of HCHO (100 ppm) at room temperature under the conditions of WHSV = 60 L (g h)−1 and RH = 50%.156 Furthermore, non-noble metal SACs have also been studied for contaminated gas decomposition. Through DFT calculations, Liu et al. proposed that Al SAs on graphene could be a potential catalyst for room temperature oxidation of HCHO via a pathway involving the formation of HCOOH followed by CO and subsequent CO2 release.157 The energy barriers associated with breaking C–H bonds in HCHO and C–O bonds in HCOOH were both determined to be 0.82 eV, which served as the kinetic limiting steps during the reaction process. To deeply understand the mechanism of SACs in AOPs, in situ experiments such as in situ diffuse reflectance infrared Fourier transform spectroscopy (DRIFTS) and in situ X-ray absorption spectroscopy (XAS) are unusually performed. For example, Hou et al. prepared a bimetal single-atom Pd1Co1/Al2O3 catalyst, in which the Co1 sites rapidly generated O
Co
O species for oxygen activation, while the Pd1 sites exhibited selective adsorption of benzene with excellent sulfur resistance.158 The Pd1Co1/Al2O3 catalyst thus showed favorable catalytic performance for benzene oxidation with approximately 90% conversion at 256 °C, and demonstrated gradual recovery after exposure to 25 ppm SO2 gas (Fig. 8d). Previous research has demonstrated that due to the π-bond in benzene, it adopts a parallel or planar configuration on densely packed transition metal surfaces. The presence of both Pd1 and Co1 active sites effectively hindered competitive adsorption between benzene and oxygen, thereby enhancing the reactivity. Additionally, in another study, upon introduction of SO2, the PdO–SO3 complex decomposed into PdO, reactive oxygen species (ROS), and aluminum sulfite at low temperatures.159 ROS and PdO sites continued to participate in the reaction, resulting in exceptional sulfur resistance.
4.2 Photocatalytic oxidation reactions of contaminated gas over SACs
SACs can also greatly promote photocatalytic oxidation performance.160 A typical photocatalytic system consists of two essential components: a catalytically active site and a light-harvesting unit.161 In the case of SA photocatalysts, metal SAs act as the catalytically active sites, while the support serves as the light-harvesting unit. Therefore, the interaction between metal SAs and the support in these single-atom photocatalytic systems plays a crucial role in determining charge-transfer dynamics during photocatalytic reactions, which ultimately governs the overall photocatalytic performance.162 It is also important to note that the SAs in photocatalysis may have roles beyond being solely catalytically active sites. For specific photocatalytic reactions, atomically dispersed SAs in SACs can be utilized to enhance the activity and selectivity while simultaneously improving the capacity for absorbing light and facilitating charge transfer. SACs can influence three crucial steps in a photocatalytic reaction, namely light harvesting, charge separation/transfer, and surface catalysis (Fig. 9a–c). Particularly, the presence of SAs on a semiconductor material can modify its electronic structure, thereby changing the light absorption properties of the semiconductor. For example, coordinating Co2+ atoms with g-C3N4 could extend light absorption up to 800 nm (Fig. 9d).163 The phenomenon of enhanced light absorption has been widely observed in various single-atom photocatalyst systems. For instance, Ye and colleagues demonstrated a noticeable red-shift in the light absorption spectrum of CdS by decorating CdS nanowires with Pt SAs via the Pt–S bond.164 A similar effect was also observed in MOS. Lee et al. showed that incorporating Cu SAs onto TiO2 hollow spheres led to significantly improved light absorption across all measured wavelengths (300–800 nm).165 They attributed this enhancement to the valence state change of Cu SAs, which effectively modulated the local TiO2 lattice. Despite these findings, the exact mechanism responsible for the enhanced light absorption induced by metal SAs remains a subject of debate. As a result, further investigation into the role played by metal SAs is highly warranted. The efficacy of the entire photocatalytic process heavily depends on the charge transfer dynamics between single metal atoms and their semiconductor support. The successful separation and transfer of photo-generated electrons and holes at the metal–semiconductor interface are pivotal for achieving optimal photocatalytic performance.166 When a metal contacts a semiconductor, an electronic equilibrium is established, enabling photogenerated electrons in the semiconductor to transfer via the formed Schottky junction to the supported metal SAs. This distinctive process of charge carrier separation across the metal–semiconductor interface can be fully harnessed by single-atom photocatalysts. Interestingly, reducing the size of metal nanoparticles to individual metal atoms can significantly impact their electronic structures and modify their interactions with support materials, ultimately influencing the charge transfer between light-harvesting materials and the individual atoms. For example, our research discovered that seamless integration of g-C3N4 with solitary Co2+ ions allowed for coordination of the light absorption unit with the catalytic site.163 Photogenerated holes were predominantly located at the atomically dispersed Co sites, while photogenerated electrons were primarily located at the g-C3N4 framework. As a result, when carriers migrated from g-C3N4 to atomically dispersed Co sites, it greatly suppressed carrier recombination. This led to a substantial enhancement in photocatalytic performance (Fig. 9e). Surface reactions play a crucial role in determining the photocatalytic activity and selectivity. Typically, these reactions are influenced by the geometric and electronic structure of the photocatalytic system. The adjustable surface structure of different supports provides diverse coordination environments for SAs. Consequently, it is possible to manipulate the geometric and electronic properties of single-atom photocatalysts through interactions with the support material, thereby allowing modification of the adsorption configurations of reactants, intermediates, and products as well as altering the reaction pathway.167 Compared to nanoclusters, nanoparticles, and bulk materials, SAs possess unique coordinatively unsaturated sites with distinctive electronic structures that can offer abundant active surface sites for catalyzing chemical reactions. Our research revealed that carbon nitride-supported cobalt SAs (C-C2N2) with asymmetric coordination exhibited a desired electronic configuration for single-electron transfer water oxidation reaction during photocatalysis, as demonstrated by near ambient-pressure XPS (NAP-XPS) measurements (Fig. 9f), in situ XPS analysis, and time-dependent DFT calculations.163 Consequently, this unique Co-C2N2 photocatalyst significantly enhanced the selectivity of CH4 oxidation to CH3OH under visible light illumination.
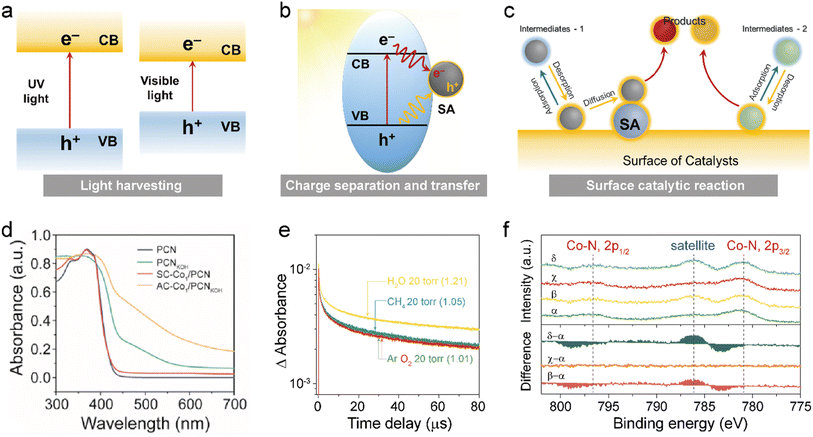 |
| Fig. 9 The role of SACs in influencing three key steps of a photocatalytic reaction, namely (a) light harvesting, (b) charge separation and transfer, and (c) surface catalytic reaction. (d) UV-vis diffuse reflection spectra of CN, PCN, Co1/CN, and Co1/PCN. (e) The comparison of in situ transient absorption decay on AC-Co1/PCNKOH at 10 000 cm−1 under Ar, CH4, O2, and H2O atmospheres (20 torr). (f) Co 2p NAP-XPS spectra of AC-Co1/PCNKOH when introducing 1 mbar of Ar (α), Ar + H2O (β), CH4 (χ), and CH4 + H2O (δ) at room temperature. Reproduced with permission from ref. 163 Copyright 2023, Elsevier. | |
Single-atom photocatalysts have demonstrated encouraging performance in oxidizing contaminated gas under light illumination.168–170 Furthermore, coupling photocatalysis with other molecules (e.g., H2O2 and PMS) to form more oxidative radicals with stronger oxidation capability is conducive to enhancing the photodegradation performance.171,172 Additionally, photo-thermocatalysis offers another powerful technique for environmental remedy.173–175
4.2.1. Photocatalysis alone.
Atomically dispersed metal sites on various semiconductors have been shown promising to promote the photocatalytic performance through tuning the band structure, and charge separation/transfer properties. Graphitic carbon nitride (GCN) is a commonly used substrate to support SAs to construct single-atom photocatalysts.176 Our research found that an antimony single-atom photocatalyst (Sb-SAPC, single Sb atoms dispersed on carbon nitride) could effectively produce H2O2 in water and ambient air under visible light irradiation, which could be used to oxidize gaseous pollutants.177 Zhang et al. dispersed single Zn atoms into the interlayer of g-C3N4 (Zn-CN-0.5) through a simple pyrolysis method.178 XAFS and DFT studies revealed that Zn-CN-0.5 possessed a coordination structure of Zn–N3 (Fig. 10a), which could enhance visible light absorption and electron–hole pair separation. As a result, Zn-CN-0.5 displayed greatly enhanced photocatalytic activity to purify NO as compared to pure GCN and Zn cluster-GCN (Fig. 10b). Besides GCN, oxides can also be used as supports to fabricate single-atom photocatalysts. Wu et al. anchored Pt single atoms onto TiO2 nanosheet-assembled hierarchical spheres (Pt1/TiO2-HS).179 The as-prepared Pt1/TiO2-HS showed excellent and stable photooxidation activity of HCHO (Fig. 10c). Chen et al. developed atomically dispersed Er on TiO2 photocatalyst (Er1–TiO2).180 Thanks to the outstanding separation of electron–hole pairs, Er1–TiO2 could degrade o-xylene and acetaldehyde with a conversion ratio of 90% and 100%, respectively under light illumination (Fig. 10d). Mori et al. prepared single-atom Eu3+ doped g-C3N4 nanosheets (Eu/g-C3N4).181 The Eu3+ ion doping not only altered the optical properties of g-C3N4, but also exhibited characteristic single-atom Eu3+ emissions due to the 5D0 → 7FJ transitions (J = 0–4). As a result, Eu/g-C3N4 showed a 12-fold activity increase in cyclohexane removal as compared to pure g-C3N4. Xia et al. reported single-atom Ag incorporated porous hollow microspheres (Ag/MnO2 PHMSs) for CH3SH removal in air.182 The Ag/MnO2 PHMSs could completely degrade 70 ppm CH3SH within 600 s under light illumination. Electron spin resonance (ESR) and scavenger experiments indicated that ·OH and 1O2 were the main reactive oxygen species engaging in methyl mercaptan degradation (Fig. 10e). The experimental results show that methyl mercaptan was decomposed into decarboxylation and sulfate ion, which finally turned into the harmless SO42−, CO2 and H2O (Fig. 10f). Besides noble metals, transition metals have also been used to fabricate single-atom photocatalysts. Hu et al. grew single Fe atoms onto TiO2 hollow microspheres to construct Fe1/TiO2-HMSs for the removal of NO.183 The Fe–O bonds and Fe–Ti bonds formed in Fe1/TiO2-HMSs ensured robust interaction between single Fe atoms and TiO2. Owing to the enhanced light adsorption, increased electron density and facilitated charge separation, Fe1/TiO2-HMSs demonstrated excellent NO removal rate under visible light irradiation with a NO removal rate as high as 47.91%, 2.3 times that of pure TiO2. Liu et al. fabricated g-C3N4 with a controlled number of carbon defects to manipulate the distribution of single-atom Pd by leveraging its affinity to nitrogen atoms resulting from carbon vacancies.184 The obtained photocatalyst exhibited exceptional and durable photocatalytic activity in NO conversion, surpassing pristine g-C3N4 by approximately 4.4 times. The improved photoactivity was attributed to the preferential separation and transport of photogenerated charge carriers due to the introduction of Pd SAs (Fig. 10g and h).
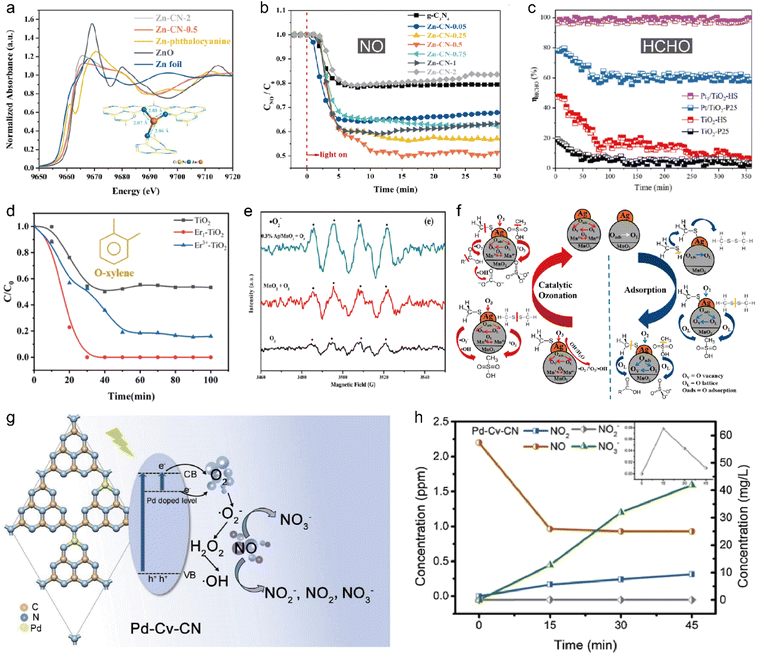 |
| Fig. 10 (a) XANES spectra of different Zn–CN samples. Inset shows the DFT-calculated Zn–CN structure. (b) NO oxidation over different Zn–CN samples. Reproduced with permission from ref. 178 Copyright 2023, Elsevier. (c) HCHO removal efficiency. Reproduced with permission from ref. 179 Copyright 2023, Elsevier. (d) The dynamic degradation curves for o-xylene. The initial concentration of o-xylene was 50 ppm. The flow rate of o-xylene and air was 20 sccm. 0.1 g photocatalysts was used. Reproduced with permission from ref. 180 Copyright 2022, Elsevier. (e) EPR signal of superoxide radical. (f) Adsorption and catalytic ozonation pathway of CH3SH over Ag/MnO2 PHMSs. Reproduced with permission from ref. 182 Copyright 2018, American Chemical Society. (g) Light-induced charge separation and the proposed mechanism for photocatalytic oxidation of NO over Pd-Cv-CN. (h) The evolution of NO and its oxidation products (NO2−, NO2, NO3−) with irradiation time over Pd-Cv-CN. All of the experiments were conducted under the same conditions: 2.2 ppm NO at a flow rate of 1.7 L min−1 was irradiated by a 300 W Xe lamp at 298 K with a relative humidity ca. 50%. Reproduced with permission from ref. 184 Copyright 2021, Elsevier. | |
4.2.2. Photo-Fenton reactions.
Photo-Fenton reaction emerges as a promising strategy to remove pollutants due to the strong oxidation power of ·OH.185,186 Wang et al. synthesized pyrrolic-type FeN4 supported on g-C3N4 for VOC removal.187 The pyrrolic-type FeN4 displayed better photo-Fenton activity than pure g-C3N4 and pyridinic-type FeN4 in a wide window of pH. Bader charge and differential charge distribution analyses disclosed that charge distribution was more favored over pyrrolic-type FeN4 (Fig. 11a). DFT calculations further confirmed that pyrrolic-type FeN4 was more stable and had higher intrinsic activity for the photo-Fenton reaction than pyridinic-type FeN4. Wu et al. chelated Fe(III) single atoms onto amidoxime with an Fe loading of 24.04 wt% (U-g-PAO/Fe), which exhibited excellent removal of H2S within 35 min via activating H2O2 under visible light.188 Furthermore, the outstanding activity could maintain over a wide pH range from 2 to 10, superior to typical Fenton catalysts. XAFS and DFT calculations disclosed that [amidoxime-Fe(OH)(H2O)3]2+ was the active site to activate H2O2 to produce ·OH (Fig. 11b). H2O2 activation was directly associated with the metal center, and thus tuning the charge state of the metal center in SACs offers a powerful strategy to improve their catalytic activity.189 Zhan et al. introduced N vacancies into a single-Fe-atom photocatalyst (Fe1-Nv/CN) to boost the activation of H2O2 (Fig. 11c).190 According to transient absorption spectroscopy measurements and DFT calculations, the N vacancies acted as the electron trap, propelling electrons to accumulate on single Fe atoms. The high electron density over the single Fe atomic sites was able to enhance H2O2 activation. As a result, the catalyst exhibited an 18-fold activity increase in degrading ciprofloxacin gas as compared to pure CN. Besides Fe, other elements were also exploited for photo-Fenton reactions. A series of M–N4 (M = Cr, Mn, Fe, Co, Cu) single-atom photocatalysts were developed for the photo-Fenton reaction,191 among which Cr–N4 is the most efficient toward removing gaseous bisphenol A under visible light irradiation in a wide pH window from 3.0 to 11.0 (Fig. 11d). The accelerated separation of photogenerated carriers and cycling of the Cr3+/Cr2+ couple could be attributed to the cooperation of photocatalysis and single atom catalysis. DFT calculations verified that Cr–N4 with a metalloporphyrin-like structure showed higher activity in decomposing H2O2 to produce ·OH. Au is another element that can trigger the photo-Fenton reaction. Han et al. found that Au@MoS2 could produce H2O2 from H2O and air under natural sunlight illumination, with H2O2 production rate (791.72 μM of H2O2 was produced in 6 hours) 2.5 times that of pure MoS2 (Fig. 11e).192 The researchers further used Mn2+ to boost H2O2 production by 2 times. These studies extended the photo-Fenton reaction to iron-free catalysts and offered new strategies to develop advanced gas purification techniques. Dual atom catalysts were also developed to further promote the photo-Fenton reaction activity. Niu et al. established an N,P-coordinated Ni, Fe dual-atom photocatalyst (CN-FeNi-P) to activate H2O2 under light irradiation.193 DFT calculations found that the single-Ni-atom center functioned as the active site to produce ·OH. The strong interaction between Fe and Ni single atoms linked with a P-bridge could efficiently decrease ·OH formation energy. Therefore, CN-FeNi-P delivered an excellent gaseous moxifloxacin removal ability, 3.7 times that of pure CN. This study introduces a dual atom photocatalyst into the photo-Fenton reaction and opens a new window for designing novel atomically dispersed photocatalysts for environmental treatment.
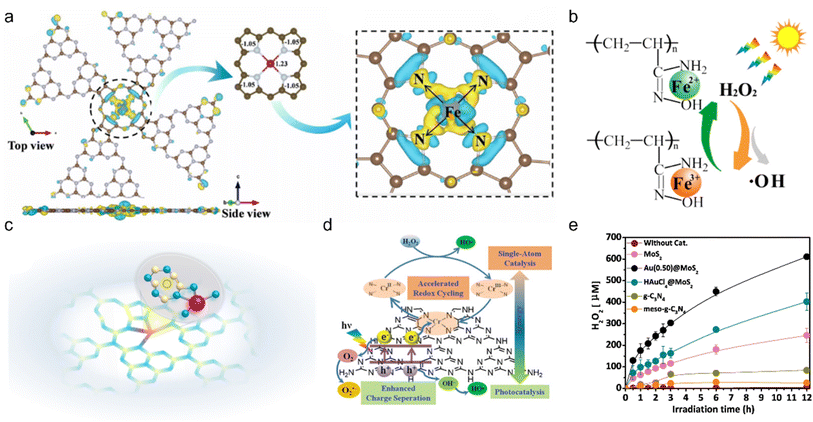 |
| Fig. 11 (a) Bader charge and differential charge distribution analyses of pyrrolic-type FeN4. Reproduced with permission from ref. 187 Copyright 2022, Elsevier. (b) Proposed photo-Fenton mechanism of U-g-PAO/Fe. Reproduced with permission from ref. 188 Copyright 2021, Elsevier. (c) Schematic model of Fe1-Nv/CN, Fe (rose red), N (blue), C (yellow) and N vacancy (black dotted circle). Reproduced with permission from ref. 190 Copyright 2021, Wiley-VCH. (d) Schematic illustration showing synergy in SA-Cr/PN-g-C3N4 + H2O2 + Vis system for producing ·OH. Reaction conditions: [BPA] = 10 mg L−1, [H2O2] = 165 μM, [Catalyst] = 0.2 g L−1, and pH = 7.0. Reproduced with permission from ref. 191 Copyright 2021, Wiley-VCH. (e) Photocatalytic H2O2 production over various catalysts. Reaction conditions: 300 W Xe lamp, 0.05 g photocatalyst, 50 mL H2O, pH = 5, 10 °C, ambient atmosphere. Reproduced with permission from ref. 192 Copyright 2019, Elsevier. | |
4.2.3. Other reactions.
Sulfite [S(IV)] activation provides another efficient method to degrade pollutants. Tong et al. fabricated single Fe atoms onto a bamboo-like porous g-C3N4 for S(IV) activation (Fig. 12).194 The catalyst with 2.5 wt‰ Fe displayed 16 times higher S(IV) activation activity and diclofenac removal rate compared to pure g-C3N4 under visible light irradiation. The much-enhanced performance was attributed to the formation of an Fe–S(IV) complex. Detailed experiments revealed that the diclofenac removal process involved decarboxylation, hydroxylation, chlorine abstraction and cleavage at the bridging N atoms, consistent with the Fukui index prediction.
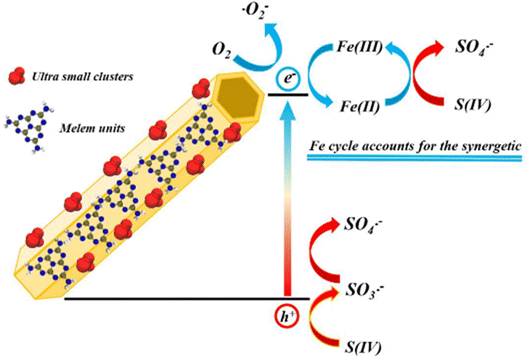 |
| Fig. 12 Schematic illustration showing the mechanisms for S(IV) activation by MF2 under visible light irradiation. Reproduced with permission from ref. 194 Copyright 2021, Elsevier. | |
4.3 Electrocatalytic reactions
SAC-based electrocatalysis has shown powerful oxidation capability and has been extensively used for environmental treatment. Electrocatalysis alone can remove contaminated gas and deliver good performance. Integrating electrocatalysis with the PMS system or applying photoelectrocatalysis demonstrates stronger oxidation ability and thus achieves better contaminated gas removal performance.195
4.3.1. Electrocatalysis alone.
Electrochemical reduction or oxidation works as a promising approach to remove contaminated gas due to the facile operation conditions and high efficiency.196 Recently, electrocatalysis has garnered unprecedented attention in nitrogen-containing molecule upgradation, which enables conversion of nitrogen-containing waste into a wide range of high value-added chemicals under mild operating conditions, including NH3, NH2OH, N2H4, urea, and cyclohexanone oxime.197–200 It represents an exemplary technical solution for transforming waste into valuable resources (Fig. 13a), which has attracted significant interest in the scientific community.201Fig. 13b shows the relationship between the calculated ΔG for NO adsorption on transition metal (TM)-N4/graphene and the d-band center of the central TM, demonstrating a clear linear correlation.202 For instance, when Fe, Co, and Cu atoms are individually incorporated into graphene, their computed d-band centers are approximately −2.97 eV, −3.19 eV, and −3.52 eV respectively. These values align well with their corresponding NO adsorption strengths (ΔG = −1.27 eV, −0.77 eV, and +0.06 eV). Therefore, it can be inferred that variations in ΔG values among different TM-N4/graphene directly correspond to shifts in the position of the d-band center.203,204 The moderate d-band center observed in Co-N4/graphene results in a balanced interaction between its surface and adsorbed NO molecules, which accounts for enhanced kinetics in the NO reduction reaction (NORR) on Co-N4/graphene. Chu et al. anchored single W atoms onto MoO3 nanosheets (W1/MoO3−x) and studied its application in NO removal.205 It was found that W1/MoO3−x not only delivered super performance towards NH3 generation, but also displayed enhanced H2O dissociation while suppressing *H dimerization to increase proton supply. In contrast to the weakly adsorbed NO on pristine MoO3, the OV-induced coordinatively unsaturated motifs of Mo1–O5 (in MoO3−x) and W1–O5 (in W1/MoO3−x) exhibited remarkable NO adsorption capability with efficient dissociation of the N
O bond.206 As a result, W1/MoO3−x exhibited outstanding catalytic performance in the NORR. Besides W, In SAs have also been extensively investigated for the NORR. As reported by Chu and co-workers, the In SAs with an In1–O5 motif confined in amorphous MoO3 achieved the highest NO reduction to ammonia faradaic efficiency (FE) of 85% with an NH3 yield rate of 0.29 mmol h−1 cm−2.207 The N–N coupling was effectively prevented over the as-developed In SAC due to lack of continuous active sites, which suppressed N2 production and improved ammonia selectivity. Li et al. reported that a NO-preoccupied transition state would occur on the single-Fe-atom site, which would limit water adsorption and the HER, leading to an outstanding nitrate reduction to ammonia performance with an ammonia yield rate of 30 mol h−1 gFe−1 and an ultrahigh ammonia FE of 100% (Fig. 13c–d).208 Besides, Cu single atoms were also scrutinized. DFT calculations showed a noticeable electronic interaction in the Cu–S3 motif having −0.24|e| electron transfer from the Cu single atom to the surrounding S atoms (Fig. 13e).209 As a result, CuS3 could produce ammonia at a rate of 0.34 mmol h−1 cm−2 in the NORR (Fig. 13f). Additionally, dual-atom catalysts (e.g., CuFe DS/NC) also exhibited remarkable efficacy in NO reduction to produce NH3 (Fig. 13g).201
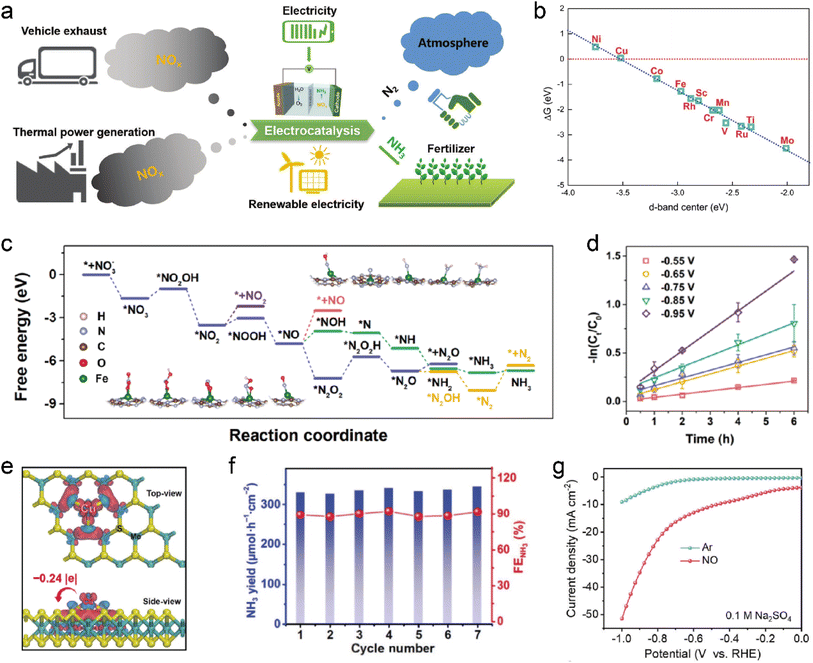 |
| Fig. 13 (a) Schematic diagram of the electrocatalytic reduction of NO to NH3. Reproduced with permission from ref. 201 Copyright 2023, Wiley-VCH. (b) Calculated NO adsorption free energy (ΔG) on TM-N4/graphene versus the d-band center of the central TM atom. Reproduced with permission from ref. 202 Copyright 2018, Royal Society of Chemistry. (c) Free energy diagram of electrochemical NO3− reduction on Fe1/g-C3N4. (d) Kinetic linear fitting for NO3-N removal at different voltages. Reproduced with permission from ref. 208 Copyright 2022, Elsevier. (e) Charge density difference of the Cu1–S3 motif on MoS2. Red and blue denote charge accumulation and depletion regions, respectively. (f) Cycling test of Cu1/MoS2 performed at −0.6 V vs. RHE. Reproduced with permission from ref. 209 Copyright 2022, Springer. (g) LSV curves of CuFe DS/NC measured in Ar and NO-saturated 0.1 M Na2SO4 in an H-type cell. Reproduced with permission from ref. 201 Copyright 2023, Wiley-VCH. | |
4.3.2. Electrochemical activation of peroxymonosulfate (electro-PMS).
Electrochemical activation of peroxymonosulfate (electro-PMS) emerges as a promising method for environmental remediation because ·SO4− has a stronger oxidation ability and a longer half-life (2.5–3.1 V vs. NHE, 30–40 μs) than ·OH (1.8–2.7 V vs. NHE, 20 ns),210,211 which can be operated in a wide pH window from 2 to 8. Liu et al. deposited Fe SAs onto Mo2TiC2Tx MXene with abundant Mo vacancies (Fe-SA/Mo2TiC2Tx) for PMS activation (Fig. 14a).212 The Mo vacancies not only provided sites to anchor Fe atoms via forming Fe–O bonds, but also served as the co-catalyst to prompt a Fenton-like reaction. The Fe-SA/Mo2TiC2Tx catalyst could completely remove gaseous sulfamethoxazole (SMX) in a single-pass mode. It is worth mentioning that the SMX degradation activity showed a 24-fold and 67-fold increase over Fe-SA/Mo2TiC2Tx as compared to that over Fe-NP/Mo2TiC2Tx and pure Mo2TiC2Tx. Experimental studies and DFT calculations revealed that the single-atom Fe sites were responsible for the adsorption and activation of PMS to generate reactive SO4·− under an electric field. Liu et al. fabricated Cu-SA/Ti3C2Tx with a Cu–O3 coordination for PMS activation to remove SMX (Fig. 14b).213 It was found that 1O2 was the main active species generated over Cu-SA/Ti3C2Tx, while ·OH was the dominant species generated over Cu-NP/Ti3C2Tx, responsible for SMX degradation, indicating the importance of size effect in PMS activation.
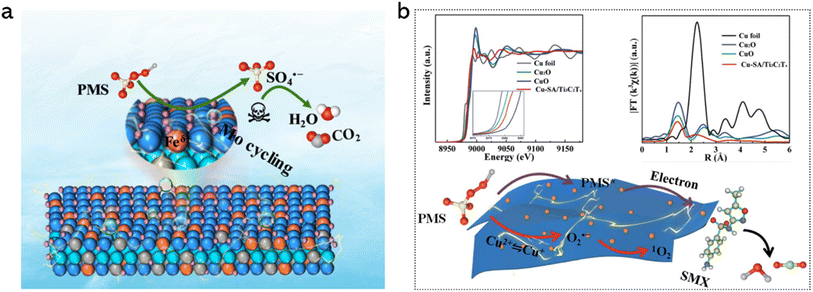 |
| Fig. 14 (a) Proposed mechanism of electro-PMS activation for SMX degradation over Fe-SA/Mo2TiC2Tx. Reproduced with permission from ref. 212 Copyright 2022, American Chemical Society. (b) Top left: XANES spectra at the Cu K-edge. Top right: the k2-weighted Fourier transformed EXAFS spectra, where χ(k) denotes the EXAFS oscillation function. Bottom: schematic illustration showing the proposed electro-PMS activation mechanism on the Cu-SA/Ti3C2Tx filter. Reproduced with permission from ref. 213 Copyright 2021, Royal Society of Chemistry. | |
4.3.3. Photoelectrocatalysis (PEC).
PEC provides another powerful method for addressing environmental issues.214–216 Zhao et al. used Cu-SA/TiO2−x to selectively reduce O2 to H2O2 in a wide pH range (7–11).217 The H2O2 concentration could reach 276.23 μmol L−1 at pH = 9 (Fig. 15a), and 96.3% of sulfamethoxazole could be degraded over Cu-SA/TiO2−x within 10 min. The catalyst also demonstrated excellent performance in five other wastewater samples, indicating the wide application potential of the developed catalyst for wastewater treatment. Zhao et al. deposited Pd SAs onto F–TiO2 with a Pd–F4O2 coordination to selectively reduce O2 to H2O2 with a selectivity up to 99% (Fig. 15b).218 Note that an additional channel bond HO–O⋯Pd–F-TiO2 propelled electron migration from the conduction band of F-TiO2 to Pd SAs to reduce Pd⋯O–OH to ·OH. The ·OH generation rate could reach 9.18 μmol L−1 min−1, one order of magnitude higher than that in conventional advanced oxidation processes reported in the literature. As a result, the kinetics of BPA and acetaminophen degradation reached as high as 0.324 min−1 and 0.175 min−1, respectively, and 93.2% of total organic carbon and 99.3% of toxicity could be removed in 60 min. Through PEC, organic pollutants can even be upgraded to value-added products. Qu et al. developed single Ru atoms on Cu2O to degrade organics,219 which achieved almost complete removal of BPA (99.6%). More importantly, NH3 could be produced concurrently (yield: ∼37.4 μg h−1 mgcat−1, FE: ∼17.1%) at low voltages. The whole system could decrease energy consumption by ∼51.8% compared to the nitrogen reduction reaction (NRR) process.
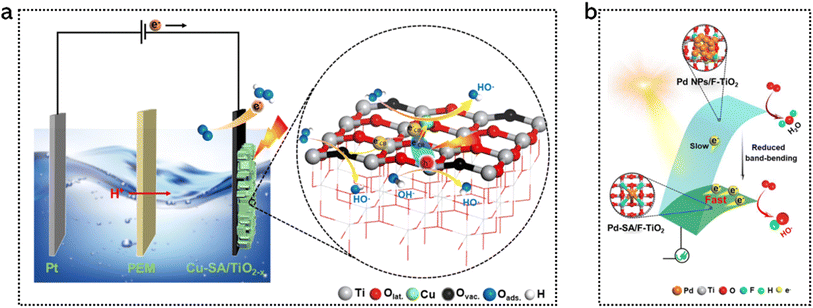 |
| Fig. 15 (a) Schematic diagram showing photoelectrocatalytic oxygen reduction on the Cu0.46-SA/TiO2−x photocathode in an alkaline solution. Reproduced with permission from ref. 217 Copyright 2022, American Chemical Society. (b) Mechanism for photoelectrocatalytic O2 reduction to HO· over Pd-SA/F-TiO2 and Pd NPs/F-TiO2. Reproduced with permission from ref. 218 Copyright 2022, American Chemical Society. | |
5. Summary and outlook
In this review, we first provided a concise introduction to the significance and urgency of gas detection and pollutant purification, followed by a comprehensive overview of the structural feature identification methods for SACs. Subsequently, the recent advances of SACs used in environmental detection and remediation were systematically elaborated. Although great efforts have been made in developing SACs for environmental applications, many issues still remain. Next, we are going to propose some solutions to address the current challenges and expedite the development of SACs in pollution gas detection and purification (Fig. 16). The development of advanced synthesis techniques enables more precise control over the size, shape, and distribution of individual atoms on the support material, thereby facilitating optimization of catalytic activity and selectivity in SACs. The utilization of model small molecules for immobilization aids in the creation of model SAC systems, which can offer valuable insights into the structure and behavior of SACs, aid in design optimization and performance prediction for gas sensing applications. In SACs, equal attention should be given to understanding the specific role played by the support materials; however, this aspect is often overlooked in current research. Future investigations shall focus on comprehending how support materials influence monatomic behavior and exploring modifications that can enhance SAC performance. Furthermore, machine learning and artificial intelligence can be employed to predict the structure, composition, and performance of SACs. A comprehensive study on the response pathway of SACs can provide significant insights into their sensing mechanisms, enabling the design of highly selective and sensitive SACs. Additionally, developing environmentally friendly SACs using non-toxic elements represents an important strategy contributing to sustainable development. These strategies have immense potential to revolutionize the field of SACs while opening up new opportunities for their applications in gas sensing and other domains.
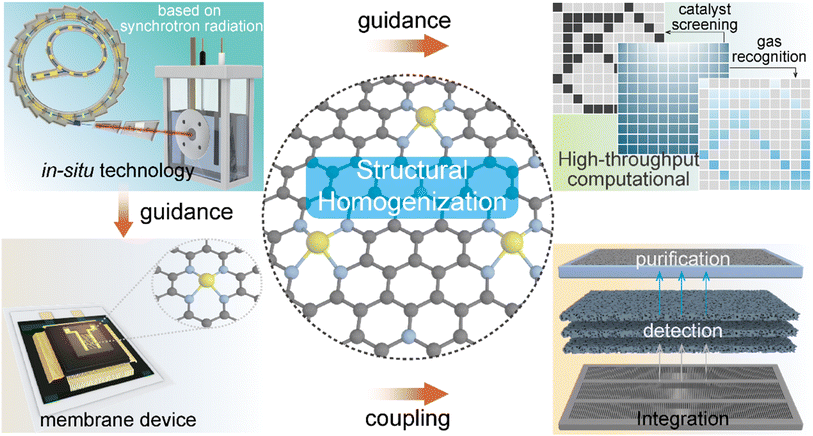 |
| Fig. 16 The outlook diagram highlighting the future research directions. | |
(1) Structural homogenization for SACs: most of the SACs are prepared via high-temperature pyrolysis, consequently, it is difficult to construct SACs with a precise coordination environment. Nowadays, only EXAFS measurement can be utilized to probe the local structure of SACs. However, EXAFS can only provide the average structure rather than the local structure information. Furthermore, EXAFS cannot distinguish M–N, M–C and M–O bonds due to the similar atomic weight of N, C and O atoms. Even if the coordination numbers are the same, the moieties can be located on the plane, at the edge, or coordinated with another vacancy. The above-mentioned factors lead to the controversy over the coordination structure of SACs. Without the precise local structure, the subsequent mechanism exploration and explanation based on the electronic states of the single metal atoms will become less meaningful. Synthesizing SACs at low temperature or even at room temperature may provide a guarantee for the development of SACs with a uniform structure.
(2) Novel SAC design: although SACs have been extensively used for environmental application, the working mechanism of SACs is still unclear because of the complex multiple electron-transfer steps. Determining the underlying working principle that governs the performance in environmental remediation is conducive to the rapid exploitation of novel efficient SACs. Employing in situ techniques, such as in situ XAS and in situ XPS, serves as a promising route to probe the structural evolution and the reaction pathway under working conditions.38,220 Also, designing dual atom catalysts based on the understanding of SACs is beneficial to further improve the catalyst's activity and stability.221
(3) High-throughput computational screening of SACs: the conventional catalyst development process heavily relying on experiments cannot meet the industrial requirements. Using DFT predictions and deploying machine learning methods for high-throughput screening can speed up catalyst design and development process to obtain promising self-powered sensing platforms for future hyperscaling of the Internet of Things systems.222 Moreover, SACs are now utilized to remove pollutants in the lab scale; scale-up experiments in a real environment, such as rivers or seas, are highly needed.
(4) Membrane device based on SACs: SACs are typically prepared in the powder form before being deposited onto a membrane for gas detection or pollutant degradation purposes. However, there exists a significant disparity between the activity of SACs and the performance in real devices incorporating SACs, resulting in unsatisfactory practical applications. Therefore, directly constructing SACs onto a membrane as a monolithic catalyst can ensure the high activity of SACs and propel the commercialization of SAC-based gas sensors and other related products.
(5) Integration of detection and purification of contaminated gas: at the current stage of study, environmental detection and remediation are conducted as separate processes. Considering the exceptional monitoring and remediation capabilities of SACs, integrating them into a single device with both detection and remediation functionalities will become a focal point for future research.
Author contributions
All of the authors contributed to the literature search, writing and editing of this review.
Conflicts of interest
There are no conflicts to declare.
Acknowledgements
This work was supported by the City University of Hong Kong startup fund (9020003), ITF – RTH – Global STEM Professorship (9446006), the ECF 88/2021 and PolyU Project of Strategic Importance (1-ZE2W) for Funding, the National Natural Science Foundation of China (No. 21976086, Grant No. 22075195), and the National Key Research and Development Program of China (No. 2019YFC1804201).
References
- D. J. Wales, J. Grand, V. P. Ting, R. D. Burke, K. J. Edler, C. R. Bowen, S. Mintova and A. D. Burrows, Gas sensing using porous materials for automotive applications, Chem. Soc. Rev., 2015, 44, 4290–4321 RSC.
- J. Liu, L. Zhang, J. Fan and J. Yu, Semiconductor gas sensor for triethylamine detection, Small, 2022, 18, 2104984 CrossRef CAS PubMed.
- L. J. Carter, Uncontrolled SO2 emissions bring acid rain, Science, 1979, 204, 1179 CrossRef CAS PubMed.
-
M. Cipolla, M. Sorgenti, C. Gentile and M. M. Bishara, in Clinical Handbook of Air Pollution-Related Diseases, ed. F. Capello and A. V. Gaddi, Springer International Publishing, Cham, 2018, pp. 327–339, DOI:10.1007/978-3-319-62731-1_18.
- T. Becker, S. Mühlberger, C. B.-v. Braunmühl, G. Müller, T. Ziemann and K. V. Hechtenberg, Air pollution monitoring using tin-oxide-based microreactor systems, Sens. Actuators, B, 2000, 69, 108–119 CrossRef CAS.
- A. Wang, J. Li and T. Zhang, Heterogeneous single-atom catalysis, Nat. Rev. Chem, 2018, 2, 65–81 CrossRef CAS.
- X. Li, H. Rong, J. Zhang, D. Wang and Y. Li, Modulating the local coordination environment of single-atom catalysts for enhanced catalytic performance, Nano Res., 2020, 13, 1842–1855 CrossRef CAS.
- X. N. Li, Y. Q. Huang and B. Liu, Catalyst: single-atom catalysis: directing the way toward the nature of catalysis, Chem, 2019, 5, 2733–2735 CAS.
-
J.-X. Liang, Y.-G. Wang, X.-F. Yang, D.-H. Xing, A.-Q. Wang, T. Zhang and J. Li, in Encyclopedia of Inorganic and Bioinorganic Chemistry, 2017, pp. 1–11 Search PubMed.
- B. Lu, Q. Liu and S. Chen, Electrocatalysis of single-atom sites: Impacts of atomic coordination, ACS Catal., 2020, 10, 7584–7618 CrossRef CAS.
- B. Han, Y. Guo, Y. Huang, W. Xi, J. Xu, J. Luo, H. Qi, Y. Ren, X. Liu, B. Qiao and T. Zhang, Strong metal-support interactions between Pt single atoms and TiO2, Angew. Chem., Int. Ed., 2020, 59, 11824–11829 CrossRef CAS PubMed.
- X.-F. Yang, A. Wang, B. Qiao, J. Li, J. Liu and T. Zhang, Single-atom catalysts: A new frontier in heterogeneous catalysis, Acc. Chem. Res., 2013, 46, 1740–1748 CrossRef CAS PubMed.
- H. B. Yang, S.-F. Hung, S. Liu, K. Yuan, S. Miao, L. Zhang, X. Huang, H.-Y. Wang, W. Cai, R. Chen, J. Gao, X. Yang, W. Chen, Y. Huang, H. M. Chen, C. M. Li, T. Zhang and B. Liu, Atomically dispersed Ni(i) as the active site for electrochemical CO2 reduction, Nat. Energy, 2018, 3, 140–147 CrossRef CAS.
- X. Liang, N. Fu, S. Yao, Z. Li and Y. Li, The progress and outlook of metal single-atom-site catalysis, J. Am. Chem. Soc., 2022, 144, 18155–18174 CrossRef CAS PubMed.
- J. Ding, Z. Wei, F. Li, J. Zhang, Q. Zhang, J. Zhou, W. Wang, Y. Liu, Z. Zhang, X. Su, R. Yang, W. Liu, C. Su, H. B. Yang, Y. Huang, Y. Zhai and B. Liu, Atomic high-spin cobalt(II) center for highly selective electrochemical CO reduction to CH3OH, Nat. Commun., 2023, 14, 6550 CrossRef CAS PubMed.
- X. Li, X. Yang, Y. Huang, T. Zhang and B. Liu, Supported noble-metal single atoms for heterogeneous catalysis, Adv. Mater., 2019, 31, 1902031 CrossRef CAS PubMed.
- G. Lei, H. Pan, H. Mei, X. Liu, G. Lu, C. Lou, Z. Li and J. Zhang, Emerging single atom catalysts in gas sensors, Chem. Soc. Rev., 2022, 51, 7260–7280 RSC.
- M. Zhang, G. Wang, J. Chen and X. Lu, Single atom catalysts for sensors, Trends Anal. Chem., 2023, 169, 117399 CrossRef CAS.
- Z. Li, E. Tian, S. Wang, M. Ye, S. Li, Z. Wang, Z. Ma, G. Jiang, C. Tang, K. Liu and J. Jiang, Single-atom catalysts: promotors of highly sensitive and selective sensors, Chem. Soc. Rev., 2023, 52, 5088–5134 RSC.
- J. Zeng, Q. Rong, B. Xiao, R. Yu, B. Zi, X. Kuang, X. Deng, Y. Ma, J. Zhang, J. Wu and Q. Liu, Single-atom silver loaded on tungsten oxide with oxygen vacancies for high performance triethylamine gas sensors, J. Mater. Chem. A, 2021, 9, 8704 RSC.
- J. Qiu, X. Hu, L. Shi, J. Fan, X. Min, W. Zhang and J. Wang, Enabling selective, room-temperature gas detection using atomically dispersed Zn, Sens. Actuators, B, 2021, 329, 129221 CrossRef CAS.
- R. Tian, P. Ji, Z. Luo, J. Li and J. Sun, Room-temperature NH3 gas sensor based on atomically dispersed Co with a simple structure, New J. Chem., 2021, 45, 10240–10247 RSC.
- T. Chu, C. Rong, L. Zhou, X. Mao, B. Zhang and F. Xuan, Progress and perspectives of single-atom catalysts for gas sensing, Adv. Mater., 2023, 35, e2206783 CrossRef PubMed.
- M. Wang, M. Ye, J. Wang, Y. Xu, Z. Wang, X. Tong, X. Han, K. Zhang, W. Wang, K. Wu and X. Wei, Recent advances and applications of single atom catalysts based electrochemical sensors, Nano Res., 2024, 17, 2993–3013 Search PubMed.
- F. Gu, C. Luo, D. Han, S. Hong and Z. Wang, Atomically dispersed Pt on 3DOM WO3 promoted with cobalt and nickel oxides for highly selective and highly sensitive detection of xylene, Sens. Actuators, B, 2019, 297, 126772 CrossRef CAS.
- T. Cai, Z. Teng, Y. Wen, H. Zhang, S. Wang, X. Fu, L. Song, M. Li, J. Lv and Q. Zeng, Single-atom site catalysts for environmental remediation: Recent advances, J. Hazard. Mater., 2022, 440, 129772 CrossRef CAS PubMed.
- Y. Shang, X. Xu, B. Gao, S. Wang and X. Duan, Single-atom catalysis in advanced oxidation processes for environmental remediation, Chem. Soc. Rev., 2021, 50, 5281–5322 RSC.
- L. Zhang, Y. Ren, W. Liu, A. Wang and T. Zhang, Single-atom catalyst: a rising star for green synthesis of fine chemicals, Natl. Sci. Rev., 2018, 5, 653–672 CrossRef CAS.
- R. Xie, K. Guo, Z. Ao, Z. Suo, H. Huang and D. Y. C. Leung, Surface-bound radicals generated from cobalt single-atom catalyst: Mechanism of boosting Fenton-like reactions, Chem. Eng. J., 2023, 461, 141920 CrossRef CAS.
- Q. Li, Z. Li, Q. Zhang, L. Zheng, W. Yan, X. Liang, L. Gu, C. Chen, D. Wang, Q. Peng and Y. Li, Porous γ-Fe2O3 nanoparticle decorated with atomically dispersed platinum: study on atomic site structural change and gas sensor activity evolution, Nano Res., 2020, 14, 1435–1442 CrossRef.
- S. Lv, H. Wang, Y. Zhou, D. Tang and S. Bi, Recent advances in heterogeneous single-atom nanomaterials: From engineered metal-support interaction to applications in sensors, Coord. Chem. Rev., 2023, 478, 214976 CrossRef CAS.
- J. Ding, J. Huang, Q. Zhang, Z. Wei, Q. He, Z. Chen, Y. Liu, X. Su and Y. Zhai, A hierarchical monolithic cobalt-single-atom electrode for efficient hydrogen peroxide production in acid, Catal. Sci. Technol., 2022, 12, 2416–2419 RSC.
- J. Gao, H. b. Yang, X. Huang, S.-F. Hung, W. Cai, C. Jia, S. Miao, H. M. Chen, X. Yang, Y. Huang, T. Zhang and B. Liu, Enabling direct H2O2 production in acidic media through rational design of transition metal single atom catalyst, Chem, 2020, 6, 658–674 CAS.
- S. Liu, H. B. Yang, S. F. Hung, J. Ding, W. Cai, L. Liu, J. Gao, X. Li, X. Ren, Z. Kuang, Y. Huang, T. Zhang and B. Liu, Elucidating the electrocatalytic CO2 reduction reaction over a model single-atom nickel catalyst, Angew. Chem., Int. Ed., 2020, 59, 798–803 CrossRef CAS PubMed.
- W. Liu, L. Zhang, X. Liu, X. Liu, X. Yang, S. Miao, W. Wang, A. Wang and T. Zhang, Discriminating catalytically active FeNx species of atomically dispersed Fe–N–C catalyst for selective oxidation of the C–H bond, J. Am. Chem. Soc., 2017, 139, 10790–10798 CrossRef CAS PubMed.
- F. Chen, X. Jiang, L. Zhang, R. Lang and B. Qiao, Single-atom catalysis: Bridging the homo- and heterogeneous catalysis, Chin. J. Catal., 2018, 39, 893–898 CrossRef CAS.
- N. Cheng, L. Zhang, K. Doyle-Davis and X. Sun, Single-atom catalysts: From design to application, Electrochem. Energy Rev., 2019, 2, 539–573 CrossRef.
- X. Li, X. Yang, J. Zhang, Y. Huang and B. Liu,
In situ/operando techniques for characterization of single-atom catalysts, ACS Catal., 2019, 9, 2521–2531 CrossRef CAS.
- Y. Liu, J. Ding, F. Li, X. Su, Q. Zhang, G. Guan, F. Hu, J. Zhang, Q. Wang, Y. Jiang, B. Liu and H. B. Yang, Modulating hydrogen adsorption via charge transfer at the semiconductor–metal heterointerface for highly efficient hydrogen evolution catalysis, Adv. Mater., 2022, 35, 2207114 CrossRef PubMed.
- Y. Liu, Q. Wang, J. Zhang, J. Ding, Y. Cheng, T. Wang, J. Li, F. Hu, H. B. Yang and B. Liu, Recent advances in carbon-supported noble-metal electrocatalysts for hydrogen evolution reaction: syntheses, structures, and properties, Adv. Energy Mater., 2022, 12, 2200928 CrossRef CAS.
- J. Yang, H. Qi, A. Li, X. Liu, X. Yang, S. Zhang, Q. Zhao, Q. Jiang, Y. Su, L. Zhang, J. F. Li, Z. Q. Tian, W. Liu, A. Wang and T. Zhang, Potential-driven restructuring of Cu single atoms to nanoparticles for boosting the electrochemical reduction of nitrate to ammonia, J. Am. Chem. Soc., 2022, 144, 12062–12071 CrossRef CAS PubMed.
- R. Qin, K. Liu, Q. Wu and N. Zheng, Surface coordination chemistry of atomically dispersed metal catalysts, Chem. Rev., 2020, 120, 11810–11899 CrossRef CAS PubMed.
- J. Yang, W. Liu, M. Xu, X. Liu, H. Qi, L. Zhang, X. Yang, S. Niu, D. Zhou, Y. Liu, Y. Su, J. F. Li, Z. Q. Tian, W. Zhou, A. Wang and T. Zhang, Dynamic behavior of single-atom catalysts in electrocatalysis: identification of Cu-N3 as an active site for the oxygen reduction reaction, J. Am. Chem. Soc., 2021, 143, 14530–14539 CrossRef CAS PubMed.
- Y. Zeng, J. Zhao, S. Wang, X. Ren, Y. Tan, Y. R. Lu, S. Xi, J. Wang, F. Jaouen, X. Li, Y. Huang, T. Zhang and B. Liu, Unraveling the electronic structure and dynamics of the atomically dispersed iron sites in electrochemical CO2 reduction, J. Am. Chem. Soc., 2023, 145, 15600–15610 CrossRef CAS PubMed.
- H. Qi, J. Yang, F. Liu, L. Zhang, J. Yang, X. Liu, L. Li, Y. Su, Y. Liu, R. Hao, A. Wang and T. Zhang, Highly selective and robust single-atom catalyst Ru1/NC for reductive amination of aldehydes/ketones, Nat. Commun., 2021, 12, 3295 CrossRef CAS PubMed.
- X. Ren, S. Liu, H. Li, J. Ding, L. Liu, Z. Kuang, L. Li, H. Yang, F. Bai, Y. Huang, T. Zhang and B. Liu, Electron-withdrawing functional ligand promotes CO2 reduction catalysis in single atom catalyst, Sci. China Chem., 2020, 63, 1727–1733 CrossRef CAS.
- Y. J. Ren, Y. Tang, L. L. Zhang, X. Y. Liu, L. Li, S. Miao, D. S. Su, A. Q. Wang, J. Li and T. Zhang, Unraveling the coordination structure-performance relationship in Pt1/Fe2O3 single-atom catalyst, Nat. Commun., 2019, 10, 4500 CrossRef PubMed.
- X. Z. Shao, X. F. Yang, J. M. Xu, S. Liu, S. Miao, X. Y. Liu, X. Su, H. M. Duan, Y. Q. Huang and T. Zhang, Iridium single-atom catalyst performing a quasi-homogeneous hydrogenation transformation of CO2 to formate, Chem, 2019, 5, 693–705 CAS.
- B. T. Qiao, A. Q. Wang, X. F. Yang, L. F. Allard, Z. Jiang, Y. T. Cui, J. Y. Liu, J. Li and T. Zhang, Single-atom catalysis of CO oxidation using Pt1/FeOx, Nat. Chem., 2011, 3, 634–641 CrossRef CAS PubMed.
- R. Lang, T. Li, D. Matsumura, S. Miao, Y. Ren, Y.-T. Cui, Y. Tan, B. Qiao, L. Li, A. Wang, X. Wang and T. Zhang, Hydroformylation of olefins by a rhodium single-atom catalyst with activity comparable to RhCl(PPh3)3, Angew. Chem., Int. Ed., 2016, 55, 16054–16058 CrossRef CAS PubMed.
- X. Su, X.-F. Yang, Y. Huang, B. Liu and T. Zhang, Single-atom catalysis toward efficient CO2 conversion to CO and formate products, Acc. Chem. Res., 2019, 52, 656–664 CrossRef CAS PubMed.
- Z. Chen, H. Niu, J. Ding, H. Liu, P. H. Chen, Y. H. Lu, Y. R. Lu, W. Zuo, L. Han, Y. Guo, S. F. Hung and Y. Zhai, Unraveling the origin of sulfur-doped Fe-N-C single-atom
catalyst for enhanced oxygen reduction activity: effect of iron spin-state tuning, Angew. Chem., Int. Ed., 2021, 60, 25404–25410 CrossRef CAS PubMed.
- L. Liu, T. Xiao, H. Fu, Z. Chen, X. Qu and S. Zheng, Construction and identification of highly active single-atom Fe1-NC catalytic site for electrocatalytic nitrate reduction, Appl. Catal., B, 2023, 323, 122181 CrossRef CAS.
- L. Fang, S. Seifert, R. E. Winans and T. Li,
Operando XAS/SAXS: Guiding design of single-atom and subnanocluster catalysts, Small Methods, 2021, 5, 2001194 CrossRef CAS PubMed.
- S. Qiao, Q. He, P. Zhang, Y. Zhou, S. Chen, L. Song and S. Wei, Synchrotron-radiation spectroscopic identification towards diverse local environments of single-atom catalysts, J. Mater. Chem. A, 2022, 10, 5771–5791 RSC.
- X. Li, C.-S. Cao, S.-F. Hung, Y.-R. Lu, W. Cai, A. I. Rykov, S. Miao, S. Xi, H. Yang, Z. Hu, J. Wang, J. Zhao, E. E. Alp, W. Xu, T.-S. Chan, H. Chen, Q. Xiong, H. Xiao, Y. Huang, J. Li, T. Zhang and B. Liu, Identification of the electronic and structural dynamics of catalytic centers in single-Fe-atom material, Chem, 2020, 6, 3440–3454 CAS.
- S. Das and V. Jayaraman, SnO2: A comprehensive review on structures and gas sensors, Prog. Mater. Sci., 2014, 66, 112–255 CrossRef CAS.
- W. Y. Chen, X. Jiang, S.-N. Lai, D. Peroulis and L. Stanciu, Nanohybrids of a MXene and transition metal dichalcogenide for selective detection of volatile organic compounds, Nat. Commun., 2020, 11, 1302 CrossRef CAS PubMed.
- J. Liu, L. Zhang, J. Fan, B. Zhu and J. Yu, Triethylamine gas sensor based on Pt-functionalized hierarchical ZnO microspheres, Sens. Actuators, B, 2021, 331, 129425 CrossRef CAS.
- W. Yang, L. Feng, S. He, L. Liu and S. Liu, Density gradient strategy for preparation of broken In2O3 microtubes with remarkably selective detection of triethylamine vapor, ACS Appl. Mater. Interfaces, 2018, 10, 27131–27140 CrossRef CAS PubMed.
- Y.-M. Jo, Y. K. Jo, J.-H. Lee, H. W. Jang, I.-S. Hwang and D. J. Yoo, MOF-based chemiresistive gas sensors: Toward new functionalities, Adv. Mater., 2023, 35, 2206842 CrossRef CAS PubMed.
- T. Lin, X. Lv, Z. Hu, A. Xu and C. Feng, Semiconductor metal oxides as chemoresistive sensors for detecting volatile organic compounds, Sensors, 2019, 19, 233 CrossRef PubMed.
- A. Marikutsa, M. Rumyantseva, E. A. Konstantinova and A. Gaskov, The key role of active sites in the development of selective metal oxide sensor materials, Sensors, 2021, 21, 2554 CrossRef CAS PubMed.
- C. Wang, L. Yin, L. Zhang, D. Xiang and R. Gao, Metal oxide gas sensors: sensitivity and influencing factors, Sensors, 2010, 10, 2088–2106 CrossRef CAS PubMed.
- R. Paul, B. Das and R. Ghosh, Novel approaches towards design of metal oxide based hetero-structures for room temperature gas sensor and its sensing mechanism: A recent progress, J. Alloys Compd., 2023, 941, 168943 CrossRef CAS.
- H. Ji, W. Zeng and Y. Li, Gas sensing mechanisms of metal oxide semiconductors: a focus review, Nanoscale, 2019, 11, 22664–22684 RSC.
- A. Dey, Semiconductor metal oxide gas sensors: A review, J. Mater. Sci. Eng. B, 2018, 229, 206–217 CrossRef CAS.
- C. C. Li, Z. F. Du, L. M. Li, H. C. Yu, Q. Wan and T. H. Wang, Surface-depletion controlled gas sensing of ZnO nanorods grown at room temperature, Appl. Phys. Lett., 2007, 91, 032101 CrossRef.
- L. Y. Zhu, L. X. Ou, L. W. Mao, X. Y. Wu, Y. P. Liu and H. L. Lu, Advances in noble metal-decorated metal oxide nanomaterials for chemiresistive gas sensors: Overview, Nano-Micro Lett., 2023, 15, 89 CrossRef CAS PubMed.
- W.-T. Koo, S.-J. Choi, S.-J. Kim, J.-S. Jang, H. L. Tuller and I.-D. Kim, Heterogeneous sensitization of metal−organic framework driven metal@metal oxide complex catalysts on an oxide nanofiber scaffold toward superior gas sensors, J. Am. Chem. Soc., 2016, 138, 13431–13437 CrossRef CAS PubMed.
- P. Wang, T. Dong, C. Jia and P. Yang, Ultraselective acetone-gas sensor based ZnO flowers functionalized by Au nanoparticle loading on certain facet, Sens. Actuators, B, 2019, 288, 1–11 CrossRef CAS.
- Y. Xu, L. Zheng, C. Yang, W. Zheng, X. Liu and J. Zhang, Oxygen vacancies enabled porous SnO2 thin films for highly sensitive detection of triethylamine at room temperature, ACS Appl. Mater. Interfaces, 2020, 12, 20704–20713 CrossRef CAS PubMed.
- V. Kumar, S. M. Majhi, K.-H. Kim, H. W. Kim and E. E. Kwon, Advances in In2O3-based materials for the development of hydrogen sulfide sensors, Chem. Eng. J., 2021, 404, 126472 CrossRef CAS.
- S. Uma and M. K. Shobana, Metal oxide semiconductor gas sensors in clinical diagnosis and environmental monitoring, Sens. Actuators, A, 2023, 349, 114044 CrossRef CAS.
- P. Cao, Y. Cai, D. Pawar, S. T. Navale, C. N. Rao, S. Han, W. Xu, M. Fang, X. Liu, Y. Zeng, W. Liu, D. Zhu and Y. Lu, Down to ppb level NO2 detection by ZnO/rGO heterojunction based chemiresistive sensors, Chem. Eng. J., 2020, 401, 125491 CrossRef CAS.
- L. Zheng, J. Xie, X. Liu, C. Yang, W. Zheng and J. Zhang, Unveiling the electronic interaction in ZnO/PtO/Pt nanoarrays for catalytic detection of triethylamine with ultrahigh sensitivity, ACS Appl. Mater. Interfaces, 2020, 12, 46267–46276 CrossRef CAS PubMed.
- K. Sun, G. Zhan, L. Zhang, Z. Wang and S. Lin, Highly sensitive NO2 gas sensor based on ZnO nanoarray modulated by oxygen vacancy with Ce doping, Sens. Actuators, B, 2023, 379, 133294 CrossRef CAS.
- L. Liu and S. Liu, Oxygen vacancies as an efficient strategy for promotion of low concentration SO2 gas sensing: the case of Au-modified SnO2, ACS Sustain. Chem. Eng., 2018, 6, 13427–13434 CrossRef CAS.
- Y. Gui, K. Tian, J. Liu, L. Yang, H. Zhang and Y. Wang, Superior triethylamine detection at room temperature by {-112} faceted WO3 gas sensor, J. Hazard. Mater., 2019, 380, 120876 CrossRef CAS PubMed.
- L. Liu, S. Shu, G. Zhang and S. Liu, Highly selective sensing of C2H6O, HCHO, and C3H6O gases by controlling SnO2 nanoparticle vacancies, ACS Appl. Nano Mater., 2018, 1, 31–37 CrossRef CAS.
- K. G. Krishna, G. Umadevi, S. Parne and N. Pothukanuri, Zinc oxide based gas sensors and their derivatives: a critical review, J. Mater. Chem. C, 2023, 11, 3906–3925 RSC.
- Q. A. Drmosh, Y. A. Al Wajih, I. O. Alade, A. K. Mohamedkhair, M. Qamar, A. S. Hakeem and Z. H. Yamani, Engineering the depletion layer of Au-modified ZnO/Ag core-shell films for high-performance acetone gas sensing, Sens. Actuators, B, 2021, 338, 129851 CrossRef CAS.
- H. J. Kim and J. H. Lee, Highly sensitive and selective gas sensors using p-type oxide semiconductors: Overview, Sens. Actuators, B, 2014, 192, 607–627 CrossRef CAS.
- T. W. Yuan, Z. J. Li, W. S. Zhang, Z. G. Xue, X. Q. Wang, Z. H. Ma, Y. Fan, J. Q. Xu and Y. E. Wu, Highly sensitive ethanol gas sensor based on ultrathin nanosheets assembled Bi2WO6 with composite phase, Sci. Bull., 2019, 64, 595–602 CrossRef CAS PubMed.
- S. M. Majhi, A. Mirzaei, H. W. Kim, S. S. Kim and T. W. Kim, Recent advances in energy-saving chemiresistive gas sensors: a review, Nano Energy, 2021, 79, 105369 CrossRef CAS PubMed.
- S. H. Hosseini-Shokouh, J. Zhou, E. Berger, Z.-P. Lv, X. Hong, V. Virtanen, K. Kordas and H.-P. Komsa, Highly selective H2S gas sensor based on Ti3C2Tx MXene–organic composites, ACS Appl. Mater. Interfaces, 2023, 15, 7063–7073 CrossRef CAS PubMed.
- Z. Shahrbabaki, S. Farajikhah, M. B. Ghasemian, F. Oveissi, R. J. Rath, J. Yun, F. Dehghani and S. Naficy, A flexible and polymer-based chemiresistive CO2 gas sensor at room temperature, Adv. Mater. Technol., 2023, 8, 2201510 CrossRef CAS.
- H. Yuan, J. Tao, N. Li, A. Karmakar, C. Tang, H. Cai, S. J. Pennycook, N. Singh and D. Zhao, On-chip tailorability of capacitive gas sensors integrated with metal–organic framework films, Angew. Chem., Int. Ed., 2019, 58, 14089–14094 CrossRef CAS PubMed.
- H. Tang, L. N. Sacco, S. Vollebregt, H. Ye, X. Fan and G. Zhang, Recent advances in 2D/nanostructured metal sulfide-based gas sensors: mechanisms, applications, and perspectives, J. Mater. Chem. A, 2020, 8, 24943–24976 RSC.
- J. Li, Y. J. Lu, Q. Ye, M. Cinke, J. Han and M. Meyyappan, Carbon nanotube sensors for gas and organic vapor detection, Nano Lett., 2003, 3, 929–933 CrossRef CAS.
- Z. Zou, Z. Zhao, Z. Zhang, W. Tian, C. Yang, X. Jin and K. Zhang, Room-temperature optoelectronic gas sensor based on core–shell g-C3N4@WO3 heterocomposites for efficient ammonia detection, Anal. Chem., 2023, 95, 2110–2118 CrossRef CAS PubMed.
- A. K. Elger and C. Hess, Elucidating the mechanism of working SnO2 gas sensors using combined operando UV/Vis, Raman, and IR spectroscopy, Angew. Chem., Int. Ed., 2019, 58, 15057–15061 CrossRef CAS PubMed.
- D. Degler, U. Weimar and N. Barsan, Current understanding of the fundamental mechanisms of doped and loaded semiconducting metal-oxide-based gas sensing materials, ACS Sens., 2019, 4, 2228–2249 CrossRef CAS PubMed.
- S. Zhou, Q. Lu, M. Chen, B. Li, H. Wei, B. Zi, J. Zeng, Y. Zhang, J. Zhang, Z. Zhu and Q. Liu, Platinum-supported cerium-doped indium oxide for highly sensitive triethylamine gas sensing with good antihumidity, ACS Appl. Mater. Interfaces, 2020, 12, 42962–42970 CrossRef CAS PubMed.
- Y. Xu, W. Zheng, X. Liu, L. Zhang, L. Zheng, C. Yang, N. Pinna and J. Zhang, Platinum single atoms on tin oxide ultrathin films for extremely sensitive gas detection, Mater. Horiz., 2020, 7, 1519–1527 RSC.
- D. Li, Y. Li, X. Wang, G. Sun, J. Cao and Y. Wang, Surface modification of In2O3 porous nanospheres with Au single atoms for ultrafast and highly sensitive detection of CO, Appl. Surf. Sci., 2023, 613, 155987 CrossRef CAS.
- P. Wang, S. Guo, Z. Hu, L. Zhou, T. Li, S. Pu, H. Mao, H. Cai, Z. Zhu, B. Chen, H. Y. Li and H. Liu, Single-atom Cu stabilized on ultrathin WO2.72 nanowire for highly selective and ultrasensitive ppb-level toluene detection, Adv. Sci., 2023, 10, e2302778 CrossRef PubMed.
- J. Hu, S. Zhai, Q. Zhang, H. Cui and X. Jiang, Two-dimensional HfTe2 monolayer treated by dispersed single Pt atom for hazardous gas Detection: A First-principles study, Appl. Surf. Sci., 2022, 605, 154572 CrossRef CAS.
- L. Mu, D. Chen and H. Cui, Single Pd atom embedded Janus HfSeTe as promising sensor for dissolved gas detection in transformer oil: A density functional theory study, Surf. Interfaces, 2022, 35, 102398 CrossRef CAS.
- A. Chen, Y. Han, Z. Wang, J. Cai, S. Ye and J. Li, Single atom modified two-dimensional bismuthenes for toxic gas detection, Phys. Chem. Chem. Phys., 2023, 25, 9249–9255 RSC.
- Q. Rong, B. Xiao, J. Zeng, R. Yu, B. Zi, G. Zhang, Z. Zhu, J. Zhang, J. Wu and Q. Liu, Pt single atom-induced activation energy and adsorption enhancement for an ultrasensitive ppb-level methanol gas sensor, ACS Sens., 2022, 7, 199–206 CrossRef CAS PubMed.
- Q. Zhou, L. Yang, Z. Kan, J. Lyu, M. Xuan Wang, B. Dong, X. Bai, Z. Chang, H. Song and L. Xu, Diverse scenarios selective perception of H2S via cobalt sensitized MOF filter membrane coated three-dimensional metal oxide sensor, Chem. Eng. J., 2022, 450, 138014 CrossRef CAS.
- V. V. Ganbavle, S. I. Inamdar, G. L. Agawane, J. H. Kim and K. Y. Rajpure, Synthesis of fast response, highly sensitive and selective Ni:ZnO based NO2 sensor, Chem. Eng. J., 2016, 286, 36–47 CrossRef CAS.
- Y. Zou, S. Chen, J. Sun, J. Liu, Y. Che, X. Liu, J. Zhang and D. Yang, Highly efficient gas sensor using a hollow SnO2 microfiber for triethylamine detection, ACS Sens., 2017, 2, 897–902 CrossRef CAS PubMed.
- W. Yang, P. Wan, M. Jia, J. Hu, Y. Guan and L. Feng, A novel electronic nose based on porous In2O3 microtubes sensor array for the discrimination of VOCs, Biosens. Bioelectron., 2015, 64, 547–553 CrossRef CAS PubMed.
- B. Zong, Q. Xu and S. Mao, Single-atom Pt-functionalized Ti3C2Tx field-effect transistor for volatile organic compound gas detection, ACS Sens., 2022, 7, 1874–1882 CrossRef CAS PubMed.
- B. Liu, L. Zhang, Y. Luo, L. Gao and G. Duan, The dehydrogenation of H-S bond into sulfur species on supported Pd single atoms allows highly selective and sensitive hydrogen sulfide detection, Small, 2021, 17, e2105643 CrossRef PubMed.
- L. Zhou, X. Chang, W. Zheng, X. Liu and J. Zhang, Single atom Rh-sensitized SnO2 via atomic layer deposition for efficient formaldehyde detection, Chem. Eng. J., 2023, 475, 146300 CrossRef CAS.
- T. Yuan, Z. Xue, Y. Chen and J. Xu, Single Pt atom-based gas sensor: Break the detection limit and selectivity of acetone, Sens. Actuators, B, 2023, 397, 134139 CrossRef CAS.
- B. Liu, Q. Zhu, Y. Pan, F. Huang, L. Tang, C. Liu, Z. Cheng, P. Wang, J. Ma and M. Ding, Single-atom tailoring of two-dimensional atomic crystals enables highly efficient detection and pattern recognition of chemical vapors, ACS Sens., 2022, 7, 1533–1543 CrossRef CAS PubMed.
- L. Liu, C. Mao, H. Fu, X. Qu and S. Zheng, ZnO nanorod-immobilized Pt single-atoms as an ultrasensitive sensor for triethylamine detection, ACS Appl. Mater. Interfaces, 2023, 15, 16654–16663 CrossRef CAS PubMed.
- Y.-Z. Liu, R.-T. Guo, C.-P. Duan, G.-L. Wu, Y.-F. Miao, J.-W. Gu and W.-G. Pan, Removal of gaseous pollutants by using 3DOM-based catalysts: A review, Chemosphere, 2021, 262, 127886 CrossRef CAS PubMed.
- J. Xiang, Y. Su, L. Zhang, S. Hong, Z. Wang, D. Han and F. Gu, Atomically dispersed Pt on three-dimensional ordered macroporous SnO2 for highly sensitive and highly selective detection of triethylamine at a low working temperature, ACS Appl. Mater. Interfaces, 2022, 14, 13440–13449 CrossRef CAS PubMed.
- F. Gu, Y. Cui, D. Han, S. Hong, M. Flytzani-Stephanopoulos and Z. Wang, Atomically dispersed Pt (II) on WO3 for highly selective sensing and catalytic oxidation of triethylamine, Appl. Catal., B, 2019, 256, 117809 CrossRef CAS.
- X.-L. Ye, S.-J. Lin, J.-W. Zhang, H.-J. Jiang, L.-A. Cao, Y.-Y. Wen, M.-S. Yao, W.-H. Li, G.-E. Wang and G. Xu, Boosting room temperature sensing performances by atomically dispersed Pd stabilized via surface coordination, ACS Sens., 2021, 6, 1103–1110 CrossRef CAS PubMed.
- R. Lang, X. Du, Y. Huang, X. Jiang, Q. Zhang, Y. Guo, K. Liu, B. Qiao, A. Wang and T. Zhang, Single-atom catalysts based on the metal–oxide interaction, Chem. Rev., 2020, 120, 11986–12043 CrossRef CAS PubMed.
- N. Murata, T. Suzuki, Y. Lin, H. Nitani, Y. Niwa, T. Wada, M. Uo and K. Asakura, Structure of atomically dispersed Pt in a SnO2 thin film under reaction conditions: origin of its high performance in micro electromechanical system gas sensor catalysis, ACS Appl. Mater. Interfaces, 2022, 14, 39507–39514 CrossRef CAS PubMed.
- J. Liu, Y. Tang, Y. Wang, T. Zhang and J. Li, Theoretical understanding of the stability of single-atom catalysts, Natl. Sci. Rev., 2018, 5, 638–641 CrossRef CAS.
- R. Qin, P. Liu, G. Fu and N. Zheng, Strategies for stabilizing atomically dispersed metal catalysts, Small Methods, 2018, 2, 1700286 CrossRef.
- T. Dai, Z. Yan, M. Li, Y. Han, Z. Deng, S. Wang, R. Wang, X. Xu, L. Shi, W. Tong, J. Bao, Z. Qiao, L. Li and G. Meng, Boosting electrical response toward trace volatile organic compounds molecules via pulsed temperature modulation of Pt anchored WO3 chemiresistor, Small Methods, 2022, 6, e2200728 CrossRef PubMed.
- L. Li, H. Su, L. Zhou, Z. Hu, T. Li, B. Chen, H.-Y. Li and H. Liu, Single-atom Ce targeted regulation SnS/SnS2 heterojunction for sensitive and stable room-temperature ppb-level gas sensor, Chem. Eng. J., 2023, 472, 144796 CrossRef CAS.
- S. Zhai, X. Jiang, D. Wu, L. Chen, Y. Su, H. Cui and F. Wu, Single Rh atom decorated pristine and S-defected PdS2 monolayer for sensing thermal runaway gases in a lithium-ion battery: A first-principles study, Surf. Interfaces, 2023, 37, 102735 CrossRef CAS.
- K. Koga, Electronic and catalytic effects of single-atom Pd additives on the hydrogen sensing properties of Co3O4 nanoparticle films, ACS Appl. Mater. Interfaces, 2020, 12, 20806–20823 CrossRef CAS PubMed.
- B. Liu, K. Li, Y. Luo, L. Gao and G. Duan, Sulfur spillover driven by charge transfer between AuPd alloys and SnO2 allows high selectivity for dimethyl disulfide gas sensing, Chem. Eng. J., 2021, 420, 129881 CrossRef CAS.
- Q. Zhou, W. Zeng, W. Chen, L. Xu, R. Kumar and A. Umar, High sensitive and low-concentration sulfur dioxide (SO2) gas sensor application of heterostructure NiO-ZnO nanodisks, Sens. Actuators, B, 2019, 298, 126870 CrossRef CAS.
- T. Wang, J. Hao, S. Zheng, Q. Sun, D. Zhang and Y. Wang, Highly sensitive and rapidly responding room-temperature NO2 gas sensors based on WO3 nanorods/sulfonated graphene nanocomposites, Nano Res., 2018, 11, 791–803 CrossRef CAS.
- H. Shin, W.-G. Jung, D.-H. Kim, J.-S. Jang, Y. H. Kim, W.-T. Koo, J. Bae, C. Park, S.-H. Cho, B. J. Kim and I.-D. Kim, Single-atom Pt stabilized on one-dimensional nanostructure support via carbon nitride/SnO2 heterojunction trapping, ACS Nano, 2020, 14, 11394–11405 CrossRef CAS PubMed.
- L. Sun, B. Wang and Y. Wang, High-temperature gas sensor based on novel Pt single atoms@SnO2 nanorods@SiC nanosheets multi-heterojunctions, ACS Appl. Mater. Interfaces, 2020, 12, 21808–21817 CrossRef CAS PubMed.
- R. Tian, S. Wang, X. Hu, J.-G. Zheng, P. Ji, J. Lin, J. Zhang, M. Xu, J. Bao, S. Zuo, H. Zhang, W. Zhang, J. Wang and L. Yu, Novel approaches for highly selective, room-temperature gas sensors based on atomically dispersed non-precious metals, J. Mater. Chem. A, 2020, 8, 23784–23794 RSC.
- J. Ding, H. Yang, X.-L. Ma, S. Liu, W. Liu, Q. Mao, Y. Huang, J. Li, T. Zhang and B. Liu, A tin-based tandem electrocatalyst for CO2 reduction to ethanol with 80% selectivity, Nat. Energy, 2023, 8, 1386–1394 CrossRef CAS.
- X. Geng, S. Li, L. Mawella-Vithanage, T. Ma, M. Kilani, B. Wang, L. Ma, C. C. Hewa-Rahinduwage, A. Shafikova, E. Nikolla, G. Mao, S. L. Brock, L. Zhang and L. Luo, Atomically dispersed Pb ionic sites in PbCdSe quantum dot gels enhance room-temperature NO2 sensing, Nat. Commun., 2021, 12, 4895 CrossRef CAS PubMed.
- Z. G. Xue, M. Y. Yan, X. Yu, Y. J. Tong, H. Zhou, Y. F. Zhao, Z. Y. Wang, Y. S. Zhang, C. Xiong, J. Yang, X. Hong, J. Luo, Y. Lin, W. X. Huang, Y. Li and Y. E. Wu, One-dimensional segregated single Au sites on step-rich ZnO ladder for ultrasensitive NO2 sensors, Chem, 2020, 6, 3364–3373 CAS.
- G. Li, H. Zhang, L. Meng, Z. Sun, Z. Chen, X. Huang and Y. Qin, Adjustment of oxygen vacancy states in ZnO and its application in ppb-level NO2 gas sensor, Sci. Bull., 2020, 65, 1650–1658 CrossRef CAS PubMed.
- S. Zhao, Y. Yang, F. Bi, Y. Chen, M. Wu, X. Zhang and G. Wang, Oxygen vacancies in the catalyst: Efficient degradation of gaseous pollutants, Chem. Eng. J., 2023, 454, 140376 CrossRef CAS.
- L. Liu, P. Zhou, X. Su, Y. Liu, Y. Sun, H. Yang, H. Fu, X. Qu, S. Liu and S. Zheng, Synergistic Ni single atoms and oxygen vacancies on SnO2 nanorods toward promoting SO2 gas sensing, Sens. Actuators, B, 2022, 351, 130983 CrossRef CAS.
- Z. Zhao, P. Zhang, H. Tan, X. Liang, T. Li, Y. Gao and C. Hu, Low concentration of peroxymonosulfate triggers dissolved oxygen conversion over single atomic Fe–N3O1 sites for water decontamination, Small, 2023, 19, 2205583 CrossRef CAS PubMed.
- B. Huang, Z. Wu, H. Zhou, J. Li, C. Zhou, Z. Xiong, Z. Pan, G. Yao and B. Lai, Recent advances in single-atom catalysts for advanced oxidation processes in water purification, J. Hazard. Mater., 2021, 412, 125253 CrossRef CAS PubMed.
- W. Ma, M. Sun, D. Huang, C. Chu, T. Hedtke, X. Wang, Y. Zhao, J.-H. Kim and M. Elimelech, Catalytic membrane with copper single-atom catalysts for effective hydrogen peroxide activation and pollutant destruction, Environ. Sci. Technol., 2022, 56, 8733–8745 CrossRef CAS PubMed.
- Y. Liu, L. Liu and Y. Wang, A critical review on removal of gaseous pollutants using sulfate radical-based advanced oxidation technologies, Environ. Sci. Technol., 2021, 55, 9691–9710 CrossRef CAS PubMed.
- Z. Wan, X. Hu, C. Li, J. Zhang, Q. Wang, L. Fang, L. Zhang, Q. Guo and D. Sun, Simultaneous oxidation and absorption of nitric oxide and sulfur dioxide by peroxymonosulfate activated by bimetallic metal-organic frameworks, J. Environ. Chem. Eng., 2023, 11, 109417 CrossRef CAS.
- M. S. Johnson, E. J. K. Nilsson, E. A. Svensson and S. Langer, Gas-phase advanced oxidation for effective, efficient in situ control of pollution, Environ. Sci. Technol., 2014, 48, 8768–8776 CrossRef CAS PubMed.
- Z. Chen, J. Li, S. Wang, J. Zhao, J. Liu, J. Shen, C. Qi and P. Yang, Structure-property-performance relationship of transition metal doped WO3 mixed oxides for catalytic degradation of organic pollutants, Chemosphere, 2023, 316, 137797 CrossRef CAS PubMed.
- B. Lin, Z. Guo, J. Li, G. Xiao, D. Ye and Y. Hu, V-Cu bimetallic oxide supported catalysts for synergistic removal of toluene and NOx from coal-fired flue gas: The crucial role of support, Chem. Eng. J., 2023, 458, 141443 CrossRef CAS.
- Z. Zhang, Y. Zhu, H. Asakura, B. Zhang, J. Zhang, M. Zhou, Y. Han, T. Tanaka, A. Wang, T. Zhang and N. Yan, Thermally stable single atom Pt/m-Al2O3 for selective hydrogenation and CO oxidation, Nat. Commun., 2017, 8, 16100 CrossRef CAS PubMed.
- S. Wang and J. Wang, Single atom cobalt catalyst derived from co-pyrolysis of vitamin B12 and graphitic carbon nitride for PMS activation to degrade emerging pollutants, Appl. Catal., B, 2023, 321, 122051 CrossRef CAS.
- J. Xu, Y. Wang, K. Wang, M. Zhao, R. Zhang, W. Cui, L. Liu, M. S. Bootharaju, J. H. Kim, T. Hyeon, H. Zhang, Y. Wang, S. Song and X. Wang, Single-atom Rh on high-index CeO2 facet for highly enhanced catalytic CO oxidation, Angew. Chem., Int. Ed., 2023, 62, e202302877 CrossRef CAS PubMed.
- M. Mahmoodinia, P.-O. Åstrand and D. Chen, Tuning the electronic properties of single-atom Pt catalysts by functionalization of the carbon support material, J. Phys. Chem. C, 2017, 121, 20802–20812 CrossRef CAS.
- B.-T. Qiao, J.-X. Liang, A.-Q. Wang, J.-Y. Liu and T. Zhang, Single atom gold catalysts for low-temperature CO oxidation, Chin. J. Catal., 2016, 37, 1580–1586 CrossRef CAS.
- R. Shan, Z. Sheng, S. Hu, H. Xiao, Y. Zhang, J. Zhang, L. Wang, C. Zhang and J. Li, Enhancing oxidation reaction over Pt-MnO2 catalyst by activation of surface oxygen, J. Environ. Sci., 2023, 134, 117–125 CrossRef CAS PubMed.
- J. Liu, X. Fang, D. Liu, X. Hu, T. Qin, J. Chen, R. Liu, D. Xu, W. Qu, Y. Dong, L. Chen, Z. Ma, X. Liu, X. Li and X. Tang, Benzene abatement catalyzed by Ceria-Supported platinum nanoparticles and single atoms, Chem. Eng. J., 2023, 467, 143407 CrossRef CAS.
- Y. Chen, Z. Huang, M. Zhou, Z. Ma, J. Chen and X. Tang, Single silver
adatoms on nanostructured manganese oxide surfaces: Boosting oxygen activation for benzene abatement, Environ. Sci. Technol., 2017, 51, 2304–2311 CrossRef CAS PubMed.
- H. Deng, S. Kang, J. Ma, C. Zhang and H. He, Silver incorporated into cryptomelane-type Manganese oxide boosts the catalytic oxidation of benzene, Appl. Catal., B, 2018, 239, 214–222 CrossRef CAS.
- J. Chen, D. Yan, Z. Xu, X. Chen, X. Chen, W. Xu, H. Jia and J. Chen, A novel redox precipitation to synthesize Au-doped α-MnO2 with high dispersion toward low-temperature oxidation of formaldehyde, Environ. Sci. Technol., 2018, 52, 4728–4737 CrossRef CAS PubMed.
- J. Chen, M. Jiang, J. Chen, W. Xu and H. Jia, Selective immobilization of single-atom Au on cerium dioxide for low-temperature removal of C1 gaseous contaminants, J. Hazard. Mater., 2020, 392, 122511 CrossRef CAS PubMed.
- H. Zhang, S. Sui, X. Zheng, R. Cao and P. Zhang, One-pot synthesis of atomically dispersed Pt on MnO2 for efficient catalytic decomposition of toluene at low temperatures, Appl. Catal., B, 2019, 257, 117878 CrossRef CAS.
- J. Chen, M. Jiang, W. Xu, J. Chen, Z. Hong and H. Jia, Incorporating Mn cation as anchor to atomically disperse Pt on TiO2 for low-temperature removal of formaldehyde, Appl. Catal., B, 2019, 259, 118013 CrossRef CAS.
- G. Liu, J. Zhou, W. Zhao, Z. Ao and T. An, Single atom catalytic oxidation mechanism of formaldehyde on Al doped graphene at room temperature, Chin. Chem. Lett., 2020, 31, 1966–1969 CrossRef CAS.
- Z. Hou, L. Dai, Y. Liu, J. Deng, L. Jing, W. Pei, R. Gao, Y. Feng and H. Dai, Highly efficient and enhanced sulfur resistance supported bimetallic single-atom palladium–cobalt catalysts for benzene oxidation, Appl. Catal., B, 2021, 285, 119844 CrossRef CAS.
- J. K. Lampert, M. S. Kazi and R. J. Farrauto, Palladium catalyst performance for methane emissions abatement from lean burn natural gas vehicles, Appl. Catal., B, 1997, 14, 211–223 CrossRef CAS.
- C. Gao, J. Low, R. Long, T. Kong, J. Zhu and Y. Xiong, Heterogeneous single-atom photocatalysts: fundamentals and applications, Chem. Rev., 2020, 120, 12175–12216 CrossRef CAS PubMed.
- J. Qin, Y. Pei, Y. Zheng, D. Ye and Y. Hu, Fe-MOF derivative photocatalyst with advanced oxygen reduction capacity for indoor pollutants removal, Appl. Catal., B, 2023, 325, 122346 CrossRef CAS.
- S. Zhao, H. Choe, S. Saqlain, C.-C. Hwang, Z. Liu, Y. Choi, Z. Peng and Y. D. Kim, Simultaneous oxidation of NO and acetaldehyde over bare and Fe-modified TiO2 under visible light irradiation, Appl. Surf. Sci., 2023, 627, 157308 CrossRef CAS.
- J. Ding, Z. Teng, X. Su, K. Kato, Y. Liu, T. Xiao, W. Liu, L. Liu, Q. Zhang, X. Ren, J. Zhang, Z. Chen, O. Teruhisa, A. Yamakata, H. Yang, Y. Huang, B. Liu and Y. Zhai, Asymmetrically coordinated cobalt single atom on carbon nitride for highly selective photocatalytic oxidation of CH4 to CH3OH, Chem, 2023, 9, 1017–1035 CAS.
- X. Wu, H. Zhang, J. Dong, M. Qiu, J. Kong, Y. Zhang, Y. Li, G. Xu, J. Zhang and J. Ye, Surface step decoration of isolated atom as electron pumping: Atomic-level insights into visible-light hydrogen evolution, Nano Energy, 2018, 45, 109–117 CrossRef CAS.
- B.-H. Lee, S. Park, M. Kim, A. K. Sinha, S. C. Lee, E. Jung, W. J. Chang, K.-S. Lee, J. H. Kim, S.-P. Cho, H. Kim, K. T. Nam and T. Hyeon, Reversible and cooperative photoactivation of single-atom Cu/TiO2 photocatalysts, Nat. Mater., 2019, 18, 620–626 CrossRef CAS PubMed.
- X. Li, Z. Le, X. Chen, Z. Li, W. Wang, X. Liu, A. Wu, P. Xu and D. Zhang, Graphene oxide enhanced amine-functionalized titanium metal organic framework for visible-light-driven photocatalytic oxidation of gaseous pollutants, Appl. Catal., B, 2018, 236, 501–508 CrossRef CAS.
- M. Wen, G. Li, H. Liu, J. Chen, T. An and H. Yamashita, Metal–organic framework-based nanomaterials for adsorption and photocatalytic degradation of gaseous pollutants: recent progress and challenges, Environ. Sci.: Nano, 2019, 6, 1006–1025 RSC.
- L. Luo, J. Luo, H. Li, F. Ren, Y. Zhang, A. Liu, W.-X. Li and J. Zeng, Water enables mild oxidation of methane to methanol on gold single-atom catalysts, Nat. Commun., 2021, 12, 1218 CrossRef CAS PubMed.
- Z.-H. Xue, D. Luan, H. Zhang and X. W. Lou, Single-atom catalysts for photocatalytic energy conversion, Joule, 2022, 6, 92–133 CrossRef CAS.
- X.-L. Wu, S. Liu, Y. Li, M. Yan, H. Lin, J. Chen, S. Liu, S. Wang and X. Duan, Directional and Ultrafast Charge Transfer in Oxygen-Vacancy-Rich ZnO@Single-Atom Cobalt Core-Shell Junction for Photo-Fenton-Like Reaction, Angew. Chem., Int. Ed., 2023, 62, e202305639 CrossRef CAS PubMed.
- Y. Zou, J. Hu, B. Li, L. Lin, Y. Li, F. Liu and X.-y. Li, Tailoring the coordination environment of cobalt in a single-atom catalyst through phosphorus doping for enhanced activation of peroxymonosulfate and thus efficient degradation of sulfadiazine, Appl. Catal., B, 2022, 312, 121408 CrossRef CAS.
- D. Li, S. Zhang, S. Li, J. Tang, T. Hua and F. Li, Mechanism of the application of single-atom catalyst-activated PMS/PDS to the degradation of organic pollutants in water environment: A review, J. Cleaner Prod., 2023, 397, 136468 CrossRef CAS.
- Z. Wang, S. Xie, Y. Feng, P. Ma, K. Zheng, E. Duan, Y. Liu, H. Dai and J. Deng, Simulated solar light driven photothermal catalytic purification of toluene over iron oxide supported single atom Pt catalyst, Appl. Catal., B, 2021, 298, 120612 CrossRef CAS.
- Y. Feng, L. Dai, Z. Wang, Y. Peng, E. Duan, Y. Liu, L. Jing, X. Wang, A. Rastegarpanah, H. Dai and J. Deng, Photothermal synergistic effect of Pt1/CuO-CeO2 single-atom catalysts significantly improving toluene removal, Environ. Sci. Technol., 2022, 56, 8722–8732 CrossRef CAS PubMed.
- E. Agbovhimen Elimian, M. Zhang, Y. Sun, J. He and H. Jia, Harnessing solar energy towards synergistic photothermal catalytic oxidation of volatile organic compounds, Sol. RRL, 2023, 7, 2300238 CrossRef CAS.
- K. Li, W. Cui, J. Li, Y. Sun, Y. Chu, G. Jiang, Y. Zhou, Y. Zhang and F. Dong, Tuning the reaction pathway of photocatalytic NO oxidation process to control the secondary pollution on monodisperse Au nanoparticles@g-C3N4, Chem. Eng. J., 2019, 378, 122184 CrossRef CAS.
- Z. Teng, Q. Zhang, H. Yang, K. Kato, W. Yang, Y.-R. Lu, S. Liu, C. Wang, A. Yamakata, C. Su, B. Liu and T. Ohno, Atomically dispersed antimony on carbon nitride for the artificial photosynthesis of hydrogen peroxide, Nat. Catal., 2021, 4, 374–384 CrossRef CAS.
- R. Zhang, Y. Cao, D. E. Doronkin, M. Ma, F. Dong and Y. Zhou, Single-atom dispersed Zn-N3 active sites bridging the interlayer of g-C3N4 to tune NO oxidation pathway for the inhibition of toxic by-product generation, Chem. Eng. J., 2023, 454, 140084 CrossRef CAS.
- X. Wu, S. Sun, R. Wang, Z. Huang, H. Shen, H. Zhao and G. Jing, Pt single atoms and defect engineering of TiO2-nanosheet-assembled hierarchical spheres for efficient room-temperature HCHO oxidation, J. Hazard. Mater., 2023, 454, 131434 CrossRef CAS PubMed.
- J. Chen, L. Chen, X. Wang, J. Sun, A. Chen and X. Xie, Er single atoms decorated TiO2 and Er3+ ions modified TiO2 for photocatalytic oxidation of mixed VOCs, Appl. Surf. Sci., 2022, 596, 153655 CrossRef CAS.
- K. Mori, T. Murakami and H. Yamashita, Luminescent single-atom Eu-coordinated graphitic carbon nitride nanosheets for selective sensing of acetone and cyclohexane, ACS Appl. Nano Mater., 2020, 3, 10209–10217 CrossRef CAS.
- D. Xia, W. Xu, Y. Wang, J. Yang, Y. Huang, L. Hu, C. He, D. Shu, D. Y. C. Leung and Z. Pang, Enhanced performance and conversion pathway for catalytic ozonation of methyl mercaptan on single-atom Ag deposited three-dimensional ordered mesoporous MnO2, Environ. Sci. Technol., 2018, 52, 13399–13409 CrossRef CAS PubMed.
- Z. Hu, X. Li, S. Zhang, Q. Li, J. Fan, X. Qu and K. Lv, Fe1/TiO2 hollow microspheres: Fe and Ti dual active sites boosting the photocatalytic oxidation of NO, Small, 2020, 16, e2004583 CrossRef PubMed.
- G. Liu, Y. Huang, H. Lv, H. Wang, Y. Zeng, M. Yuan, Q. Meng and C. Wang, Confining single-atom Pd on g-C3N4 with carbon vacancies towards enhanced photocatalytic NO conversion, Appl. Catal., B, 2021, 284, 119683 CrossRef CAS.
- Y. Liu, S. Shi and Y. Wang, Removal of pollutants from gas streams using Fenton (-like)-based oxidation systems: A review, J. Hazard. Mater., 2021, 416, 125927 CrossRef CAS.
- S. Liu, Y. Hu, H. Xu, Z. Lou, J. Chen, C.-Z. Yuan, X. Lv, X. Duan, S. Wang and X.-L. Wu, Directional electron transfer in single-atom cobalt nanozyme for enhanced photo-Fenton-like reaction, Appl. Catal., B, 2023, 335, 122882 CrossRef CAS.
- S. Liu, D. Liu, Y. Sun, P. Xiao, H. Lin, J. Chen, X.-L. Wu, X. Duan and S. Wang, Enzyme-mimicking single-atom FeN4 sites for enhanced photo-Fenton-like reactions, Appl. Catal., B, 2022, 310, 121327 CrossRef CAS.
- L. Mu, M. Wang, F. Jiang, Q. Gao, M. Zhang, Z. Xiong, Y. Li, R. Shen, J. Hu and G. Wu, Boosting Photo-Fenton reactions by amidoxime chelated ferrous iron (Fe(III)) catalyst for highly efficient pollutant control, Appl. Catal., B, 2021, 298, 120574 CrossRef CAS.
- H. Yang, Y. Wu, X. Zheng, S. Wu, B. Zhang, L. Hu, X. Guo and B. Wu, A review on gaseous pollutants purification using H2O2-based Fenton-like reactions, J. Environ. Chem. Eng., 2023, 11, 111066 CrossRef CAS.
- L. Su, P. Wang, X. Ma, J. Wang and S. Zhan, Regulating local electron density of iron single sites by introducing nitrogen vacancies for efficient photo-Fenton process, Angew. Chem., Int. Ed., 2021, 60, 21261–21266 CrossRef CAS PubMed.
- F. Chen, X.-L. Wu, C. Shi, H. Lin, J. Chen, Y. Shi, S. Wang and X. Duan, Molecular engineering toward pyrrolic N-rich M-N4 (M = Cr, Mn, Fe, Co, Cu) single-atom sites for enhanced heterogeneous Fenton-like reaction, Adv. Funct. Mater., 2021, 31, 2007877 CrossRef CAS.
- H. Song, L. Wei, C. Chen, C. Wen and F. Han, Photocatalytic production of H2O2 and its in situ utilization over atomic-scale Au modified MoS2 nanosheets, J. Catal., 2019, 376, 198–208 CrossRef CAS.
- Y. Zhou, M. Yu, Q. Zhang, X. Sun and J. Niu, Regulating electron distribution of Fe/Ni-N4P2 single sites for efficient photo-Fenton process, J. Hazard. Mater., 2022, 440, 129724 CrossRef CAS PubMed.
- Z. Zhao, W. Zhang, W. Liu, Y. Li, J. Ye, J. Liang and M. Tong, Activation of sulfite by single-atom Fe deposited graphitic carbon nitride for diclofenac removal: The synergetic effect of transition metal and photocatalysis, Chem. Eng. J., 2021, 407, 127167 CrossRef CAS.
- X. Li, X. Huang, S. Xi, S. Miao, J. Ding, W. Cai, S. Liu, X. Yang, H. Yang, J. Gao, J. Wang, Y. Huang, T. Zhang and B. Liu, Single cobalt atoms anchored on porous N-doped graphene with dual reaction sites for efficient Fenton-like catalysis, J. Am. Chem. Soc., 2018, 140, 12469–12475 CrossRef CAS PubMed.
- H. Tang, B. Ma, Z. Bian and H. Wang, Selective dechlorination degradation of chlorobenzenes by dual single-atomic Fe/Ni catalyst with M-N/M-O active sites synergistic, J. Hazard. Mater., 2023, 443, 130315 CrossRef CAS PubMed.
- S. Liu, G. Xing and J.-y. Liu, Computational screening of single-atom catalysts for direct electrochemical NH3 synthesis from NO on defective boron phosphide monolayer, Appl. Surf. Sci., 2023, 611, 155764 CrossRef CAS.
- H. Yin, Y. Peng and J. Li, Electrocatalytic Reduction of Nitrate to Ammonia via a Au/Cu Single Atom Alloy Catalyst, Environ. Sci. Technol., 2023, 57, 3134–3144 CrossRef CAS PubMed.
- K. Chen, G. Wang, Y. Guo, D. Ma and K. Chu, Iridium single-atom catalyst for highly efficient NO electroreduction to NH3, Nano Res., 2023, 16, 8737–8742 CrossRef CAS.
- L. Liu and S. Zheng, Advancements in single atom catalysts for electrocatalytic nitrate reduction reaction, ChemCatChem, 2024, e202301641 CrossRef.
- D. Wang, X. Zhu, X. Tu, X. Zhang, C. Chen, X. Wei, Y. Li and S. Wang, Oxygen-bridged copper-iron atomic pair as dual-metal active sites for boosting electrocatalytic NO reduction, Adv. Mater., 2023, 35, e2304646 CrossRef PubMed.
- Z. Wang, J. Zhao, J. Wang, C. R. Cabrera and Z. Chen, A Co–N4 moiety embedded into graphene as an efficient single-atom-catalyst for NO electrochemical reduction: a computational study, J. Mater. Chem. A, 2018, 6, 7547–7556 RSC.
- B. Hammer and J. K. Norskov, Why gold is the noblest of all the metals, Nature, 1995, 376, 238–240 CrossRef CAS.
- J. Greeley, J. K. Nørskov and M. Mavrikakis, Electronic structure and catalysis on metal surfaces, Annu. Rev. Phys. Chem., 2002, 53, 319–348 CrossRef CAS PubMed.
- K. Chen, J. Wang, H. Zhang, D. Ma and K. Chu, Self-tandem electrocatalytic NO reduction to NH3 on a W single-atom catalyst, Nano Lett., 2023, 23, 1735–1742 CrossRef CAS PubMed.
- B. He, P. Lv, D. Wu, X. Li, R. Zhu, K. Chu, D. Ma and Y. Jia, Confinement catalysis of a single atomic vacancy assisted by aliovalent ion doping enabled efficient NO electroreduction to NH3, J. Mater. Chem. A, 2022, 10, 18690–18700 RSC.
- K. Chen, N. Zhang, F. Wang, J. Kang and K. Chu, Main-group indium single-atom catalysts for electrocatalytic NO reduction to NH3, J. Mater. Chem. A, 2023, 11, 6814–6819 RSC.
- Q. Song, M. Li, X. Hou, J. Li, Z. Dong, S. Zhang, L. Yang and X. Liu, Anchored Fe atoms for N=O bond activation to boost electrocatalytic nitrate reduction at low concentrations, Appl. Catal., B, 2022, 317, 121721 CrossRef CAS.
- K. Chen, G. Zhang, X. Li, X. Zhao and K. Chu, Electrochemical NO reduction to NH3 on Cu single atom catalyst, Nano Res., 2022, 16, 5857–5863 CrossRef.
- M. Ran, H. Xu, Y. Bao, Y. Zhang, J. Zhang and M. Xing, Selective production of CO from organic pollutants by coupling piezocatalysis and advanced oxidation processes, Angew. Chem., Int. Ed., 2023, 62, e202303728 CrossRef CAS PubMed.
- Z. Chen, F. An, Y. Zhang, Z. Liang, W. Liu and M. Xing, Single-atom Mo–Co catalyst with low biotoxicity for sustainable degradation of high-ionization-potential organic pollutants, Proc. Natl. Acad. Sci. U. S. A., 2023, 120, e2305933120 CrossRef CAS PubMed.
- L. Jin, S. You, N. Ren, B. Ding and Y. Liu, Mo vacancy-mediated activation of peroxymonosulfate for ultrafast micropollutant removal using an electrified MXene filter functionalized with Fe single atoms, Environ. Sci. Technol., 2022, 56, 11750–11759 CrossRef CAS PubMed.
- L. Jin, S. You, Y. Yao, H. Chen, Y. Wang and Y. Liu, An electroactive single-atom copper anchored MXene nanohybrid filter for ultrafast water decontamination, J. Mater. Chem. A, 2021, 9, 25964–25973 RSC.
- C. Zhou, J. Li, Y. Zhang, J. Bai, P. Wang, B. Zhang, L. Zha, M. Long and B. Zhou, A novel SO3˙− mediated photoelectrocatalytic system based on MoS2/Fe2O3 and CuNW@CF for the efficient treatment of sulfurous and nitrogenous oxides, Appl. Catal., B, 2023, 330, 122579 CrossRef CAS.
- S. Li, H. Shang, Y. Tao, P. Li, H. Pan, Q. Wang, S. Zhang, H. Jia, H. Zhang, J. Cao, B. Zhang, R. Zhang, G. Li, Y. Zhang, D. Zhang and H. Li, Hydroxyl radical-mediated efficient photoelectrocatalytic NO oxidation with simultaneous nitrate storage using a flow photoanode reactor, Angew. Chem., Int. Ed., 2023, 62, e202305538 CrossRef CAS PubMed.
- J.-J. Liu, S.-N. Sun, J. Liu, Y. Kuang, J.-W. Shi, L.-Z. Dong, N. Li, J.-N. Lu, J.-M. Lin, S.-L. Li and Y.-Q. Lan, Achieving high-efficient photoelectrocatalytic degradation of 4-chlorophenol via functional reformation of titanium-oxo clusters, J. Am. Chem. Soc., 2023, 145, 6112–6122 CrossRef CAS PubMed.
- J. Zhang, J. Sun, H. Zhao and G. Zhao, Single-atom copper promotes efficient generation of hydroxyl radicals under alkaline circumstances in a photoelectrochemical oxygen reduction process, ACS EST Engg., 2022, 2, 1953–1963 CrossRef CAS.
- J. Zhang, Z. Zhou, Z. Feng, H. Zhao and G. Zhao, Fast generation of hydroxyl radicals by rerouting the electron transfer pathway via constructed chemical channels during the photo-electro-reduction of oxygen, Environ. Sci. Technol., 2022, 56, 1331–1340 CrossRef CAS PubMed.
- J. Zhang, G. Zhang, H. Lan, H. Liu and J. Qu, Sustainable nitrogen fixation over Ru single atoms decorated Cu2O using electrons produced from photoelectrocatalytic organics degradation, Chem. Eng. J., 2022, 428, 130373 CrossRef CAS.
- X. Li, H.-Y. Wang, H. Yang, W. Cai, S. Liu and B. Liu, In Situ/Operando Characterization Techniques to Probe the Electrochemical Reactions for Energy Conversion, Small
Methods, 2018, 2, 1700395 CrossRef.
- J. Ding, F. Li, J. Zhang, Q. Zhang, Y. Liu, W. Wang, W. Liu, B. Wang, J. Cai, X. Su, H. B. Yang, X. Yang, Y. Huang, Y. Zhai and B. Liu, Circumventing CO2 Reduction Scaling Relations Over the Heteronuclear Diatomic Catalytic Pair, J. Am. Chem. Soc., 2023, 145, 11829–11836 CrossRef CAS PubMed.
- S. Wei, Z. Li, K. Murugappan, Z. Li, F. Zhang, A. G. Saraswathyvilasam, M. Lysevych, H. H. Tan, C. Jagadish, A. Tricoli and L. Fu, A self-powered portable nanowire array gas sensor for dynamic NO2 monitoring at room temperature, Adv. Mater., 2023, 35, 2207199 CrossRef CAS PubMed.
|
This journal is © The Royal Society of Chemistry 2024 |