DOI:
10.1039/D4SC00175C
(Review Article)
Chem. Sci., 2024,
15, 6269-6284
Electrocatalytic functional group conversion-based carbon resource upgrading
Received
9th January 2024
, Accepted 23rd March 2024
First published on 2nd April 2024
Abstract
The conversions of carbon resources, such as alcohols, aldehydes/ketones, and ethers, have been being one of the hottest topics most recently for the goal of carbon neutralization. The emerging electrocatalytic upgrading has been regarded as a promising strategy aiming to convert carbon resources into value-added chemicals. Although exciting progress has been made and reviewed recently in this area by mostly focusing on the explorations of valuable anodic oxidation or cathodic reduction reactions individually, however, the reaction rules of these reactions are still missing, and how to purposely find or rationally design novel but efficient reactions in batches is still challenging. The properties and transformations of key functional groups in substrate molecules play critically important roles in carbon resources conversion reactions, which have been paid more attention to and may offer hidden keys to achieve the above goal. In this review, the properties of functional groups are addressed and discussed in detail, and the reported electrocatalytic upgrading reactions are summarized in four categories based on the types of functional groups of carbon resources. Possible reaction pathways closely related to functional groups will be summarized from the aspects of activation, cleavage and formation of chemical bonds. The current challenges and future opportunities of electrocatalytic upgrading of carbon resources are discussed at the end of this review.
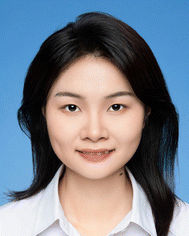 Di Si | Di Si is now a doctoral candidate at the School of Chemistry and Molecular Engineering, East China Normal University (ECNU) under the supervision of Prof. Jianlin Shi. Her research is focused on the electrocatalytic conversion of organic molecules to value-added chemicals. |
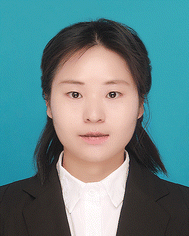 Xue Teng | Xue Teng received her PhD degree from Tongji University in 2021. She is now a postdoctor at East China Normal University (ECNU). Her research interest is in electrocatalysts for renewable energy. |
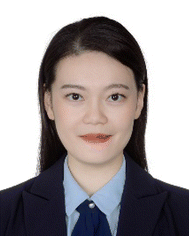 Bingyan Xiong | Bingyan Xiong received her PhD degree from East China Normal University (ECNU) under the supervision of Prof. Jianlin Shi. She has been being an Assistant Professor at Tongji University since 2021. Her research interests are focused on the design and synthesis of nanomaterials and the catalytic and biomedical performances of the materials for applications in energy conversion and nanomedicine. |
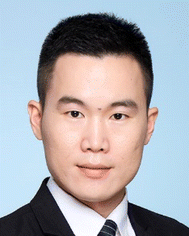 Lisong Chen | Lisong Chen received his PhD degree under the supervision of Prof. Jianlin Shi from the Shanghai Institute of Ceramics, Chinese Academy of Sciences (SICCAS) in 2016. He is now an associate professor at East China Normal University (ECNU). His research interest is in electrocatalysts for renewable energy. |
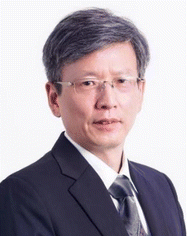 Jianlin Shi | Prof. Jianlin Shi received his PhD degree from the Shanghai Institute of Ceramics, Chinese Academy of Sciences (SICCAS). He is now a professor there and a member of the Chinese Academy of Sciences. His research areas include the synthesis of mesoporous-based nanomaterials and their catalytic and biomedical applications, and more recently, electrocatalysis. |
1. Introduction
The production of fuels, plastics, and pharmaceuticals on a large scale is greatly reliant on the capacity of the chemical industry. The chemical industry is inseparable from thermal catalytic processes, which play a fundamental role in converting various feedstocks into a diverse array of commodity chemicals. However, it heavily relies on finite fossil fuels as feedstocks and energy inputs, making it the largest energy consumer among all industrial sectors. To meet the growing demand for clean energy and address the environmental issues caused by industrial expansion, carbon peaking and carbon neutrality have gained significant attention worldwide. These topics have emerged as some of the most pressing concerns in current chemical and energy research.1–4 Consequently, the exploration of new methods for fuel and chemical synthesis is of utmost importance, with electrocatalysis being deemed as a highly potential avenue among them.5
Electrocatalysis is a green chemistry process that employs renewable and plentiful feedstocks such as air, water, and carbonaceous compounds, as well as renewable energy sources including wind, hydro, and solar power. This approach offers several advantages such as high activity and selectivity, 100% atom utilization efficiency, mild reaction conditions, and flexible modular production, making it a promising avenue for sustainable chemical synthesis.6 When powered by electricity, the electrocatalytic system can oxidize and reduce precursors at the anode and cathode respectively, without the need for chemical oxidants/reductants that may cause serious pollution. It has been confirmed that the electrocatalytic semi-hydrogenation conversion of acetylene to ethylene process is completely feasible through the technical and economic analysis.7 The well-controlled electric field acts as a strong nucleophilic/electrophilic agent, enabling high-precision activation of chemical bonds in the precursors for chemical reactions. The theoretically infinite redox range endows electrochemistry with the capability of oxidizing/reducing some of the most resilient compounds.
The common kinds of organic compounds include oxygen-containing organic compounds and hydrocarbons, which are classified according to their functional group. The functional groups commonly refer to atoms or clusters of atoms, including –C
C/C
C, –OH, –CHO, –COOH, –NO2, –SO3H, –NH2 and RCO–, which belong to unsaturated hydrocarbons, alcohols or phenols, aldehydes, carboxylic acids, nitro compounds or nitriles, sulfonic acid organics, amines, and amides, respectively. The distinct properties exhibited by these compounds arise from the varying functional groups they possess. Furthermore, functional groups serve as reactive centers in organic chemical reactions. The essence of electrocatalytic conversion of organic matter lies in the transformation of these functional groups at the catalyst–electrolyte interface, involving sequential multistep electron transfer reactions that encompass the cleavage and formation of chemical bonds. Hence, the electrocatalytic conversion process is intricately intertwined with the functional group of the reactant, the applied potential and the electrocatalyst.8,9 Among these, electrocatalysts have garnered significant attention and are widely studied.10–13 Strategies to enhance their electrocatalytic performance are rapidly expanding, such as doping, atomic dispersion, nanostructures, and heterointerface engineering, all of which show effective utilization of the intrinsic atoms involved. It makes a significant contribution to the activity and selectivity of electrocatalysts.
Although exciting progress has been achieved and even some reviews have been reported in this area recently, especially with a focus on exploration of valuable anodic oxidation or cathodic reduction reactions one by one, however, the reaction rules of these reactions are still missing and how to systematically find or design novel reactions in batches is still challenging. The properties and transformations of functional groups, which are critically important for carbon resource conversion reactions, may provide an approach to achieve the above goal.14 In this review, the properties of functional groups are described and the reported electrocatalytic upgrading reactions are summarized in four categories based on the classification of functional groups of carbon resources (Fig. 1): (i) electrocatalytic upgrading of alcohols, (ii) electrocatalytic upgrading of carbonyls, (iii) electrocatalytic upgrading of ethers, and (iv) electrocatalytic upgrading of hydrocarbons. Possible reaction paths for one functional group will be summarized from the aspects of activation, cleavage, and formation of chemical bonds. The current challenges and related prospects will be discussed, with the aim of benefiting the progress of electrocatalytic upgrading of carbon resources.
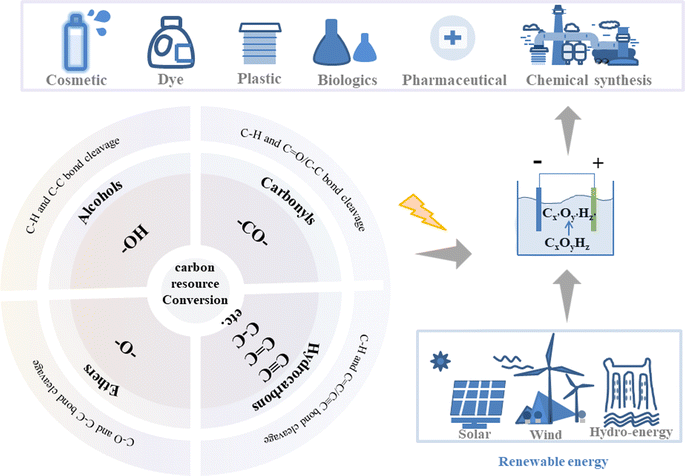 |
| Fig. 1 The illustration of electrocatalytic upgrading of carbon resources based on the classification of functional groups to generate commodity chemicals. | |
2. Electrocatalytic upgrading of alcohols
The hydroxyl group is the pivotal functional group of alcohols, playing a crucial role in various electrocatalytic conversion reactions and the properties of the hydroxyl group are crucial in determining the conversion efficiency of alcohols. The presence of a lone pair of electrons on the oxygen atom in the hydroxyl group enables it to attack positively charged atoms, which allows alcohols to undergo nucleophilic substitution reactions. Additionally, the electron-withdrawing induction effect of the hydroxyl group enhances the reactivity of the β-H in alcohols, favoring β-H elimination reactions. Moreover, alcohols are susceptible to oxidation reactions when hydrogen is present on their α-C (Fig. 2a). The alcohol oxidation reaction has attracted significant attention due to its potential applications in direct alcohol fuel cells, the conversion of biomass derivatives, and fine-chemical synthesis. Recently, electrocatalytic upgrading of various alcohols has been reported for synthesizing value-added products.15–17 In an electrocatalytic system, the electrooxidation of alcohol typically occurs in an aqueous electrolyte, and thus high purity hydrogen can also be obtained at the cathode, which is a hot topic. According to the number of hydroxyl groups contained in the molecule, alcohols can be divided into monohydric alcohols, diols and polyols.
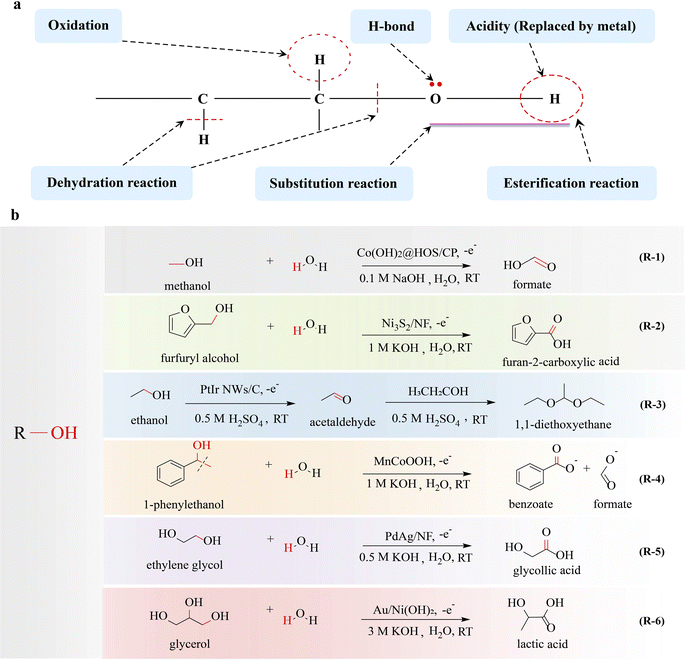 |
| Fig. 2 Electrocatalytic upgrading of alcohols. (a) The illustration of chemical properties of alcohols, and (b) the reports on alcohol electrocatalytic upgrade. | |
2.1. Electrocatalytic upgrading of monohydric alcohol
Many studies have been reported on the production of high-value chemicals by electrocatalytic upgrading of monohydric alcohol focusing on the C–H breakage and nucleophilic substitution at the α-C.
2.1.1. Transformation of the C–H bond.
2.1.1.1 Monohydric alcohols with one-carbon atoms.
Methanol is not only a significant renewable and clean fuel, but also serves as a fundamental organic raw material for the production of diverse organic products, including formate, methylamine, and dimethyl sulfate. Fu et al.18 converted methanol to formate by eletrooxidation (MOR: CH3OH + 4OH− → HCOOH + H2O + 4e−) (R-1 in Fig. 2). Benefiting from the efficient electrocatalyst Co(OH)2@HOS/CP, the elimination of α-H resulted in C–H bond breakage, followed by OH− attack on the α-C, forming a C
O bond and formate was obtained. Sulfurization can regulate the electronic structure of active sites, optimizing the adsorption energy of OH− and intermediates, which facilitates electron transfer from the antibonding orbital of metal–S bonds to metal–O bonds, thereby promoting C
O bond formation. In electrocatalysis, CO adsorption often acts as a poison or intermediate that causes poisoning, while the adsorption behavior of CO can be modulated by the rational design of electrocatalyst. Koper's group19 has concluded that CO adsorbed on an Au(111) surface promotes the electrocatalytic oxidation of methanol via facilitation of α-H elimination. It has been confirmed that the adsorbed CO alters the electrostatic surface potential, thereby enhancing the adsorption of OH− on the hexagonal gold surface. This induces an enhancement in the electrostatic bonding component of OH−, which serves as the active species triggering C–H bond cleavage and C
O bond formation. Through co-catalysis, complex chemicals can be obtained. The conversion of methanol and ammonia to formamide was reported by Zhang's group.20 The electrochemical reaction achieved a formamide selectivity of 74.26% and a faradaic efficiency of 40.39%, in which the C–H bond in methanol is broken, leading to the formation of an aldehyde-like intermediate through methanol electrooxidation. Subsequently, this intermediate undergoes a nucleophilic attack by NH3, resulting in the formation of the C–N bond (cleavage of the C–H bond and O–H band).
2.1.1.2 Monohydric alcohols with multi-carbon atoms.
Inspired by the successful conversions of methanol, the upgrading of monohydric alcohols with more than one carbon atom has also been investigated. The electrocatalytic conversion of ethanol, isopropanol, n-propanol, benzyl alcohol, phenylethyl alcohol, and furfuryl alcohol to acetate, ethyl acetate, acetone, propionaldehyde, propionic acid, benzaldehyde, benzoic acid, phenylacetic acid, and 2-furoic acid has been reported, which is also focused on the cleavage of the C–H bond.21–25 Vizza21 has reported the electrooxidation of ethanol to acetate, in which the C–H bond is broken and a C
O bond is formed. A net profit analysis shows that electrocatalytic production of sodium acetate through ethanol oxidation is highly rewarding. The electrocatalytic conversion of cyclic polycarbonate alcohols into polycarbonate carboxylates is a crucial method, where the hydroxyl functional group is oxidized to carboxyl groups during the reaction process. You et al.26 prepared Ni3S2/NF as an electrocatalyst at the anode for the oxidation of biomass derived organic chemicals (R-2 in Fig. 2). The electrocatalytic oxidation of furfural alcohol and benzyl alcohol can effectively produce 2-furoic acid and benzoic acid with high efficiency and selectivity. With hydrogen on the α-C of these organic chemicals, it is easy for C–H bond cleavage and C
O and C–O bond formation. In addition to being converted into carboxylates, alcohols also serve as important precursors for the synthesis of aldehydes and ketones.27
Under uncontrolled oxidizing conditions, alcohols undergo oxidation to produce aldehydes, which are widely used in the chemical, wood, and textile industries.28 Conventional methods for oxidizing alcohols to aldehydes rely on the use of environmentally unfriendly stoichiometric oxidants such as chromium, manganese, and ruthenium salts. Electro-oxidation offers a sustainable and efficient alternative to traditional thermocatalytic methods. Due to the fact that aldehydes are susceptible to over-oxidation to acid, the selective oxidation of alcohols to corresponding aldehydes remains a significant challenge although numerous electrocatalysts have been developed.29–31 Currently, alkaline electrolyte is the most commonly utilized medium for the oxidation of alcohols; however, aldehydes are susceptible to condensation reactions and over-oxidation into carboxylate at high pH. It is considered that the neutral/near-neutral electrolyte would overcome these issues and enable selective oxidation of alcohols to aldehydes. It has been demonstrated17 that the electrooxidation of 5-hydroxymethyl furfural to 2,5-furandicarboxaldehyde in a neutral medium is more favorable than that in a alkaline medium with Ru1–NiO as the electrocatalyst, in which the C–H bond is broken to form a C
O bond. Wang's group32 realized the selective electrooxidation of alcohol to aldehyde on NiO by adjusting the local microenvironment and effectively separating the generated aldehyde from the reaction system. The conversion of 5-hydroxymethyl furfural to 2,5-furandicarboxaldehyde is achieved with high selectivity, which originates from the inhibition of aldehyde hydration by a salting-out effect, which specifically breaks the C–H bond.
Efficient cascade reactions are critical for the production of carbon-chain growth chemicals. Guo and coworkers33 reported the use of PtIr nanowires as catalysts for electrolytic production of multicarbon-1,1-dimethoxyethane (DEE) from the oxidation of ethanol via α-H elimination, in which electrocatalytic reaction was integrated with a condensation reaction driven by acid-catalysis (R-3 in Fig. 2). First, ethanol was dehydrogenated (C–H bond cleavage) and converted to acetaldehyde. Then, the acetaldehyde was transformed into DEE through acid-catalyzed acetalation (C–O–C bond formation), in which the shared electron pair between carbon and oxygen in the C–O bond was biased towards oxygen, enabling the nucleophilic substitution reaction to occur on the α-C of acetaldehyde, resulting in acetylation. The PtIr NWs/C catalyst shows excellent activity, achieving a high Faraday efficiency of 85% for DEE. Compared with other reactions where the carbon-chain is reduced or unchanged, the carbon-chain growth reaction is more difficult, and the reported literature is very rare.
2.1.2. Transformation of the C–C bond.
2.1.2.1 Monohydric alcohols with multi-carbon atoms.
In the electrocatalytic conversion of polycarbon monoalcohol, not only C–H bonds are broken but also C–C bonds can be cleaved to form new chemical bonds. Duan and coworkers34 synthesized an Mn-doped CoOOH electrocatalyst that effectively upgrades 1-phenylethanol into benzoate and formate through the cleavage of C(OH)–C bonds by electrocatalytic oxidation (R-4 in Fig. 2). During the reaction, 1-phenylethanol reacts with OH* species on the surface of MnCoOOH featuring the cleavage of C–H and C–C bonds and the formation of a C
O bond. It is noteworthy that an oxidative C–C cleavage process takes place to generate benzoate and formate as the final products. This method has the potential to be generalized to a range of carboxylates containing C(OH)–C or C(O)–C motifs, enabling the production of carboxylic acids with high yield. The cleavage of the C–C bond is possible due to the activity of the hydrogen in the hydroxyl functional group and the activity of the β-H. Duan and coworkers35 also reported an efficient electrocatalytic strategy for cleavage of the Cα–Cβ bond of β-O-4 linkage in 2-phenoxy-1-phenylethanol, exhibiting high yields for the products of aromatic aldehydes and phenols. The oxidative cleavage of the C(OH)–C bond to produce carboxylates is of significance for the upgrading of carbon resources.
2.2. Electrocatalytic upgrading of diols
In addition to monohydric alcohols, some exciting progress has been achieved in the electrocatalytic conversion of diols in recent years. Value added chemicals such as formic acid, acetic acid, 3-hydroxypropionic acid, and malonic acid have been obtained from the electrocatalytic conversion of ethylene glycol, 1,3-propanediol, 1,2-propanediol, 1,4-butanediol and 1,5-pentanediol with high efficiencies.16,36–41
2.2.1. Transformation of the C–C bond.
Ethylene glycol, the most representative diol with active α-H, is an important raw chemical that can be obtained from recycled plastics. Electrocatalysis offers a sustainable approach for the production of high-value chemicals by the oxidation of ethylene glycol. The cleavage of C–H and C–C bonds is prone to occur in diols, with the latter bond considered particularly crucial. Duan and coworkers42 developed a nickel-modified cobalt phosphide (CoNi0.25P) electrocatalyst for ethylene glycol oxidation, which achieved high Faraday efficiency of formate (>80%). Detailed characterization studies have revealed that the in situ formed low-crystalline metal oxy(hydroxide) serves as the active site for ethylene glycol oxidation, potentially explaining the high selectivity towards the targeted formate product.
2.2.2. Transformation of the C–H bond.
The production of C2+ organic chemicals without undergoing C–C cleavage during the electrocatalytic process is considered more attractive due to significantly enhanced product values. Noble metal-based catalysts exhibit high selectivity towards C–C bond retention products in the electrooxidation of alcohols.11 In a typical reaction, noble metal surfaces facilitate the electrooxidation of H2O or OH− at low potentials, generating reactive oxygen species, which are commonly found in the form of adsorbed hydroxyl (OH*) groups. The OH* attacks alcohol, leading to C–H cleavage without causing C–C bond breakage; therefore value-added chemicals such as aldehyde, ketone, and carboxylic acid can be obtained. Our group43 reported an electrocatalytic strategy for upcycling ethylene glycol to glycolic acid by applying PdAg/NF as the electrocatalyst, in which the hydroxyl group is selectively oxidized while the C–C bond is maintained (R-5 in Fig. 2). PdAg/NF accelerates the ethylene glycol oxidation kinetics, which exhibits impressive ethylene glycol oxidation electrocatalytic performance with a high Faraday efficiency of 92% for glycolic acid. First, ethylene glycol undergoes dehydrogenation (C–H cleavage) due to the activity of α-H, to form intermediate 2-hydroxyacetyl species (O = *CCH2OH). Then OH− species attack α-C of O = *CCH2OH through nucleophilic substitution and glycolic acid is obtained. The activity of α-H and α-C serves as a crucial determinant for the cleavage of the C–H bond and the formation of C
O/C–O bonds. Additionally, the β-H activity facilitates the formation of C
C bonds, leading to the production of olefinic compounds. The electrocatalytic oxidation of 1,3-propanediol, which has two β-H atoms, is crucial for the formation of C
C bonds. Miller44 developed protocols to convert 1,3-propanediol into acrylate with Pd/C–CeO2 as the electrocatalyst exhibiting a high selectivity of 77%. The formation of acrylate requires the elimination of both α-H, β-H combined with C–O bond cleavage to form the C
O and C
C bonds. The electrocatalytic upgrading of diols to produce high-valued products with excellent selectivity is promising.27
2.3. Electrocatalytic upgrading of polyhydroxy alcohols
In addition to mono- and diols, electrocatalysis has also been used for the upgrading of polyhydroxy alcohols. Unlike mono- and diols, the conversion of polyols is more complex and a diversity of products can be obtained. A series of valuable C3, C2, and C1 fine chemicals including tartronic acid (TA), lactic acid (LA), dihydroxyacetone (DHA), glyceraldehyde (GLYD), glyceric acid (GLYC), hydroxypyruvic acid (HYDP), oxalic acid (OA) and formic acid (FA) can be obtained by electrocatalytic upgrading of polyhydric alcohols, such as glycerol and sorbitol.45–48
2.3.1. Transformation of the C–C bond.
The breakage of the C–C bond is prone to occur in the oxidation of polyhydroxy alcohols. The state-of-the-art C–C bond cleavage of polycarbon molecules heavily relies on energy consumption thermocatalysis, and the selectivity of products is still unsatisfactory. Electrocatalysis offers precise control over the active site, ensuring high selectivity for C–C bond cleavage. Consequently, the efficient electrocatalytic conversion of polycarbon molecules into smaller chemical molecules holds great promise, yet it still poses significant challenges that need to be addressed. Under the catalysis of non-noble metal nickel–molybdenum–nitride nanoplates supported on carbon fiber cloth (Ni–Mo–N/CFC), C–H, C–O and stable C–C bonds of glycerol were broken successfully and value-added chemical, FA, was produced with a high Faraday efficiency of 95%.49 The glycerol-to-FA begins with the breakage of C–H and H–O bonds and the formation of a C
O bond, and then the obtained glyceraldehyde is oxidized to FA and glycolaldehyde. With the breakage of the C–C bond and nucleophilic substitution of α-C, glycolaldehyde is converted to FA. The electrocatalytic upgrading of sorbitol has been reported by No'e Arjona,50 and various products are obtained, including glycerol, ethylene glycol, formic acid, and γ-butyrolactone. In this electrocatalytic system, the PdxAuy/C catalyst promotes C–C bond breakage, which is certainly beneficial for the production of series of value-added molecules. Cascade catalysis of electrocatalysis and acid catalysis have been exploited for C–C bond breakage. Li and co-workers51 exploited polyoxometalates (H6[PV3Mo9O40]) as the redox anolyte for the anodic oxidation of glucose (GOR), in which the C–C bond was broken to produce FA with a yield of 62.5%, while H2 was evolved continuously at the cathode. During the reaction, H6[PV3Mo9O40] plays a key role in the breakage of the C–C bond and acid catalysis is an important step for the final product generation. First, the acid catalyzes glucose to produce fructose by isomerization. Following the electrocatalysis reaction, decarboxylation of the α-hydroxy acid takes place, which subsequently leads to the breakage of the C–C bond in the α-hydroxy aldehyde. This process results in the formation of low-carbon number intermediates such as arabinose, glycolaldehyde, glyceraldehyde, and glyoxal, which are further catalytically oxidized to produce the final product FA.
2.3.2. Transformation of the C–H bond.
Though high value-added formate has been achieved with high selectivity by electrocatalytic upgrading of glycerol, C3 products with much higher value through C–H bond cleavage without C–C cleavage are also desired. With the aid of Au/Ni(OH)2 catalysts, Duan's group52 achieved a high selectivity of 77% for the conversion of glycerol to lactic acid. Glycerol is easier to form alkoxide via O–H bond cleavage in alkaline electrolyte. The alkoxides adsorbed on Au/Ni(OH)2 transfer electrons to the catalyst, resulting in the formation of a nucleophilic species, (HOCH2)2CHO*. Simultaneously, electrophilic OH* is generated and attacks the α-C of (HOCH2)2CHO* to generate DHA ((HOCH2)2CO*) along with C–H cleavage. Finally, DHA undergoes base-catalyzed dehydration and Cannizzaro rearrangement to produce lactic acid (LA) (R-6 in Fig. 2).
3. Electrocatalytic upgrading of carbonyls
Carbonyl is the functional group as well as the active site of aldehydes and ketones. The properties of carbonyl are very important for the conversion of aldehydes and ketones. The carbon–oxygen π bond is susceptible to electron-deficient substances, leading to reduction and addition reactions. Additionally, aldehydes and ketones undergo nucleophilic addition reactions due to higher electronegativity of oxygen compared to carbon. Conversely, carbon carries a slight positive charge, making it susceptible to nucleophilic attack. The electron-withdrawing effect of the carbonyl group activates the α-H, favoring enolization reactions in aldehydes and ketones (Fig. 3a).
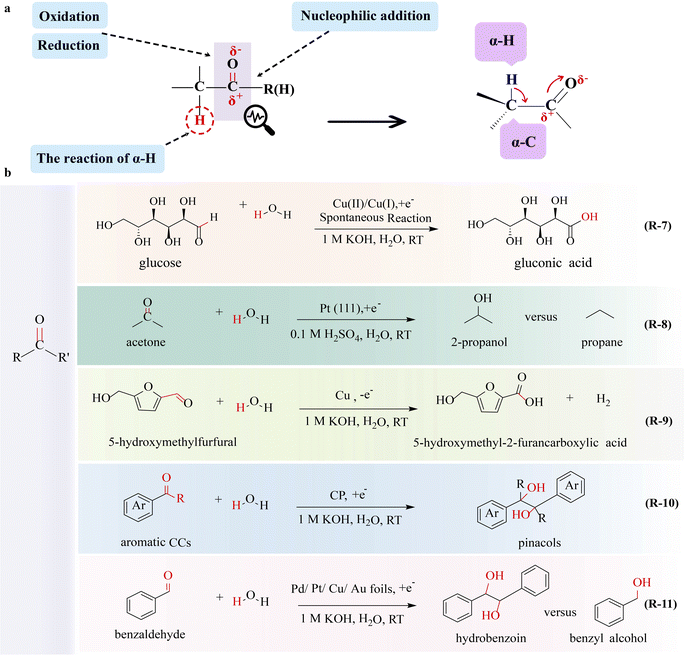 |
| Fig. 3 Electrocatalytic upgrading of carbonyls. (a) The illustration of chemical properties of aldehydes and ketones, and (b) the reports on carbonyl electrocatalytic upgrade. | |
3.1. Electrocatalytic upgrading of saturated carbonyls
Due to the presence of active α-H and electropositive carbon which is susceptible to nucleophilic attack, oxidation reactions and nucleophilic addition reactions are successfully applied for the electrocatalytic conversion of aldehydes and ketones. The oxidation of aldehydes and ketones is crucial for the synthesis of fine chemicals. In an electrocatalysis system, the electrooxidation of aldehydes and ketones usually takes place in an H-type electrolytic cell, in which the reduction of aldehydes and ketones by the cathode is hindered.
3.1.1. Transformation of the C–H or/and C
O bond.
Significant progress has been reported in the production of various chemicals through the electrocatalytic reaction of saturated aldehyde and ketone focusing on the C–H breakage and nucleophilic substitution at the α-C. Formate, a product of formaldehyde oxidation (FOR), is a valuable chemical. During the reaction, nucleophilic reagents tend to attack positively charged carbon, leading to the cleavage of the C
O π-bond and the formation of two σ-bonds. For two-electron-FOR electrolysis, formaldehyde undergoes partial oxidation to produce both formate and hydrogen. Sun's group53 reported the partial electrooxidation of formaldehyde assisted-HER system by using Cu3Ag7 as an anode electrocatalyst, which could concurrently produce formic acid and hydrogen with high Faraday efficiency. During the reaction, C–H and C
O bonds of the aldehyde group are broken to form a C–OH σ-bond with the aid of the catalyst. The stability of the electrocatalyst is very important for electrocatalytic reactions and it will be a promising strategy for utilizing in situ generated hydrogen at the anode to activate the electrocatalyst. Zou's group54 explored the conversion of formaldehyde to both formate and hydrogen at the anode. Benefiting from the in situ generated hydrogen at the anode, the Cu2+ in electrocatalyst Cu2O is reduced to Cu+, which significantly enhances the catalytic performance of Cu2O, exhibiting nearly 100% selectivity for formate and hydrogen production.
The electrooxidation of multivalent metals is prevalent in electrochemistry such as rechargeable ion batteries and electroplating,55 in which a mediator (or a redox catalyst) is used as the catalyst undergoing electron transfer to form a reactive intermediate. Then this reactive species oxidizes or reduces a substrate molecule to produce the target material homogeneously in an indirect electrolytic process with high-cost effectiveness and energy savings. A novel strategy of glucose-assisted Cu(I)/Cu(II) redox reaction to reduce the overpotential of the GOR producing GNA has been reported,56 in which Cu2O is applied as the anode catalyst (R-7 in Fig. 3). During the electrolysis, Cu2O is converted to Cu(OH)2(Cu(II)) by losing electrons from Cu(I), and then Cu(OH)2 spontaneously interacts with glucose to form Cu(I) and GNA, which allows the continuous electrooxidation of Cu(I) and completes the redox cycle of Cu(I)/Cu(II). This system exhibits a high current density of 100 mA cm−2 at 0.92 V and specifically activates the aldehyde group for GNA production, without affecting the hydroxyl group.
Due to the presence of a C
O π bond, the reduction of aldehydes and ketones to alcohols and alkanes has garnered the interest of researchers. Steinberg and co-workers57 achieved the electrocatalytic hydrogenation of formaldehyde to methanol with a faradaic efficiency exceeding 90%, in which the C
O bond is broken to form a C–O bond. Koper and co-workers58 demonstrated that 2-propanol and propane can be obtained by acetone electroreduction over platinum single-crystal electrodes (R-8 in Fig. 3). The structural sensitivity of the C–C, C
O, C–O, and C–H bonds at platinum single-crystal electrodes allows for the manipulation of specific chemical bonds through the application of varying potentials. The presence of aldehyde groups in glucose makes the reduction reaction feasible. There are some research studies on the production of mannitol and sorbitol by the electrochemical reduction of glucose, in which the C
O bond is converted to a C–O/C–H bond. For example, Roesky and co-workers59 reported the generation of sorbitol by the electrolysis of glucose at a constant potential of 4.5 V with high conversion over 90% and yield of 80%, in which a hydrogen-storage alloy was used as a catalytic reduction electrode. The hydrogen-storage alloy electrode is highly active for the cleavage of the C
O bond affording a hydrogenation product with the C–O/C–H bond.
The conversion of FA, a promising product from CO2 industrial utilization, plays a crucial role in the effective utilization of carbon resources. It has been reported that the co-reduction of FA and nitrite leads to the production of high-value formamide with a selectivity of 90.0%.60 During the reaction, HCOO− and nitrite are reduced to *CHO and *NH2, respectively, which couple with each other to form the key C–N coupling, leading to high-performance formamide electrosynthesis on the catalyst low-coordinated Cu.
3.1.2. Transformation of the C–C bond.
The electrochemical oxidation of ketone into acid by C–C bond cleavage has also been reported. Duan's group61 reported the oxidation of cyclohexanone to adipic acid with a high faradaic efficiency of 93% wherein a nickel hydroxide catalyst modified using sodium dodecyl sulfonate (SDS) in the interlayer (Ni(OH)2-SDS) served as the catalyst. During the reaction, the catalyst of Ni(OH)2-SDS facilitates the breakage of C–H and C–C bonds, and thus adipic acid is obtained. The oxidation of aldehydes and ketones to acids is one of the most common strategies in electrocatalytic reactions.
3.2. Electrocatalytic upgrading of unsaturated carbonyls
Furthermore, the electrocatalytic reactions of unsaturated aldehydes and ketones, such as cinnamaldehyde, furfural, 5-hydroxymethylfurfural (HMF) and acetophenone, are more interesting.62,63 Especially noteworthy is that HMF, which is the dehydration product of C6 carbohydrates, can serve as a versatile platform precursor for synthesizing a diverse array of commodities, including fine chemicals, plastics, pharmaceuticals, and liquid fuels.
3.2.1. Transformation of the C–H bond.
For instance, electrocatalytic selective oxidation of HMF (HMFOR) through C–H bond activation has been achieved to produce a series of valuable chemicals, such as 2,5-furandicarboxylic acid (FDCA), 5-hydroxymethyl-2-furancarboxylic acid (HMFCA), dialdehyde 2,5-diformylfuran (DFF), and 5-formyl-2-furancarboxylic acid (FFCA).64,65 It has been demonstrated that the HMFOR to FDCA is possible with a yield of 96.2% and faradaic efficiency of 93.7% by using H2O as the oxygen and hydrogen sources under the catalysis of CuO–PdO.66 The synergetic catalytic effect between CuO and PdO facilitates the breakage of C–H in the aldehyde group, forming FDCA. In addition to the aldehyde group, unsaturated aldehydes often contain other functional groups such as hydroxyl groups, unsaturated carbon–carbon double bonds, triple bonds, etc. According to the properties of functional groups, a specific substrate can be selectively converted to a target product at a certain potential. Wang's group67 adopted a simple Cu catalyst that effectively promotes the breakage of the C–H bond in the aldehyde group of HMF, leading to the concurrent production of HMFCA and hydrogen, in which the –CHO group is transformed into –COOH at relatively low potentials (R-9 in Fig. 3). By varying the potential, the aldehyde group can be selectively oxidized without affecting the hydroxyl group, allowing for the preferential conversion of HMF to HMFCA and hydrogen at the anode. To enhance the kinetics of these electrochemical reactions, high-performance catalysts are essential. Co-based catalysts have emerged as promising candidates for the HMFOR. During the electro-oxidation process, the formation of high-valence Co ions (Co3+ and Co4+) is observed, and their roles in the HMFOR are distinctly different. Deng et al.65 reported selectivity-tuned HMFOR by varying the oxidation states of CoOxHy. Co3+ generated at a quite low potential can act as an oxidant exclusively towards the transformation of –CHO into –COOH without oxidizing the hydroxyl group. In contrast, Co4+ generated at higher applied potentials plays a crucial role in initiating the oxidation of the hydroxyl group present in HMF molecules. Therefore, HMFCA and FDCA with high faradaic efficiency and selectivity can be obtained by regulating the valence state of Co.
3.2.2. Transformation of the C
O bond or/and unsaturated bond.
In particular, the electrocatalytic hydrogenations of HMF to 2,5-dihydroxymethylfuran (DHMF), 2,5-bis (hydroxymethyl)furan (BHMF) and 2,5-hexanedione (HD) have been verified to be feasible.68–71 These reactions are often accompanied by a ring–opening reaction, in which the C
O, C
C, and O–C–O bonds of the ring are broken. The conversion of HMF to HD involves the reduction of both hydroxyl and aldehyde groups, as well as the opening of the furan ring. Choi and coworkers71 demonstrated electrochemical conversion of HMF to HD, in which water and zinc were used as the hydrogen source and the catalytic electrode respectively. During the reaction, hydrogenolysis, Clemmensen reduction and furan ring opening (cleavage of C
C and C–O–C bonds) take place, followed by the formation of C–H and C
O bonds. The opening reaction of the furan ring involves the ring opening between C and the furan oxygen in the C–O–C bond by nucleophilic attack. The Faraday efficiency and selectivity of HD reached up to 72.4% and 81.6%, respectively, during the reduction of HMF. Holladay's group72 reported the electroreduction of benzaldehyde to benzyl alcohol by using a palladium electrocatalyst, in which the C
O bond is activated to form a C–OH bond. Nevertheless, innovative electrocatalytic systems are necessary to reduce energy input. Recently, a novel bifunctional Zn–organic battery has been reported by Chen group73 that simultaneously achieves enhanced electricity output and electrochemical reduction of a range of biomass aldehyde derivatives. With the help of a Cu NS/Cu foil electrocatalyst, the C
O bond of furfural is broken to form C–O and C–H bonds, producing furfural alcohol with a 93.5% conversion ratio and 93.1% selectivity.
Unsaturated aldehydes and ketones typically contain multiple functional groups, whose presence can influence the activity and selectivity of electrocatalytic reactions. Koper's group74 studied the electrochemical hydrogenation of functionalized ketones on platinum single-crystal electrodes. By defining the adsorption mode on the electrode, a new concept for electrocatalysis has been proposed that a secondary inert functional group can affect the reactivity of the other functional group and break the specific bond, and thus the desired product is obtained. The catalytic activity of an electrocatalyst crucially determines the reduction performance of unsaturated aldehydes and ketones, enabling preferential reduction of specific groups. Coincidentally, Zhang et al.62 designed CoS2 and CoS2−x nanocapsules (NCs) for the electrocatalytic hydrogenation of cinnamaldehyde with excellent performance. The specific adsorption of the styryl block on pristine CoS2 NCs is conducive to the prioritized activation of the C
C bond, which favors the selective formation of half-hydrogenated hydrocinnamaldehyde with a 91.7% selectivity. The preferential adsorption of the C
O group, facilitated by sulfur vacancies in defective CoS2−x NCs, results in the activation of both C
C and C
O bonds, which favors the production of fully hydrogenated hydrocinnamyl alcohol with a selectivity of 92.1%. When hydrocinnamaldehyde is the main hydrogenation product of cinnamaldehyde, flat adsorption of cinnamaldehyde is dominant on the CoS2 NCs; while when hydrocinnamyl alcohol is the main hydrogenation product of cinnamaldehyde, vertical adsorption of cinnamaldehyde is dominant on the CoS2−x NCs. Furthermore, the hydrogenation method is also effective for other α,β-unsaturated aldehydes, demonstrating the versatility of the method.
The feasibility of preparing carbon-enhancing compounds through the combination of electrocatalytic reductions of unsaturated aldehydes and ketones with radical polymerization has been demonstrated. Zhang's group75 reported the electrocatalytic coupling reactions of benzaldehyde to pinacols (R-10 in Fig. 3). As electrocatalysts play a crucial role in enhancing selectivity, a variety of catalysts have been screened, and carbon paper has been identified to provide 99% selectivity, 96% faradaic efficiency, and a reaction rate of 0.6 mmol cm−2 h−1 towards pinacols. During the reaction, the C
O bond of benzaldehyde is activated, leading to the generation of a critical ketyl radical intermediate. The dimerization of two neighboring ketyl radicals on the CP surface results in the formation of a pinacol product (C–C coupling) that is subsequently released. The CP plays a crucial role in the selective production of pinacols; otherwise both benzyl alcohol and pinacols will be produced. To enhance the kinetics of these electrochemical reactions, further improvement of the electrocatalytic performance of the catalyst is necessary. Excitingly, Xu and co-workers76 proposed that the catalytic performance in producing and stabilizing ketyl radical intermediates is important for mediating the C–C coupling chemistry of aldehydes and ketones. The electrocatalytic reduction of benzaldehyde serves as a model reaction to investigate reductive C–C coupling to produce a diol product, in which the Cu catalyst stabilizes the ketyl radical, thereby facilitating the C–C coupling of benzaldehyde (R-11 in Fig. 3). By employing these radical cascade reactions, the objective of increasing the molecular weight of substrates through C–C bond formation can be achieved.
4. Electrocatalytic upgrading of ethers
The ether group is the functional group and the active site of the ether. As the electronegativity of oxygen is greater than that of carbon, the carbon–oxygen bond can be heterogeneously cleaved under appropriate conditions, resulting in a nucleophilic substitution reaction on the saturated carbon atom. Additionally, the α-C of ethers is susceptible to oxidation when hydrogen is present due to its electronic effect. The properties of the ether group are very important for the conversion of ethers and the electrocatalytic upgrading of ethers focusing on lignin (Fig. 4a).
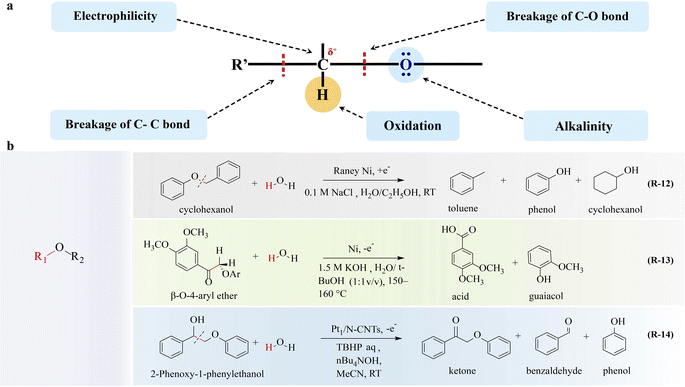 |
| Fig. 4 Electrocatalytic upgrading of ethers. (a) The illustration of chemical properties of ether, and (b) the reports on ether electrocatalytic upgrade. | |
4.1. Electrocatalytic upgrading of lignin
The lignin molecule is randomly bonded by three phenylpropane structural units, guaiacyl propane, syringyl propane, and p-hydroxyphenyl propane, of which the C–O ether bond accounts for about 2/3, and the rest are C–C bonds, including β-O-4, α-O-4, 4-O-5, β-β, β-5, 5-5, β-1 bonds, etc.41,77,78 The breakage of these linkages is possible depending on the different electronegativities of carbon and oxygen. By cleaving these linkages, small molecule depolymerization products containing various functional groups such as aromatic groups, methoxy groups, hydroxyl groups, carbonyl groups, and carboxyl groups can be obtained. Due to the C/H ratio of these small-molecule compounds is similar to that of petroleum, they have great application potential in the fields of high-quality liquid fuel and high-value-added chemical production. Lignin conversion is regarded as one of the most promising strategies for carbon resource upgrading. The conversion of lignin into chemical and fuel products necessitates effective methods for lignin depolymerization. Electrochemical strategies play a crucial role in enhancing lignin depolymerization and upgrading.
4.1.1. Transformation of the C–O bond.
The electrocatalytic method for lignin degradation and upgrading offers an attractive route for producing valuable cyclic and aromatic compounds such as phenol, toluene, cyclohexanol, and cyclohexanone.41,79,80 To obtain the above-mentioned products, the kinetics of aryl ether bond (C–O–C) hydrogenolysis should be faster than that of aromatic ring (C
C) hydrogenation;81 therefore the adsorption strength and configuration of reactants and intermediates should be optimized. Brossard82 reported that the electron-rich Ni electrocatalyst exhibited high efficiency toward cleavage of the C–O bond in aryl ethers (R-12 in Fig. 4). Also, the type and position of various functional groups (e.g., methoxy, methyl, phenolic, and aniline groups) affecting the electronic structures of aromatic rings and O–C–O are decisive to the catalytic conversion process; thus through reasonable functional group modification, the C–O bond in the ether can be broken to obtain high value products.
4.1.2. Transformation of the C–C bond.
Selective cleavage of C–C linkages is a crucial step, but it remains a challenge in lignin degradation for obtaining value-added aromatic compounds. In particular, the C–C bond typically has a higher dissociation energy compared to the C–O bond in lignin, hindering the selective cleavage of the C–C bond. In this regard, various strategies, such as hydrolysis, pyrolysis, reduction, and oxidation, have been developed.83,84 Electrocatalytic oxidation is a potentially promising technique to cleave the C–C linkage while preserving the aromatic ring structure, transforming lignin into highly functionalized monomeric aromatic compounds.14,85–88 Pardini and co-workers89 reported electrocatalytic oxidation of a lignin β-O-4 dimeric model compound using a bulk Ni electrocatalyst. This study targeted the cleavage of the Cα–Cβ bond and achieved a 20.9% yield of aromatic aldehydes and carboxylic acids (R-13 in Fig. 4). A similar Cα–Cβ bond cleavage reaction was reported by the same group and Pt foil was explored as the anode electrocatalyst which delivered an improved yield of aromatic aldehyde.90 Alternatively, Rochefort and co-workers91 reported an indirect electrooxidation approach by using 2,2′-azinobis(3-ethylbenzthiazoline-6-sulfonate) (ABTS) as a redox mediator to facilitate Cα–Cβ bond cleavage with 32.5% yield of the aldehyde product.
To further elevate the conversion rate and selectivity of ether oxidation, electrocatalysts have gained great attention. Li and coworkers92 showed that Pt1/N-CNTs exhibited high activity and selectivity towards Cα–Cβ bond cleavage in β-O-4 model compounds (R-14 in Fig. 4). Pt1/N-CNTs achieves 81% yield of benzaldehyde arising from the atomically dispersed Pt–N3C1 sites, which facilitates the formation of a crucial Cβ radical intermediate. Then this intermediate promotes a radical/radical cross-coupling pathway, ultimately leading to the cleavage of the Cα–Cβ bond.
5. Electrocatalytic upgrading of hydrocarbons
In addition to the above organics, a lot of exciting progress has also been achieved in the electrocatalytic upgrading of other kinds of compounds, especially hydrocarbons.
5.1. Electrocatalytic upgrading of alkenes and alkynes
The carbon–carbon double/triple bond is the functional group as well as the active site of alkenes and alkynes (Fig. 5a). Compared to the carbon–carbon σ bond, the dissociation energy of the π bond in alkenes and alkynes is lower, leading to a more reactive π bond. It is necessary to study the properties of the carbon–carbon double/triple bond for the upgrading of alkenes and alkynes.
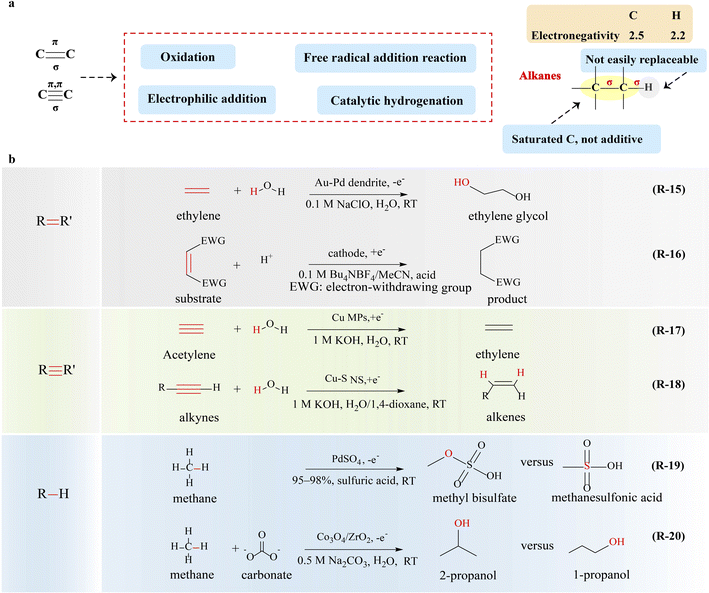 |
| Fig. 5 Electrocatalytic upgrading of alkenes, alkynes and alkanes. (a) The illustration of chemical properties of alkenes, alkynes, and alkanes, and (b) the reports on alkenes, alkynes and alkanes electrocatalytic upgrade. | |
5.1.1. Transformation of the C
C bond.
Selective oxidation of alkenes is a vital strategy for the production of aldehydes, alcohols, and epoxides, involving the breakage of C
C bonds and the formation of C–O bonds.93 Traditional thermal catalysis of alkenes suffers from serious environmental pollution and poor selectivity, and novel strategies are urgently needed. Recently, electrocatalytic routes using water as the sole oxygen atom source (*O or *OH) for several alkene oxidation reactions have been employed exhibiting high selectivity and yield.94–97 Sargent and coworkers94 reported one-step selective electrooxidation of ethylene to ethylene glycol in aqueous media by activating the C
C bond (R-15 in Fig. 5), in which a gold-doped palladium was used as a catalyst with a faradaic efficiency of 80%. Simultaneously, the counter electrode continuously generates hydrogen, when the selective electrooxidation of ethylene is coupled with the HER. In the selective electrooxidation of ethylene to ethylene glycol, the breakage of the C
C bond and the formation of the C–O bond are achieved through the successive transfer of two OH groups to ethylene. Apart from the design of the catalyst, the ion effect of electrolyte is also a key factor in the alternation of the reaction pathway and mechanism. The same group98 achieved the conversion of ethylene to ethylene oxide by utilizing Cl− as the redox mediator at the anode. The generated HOCl from Cl− reacts with ethylene to form ethylene chlorohydrin (HOCH2CH2Cl), which then undergoes a reaction with OH− to generate ethylene oxide, and chloride returns to the Cl− state for the next cycle of the reaction. This system employs chloride as the redox mediator at the anode, enabling the selective partial oxidation of ethylene to ethylene oxide with a faradaic efficiency of 70% and product specificity of 97%. The preparation of epoxides from propylene is also feasible. Epichlorohydrin, an essential industrial feedstock, has been reported by Zhang and co-workers through Br radical-mediated electrosynthesis.99 During the reaction, ˙Br, which activates and adds to the double bond of chloropropene, causes the formation of carbon radicals and then condenses with *OH from water oxidation to produce bromohydrin over the anode. Subsequently, hydrogen evolution on the cathode releases lots of OH− ions, which makes the electrolyte alkaline. Thus, HBr will be spontaneously eliminated from bromohydrin under alkaline condition to synthesize epichlorohydrin and release Br− for the next route. This strategy for the electrosynthesis of epichlorohydrin, utilizing NiCo2O4 NTs as the catalyst, demonstrates remarkable performance, achieving a faradaic efficiency of 47% and a rapid reaction rate of 1.4 mol h−1 gcat−1 when operated at a high current density of 100 mA cm−2. It exceeds the profitable target set by techno-economic analysis, thus validating its feasibility and profitability.
Due to the high reduction potentials of the C
C bond, the electrocatalytic reduction of the unactivated C
C bond is extremely difficult. Nevertheless, the C
C bond will become relatively fragile when substituted with electron-withdrawing groups, making it more susceptible to cathodic reduction.100 In the presence of a hydrogen donor, the hydrogenation of alkene can proceed smoothly. For example, electro-hydrogenation of activated alkenes has been reported by Tajima and co-workers,101 and polymer-supported sulfonic acid (Si-SO3H) (R-16 in Fig. 5) was used to promote the protonation step. Dimethyl maleate with two electron-withdrawing ester groups was used as the feedstock, which was converted to radical anions while the C
C bond was broken at the cathode. The added Si–SO3H promotes the protonation step in electroreductive hydrogenation of dimethyl maleate to minimize and even eliminate the competing coupling of the radical anion intermediate and its polymerization.102 Electrochemical dimerizations of trimethyl aconitate,103 methyl cinnamate,104 flavone,105 α,β-unsaturated ketones,106 and nitroolefins107 have also been reported by similar approaches. The electrocatalytic hydrogenation of alkenes using water as the hydrogen source shows significant advantages.
5.1.2. Transformation of the C
C bond.
Selective hydrogenation of alkynes to alkenes (HEA), in which the hydrogen source attacks the C
C bond to form the C–H bond, is a fundamental and significant transformation in synthetic chemistry.108,109 Traditional thermal catalysis of HEA suffers from serious pollution and poor selectivity, and thus it is of great significance to develop a more economical, energy-efficient, and highly selective route.110 Electrocatalytic HEA with water as the hydrogen source using a low-cost noble-metal-free catalyst is highly desirable. Deng's111 group investigated an efficient and selective electrocatalytic process for HEA using carbon-supported Cu microparticles (MPs) as the catalyst (R-17 in Fig. 5). During the reaction, electron transfer occurs from the Cu surface to the adsorbed acetylene, leading to preferential adsorption and hydrogenation of the acetylene over hydrogen formation and favoring the C
C cleavage and C–H formation through the electron-coupled proton transfer mechanism. A faradaic efficiency of 83.2% for the production of ethylene has been achieved. Nevertheless, though the electrocatalytic HEA process is able to produce alkenes, the faradaic efficiency and selectivity of this system are still unsatisfactory. Zhang and co-workers112 succeeded in electrocatalytic semi-hydrogenation of alkynes with over 99% selectivity using water as the hydrogen source when surface sulfur-doped and adsorbed Cu nanowire sponges (Cu-S NSs) were used as catalysts (R-18 in Fig. 5). In order to evaluate the practical feasibility of this electrochemical process, the same group7 conducted the techno-economic analysis of electrocatalytic HEA. Feverishly, results show that this process becomes profitable if the Faraday efficiency exceeds 85% at a current density of 0.2 A cm−2. A Cu nanoparticle catalyst with coordinatively unsaturated sites was designed for the reaction, which shows a high C2H4 yield rate of 70.15 mmol mg−1 h−1 and a Faraday efficiency of 97.7% at an industrially relevant current density of 0.5 A cm−2. This electrocatalytic selective hydrogenation strategy is highly versatile and can be applied to synthesize a wide range of alkenes and alkanes with high yield.
5.2. Electrocatalytic upgrading of alkanes
The cleavage of C–C and C–H bonds in alkanes requires about 347 kJ mol−1 and 308–435 kJ mol−1 of bond dissociation energy, respectively, and thus alkanes have great chemical stability and do not react with strong acids, strong bases, commonly used oxidants and reducing agents. In addition, the electronegativity difference between carbon and hydrogen is negligible in alkanes, thus there is no special affinity for nucleophiles or electrophiles (Fig. 5a). On the other hand, under the action of electricity, homolytic cleavage of bonds can occur to generate free radicals inducing radical reactions.
5.2.1. Transformation of the C–H bond.
The electrocatalytic oxidation of alkanes is a highly studied topic, which can lead to the production of various products, such as aldehydes, ketones, alcohols, and alkenes (e.g., CH3OH, HCHO, C2H4, C2H5OH, and C3H7OH) by electrocatalytic C–H bond breakage.113,114 Surendranath and coworkers115 introduced rapid electrocatalytic oxidation of methane to methanol precursors catalyzed by PdSO4 in concentrated sulfuric acid at high methane pressure (R-19 in Fig. 5). During the reaction, the conversion of PdSO4 into putative Pd2III,III species rapidly activates methane, overcoming a energy barrier of 25.9 (±2.6) kcal mol−1 to form methanol precursors methyl bisulfate (CH3OSO3H) and methanesulfonic acid (CH3SO3H) via concurrent faradaic and nonfaradaic reaction pathways, in which generation of electrophilic high-valent metal species to break the C–H bond overcomes the rate-limiting redox step in thermocatalysis by electrochemical polarization featuring electron transfer. The activity of the reaction relies on the availability of *O species to react with methane, necessitating the adsorption of oxygen on the catalyst material. SnO2 and TiO2 are promising candidates for the electrocatalytic oxidation of methane, which favor C–H bond breakage.116
Apart from the formation of methanol and its derivatives, others products with multi-carbon atoms are also expected, such as C2H4, C2H5OH, and C3H7OH.117 The adsorption and conversion of the *CH3 intermediate are the keys to modulate the selectivity of products. Notably, during methane oxidation, certain reaction steps, particularly C–C coupling, may be homogeneous or surface mediated (e.g., nucleophilic addition and free radical addition);118 thus the potential integration of heterogeneous electrocatalysis, homogeneous catalysis, and spontaneous reactions presents a promising avenue for enhancing the selectivity of complex chemical reactions (R-20 in Fig. 5). Although the strategy of electrocatalytic oxidation of alkanes for the production of multi-carbon products is of great significance, it still remains a challenging task and requires further exploration.
6. Concluding remarks
Electrocatalytic upgrading of carbon resources is an effective way for sustainable development and carbon neutrality, which necessitates great efforts from multiple disciplines. In this review, we have summarized recent advances in the electrocatalytic upgrading of carbon resources in four categories based on the types of functional groups of carbon resources, by focusing on key functional groups as the active sites of substrates and corresponding chemical bond transformations, as well as the optimization of the whole system. (i) Electrocatalytic upgrading of alcohols, (ii) electrocatalytic upgrading of carbonyls, (iii) electrocatalytic upgrading of ethers, and (iv) electrocatalytic upgrading of hydrocarbons. To enhance the efficiency of electrocatalytic upgrading of carbon resource, special attention should be paid to the following aspects:
(1) Novel electrocatalytic upgrading configuration design
Although intensive efforts have been made to develop electrocatalytic upgrading systems and significant progress has been achieved, more novel and efficient electrocatalytic upgrading configurations should be designed for the upgrading of carbon resources. Functional groups play a decisive role in determining the properties of organic compounds, which are the core of electrocatalytic upgrading of carbon compounds. Thus, it is particularly important to make use of the specific properties of functional groups in the design of novel electrocatalytic upgrading reactions. First, thorough exploration and understanding of the properties of functional groups is the premise for the upgrading of carbon compounds. For example, aldehyde groups in aldehydes can undergo reduction at the cathode or oxidation reactions at the anode, enabling the electrocatalytic upgrading of aldehydes in a controlled manner to produce the desired product. In addition, a favorable combination of two or more transformations of functional groups in one system, as discussed above, is an efficient approach to design novel electrocatalytic upgrading configurations. For example, the co-reduction of two/more substrates at the cathode and the coupling of anodic oxidation and cathodic reduction are novel strategies for electrocatalytic upgrading, in which two or more carbon substrates are converted to the corresponding intermediates, followed by subsequent interaction of these intermediates for the target products at suitable potentials. Additionally, factors such as the electrolyte, membrane, and electrolyzer configuration should be further investigated and optimized for efficient electrocatalytic upgrading reactions.
(2) Interaction between functional groups
Functional group conversions and interactions between different groups in the molecule(s) of substances have been considered important for electrocatalytic upgrading of carbon sources, including one molecule containing multiple functional groups, or identical/different functional groups belonging to different molecules. However, currently prevailing electrocatalytic reactions, such as aldehyde redox reactions, carbohydrate redox reactions, and aromatic compound redox reactions, focus on the conversion of single-functional groups, which suffers from limited range of reactions and/or infertile mechanisms of reactions at electrodes. Therefore, the interactions between functional groups in one electrolytic system should be brought to the forefront of electrocatalytic upgrading reactions.
(3) Mechanism investigation
The core of electrocatalytic transformation of organic molecules is the transformation of functional groups at the catalyst–electrolyte interface featuring sequential multistep electron transfers, which mainly involve chemical bond breakage and formation. Therefore, the reaction pathways of electrocatalytic systems are closely related to the functional groups of reactants, and intensive investigations have been conducted on the reaction mechanisms and detailed reaction pathways. According to the properties of functional groups, a specific substrate can be selectively converted to desired product(s) by regulating the reaction pathway. In situ or operando observation technologies,119 such as in situ electrochemical impedance spectroscopy (EIS), Fourier transform infrared spectroscopy (FT-IR), and Raman spectroscopy, are necessary and helpful in investigating the transformation of functional groups as well as mechanisms. DFT calculations are essential tools for explaining, understanding, and predicting reaction mechanisms as well as the interactions between functional groups of substrates and intermediates.
(4) Efficient electrocatalyst design
During electrochemical catalysis, the most important steps are the adsorption and activation of substrate molecules, which may be prevented by harsh redox potentials for the formation of intermediates. Electrocatalysts can adsorb and activate the substrates by forming a chemical bond, leading to the activation of substrate molecules at lowered overpotentials.120 The electrocatalytic performances of reactions can be modulated by optimizing the performances of catalysts, while the activity towards specific functional groups and the density of active sites are determined by multiple parameters such as crystal facets, electronic and surface structures, vacancy, strain, etc. Therefore, additional efforts should be dedicated to the development of novel and efficient electrocatalysts by investigating the structure–performance relationship.
(5) Electrolyte
Electrolyte has a huge impact on both the yield and selectivity of electrocatalytic reactions. The local reaction environment, such as the composition and concentration of anions and cations of the electrolytes, may induce great changes in the electrostatic interactions and acidity/alkalinity. The rate of electrocatalytic reactions varies with electrolyte pH values primarily through three mechanisms.121 (i) Changes in concentrations of the proton donor or oxidant, (ii) adsorbate dipole–field interactions, and (iii) solution-phase reactions. The relative increments of current densities at a specific potential can vary by several orders of magnitude for several proton–electron transfer reactions on pH change (ΔpH). The acidity affects the mechanisms of electrocatalytic reactions, thus regulating the selectivity of the products.
(6) The selection of membrane
The integration of membrane technology into electrolysis represents a groundbreaking innovation in electrochemical technology. Common categories of ion exchange membranes include the cation exchange membrane, anion exchange membrane, proton exchange membrane, and bipolar membrane. The ion exchange membrane serves to isolate two polar regions, preventing material contact between them and thus averting chemical reactions. It selectively allows ions to pass through, maintaining charge balance and establishing a closed loop. Additionally, it enables material separation and impurity removal. The selection of an appropriate ion exchange membrane plays a crucial role in determining the selectivity and conversion efficiency of the electrocatalytic reaction.
(7) Product purification
More than one product can be generated in an electrocatalytic upgrading system, which brings about difficulties in the extraction and purification of the desired product from the mixture in most cases. Some products are highly dissoluble in the aqueous electrolytes, which should be purified or concentrated for further applications; thus a focus beyond aqueous electrolytes is crucial for purification. Solid or quasi-solid polymeric electrolytes are highly attractive for producing high-purity target chemicals, particularly when solubility is enhanced. Moreover, additional efforts are required to develop innovative and/or optimized electrocatalytic upgrading systems for the production of high-purity and/or high-concentration products in a cost-effective and practical manner.
Author contributions
D. S. and X. T. contributed equally to this work. L. C. and J. S. structured this review. B. X. collected papers related to the topic. The manuscript was revised by all authors.
Conflicts of interest
There are no conflicts to declare.
Acknowledgements
This work was supported by the National Key R&D Program of China (2022YFB4002700), the National Natural Science Foundation of China (Grant no. 52201271), the Shanghai Science and Technology Committee Rising-Star Program (22QA1403400) and the Natural Science Foundation of Shanghai (21ZR1418700).
References
- J. M. Thomas, Nature, 2008, 455, 732–733 CrossRef CAS.
- C. J. Li and P. T. Anastas, Chem. Soc. Rev., 2012, 41, 1413–1414 RSC.
- P. T. Anastas and J. C. Warner, Abstr. Pap. Am. Chem. Soc., 1998, 244, 19758–19771 Search PubMed.
- J. B. Zimmerman, P. T. Anastas, H. C. Erythropel and W. Leitner, Science, 2020, 367, 397–400 CrossRef CAS PubMed.
- L. Chen and J. Shi, Sci. China Mater., 2022, 65, 1–9 CrossRef CAS.
- D. M. Koshy, S. S. Nathan, A. S. Asundi, A. M. Abdellah, S. M. Dull, D. A. Cullen, D. Higgins, Z. N. Bao, S. F. Bent and T. F. Jaramillo, Angew. Chem., Int. Ed., 2021, 60, 17472–17480 CrossRef CAS PubMed.
- B.-H. Zhao, F. Chen, M. Wang, C. Cheng, Y. Wu, C. Liu, Y. Yu and B. Zhang, Nat. Sustain., 2023, 6, 827–837 CrossRef.
- R. D. Little and K. D. Moeller, Electrochem. Soc. Interface, 2002, 11, 36–42 CrossRef CAS.
- F. Tang, C. Chen and K. D. Moeller, Synthesis, 2007, 21, 3411–3420 Search PubMed.
- H. Xu, L. Chen and J. Shi, Energy Environ. Sci., 2023, 16, 1334–1363 RSC.
- W. Chen, C. Xie, Y. Wang, Y. Zou, C.-L. Dong, Y.-C. Huang, Z. Xiao, Z. Wei, S. Du, C. Chen, B. Zhou, J. Ma and S. Wang, Chem, 2020, 6, 2974–2993 CAS.
- X. Wang, H. Tian, X. Yu, L. Chen, X. Cui and J. Shi, Chin. J. Catal., 2023, 51, 5–48 CrossRef CAS.
- Y. Zeng, M. Zhao, H. Zeng, Q. Jiang, F. Ming, K. Xi, Z. Wang and H. Liang, eScience, 2023, 3, 100156 CrossRef.
- C. Tang, Y. Zheng, M. Jaroniec and S.-Z. Qiao, Angew. Chem., Int. Ed., 2021, 60, 19572–19590 CrossRef CAS.
- A. Badalyan and S. S. Stahl, Nature, 2016, 535, 406–410 CrossRef CAS.
- K. Shi, D. Si, X. Teng, L. Chen and J. Shi, Chin. J. Catal., 2023, 53, 143–152 CrossRef CAS.
- R. Ge, Y. Wang, Z. Li, M. Xu, S.-M. Xu, H. Zhou, K. Ji, F. Chen, J. Zhou and H. Duan, Angew. Chem., Int. Ed., 2022, 61, e202200211 CrossRef CAS.
- K. Xiang, D. Wu, X. Deng, M. Li, S. Chen, P. Hao, X. Guo, J.-L. Luo and X.-Z. Fu, Adv. Funct. Mater., 2020, 30, 1909610 CrossRef CAS.
- P. Rodriguez, Y. Kwon and M. T. M. Koper, Nat. Chem., 2012, 4, 177–182 CrossRef CAS PubMed.
- N. Meng, J. Shao, H. Li, Y. Wang, X. Fu, C. Liu, Y. Yu and B. Zhang, Nat. Commun., 2022, 13, 5452 CrossRef CAS PubMed.
- Y. X. Chen, A. Lavacchi, H. A. Miller, M. Bevilacqua, J. Filippi, M. Innocenti, A. Marchionni, W. Oberhauser, L. Wang and F. Vizza, Nat. Commun., 2014, 5, 4036 CrossRef CAS PubMed.
- Y. Zhao, S. H. Xing, X. Y. Meng, J. H. Zeng, S. B. Yin, X. F. Li and Y. Chen, Nanoscale, 2019, 11, 9319–9326 RSC.
- I. A. Rodrigues, K. Bergamaski and F. C. Nart, J. Electrochem. Soc., 2003, 150, E89–E94 CrossRef CAS.
- J. Zheng, X. L. Chen, X. Zhong, S. Q. Li, T. Z. Liu, G. L. Zhuang, X. N. Li, S. W. Deng, D. H. Mei and J. G. Wang, Adv. Funct. Mater., 2017, 27, 1704169 CrossRef.
- B. You, X. Liu, X. Liu and Y. J. Sun, ACS Catal., 2017, 7, 4564–4570 CrossRef CAS.
- B. You, X. Liu, N. Jiang and Y. Sun, J. Am. Chem. Soc., 2016, 138, 13639–13646 CrossRef CAS PubMed.
- D. Wang, P. Wang, S. Wang, Y.-H. Chen, H. Zhang and A. Lei, Nat. Commun., 2019, 10, 2796 CrossRef PubMed.
- K. Qin, D. Zang and Y. Wei, Chin. Chem. Lett., 2023, 34, 107999 CrossRef CAS.
- X. Huang, Y. Zou and J. Jiang, ACS Sustainable Chem. Eng., 2021, 9, 14470–14479 CrossRef CAS.
- C. Liu, M. Hirohara, T. Maekawa, R. Chang, T. Hayashi and C.-Y. Chiang, Appl. Catal., B, 2020, 265, 118543 CrossRef CAS.
- S. Choi, M. Balamurugan, K.-G. Lee, K. H. Cho, S. Park, H. Seo and K. T. Nam, J. Phys. Chem. Lett., 2020, 11, 2941–2948 CrossRef CAS PubMed.
- L. Xu, Z. Huang, M. Yang, J. Wu, W. Chen, Y. Wu, Y. Pan, Y. Lu, Y. Zou and S. Wang, Angew. Chem., Int. Ed., 2022, 61, e202210123 CrossRef CAS PubMed.
- K. Yin, Y. G. Chao, F. Lv, L. Tao, W. Y. Zhang, S. Y. Lu, M. G. Li, Q. H. Zhang, L. Gu, H. B. Li and S. J. Guo, J. Am. Chem. Soc., 2021, 143, 10822–10827 CrossRef CAS PubMed.
- H. Zhou, Z. Li, S. M. Xu, L. Lu, M. Xu, K. Ji, R. Ge, Y. Yan, L. Ma, X. Kong, L. Zheng and H. Duan, Angew. Chem., Int. Ed., 2021, 60, 8976–8982 CrossRef CAS.
- L. Ma, H. Zhou, X. Kong, Z. Li and H. Duan, ACS Sustainable Chem. Eng., 2021, 9, 1932–1940 CrossRef CAS.
- D. Si, M. Wang, X. Yang, C. Wang, K. Shi, B. Huang, L. Chen and J. Shi, Appl. Catal., B, 2023, 331, 122664 CrossRef CAS.
- M. M. Dimos and G. J. Blanchard, J. Phys. Chem. C, 2011, 115, 11247–11256 CrossRef CAS.
- M. Salciccioli, W. Yu, M. A. Barteau, J. G. Chen and D. G. Vlachos, J. Am. Chem. Soc., 2011, 133, 7996–8004 CrossRef CAS.
- S. C. Chang, Y. Ho and M. J. Weaver, J. Am. Chem. Soc., 1992, 23, 170–178 Search PubMed.
- M. E. Chbihi, D. Takky, F. Hahn, H. Huser, J. M. Leger and C. Lamy, J. Electroanal. Chem., 1999, 463, 63–71 CrossRef CAS.
- M. Garedew, F. Lin, B. Song, T. M. DeWinter, J. E. Jackson, C. M. Saffron, C. H. Lam and P. T. Anastas, ChemSusChem, 2020, 13, 4214–4237 CrossRef CAS PubMed.
- H. Zhou, Y. Ren, Z. H. Li, M. Xu, Y. Wang, R. X. Ge, X. G. Kong, L. R. Zheng and H. H. Duan, Nat. Commun., 2021, 12, 4679 CrossRef CAS PubMed.
- D. Si, B. Y. Xiang, L. S. Chen and J. L. Shi, Chem Catal., 2021, 1, 941–955 CrossRef CAS.
- J. Mahmoudian, M. Bellini, M. V. Pagliaro, W. Oberhauser, M. Innocenti, F. Vizza and H. A. Miller, ACS Sustainable Chem. Eng., 2017, 5, 6090–6098 CrossRef CAS.
- Y. Kwon, Y. Birdja, I. Spanos, P. Rodriguez and M. Koper, ACS Catal., 2012, 2, 759–764 CrossRef CAS.
- B. Tpa, C. Adlr, D. Jab, E. Lc, B. Gb and A. Na, Fuel, 2021, 314, 122788 Search PubMed.
- M. Simões, S. Baranton and C. Coutanceau, ChemSusChem, 2012, 5, 2106–2124 CrossRef PubMed.
- Z. He, J. Hwang, Z. Gong, M. Zhou, N. Zhang, X. Kang, J. W. Han and Y. Chen, Nat. Commun., 2022, 13, 3777 CrossRef CAS PubMed.
- Y. Li, X. Wei, L. Chen, J. Shi and M. He, Nat. Commun., 2019, 10, 5335 CrossRef PubMed.
- L. J. Torres-Pacheco, A. De Leon Rodriguez, J. A. Bañuelos, L. Álvarez-Contreras, M. Guerra-Balcázar and N. Arjona, Fuel, 2022, 314, 122788 CrossRef CAS.
- W. Tang, L. Zhang, T. Qiu, H. Tan, Y. Wang, W. Liu and Y. Li, Angew. Chem., Int. Ed., 2023, 62, e202305843 CrossRef CAS PubMed.
- Y. Yan, H. Zhou, S.-M. Xu, J. Yang, P. Hao, X. Cai, Y. Ren, M. Xu, X. Kong, M. Shao, Z. Li and H. Duan, J. Am. Chem. Soc., 2023, 145, 6144–6155 CrossRef CAS.
- G. Li, G. Han, L. Wang, X. Cui, N. K. Moehring, P. R. Kidambi, D.-e. Jiang and Y. Sun, Nat. Commun., 2023, 14, 525 CrossRef CAS.
- M. Li, T. Wang, W. Zhao, S. Wang and Y. Zou, Nano-Micro Lett., 2022, 14, 211 CrossRef CAS PubMed.
- Y. L. Liang, H. Dong, D. Aurbach and Y. Yao, Nat. Energy, 2020, 5, 646–656 CrossRef CAS.
- Y. Q. Zhang, B. Zhou, Z. X. Wei, W. Zhou, D. D. Wang, J. Tian, T. H. Wang, S. L. Zhao, J. L. Liu, L. Tao and S. Y. Wang, Adv. Mater., 2021, 33, 2104791 CrossRef CAS.
- P. G. Russell, N. Kovac, S. Srinivasan and M. Steinberg, J. Electrochem. Soc., 1977, 124, 1329 CrossRef CAS.
- C. J. Bondue, F. Calle-Vallejo, M. C. Figueiredo and M. T. M. Koper, Nat. Catal., 2019, 2, 243–250 CrossRef CAS.
- Y. Tang, H. Chen, J. Z. Jiang, Z. Tang, B. Y. Huang and H. W. Roesky, J. Power Sources, 2004, 130, 56–60 CrossRef CAS.
- C. Guo, W. Zhou, X. Lan, Y. Wang, T. Li, S. Han, Y. Yu and B. Zhang, J. Am. Chem. Soc., 2022, 144, 16006–16011 CrossRef CAS.
- Z. H. Li, X. F. Li, H. Zhou, Y. Xu, S. M. Xu, Y. Ren, Y. F. Yan, J. R. Yang, K. Y. Ji, L. Li, M. Xu, M. F. Shao, X. G. Kong, X. M. Sun and H. H. Duan, Nat. Commun., 2022, 13, 5099 CrossRef PubMed.
- S. Han, Y. Shi, C. Wang, C. Liu and B. Zhang, Cell Rep. Phys. Sci., 2021, 2, 100337 CrossRef CAS.
- M. A. Villalba and M. T. M. Koper, J. Phys. Chem. C, 2020, 124, 25884–25891 CrossRef CAS.
- L. Du, Y. Shao, J. Sun, G. Yin, C. Du and Y. Wang, Catal. Sci. Technol., 2018, 8, 3216–3232 RSC.
- X. Deng, G.-Y. Xu, Y.-J. Zhang, L. Wang, J. Zhang, J.-F. Li, X.-Z. Fu and J.-L. Luo, Angew. Chem., Int. Ed., 2021, 60, 20535–20542 CrossRef CAS.
- P. Zhou, X. Lv, S. Tao, J. Wu, H. Wang, X. Wei, T. Wang, B. Zhou, Y. Lu, T. Frauenheim, X. Fu, S. Wang and Y. Zou, Adv. Mater., 2022, 34, 2204089 CrossRef CAS.
- T. Wang, L. Tao, X. Zhu, C. Chen, W. Chen, S. Du, Y. Zhou, B. Zhou, D. Wang, C. Xie, P. Long, W. Li, Y. Wang, R. Chen, Y. Zou, X.-Z. Fu, Y. Li, X. Duan and S. Wang, Nat. Catal., 2022, 5, 66–73 CrossRef CAS.
- K. Ji, M. Xu, S. M. Xu, Y. Wang, R. Ge, X. Hu, X. Sun and H. Duan, Angew. Chem., Int. Ed., 2022, 61, e202209849 CrossRef CAS PubMed.
- J. J. Roylance, T. W. Kim and K.-S. Choi, ACS Catal., 2016, 6, 1840–1847 CrossRef CAS.
- X. H. Chadderdon, D. J. Chadderdon, T. Pfennig, B. H. Shanks and W. Li, Green Chem., 2019, 21, 6210–6219 RSC.
- J. J. Roylance and K.-S. Choi, Green Chem., 2016, 18, 2956–2960 RSC.
- J. A. Lopez-Ruiz, U. Sanyal, J. Egbert, O. Y. Gutiérrez and J. Holladay, ACS Sustainable Chem. Eng., 2018, 6, 16073–16085 CrossRef CAS.
- H. Xu, G. Xu, B. Huang, J. Yan, M. Wang, L. Chen and J. Shi, Angew. Chem., Int. Ed., 2023, 62, e202218603 CrossRef CAS PubMed.
- C. J. Bondue and M. T. M. Koper, J. Am. Chem. Soc., 2019, 141, 12071–12078 CrossRef CAS PubMed.
- C. Liu, R. Li, W. Zhou, Y. Liang, Y. Shi, R.-L. Li, Y. Ling, Y. Yu, J. Li and B. Zhang, ACS Catal., 2021, 11, 8958–8967 CrossRef CAS.
- J. Anibal, A. Malkani and B. Xu, Catal. Sci. Technol., 2020, 10, 3181–3194 RSC.
- C. Li, X. Zhao, A. Wang, G. W. Huber and T. Zhang, Chem. Rev., 2015, 115, 11559–11624 CrossRef CAS PubMed.
- S. S. Wong, R. Shu, J. Zhang, H. Liu and N. Yan, Chem. Soc. Rev., 2020, 49, 5510–5560 RSC.
- M. Garedew, D. Young-Farhat, S. Bhatia, P. Hao, J. E. Jackson and C. M. Saffron, Sustainable Energy Fuels, 2020, 4, 1340–1350 RSC.
- Y. Zhou, G. E. Klinger, E. L. Hegg, C. M. Saffron and J. E. Jackson, J. Am. Chem. Soc., 2020, 142, 4037–4050 CrossRef CAS.
- U. Sanyal, J. Lopez-Ruiz, A. B. Padmaperuma, J. Holladay and O. Y. Gutiérrez, Org. Process Res. Dev., 2018, 22, 1590–1598 CrossRef CAS.
- B. Mahdavi, A. Lafrance, A. Martel, J. Lessard, H. Me'nard and L. Brossard, J. Appl. Electrochem., 1997, 27, 605–611 CrossRef CAS.
- J. Zakzeski, P. C. A. Bruijnincx, A. L. Jongerius and B. M. Weckhuysen, Chem. Rev., 2010, 110, 3552–3599 CrossRef CAS.
- C. P. Xu, R. A. D. Arancon, J. Labidi and R. Luque, Chem. Soc. Rev., 2014, 43, 7485–7500 RSC.
- C. Liu, S. L. Wu, H. Y. Zhang and R. Xiao, Fuel Process. Technol., 2019, 191, 181–201 CrossRef CAS.
- I. Bosque, G. Magallanes, M. Rigoulet, M. D. Karkas and C. R. J. Stephenson, ACS Cent. Sci., 2017, 3, 621–628 CrossRef CAS.
- S. Mohle, M. Zirbes, E. Rodrigo, T. Gieshoff, A. Wiebe and S. R. Waldvogel, Angew. Chem., Int. Ed., 2018, 57, 6018–6041 CrossRef.
- S. Han, Y. Shi, C. Wang, C. Liu and B. Zhang, Cell Rep. Phys. Sci., 2021, 2, 100337 CrossRef CAS.
- V. L. Pardini, C. Z. Smith, J. H. P. Utley, R. R. Vargas and H. Viertler, J. Org. Chem., 2002, 56, 7305–7313 CrossRef.
- V. L. Pardini, R. R. Vargas, H. Viertler and J. H. P. Utley, Tetrahedron, 1992, 48, 7221–7228 CrossRef CAS.
- D. Rochefort, R. Bourbonnais, D. Leech and M. G. Paice, Chem. Commun., 2002, 11, 1182–1183 RSC.
- T. Cui, L. Ma, S. Wang, C. Ye, X. Liang, Z. Zhang, G. Meng, L. Zheng, H.-S. Hu, J. Zhang, H. Duan, D. Wang and Y. Li, J. Am. Chem. Soc., 2021, 143, 9429–9439 CrossRef CAS PubMed.
- J. Ke, J. Zhao, M. Chi, M. Wang, X. Kong, Q. Chang, W. Zhou, C. Long, J. Zeng and Z. Geng, Nat. Commun., 2022, 13, 932 CrossRef CAS.
- Y. Lum, J. E. Huang, Z. Y. Wang, M. C. Luo, D. H. Nam, W. R. Leow, B. Chen, J. Wicks, Y. G. C. Li, Y. H. Wang, C. T. Dinh, J. Li, T. T. Zhuang, F. W. Li, T. K. Sham, D. Sinton and E. H. Sargent, Nat. Catal., 2020, 3, 14–22 CrossRef CAS.
- K. Jin, J. H. Maalouf, N. Lazouski, N. Corbin, D. T. Yang and K. Manthiram, J. Am. Chem. Soc., 2019, 141, 6413–6418 CrossRef CAS PubMed.
- J. S. Jirkovsky, M. Busch, E. Ahlberg, I. Panas and P. Krtil, J. Am. Chem. Soc., 2011, 133, 5882–5892 CrossRef CAS PubMed.
- A. Winiwarter, L. Silvioli, S. B. Scott, K. Enemark-Rasmussen, M. Saric, D. B. Trimarco, P. C. K. Vesborg, P. G. Moses, I. E. L. Stephens, B. Seger, J. Rossmeisl and I. Chorkendorff, Energy Environ. Sci., 2019, 12, 1055–1067 RSC.
- W. R. Leow, Y. Lum, A. Ozden, Y. H. Wang, D. H. Nam, B. Chen, J. Wicks, T. T. Zhuang, F. W. Li, D. Sinton and E. H. Sargent, Science, 2020, 368, 1228–1233 CrossRef CAS PubMed.
- Y. Gao, M. Yan, C. Cheng, H. Zhong, B.-H. Zhao, C. Liu, Y. Wu and B. Zhang, J. Am. Chem. Soc., 2023, 146, 714–722 CrossRef PubMed.
-
O. Hammerich and B. Speiser, Organic Electrochemistry: Revised and Expanded, CRC Press, 5th edn, 2015, p. 18, DOI:10.1201/b19122-54.
- S. Tomida, R. Tsuda, S. Furukawa, M. Saito and T. Tajima, Electrochem. Commun., 2016, 73, 46–49 CrossRef CAS.
- I. Fussing, M. Güllü, O. Hammerich, A. Hussain, M. F. Nielsen and J. H. P. Utley, J. Chem. Soc., Perkin Trans. 2, 1996, 4, 649–658 RSC.
- S. Kratschmer, H. J. Schafer and R. Frohlich, J. Electroanal. Chem., 2001, 507, 2–10 CrossRef CAS.
- T. Kojima, R. Obata, T. Saito, Y. Einaga and S. Nishiyama, Beilstein J. Org. Chem., 2015, 11, 200–203 CrossRef PubMed.
- A. H. Chen, C. Y. Cheng and C. W. Chen, J. Chin. Chem. Soc., 2002, 49, 1105–1109 CrossRef CAS.
- A. Jones, H. Kronenwetter and R. Manchanayakage, Electrochem. Commun., 2012, 25, 8–10 CrossRef CAS.
- M. Weling and H. J. Schafer, Beilstein J. Org. Chem., 2015, 11, 1163–1174 CrossRef PubMed.
- R. Shi, Z. P. Wang, Y. X. Zhao, G. I. N. Waterhouse, Z. H. Li, B. K. Zhang, Z. M. Sun, C. A. Xia, H. T. Wang and T. R. Zhang, Nat. Catal., 2021, 4, 565–574 CrossRef CAS.
- J. Bu, Z. Liu, W. Ma, L. Zhang and J. Zhang, Nat.
Catal., 2021, 4, 557–564 CrossRef CAS.
- A. J. McCue, A. Guerrero-Ruiz, I. Rodriguez-Ramos and J. A. Anderson, J. Catal., 2016, 340, 10–16 CrossRef CAS.
- S. Wang, K. Uwakwe, L. Yu, J. Ye, Y. Zhu, J. Hu, R. Chen, Z. Zhang, Z. Zhou, J. Li, Z. Xie and D. Deng, Nat. Commun., 2021, 12, 7072 CrossRef CAS PubMed.
- Y. Wu, C. Liu, C. Wang, Y. Yu, Y. Shi and B. Zhang, Nat. Commun., 2021, 12, 3881 CrossRef CAS PubMed.
- L. Ye, X. Duan and K. Xie, ACS Catal., 2020, 10, 3505–3513 CrossRef.
- P. Vernoux, F. Gaillard, L. Bultel, E. Siebert and M. Primet, J. Catal., 2002, 208, 412–421 CrossRef CAS.
- M. O'Reilly, R. S. Kim, S. Oh and Y. Surendranath, ACS Cent. Sci., 2017, 3, 1174–1179 CrossRef PubMed.
- L. Arnarson, P. S. Schmidt, M. Pandey, A. Bagger, K. S. Thygesen, I. E. L. Stephens and J. Rossmeisl, Phys. Chem. Chem. Phys., 2018, 20, 11152–11159 RSC.
- Y. F. Song, Y. H. Zhao, G. Z. Nan, W. Chen, Z. K. Guo, S. G. Li, Z. Y. Tang, W. Wei and Y. H. Sun, Appl. Catal., B, 2020, 270, 118888 CrossRef CAS.
- M. Ming, J. J. Bing, L. Ping, M. S. Jung, I. K. Jin, Y. Cho, S. Kim, J. H. Moon and J. H. Park, Adv. Sci., 2017, 4, 1700379 CrossRef.
- D.-Q. Liu, B. Zhang, G. Zhao, J. Chen, H. Pan and W. Sun, Chin. J. Catal., 2023, 47, 93–120 CrossRef CAS.
- Q. Xue, Z. Wang, Y. Ding, F. Li and Y. Chen, Chin. J. Catal., 2023, 45, 6–16 CrossRef CAS.
- N. Govindarajan, A. Xu and K. Chan, Science, 2022, 375, 379–380 CrossRef CAS.
Footnote |
† These authors contributed equally to this work. |
|
This journal is © The Royal Society of Chemistry 2024 |