DOI:
10.1039/D4SC00393D
(Edge Article)
Chem. Sci., 2024,
15, 7293-7299
Photoinduced C–H arylation of 1,3-azoles via copper/photoredox dual catalysis†
Received
17th January 2024
, Accepted 15th April 2024
First published on 16th April 2024
Abstract
The visible light-induced C–H arylation of azoles has been accomplished by dual-catalytic system with the aid of an inexpensive ligand-free copper(I)-catalyst in combination with a suitable photoredox catalyst. An organic photoredox catalyst, 10-phenylphenothiazine (PTH), was identified as effective, cost-efficient and environmentally-benign alternative to commonly-used, expensive Ir(III)-based complexes. The method proved applicable for the C–H arylation of various azole derivatives, including oxazoles, benzoxazoles, thiazoles, benzothiazoles as well as more challenging imidazoles and benzimidazoles. Moreover, the derivatization of complex molecules and the gram scale synthesis of the natural product balsoxin reflected the synthetic utility of the developed strategy. Mechanistic studies were indicative of a single electron transfer-based (SET) mechanism with an aryl radical as key intermediate.
Introduction
The direct functionalization of C–H bonds represents a highly efficient, powerful, and sustainable transformation in modern organic synthesis outperforming traditional cross-coupling reactions with regards to step- and atom-economy.1–6 Utilizing the C–H activation approach, valuable and important molecular scaffolds with broad applications ranging from material sciences7,8 to drug discovery9–11 and crop protection can be elegantly accessed.12,13
As 2-aryl benzimidazoles and imidazoles often exhibit interesting bioactive properties,14–21 these structural motifs are therefore important building blocks in pharmacologically relevant molecules (Scheme 1a). Classical methods for their synthesis comprise the de novo synthesis of the heterocyclic core via condensation–cyclization reactions. Most of these strategies imply multi-step syntheses of starting materials and require stoichiometric amounts of toxic reagents, strong acids or harsh reaction conditions.22–25 Due to the high relevance of these scaffolds, a sustainable, mild, and efficient synthesis is highly desirable, as represented by the direct C–H arylation of the corresponding core heterocycles. In this context, the C–H acidity of the targeted heterocycle is an important indicator for the reactivity of a certain C–H bond (Scheme 1b).26
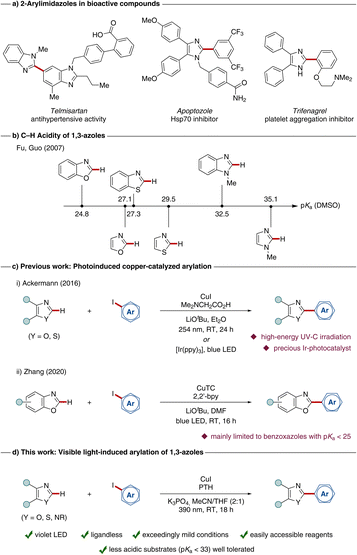 |
| Scheme 1 (a) 2-Aryl imidazole motifs in pharmaceuticals. (b) Predicted pKa-values for 1,3-azoles in DMSO.26 Copper-catalyzed C–H arylations of azoles via (c) photo-induced copper-catalyzed procedures for the arylation of oxazoles and thiazoles and (d) our approach for arylations under mild visible light irradiation. | |
Although the application of 4d27–31 and 5d32–36 transition metals has been well-demonstrated for C–H activation strategies, their high price and rather toxic properties limit real application. During the last years, remarkable progress could be observed in the field of first row transition-metal catalyzed C–H functionalizations, thus highlighting earth-abundant and environmentally-benign 3d metals as powerful substitute for their heavier analogues.37,38 Especially, copper(I) salts proved to be a powerful catalyst in the direct functionalization of azole-based compounds, with major contributions by Daugulis,39–41 Miura,42 and Ackermann,43,44 among others.45–47 However, these methods typically require high reaction temperatures ranging from 100–160 °C.
In sharp contrast, the merger of photocatalysis48–55 with copper-catalyzed transformations allows to perform organic transformations under significantly milder conditions.56 In this context, our group reported in 2016 on a photoinduced C–H functionalization of benzoxazoles and benzothiazoles (Scheme 1c(i)) using UV-C irradiation.57 Interestingly, copper(I)-complexes were employed as dual-functional catalysts, which mediate the C–C bond formation and also serve as the photocatalyst. Additionally, a first example for the arylation using visible light in combination with an iridium-photocatalyst was reported in the same work.
More recent studies focused on the use of visible light, as demonstrated in cross-coupling reactions.58 In 2020, Zhang employed a photoactive copper(I) complex as sole catalyst for C–H arylations of benzoxazoles, benzothiazoles and electron-deficient thiophenes (Scheme 1c(ii)).59 However, known strategies for the photoinduced arylation of azoles are mainly limited to benzoxazoles and benzothiazoles with a pKa value below 27.5.26 In contrast, only few precedents are known for the direct arylation of more challenging benzimidazoles (pKa = 32.5) under photochemical conditions. In this context, our group has enabled the direct arylation utilizing an immobilized copper(I) catalyst,60 however, UV-C irradiation is typically required.
Within our program on photoinduced C–H functionalizations,61–65 we have now developed a strategy for the direct arylation of benzimidazoles at room temperature enabled by visible light irradiation (Scheme 1d). Key features of our method include (i) a dual catalytic, ligand-free system with a cost-efficient copper(I) precursor and an exogeneous photoredox catalyst which allows for (ii) visible light-induced transformations under (iii) mild conditions at ambient temperature with (iv) broad applicability to various azole-based compounds.
Results and discussion
Optimization of the reaction conditions
We commenced our studies by probing various reaction conditions to enable the envisioned copper-catalyzed arylation of benzimidazole 1a with aryl iodide 2a (Table 1) under blue LED (450 nm) irradiation. The desired arylation product 3a was obtained with 71% yield using CuI as copper source in combination with [Ir(p-F-ppy)3] as photoredox catalyst and K3PO4 as base in a solvent mixture of MeCN and THF (2
:
1) (entry 1). THF or MeCN as sole solvents furnished product 3a in significantly reduced yields of 38% or 7%, respectively (entries 2–3). Other bases, such as K2CO3, led to a diminished yield, while LiOtBu failed in the transformation (entries 4–5). Alternative copper(I) sources, such as CuTC (copper(I) thiophene-2-carboxylate) or CuOAc instead of CuI caused a slightly decreased efficacy in the arylation (entries 6–7). In contrast to [Ir(p-F-ppy)3] photoredox catalyst, the commonly used fac-[Ir(ppy)3] photoredox catalyst furnished product 3a in an unsatisfactory yield of 20% (entry 8). In order to circumvent the use of rare and expensive iridium(III)-based catalysts and to reduce undesired, potential trace metal impurities in the product, we thereafter probed organic photoredox catalysts (entries 9–10). Acridinium salt [Mes-Acr]BF4 failed to enable the reaction (entry 9). In contrast, 10-phenylphenothiazine (PTH)66,67 exhibited notable efficacy as photoredox catalyst using violet LED irradiation with a wavelength of 390 nm (entry 10). The modified conditions furnished product 3a in 77% yield. 4-Bromotoluene (entry 11) proved viable as electrophile, albeit with lower catalytic efficacy.68 Control experiments confirmed the essential role of the copper(I) salt and the photochemical nature of the reaction (entry 12). Furthermore, the essential role and positive synergistic effects of the addition of a photoredox catalyst were highlighted in further control experiments (entries 13–14).69
Table 1 Optimization of reaction conditions

|
Entry |
Deviation from standard conditions |
Yielda (%) |
Reaction conditions: 1a (0.25 mmol), 2a (0.75 mmol), CuI (20 mol%), [Ir(p-F-ppy)3] (2.0 mol%), K3PO4 (0.75 mmol), MeCN/THF (2 : 1) (1 mL), 35 °C, 18 h, under N2, blue LEDs (450 nm); yield of isolated products.
The yield was determined by 1H-NMR spectroscopy using 1,3,5-trimethoxybenzene as internal standard.
10 mol% [Mes-Acr]BF4.
5.0 mol% PTH. Mes-Acr = 9-mesity-10-methylacridinium. PTH = 10-phenylphenothiazine.
|
1 |
None |
71 |
2 |
THF instead of MeCN/THF (2 : 1) |
38 |
3 |
MeCN instead of MeCN/THF (2 : 1) |
7b |
4 |
K2CO3 instead of K3PO4 |
58 |
5 |
LiOtBu instead of K3PO4 |
0b |
6 |
CuTC instead of CuI |
62 |
7 |
CuOAc instead of CuI |
63 |
8 |
fac-[Ir(ppy)3] instead of [Ir(p-F-ppy)3] |
20 |
9 |
[Mes-Acr]BF4 instead of [Ir(p-F-ppy)3] |
0b,c |
10 |
PTH instead of [Ir(p-F-ppy)3] at 390 nm |
77d |
11 |
4-Br-Tol instead of 2a and PTH instead of [Ir(p-F-ppy)3] at 390 nm |
45b |
12 |
No CuI or no light |
0b |
13 |
Without photocatalyst at 450 nm |
0b |
14 |
Without photocatalyst at 390 nm |
44 |
Photocatalysis robustness
With the optimized conditions in hand, we probed the versatility of the photo-induced copper-catalyzed C–H arylation with PTH for a set of differently substituted aryl iodides 2 (Scheme 2a). Both, electron-donating as well as electron-withdrawing substituents in various positions proved viable (3a–3o) with yields up to 77%. The mild photocatalysis also tolerated aryl iodides with sensitive functionalities, such as synthetically useful nitrile (3e) and ester (3i) substituents, which were transformed in a chemoselective manner. Also, the chloro-containing product 3h could be isolated, albeit with a reduced yield due to partial protodehalogenation. Finally, 2-iodothiophene (3o) and natural product-embedded aryl iodides (3p–3r) proved to be suitable substrates setting the stage for more complex molecule diversifications through the developed method (Scheme 2b).
 |
| Scheme 2 Substrate scope for aryl iodides in photo-induced copper(I)-catalyzed C–H arylations at room temperature. | |
Moreover, the photo-induced copper-catalyzed C–H arylation was also suitable for differently substituted benzimidazoles (5a–5f) (Scheme 3a). Additionally, 4,5- and 4-substituted imidazoles (5g, 5h), as well as other heterocyclic compounds with more acidic C–H bonds like benzoxazole (5i), oxazoles (5j–5l), benzothiazole (5m) and thiazole (5n) have been efficiently converted in the photoinduced arylation reaction. The scalability and synthetic utility of our approach were further demonstrated by the gram scale synthesis of the natural product balsoxin (5o), which was isolated in 72% yield (Scheme 3b).
 |
| Scheme 3 Substrate scope for differently substituted azole. [a] 4 (0.25 mmol), 2a (1.00 mmol), [Cu(MeCN)4]PF6 (30 mol%), PTH (5.0 mol%), K3PO4 (0.75 mmol), MeCN/THF (2 : 1, 0.5 mL), 390 nm, RT, 48 h. | |
To further advance the developed methodology, we aimed to realize a concept for the arylation of benzimidazoles in an enantioselective fashion. At the outset of our studies, we identified that the introduction of a sterically demanding substituent at the N(1) position, as represented by naphthyl-substituted benzimidazole 6, resulted in an atropostable C–N axis through C–H arylation. Interestingly, the combination of CuI with the anionic cyano-bisoxazoline ligand (CN-BOX) achieved promising results after initial optimizations,68 furnishing 26% of the atropostable product 7 with 79.5
:
20.5 e.r. (Scheme 4).
 |
| Scheme 4 Initial results on the photo-induced atroposelective C–H arylation of benzimidazoles. | |
Mechanistic considerations
Next, we investigated the mode of action of the dual-catalytic C–H arylation by carrying out a set of mechanistic experiments. An intermolecular competition experiment between 4-iodotoluene (2a) and 4-iodobenzotrifluoride (2f) revealed an inherently higher reactivity of the electron-deficient 4-iodobenzotrifluoride (Scheme 5a). These findings could suggest a single-electron reduction of the aryl iodide.70,71 A kinetic isotope effect experiment revealed a rather unusual kH/kD of 0.70 (Scheme 5b) which could indicate a change in hybridization of the C(2) of benzimidazole 1a. To probe, whether a SET-type regime is operative, radical scavenger experiments (Scheme 5c) were conducted. The presence of TEMPO (2,2,6,6-tetramethylpiperidinyloxyl) caused a significant inhibition of the arylation, while galvinoxyl or BHT (butylated hydroxytoluene) completely suppressed the reaction, thereby suggesting a radical mechanism. Moreover, the formation of the TEMPO- and galvinoxyl-tolyl adducts were detected via HRMS analysis, which further supported a single-electron reduction pathway generating an aryl radical species.68 The role of the violet LED irradiation was further elucidated by an on/off experiment. As the reaction completely ceased in the absence of light (Scheme 5d), a radical chain pathway seemed unlikely for the reaction. Additionally, a low quantum yield substantiated the absence of a radical chain mechanism.68 Fluorescence quenching studies at 390 nm furthermore showed an effective quenching of the excited state of the PTH photocatalyst by aryl iodide 2a indicating an one-electron oxidative quenching pathway.68
 |
| Scheme 5 Key mechanistic experiments. | |
Based on literature precedents and our mechanistic findings, a plausible mechanistic scenario for the photo-induced copper-catalyzed C–H arylation was proposed (Scheme 6). Starting with copper complex I which is formed in situ from copper(I) iodide through coordination of the substrate and solvent molecules. The coordination of copper at the Lewis-basic N(3) nitrogen of 1a causes an increased C–H acidity facilitating the copper-assisted C–H bond cleavage in C(2) position forming copper complex II.72 Oxidative quenching of the excited PTH* species through a SET process generates an aryl radical, which is captured by copper complex III formed by the oxidation of complex II and thus regenerating the photoredox catalyst. The resulting copper(III) complex IV undergoes reductive elimination thereby forming the desired C–C bond. After ligand exchange with another benzimidazole molecule 1a, the product is released and, at the same time, the catalyst is regenerated.
 |
| Scheme 6 Proposed catalytic cycle (L = MeCN, THF, 1a). | |
Conclusions
In conclusion, we introduced a versatile synergistic, copper-catalyzed C–H arylation which enables the efficient functionalization of a variety of azoles at ambient temperature. Notably, the C–H functionalization has not only been successfully performed on oxazoles and thiazoles, but also on more challenging azole-based compounds, including benzimidazoles. Exceedingly mild conditions enabled an ample scope. Additionally, the use of the readily available, inexpensive organic PTH as photoredox catalyst proved to be a powerful alternative to high-priced iridium-photocatalysts, thereby improving the cost-efficiency and the environmental footprint of our method.
Data availability
The data supporting this article have been uploaded as part of the ESI.†
Author contributions
Conceptualization, L. A.; funding acquisition, L. A.; investigation S. T. and J. X.; methodology, S. T. and J. X.; resources, L. A.; supervision, L. A.; writing – original draft, L. A. and S. T.; writing – reviewing & editing, L. A., S. T. and J. X.
Conflicts of interest
There are no conflicts to declare.
Acknowledgements
Generous support by DZHK, the CSC (fellowship to J. X.), the DFG (Gottfried Wilhelm Leibniz award to L. A.) and the ERC Advanced Grant no. 101021358 (L. A.) is gratefully acknowledged.
Notes and references
- T. Gensch, M. N. Hopkinson, F. Glorius and J. Wencel-Delord, Mild metal-catalyzed C–H activation: examples and concepts, Chem. Soc. Rev., 2016, 45, 2900–2936 RSC.
- T. Rogge, N. Kaplaneris, N. Chatani, J. Kim, S. Chang, B. Punji, L. L. Schafer, D. G. Musaev, J. Wencel-Delord, C. A. Roberts, R. Sarpong, Z. E. Wilson, M. A. Brimble, M. J. Johansson and L. Ackermann, C–H activation, Nat. Rev. Methods Primers, 2021, 1, 43 CrossRef CAS.
- Ł. Woźniak and N. Cramer, Enantioselective CH Bond Functionalizations by 3d Transition-Metal Catalysts, Trends Chem., 2019, 1, 471–484 CrossRef.
- U. Dutta, S. Maiti, T. Bhattacharya and D. Maiti, Arene diversification through distal C(sp2)−H functionalization, Science, 2021, 372, eabd5992 CrossRef CAS PubMed.
- Y. Park, Y. Kim and S. Chang, Transition Metal-Catalyzed C–H Amination: Scope, Mechanism, and Applications, Chem. Rev., 2017, 117, 9247–9301 CrossRef CAS PubMed.
- S. Rej, Y. Ano and N. Chatani, Bidentate Directing Groups: An Efficient Tool in C–H Bond Functionalization Chemistry for the Expedient Construction of C–C Bonds, Chem. Rev., 2020, 120, 1788–1887 CrossRef CAS PubMed.
- J.-R. Pouliot, F. Grenier, J. T. Blaskovits, S. Beaupré and M. Leclerc, Direct (Hetero)arylation Polymerization: Simplicity for Conjugated Polymer Synthesis, Chem. Rev., 2016, 116, 14225–14274 CrossRef CAS PubMed.
- Y. Segawa, T. Maekawa and K. Itami, Synthesis of Extended π-Systems through C–H Activation, Angew. Chem., Int. Ed., 2015, 54, 66–81 CrossRef CAS PubMed.
- D. C. Blakemore, L. Castro, I. Churcher, D. C. Rees, A. W. Thomas, D. M. Wilson and A. Wood, Organic synthesis provides opportunities to transform drug discovery, Nat. Chem., 2018, 10, 383–394 CrossRef CAS PubMed.
- S. D. Friis, M. J. Johansson and L. Ackermann, Cobalt-catalysed C–H methylation for late-stage drug diversification, Nat. Chem., 2020, 12, 511–519 CrossRef CAS PubMed.
- L. Guillemard, N. Kaplaneris, L. Ackermann and M. J. Johansson, Late-stage C–H functionalization offers new opportunities in drug discovery, Nat. Rev. Chem., 2021, 5, 522–545 CrossRef CAS PubMed.
- J. Hubrich, T. Himmler, L. Rodefeld and L. Ackermann, Ruthenium(II)-Catalyzed C–H Arylation of Azoarenes by Carboxylate Assistance, ACS Catal., 2015, 5, 4089–4093 CrossRef CAS.
- G. Wang, P. Cui, H. Bai, S. Wei and S. Li, Late-Stage C–H Functionalization of Nicotinamides for the Expedient Discovery of Novel Antifungal Leads, J. Agric. Food Chem., 2019, 67, 11901–11910 CrossRef CAS PubMed.
- S. L. Abrahams, R. J. Hazen, A. G. Batson and A. P. Phillips, Trifenagrel: a chemically novel platelet aggregation inhibitor, J. Pharmacol. Exp. Ther., 1989, 249, 359–365 CAS.
- A. A. Adegboye, K. M. Khan, U. Salar, S. A. Aboaba, Kanwal, S. Chigurupati, I. Fatima, M. Taha, A. Wadood, J. I. Mohammad, H. Khan and S. Perveen, 2-Aryl benzimidazoles: Synthesis, In vitro α-amylase inhibitory activity, and molecular docking study, Eur. J. Med. Chem., 2018, 150, 248–260 CrossRef CAS PubMed.
- M. Gaba and C. Mohan, Development of drugs based on imidazole and benzimidazole bioactive heterocycles: recent advances and future directions, Med. Chem. Res., 2016, 25, 173–210 CrossRef CAS.
- V. L. Nayak, N. Nagesh, A. Ravikumar, C. Bagul, M. V. P. S. Vishnuvardhan, V. Srinivasulu and A. Kamal, 2-aryl benzimidazole conjugate induced apoptosis in human breast cancer MCF-7 cells through caspase independent pathway, Apoptosis, 2017, 22, 118–134 CrossRef CAS PubMed.
- A. Reyes-Arellano, O. Gómez-García and J. Torres-Jaramillo, Synthesis of azolines and imidazoles and their use in drug design, Med. Chem., 2016, 6, 561–570 Search PubMed.
- T. Roth, M. L. Morningstar, P. L. Boyer, S. H. Hughes, R. W. Buckheit and C. J. Michejda, Synthesis and Biological Activity of Novel Nonnucleoside Inhibitors of HIV-1 Reverse Transcriptase. 2-Aryl-Substituted Benzimidazoles, J. Med. Chem., 1997, 40, 4199–4207 CrossRef CAS PubMed.
- W. Wienen, M. Entzeroth, J. C. A. van Meel, J. Stangier, U. Busch, T. Ebner, J. Schmid, H. Lehmann, K. Matzek, J. Kempthorne-Rawson, V. Gladigau and N. H. Hauel, A Review on Telmisartan: A Novel, Long-Acting Angiotensin II-Receptor Antagonist, Cardiovasc. Drug Rev., 2000, 18, 127–154 CrossRef CAS.
- J. Yang, Y. Xu, Y. Yan, W. Li, L. Zhao, Q. Dai, Y. Li, S. Li, J. Zhong, R. Cao and W. Zhong, Small Molecule Inhibitor of ATPase Activity of HSP70 as a Broad-Spectrum Inhibitor against Flavivirus Infections, ACS Infect. Dis., 2020, 6, 832–843 CrossRef CAS PubMed.
- D. Yang, D. Fokas, J. Li, L. Yu and C. M. Baldino, A versatile method for the synthesis of benzimidazoles from o-nitroanilines and aldehydes in one step via a reductive cyclization, Synthesis, 2005, 47–56 Search PubMed.
- J. B. Wright, The chemistry of the benzimidazoles, Chem. Rev., 1951, 48, 397–541 CrossRef CAS PubMed.
- P. Preston, Synthesis, reactions, and spectroscopic properties of benzimidazoles, Chem. Rev., 1974, 74, 279–314 CrossRef CAS.
- J. Chen, W. Chen, Y. Yu and G. Zhang, One-pot synthesis of disubstituted imidazole derivatives from α-azido ketones catalyzed by potassium ethylxanthate, Tetrahedron Lett., 2013, 54, 1572–1575 CrossRef CAS.
- K. Shen, Y. Fu, J.-N. Li, L. Liu and Q.-X. Guo, What are the pKa values of C–H bonds in aromatic heterocyclic compounds in DMSO?, Tetrahedron, 2007, 63, 1568–1576 CrossRef CAS.
- L. Ackermann, Carboxylate-Assisted Ruthenium-Catalyzed Alkyne Annulations by C–H/Het–H Bond Functionalizations, Acc. Chem. Res., 2014, 47, 281–295 CrossRef CAS PubMed.
- P. B. Arockiam, C. Bruneau and P. H. Dixneuf, Ruthenium(II)-Catalyzed C–H Bond Activation and Functionalization, Chem. Rev., 2012, 112, 5879–5918 CrossRef CAS PubMed.
- D. A. Colby, R. G. Bergman and J. A. Ellman, Rhodium-Catalyzed C−C Bond Formation via Heteroatom-Directed C−H Bond Activation, Chem. Rev., 2010, 110, 624–655 CrossRef CAS PubMed.
- J. A. Leitch and C. G. Frost, Ruthenium-catalysed σ-activation for remote meta-selective C–H functionalisation, Chem. Soc. Rev., 2017, 46, 7145–7153 RSC.
- J. He, M. Wasa, K. S. L. Chan, Q. Shao and J.-Q. Yu, Palladium-Catalyzed Transformations of Alkyl C–H Bonds, Chem. Rev., 2017, 117, 8754–8786 CrossRef CAS PubMed.
- Y. Qiu, M. Stangier, T. H. Meyer, J. C. A. Oliveira and L. Ackermann, Iridium-Catalyzed Electrooxidative C−H Activation by Chemoselective Redox-Catalyst Cooperation, Angew. Chem., Int. Ed., 2018, 57, 14179–14183 CrossRef CAS PubMed.
- M. A. Larsen and J. F. Hartwig, Iridium-Catalyzed C–H Borylation of Heteroarenes: Scope, Regioselectivity, Application to Late-Stage Functionalization, and Mechanism, J. Am. Chem. Soc., 2014, 136, 4287–4299 CrossRef CAS PubMed.
- C. Haldar, M. Emdadul Hoque, R. Bisht and B. Chattopadhyay, Concept of Ir-catalyzed CH bond activation/borylation by noncovalent interaction, Tetrahedron Lett., 2018, 59, 1269–1277 CrossRef CAS.
- C. Yuan and B. Liu, Total synthesis of natural products via iridium catalysis, Org. Chem. Front., 2018, 5, 106–131 RSC.
- J. Choi and A. S. Goldman, Top. Organomet. Chem., 2011, 34, 139–167 CrossRef CAS PubMed.
- J. Loup, U. Dhawa, F. Pesciaioli, J. Wencel-Delord and L. Ackermann, Enantioselective C−H Activation with Earth-Abundant 3d Transition Metals, Angew. Chem., Int. Ed., 2019, 58, 12803–12818 CrossRef CAS PubMed.
- P. Gandeepan, T. Müller, D. Zell, G. Cera, S. Warratz and L. Ackermann, 3d Transition Metals for C–H Activation, Chem. Rev., 2019, 119, 2192–2452 CrossRef CAS PubMed.
- H.-Q. Do and O. Daugulis, Copper-Catalyzed Arylation of Heterocycle C−H Bonds, J. Am. Chem. Soc., 2007, 129, 12404–12405 CrossRef CAS PubMed.
- H.-Q. Do, R. M. K. Khan and O. Daugulis, A General Method for Copper-Catalyzed Arylation of Arene C−H Bonds, J. Am. Chem. Soc., 2008, 130, 15185–15192 CrossRef CAS PubMed.
- H.-Q. Do and O. Daugulis, Copper-Catalyzed Arylation and Alkenylation of Polyfluoroarene C−H Bonds, J. Am. Chem. Soc., 2008, 130, 1128–1129 CrossRef CAS PubMed.
- T. Yoshizumi, H. Tsurugi, T. Satoh and M. Miura, Copper-mediated direct arylation of benzoazoles with aryl iodides, Tetrahedron Lett., 2008, 49, 1598–1600 CrossRef CAS.
- L. Ackermann, H. K. Potukuchi, D. Landsberg and R. Vicente, Copper-Catalyzed “Click” Reaction/Direct Arylation Sequence: Modular Syntheses of 1,2,3-Triazoles, Org. Lett., 2008, 10, 3081–3084 CrossRef CAS PubMed.
- R. Jeyachandran, H. K. Potukuchi and L. Ackermann, Copper-catalyzed CuAAC/intramolecular C–H arylation sequence: Synthesis of annulated 1, 2, 3-triazoles, Beilstein J. Org. Chem., 2012, 8, 1771–1777 CrossRef CAS PubMed.
- N.-N. Jia, X.-C. Tian, X.-X. Qu, X.-X. Chen, Y.-N. Cao, Y.-X. Yao, F. Gao and X.-L. Zhou, Copper-catalyzed Direct 2-Arylation of Benzoxazoles and Benzoimidazoles with Aryl Bromides and Cytotoxicity of Products, Sci. Rep., 2017, 7, 43758 CrossRef PubMed.
- Y. Li, Y. Xie, R. Zhang, K. Jin, X. Wang and C. Duan, Copper-Catalyzed Direct Oxidative C–H Amination of Benzoxazoles with Formamides or Secondary Amines under Mild Conditions, J. Org. Chem., 2011, 76, 5444–5449 CrossRef CAS PubMed.
- L. Chen, L. Ju, K. A. Bustin and J. M. Hoover, Copper-catalyzed oxidative decarboxylative C–H arylation of benzoxazoles with 2-nitrobenzoic acids, Chem. Commun., 2015, 51, 15059–15062 RSC.
- J.-P. Goddard, C. Ollivier and L. Fensterbank, Photoredox Catalysis for the Generation of Carbon Centered Radicals, Acc. Chem. Res., 2016, 49, 1924–1936 CrossRef CAS PubMed.
- L. Marzo, S. K. Pagire, O. Reiser and B. König, Visible-Light Photocatalysis: Does It Make a Difference in Organic Synthesis?, Angew. Chem., Int. Ed., 2018, 57, 10034–10072 CrossRef CAS PubMed.
- C. K. Prier, D. A. Rankic and D. W. C. MacMillan, Visible Light Photoredox Catalysis with Transition Metal Complexes: Applications in Organic Synthesis, Chem. Rev., 2013, 113, 5322–5363 CrossRef CAS PubMed.
- F. Strieth-Kalthoff, M. J. James, M. Teders, L. Pitzer and F. Glorius, Energy transfer catalysis mediated by visible light: principles, applications, directions, Chem. Soc. Rev., 2018, 47, 7190–7202 RSC.
- J. Twilton, C. Le, P. Zhang, M. H. Shaw, R. W. Evans and D. W. C. MacMillan, The merger of transition metal and photocatalysis, Nat. Rev. Chem., 2017, 1, 0052 CrossRef CAS.
- R. Brimioulle, D. Lenhart, M. M. Maturi and T. Bach, Enantioselective Catalysis of Photochemical Reactions, Angew. Chem., Int. Ed., 2015, 54, 3872–3890 CrossRef CAS PubMed.
- A. R. Allen, E. A. Noten and C. R. J. Stephenson, Aryl
Transfer Strategies Mediated by Photoinduced Electron Transfer, Chem. Rev., 2022, 122, 2695–2751 CrossRef CAS PubMed.
- P. Melchiorre, Introduction: Photochemical Catalytic Processes, Chem. Rev., 2022, 122, 1483–1484 CrossRef CAS PubMed.
- A. Hossain, A. Bhattacharyya and O. Reiser, Copper's rapid ascent in visible-light photoredox catalysis, Science, 2019, 364, eaav9713 CrossRef PubMed.
- F. Yang, J. Koeller and L. Ackermann, Photoinduced Copper-Catalyzed C−H Arylation at Room Temperature, Angew. Chem., Int. Ed., 2016, 55, 4759–4762 CrossRef CAS PubMed.
- Z. Zhang, Y. Xu, Q. Zhang, S. Fang, H. Sun, W. Ou and C. Su, Semi-heterogeneous photo-Cu-dual-catalytic cross-coupling reactions using polymeric carbon nitrides, Sci. Bull., 2022, 67, 71–78 CrossRef CAS PubMed.
- X. Ma and G. Zhang, Visible Light-Induced Copper-Catalyzed C—H Arylation of Benzoxazoles, Chin. J. Chem., 2020, 38, 1299–1303 CrossRef CAS.
- I. Choi, V. Müller, G. Lole, R. Köhler, V. Karius, W. Viöl, C. Jooss and L. Ackermann, Photoinduced Heterogeneous C−H Arylation by a Reusable Hybrid Copper Catalyst, Chem.–Eur. J., 2020, 26, 3509–3514 CrossRef CAS PubMed.
- Y. Qiu, A. Scheremetjew, L. H. Finger and L. Ackermann, Electrophotocatalytic Undirected C−H Trifluoromethylations of (Het)Arenes, Chem.–Eur. J., 2020, 26, 3241–3246 CrossRef CAS PubMed.
- J. Struwe and L. Ackermann, Photoelectrocatalyzed undirected C–H trifluoromethylation of arenes: catalyst evaluation and scope, Faraday Discuss., 2023, 247, 79–86 RSC.
- W. Wei, S. L. Homölle, T. von Münchow, Y. Li, I. Maksso and L. Ackermann, Photoelectrochemical Si–H and Ge–H activation by iron catalysis, Cell Rep. Phys. Sci., 2023, 4, 101550 CrossRef CAS.
- K. Korvorapun, J. Struwe, R. Kuniyil, A. Zangarelli, A. Casnati, M. Waeterschoot and L. Ackermann, Photo-Induced Ruthenium-Catalyzed C−H Arylations at Ambient Temperature, Angew. Chem., Int. Ed., 2020, 59, 18103–18109 CrossRef CAS PubMed.
- J. Struwe, K. Korvorapun, A. Zangarelli and L. Ackermann, Photo-Induced Ruthenium-Catalyzed C−H Benzylations and Allylations at Room Temperature, Chem.–Eur. J., 2021, 27, 16237–16241 CrossRef CAS PubMed.
- E. H. Discekici, N. J. Treat, S. O. Poelma, K. M. Mattson, Z. M. Hudson, Y. Luo, C. J. Hawker and J. Read de Alaniz, A highly reducing metal-free photoredox catalyst: design and application in radical dehalogenations, Chem. Commun., 2015, 51, 11705–11708 RSC.
- S. O. Poelma, G. L. Burnett, E. H. Discekici, K. M. Mattson, N. J. Treat, Y. Luo, Z. M. Hudson, S. L. Shankel, P. G. Clark, J. W. Kramer, C. J. Hawker and J. Read de Alaniz, Chemoselective Radical Dehalogenation and C–C Bond Formation on Aryl Halide Substrates Using Organic Photoredox Catalysts, J. Org. Chem., 2016, 81, 7155–7160 CrossRef CAS PubMed.
- For detailed information, see the ESI.†.
- In general the mass balance accounted for unreacted starting material and primarily protodehalogention.
- S. E. Creutz, K. J. Lotito, G. C. Fu and J. C. Peters, Photoinduced Ullmann C–N Coupling: Demonstrating the Viability of a Radical Pathway, Science, 2012, 338, 647–651 CrossRef CAS PubMed.
- C. Uyeda, Y. Tan, G. C. Fu and J. C. Peters, A New Family of Nucleophiles for Photoinduced, Copper-Catalyzed Cross-Couplings via Single-Electron Transfer: Reactions of Thiols with Aryl Halides Under Mild Conditions (O °C), J. Am. Chem. Soc., 2013, 135, 9548–9552 CrossRef CAS PubMed.
- F. Bellina, S. Cauteruccio and R. Rossi, Palladium- and Copper-Mediated Direct C-2 Arylation of Azoles — Including Free (NH)-Imidazole, -Benzimidazole and -Indole — Under Base-Free and Ligandless Conditions, Eur. J. Org Chem., 2006, 1379–1382 CrossRef CAS.
|
This journal is © The Royal Society of Chemistry 2024 |