DOI:
10.1039/D4RA04569F
(Paper)
RSC Adv., 2024,
14, 26788-26800
Pristine and aurum-decorated tungsten ditellurides as sensing materials for VOCs detection in exhaled human breath: DFT analysis
Received
22nd June 2024
, Accepted 14th August 2024
First published on 23rd August 2024
Abstract
In this research, we employed density functional theory (DFT) to evaluate the sensing capabilities of transition metal-decorated two-dimensional WTe2 TMDs nanosheets toward VOCs such as (acetone, ethanol, methanol, toluene, and formaldehyde) that are exhaled in human breath and can serve as potential biomarkers for detecting specific physiological disorders and also gases interfering in exhaled breath (CO2 and H2O) detection. Au can be physically decorated onto the surface of WTe2. We analyzed the density of states (DOS), adsorption energy, charge transfer, and sensing behavior. The pristine WTe2 monolayer, exhibiting a semiconductor characteristic with a band gap of 0.63 eV, transitions to a metallic state upon Au-decoration, due to its actively stable nature and promising negative adsorption energy value, it triggers the emergence of novel states within the DOS. Computed adsorption energies of VOCs range from −0.08 to −0.57 eV, with greater interaction distances confirming the physisorption behavior of these VOCs biomarkers on Au-WTe2. Ethanol displays greater sensitivity compared to other considered VOCs. Au-WTe2 exhibits promising potential as a viable option for detecting VOCs in breath analysis applications at room temperature, owing to its excellent adsorption capabilities and sensitivity. Overall, our results highlight aurum-decorated tungsten ditelluride's potential as an efficient nano-sensor for detecting VOCs associated with early-stage lung cancer diagnoses.
1. Introduction
Today, detecting over 870 types of volatile organic compounds (VOCs) in human breath is crucial due to the rise in indoor air pollution, which poses risks to both the environment and human health.1–4 VOCs, due to their low binding to solid surfaces and non-reactivity, can aid in the early identification of ailments, providing a non-invasive and speedy diagnosis method.2,5 Exhaled breath primarily consists of nitrogen (78.04%), oxygen (16%), carbon dioxide (4–5%), and hydrogen, with ammonia concentrations ranging from 0.5 to 2 ppm and carbon monoxide concentrations ranging from 0 to 6 ppm.6 Breath analysis is a diagnostic tool used to identify significant diseases like liver cirrhosis, multiple sclerosis, skin conditions, central nervous system disorders, and various types of cancer.7 Acetone concentration in human breath is a key indicator of diabetes, with concentrations exceeding 1.7 ppm in type 2 and 2.2 ppm in type 1 diabetics. Formaldehyde, a carcinogenic, irritant, and toxic substance, can also cause respiratory diseases.8 Dongzhi Zhang et al. have made significant strides in sensor technology, exploring innovative materials and mechanisms for self-powered, high-performance detection of formaldehyde, hydrogen, and tactile sensing in wearable applications. Their investigation focuses on a room-temperature formaldehyde sensor constructed from an MXene/Co3O4 composite and a ZnO/MXene nanowire array piezoelectric nanogenerator. The study examines the sensor's self-powering capability and the synergistic interactions between MXene and Co3O4, highlighting its potential for wearable applications.9 They investigated at 300 °C, a new sandwich-structured hydrogen gas sensor made of Ag nanoparticles, SnO2, and an electron supply layer that exhibits remarkable performance. It has long-term stability, a low detection limit, great selectivity, and quick reaction/recovery times. Strong adsorption energy and hybridization between H2 and SnO2 orbitals are responsible for the sensor's efficacy in detecting hydrogen, which makes it valuable for forecasting thermal runaway in batteries and also introduces a flexible triboelectric nanogenerator sensor for wireless, self-powered tactile sensing, and intelligent material recognition in wearable electronics and smart sensing. They also developed a wearable triboelectric nanogenerator (S-TENG) with stability, large-scale detection, and energy harvesting, making it ideal for electronic devices and self-powered wearable sensors.10–12 Shuaipeng Wang et al.13 introduced a novel cantilever array biosensor to detect biomarkers for liver cancer, while Nir Peled et al.14 Lung cancer research focuses on volatile emissions linked to cancer-specific mutations, highlighting the need for nanostructured chemiresistive gas sensors for rapid, sensitive, and cost-effective detection.15 Yuvaraj et al. investigated Sc2CO2 MXene nanosheets that detected volatile organic compounds in human breath, showing high sensitivity to acetonitrile and potential for room-temperature illness sensors.16 Transition Metal Di-chalcogenide (TMD), a 2-D material, is widely used as a sensing material in biosensors.17 Molybdenum and tungsten dichalcogenides are well-regarded 2D layered materials because of their distinctive chemical and physical properties.18 TMD, including MoSe2, WS2, MoS2, and MoTe2, are two-dimensional materials with few layers with potential applications in energy storage, catalysis, optoelectronics, and microelectronics.19–22 WTe2, with superconductivity and topological states, is a promising candidate for studying topological superconductivity and its role in quantum computing applications.23 WTe2 is a TMDC known for its unique electronic structure and optical properties, with electron mobility exhibiting a pattern of WS2 < WSe2 < WTe2.24 Nan Gao et al. evaluates the adsorption and sensing capabilities of six VOCs on both pristine and Li-doped F-diamine, suggesting its suitability as a sensor for detecting C2HCl3.25 R. Chandiramouli et al. explored the adsorption properties, rapid recovery times, and resistance changes of MoSe2 nanosheets when exposed to ML and EL vapor molecules.26 Hao Cui et al. examined the operating conditions of oil-immersed transformers and assesses the potential of Ni-doped WTe2 monolayer to improve adsorption and sensing in transformer oil.27 Zhang et al. investigated that the Pd-decorated WTe2 monolayer exhibits promising gas sensing capabilities for SO2 and SOF2.28 Xiqian Hu et al. explored that Pd-doped MoTe2 enhances gas sensing by reducing the band gap, increasing conductivity, and improving adsorption compared to pure MoTe2.29 Ran Jia et al. investigated the adsorption of VOCs in breath, aiming to develop rapid and cost-effective screening methods for early lung cancer detection.30 In this study, we used DFT to assess the effectiveness of pristine WTe2 and Au-decorated WTe2 in detecting VOCs in human breath. We analyzed their electronic properties, such as band structure and density of states, ELF, and studied WTe2's adsorption behavior towards different VOCs, including alcohols, ketones, nitriles, and hydrocarbons. Comparisons with other 2D materials highlighted WTe2 superior adsorption capabilities. Hirshfeld charge analysis quantified charge transfer upon VOCs adsorption, while we evaluated gas-sensing performance in terms of recovery time, sensitivity, and selectivity. Additionally, we explored how VOC adsorption affects WTe2 work function, suggesting its potential as a work function-based sensor for early disease diagnosis through breath analysis.
2. Methods
2.1. Calculation methods
The ADF-BAND package was utilized to analyze energy parameters, configuration, gas adsorption, and electronic simulation models using the DFT methodology. Realistic orbital behavior around the nucleus within the examined structure was accomplished by employing ADF's Slater-type orbitals (STOs).31,32 The atomic, and electronic wave functions were considered using a triple zeta polarization (TZP) basis set, standard numerical precision, and a non-frozen core approach.33 Investigation delved into the influence of electron density on ion exchange-correlation energies by employing Perdew–Burke–Ernzerhof (PBE) generalized gradient approximation (GGA).34 Incorporating van der Waals (vdW) interactions through D3-Grimme correction (DFT-D3) notably enhances the precise depiction of long-range interaction between gas molecules and Au-decorated WTe2.35 Fully optimized lattice constants and atomic positions were attained using five (05) k-points. Selection of k-grid, basis set, and density fitting parameters critically influences numerical accuracy in ADF. These chosen k-points, derived from Becke's fuzzy cell technique, are pivotal in sampling the Brillouin zone and establishing numerical precision in calculations.36 Convergence criteria for structure relaxation were set, including energy (10−5 eV), gradient (0.02 eV Å−1), and step size (10−3 Å). These criteria were consistently applied to all relaxation processes.37 The convergence criteria in the ADF-BAND package are designed to ensure the reliability of the results by requiring that iterative calculations reach a stable solution. These criteria typically involve thresholds for changes in total energy, electron density, band structure convergence, and forces. By adhering to these stringent criteria, the package guarantees that the results are accurate, reproducible, and reliable for further analysis.
2.2. Analytical methods
The stability of WTe2, a monolayer, decorated with Au, is often assessed using binding energy Eb, as described by eqn (1). |
Eb = EWTe2/Au − EWTe2 − EAu
| (1) |
In which EWTe2/Au signify the energy of transition metal (Au) decorated TMDs, EAu denotes the energy of aurum metal, and EWTe2 signify the energy of the WTe2 monolayer. Term adsorption energy Eads is used to characterize interaction energy and constancy between VOCs and TMDs, which is as eqn (2):
|
Eads = Etotal − EVOC −Esubstrate
| (2) |
where
Etotal is the energy of a total adsorbed system and
EVOC denotes the total energy of the gas molecules and
Esubstrate energy of Au-WTe
2. Hirshfeld analysis was employed concurrently to examine charge transfer (Δ
Q) between the target molecule and the adorned surface, as expressed in
eqn (3):
A positive ΔQ signifies an electron donor, while a negative electron-accepting behavior in the target molecule. Conductivity (σ) and sensitivity of the WTe2 base monolayer and adsorption system can be predicted using eqn (4) and (5) based on frontier orbital theory.
|
 | (4) |
|
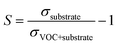 | (5) |
A smaller band gap Eg, combined with constant A, Boltzmann constant (k), and temperature (T), implies higher conductivity at a given temperature. This correlation is crucial for investigating the electrical properties effectively of Au-WTe2 for gas sensing at different temperatures and σVOC+substrate and σsubstrate electrically conductive values of Au-decorated WTe2 with VOCs, and Au-WTe2 respectively. Additionally, the ability of the Au-WTe2 monolayer to recover can be examined through van't Hoff–Arrhenius/transition state theory and is expressed in eqn (6).38,39
|
 | (6) |
A, attempted frequency, is determined to be 10
12 s
−1.
Eads represent adsorption energy,
kB and
T denote Boltzmann constant, and the sensor's temperature (8.6173303 × 10
−5 eV K
−1).
25,29,40,41 A valuable approach for gas sensors includes tracking changes in the work function of the sensing substrate. The work function (
Φ), calculated as.
V(
Φ) represents electrostatic potential relative to vacuum level, and
Ef denotes potential relative to Fermi energy level. Variable
Φ responds to surface changes induced by gas adsorption.
16,42
3. Results and discussion
3.1 Structural property of pristine and Au-decorated WTe2
Firstly, the honeycomb structure of pristine WTe2 monolayer (WTe2-ML) was configured with a supercell of dimensions 4 × 4 × 1, housing a total of 16 W atoms and 32 Te atoms with W atoms positioned between two Te sublayers, as illustrated in Fig. 1(a). According to our computations, the lattice constant of the WTe2 monolayer is determined to be 3.54 Å, the W–Te bond length measures 2.70 Å, and the bond angle is 72.3° is shorter, indicating a smaller atomic radius compared to the previously reported value of 2.76 Å bond length remains consistent after optimization. These values align with a previous theoretical report indicating a lattice constant of 3.6 Å.43 WTe2 primarily exists in 2H- and Td-phases, the Td-phase, often referred to as 1T-phase, has received the most research attention. Furthermore, because of its remarkable superconducting features, the T-phase of WTe2 has garnered interest.23,44,45 For dynamic stability of WTe2 phonon dispersion in the reported structure.46 WTe2-ML was optimized to attain relaxed geometric configuration and electronic properties of decorated ad-atom and adsorbed gases.
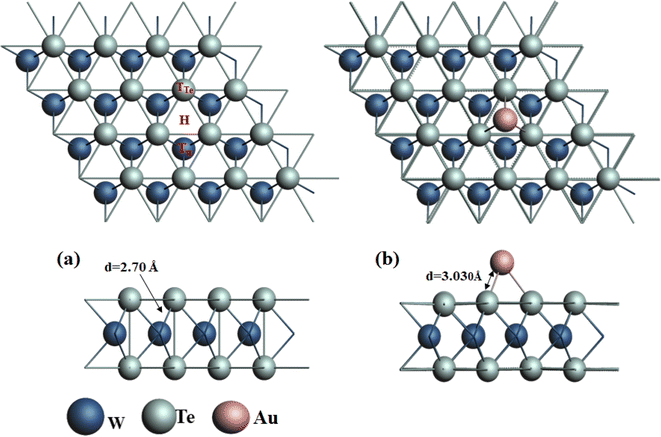 |
| Fig. 1 (a) Geometric structure of pristine WTe2 with three typical locations and (b) Au-decorated WTe2. | |
Notably, decorating with metal elements is an effective strategy to enhance the sensitivity of adsorbent. The adsorption performance of the WTe2 was enhanced by decorating this with Au atom. For the most stable configuration of Au-WTe2, three distinct locations on pristine WTe2 monolayer surfaces were examined for ideal locations for VOCs. These positions encompassed the Tw site positioned over the W atom, the TTe site positioned over the Te atom, and the H site located overhead center of the six-membered ring as illustrated in Fig. 1(a). The optimal configuration for Au-decoration on the WTe2 monolayer is found at the H site, exhibiting the highest interaction strength with Eb of −8.61 eV which indicates Au-WTe2 configuration showed superior stability and strong interaction at the H site, with Au decoration forming a bond with three Te atoms with bond lengths of 3.030 Å is illustrated in Fig. 1(b).
The bond length between W and Te atoms was 2.7 Å. The Au atom is adsorbed on the hollow site of WTe2. In this structure, the W atoms are sandwiched between the Te atoms, as shown in Fig. 1. The hexagonal structure of WTe2 has three Te and three W atoms, and only all Te atoms form a bond with Au, with a bond length of 3.030 Å.
3.2 Electronic properties of pristine and Au-decorated WTe2
Investigated electronic properties of Au-decorated WTe2 compared to pure WTe2 by examining total DOS and projected DOS, as depicted in Fig. 2(a–c). DOS analysis of pristine WTe2 indicates an absence of states at the Fermi level, suggesting its semiconducting nature with a bandgap of 0.63 eV. Moreover, the TDOS and PDOS curves of Au-WTe2 shift to a higher energy region after Au decoration. This shift is attributed to the electron-donating behavior of WTe2, leading to an improved effective Coulomb potential. Additionally, a transition from semiconducting to metallic behavior is observed. Comparison with the pristine WTe2 system reveals that Au-decoration introduces novel states, some of which fill the bandgap, transforming the Au-decorated WTe2 monolayer into a metallic state. Atomic DOS analysis demonstrates significant overlap between Au 5d, 6s orbital, and Te 5p orbital at −1.0 and 1.5 eV, indicating durable orbital interactions between Au and Te atoms. This interaction further contributes to alteration in the electronic states of the Au-decorated WTe2 system.
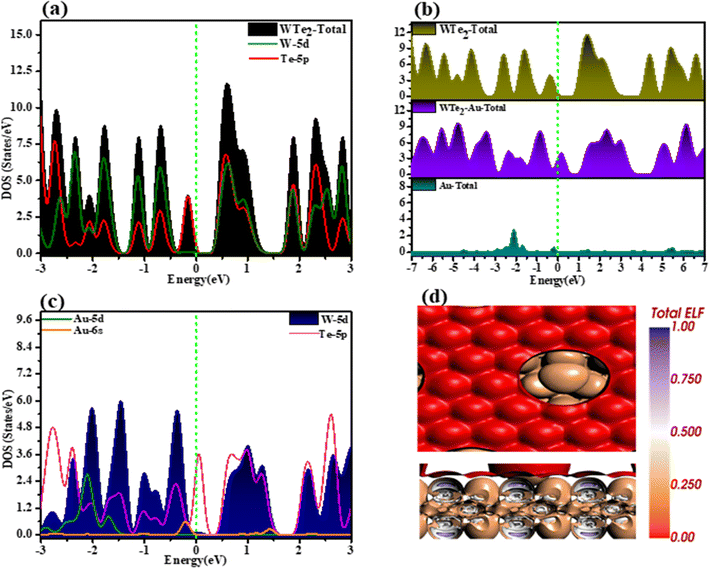 |
| Fig. 2 (a–c) TDOS and PDOD curves of pristine WTe2, Au-decorated WTe2, and green dashed lines are Fermi level, (d) ELF of Au-WTe2. | |
In the context of Hirshfeld analysis, Au exhibits a negative charge of −0.211e, acting as an electron acceptor and withdrawing 0.063e from WTe2. Conversely, each of the three bonded Te atoms carries a positive charge of 1.276e, demonstrating an electron-donating behavior. W atoms act as electron acceptors, whereas Te atoms act as donors. This highlights the electronegativity of W and Te atoms within a system, along with newly formed Au–Te bonds. Moreover, Au–Te bonds display electron accumulation, confirming the electron-donating property of Te atoms and emphasizing robust orbital interactions in Au–Te bond formation.
We have calculated ELF which is a computational tool used to visualize different degrees of electron localization and the value lies in the range of 0 to 1. Where 0 represents delocalized electrons (metallic behavior), and 1 indicates highly localized electrons (covalent or ionic behavior) also calculation is used to understand chemical bonds and interactions that is illustrated in Fig. 2(d). It provides insights into electron pairs and bonding types through varied colors. Red on WTe2 indicates strong electron localization associated with covalent or directional bonds and signifies regions with high electron localization while brown hues on Au suggest lower electron localization characteristic of metallic bonding and indicate specific electron densities that are known for their metallic properties and in the context of WTe2 sheets, they likely form metallic bonds.
3.3. Structures of adsorption and electronic properties of VOCs over Au-decorated WTe2
Investigation delves into enduring adsorption, dynamics, and electronic configuration of gases onto pristine WTe2 and decorated with metal (Au). Results indicate that some gas molecules adsorbed physically on the structure. The adsorption energies of WTe2 towards gases are classified from higher to lower as follows: CH4O > CO2 > C2H5OH > C7H8 > C3H6O > H2O, CH2O. However, the incorporation of the Au atom significantly enhances WTe2 adsorption capabilities. Decorating WTe2 with Au atom notably augments the monolayer's capacity to adsorb gas molecules, with increasing adsorption hierarchy arranged as follows: C2H5OH > C7H8 > H2O > CH2O > CO2 > CH4O > C3H6O as summarized in Table 1.
Table 1 Theoretically reviewed (Eads) eV of VOCs with other 2D nano-sensors
Sensing materials |
H2O |
CO2 |
CH2O |
CH4O |
C7H8 |
C3H6O |
C2H5OH |
References |
WTe2 |
−0.01 |
−0.09 |
−0.01 |
−0.10 |
−0.07 |
−0.06 |
−0.08 |
This work |
Au-WTe2 |
−0.28 |
−0.14 |
−0.24 |
−0.11 |
−0.55 |
−0.08 |
−0.57 |
This work |
BC6N |
— |
— |
−0.22 |
— |
— |
— |
−0.37 |
38 |
Tellurene-MoS2 |
— |
— |
— |
— |
— |
−0.32 |
−0.21 |
47 and 48 |
Phosphorus |
— |
— |
— |
— |
−0.43 |
— |
−0.21 |
49 |
MoS2 |
— |
— |
−0.25 |
— |
— |
— |
−0.25 |
50 |
B4C3 |
−0.19 |
−0.15 |
−0.19 |
— |
−0.21 |
— |
— |
51 |
N-WS2 |
−0.15 |
— |
— |
— |
— |
— |
— |
30 |
Sc2CO2 |
−0.44 |
−0.19 |
— |
— |
−0.18 |
−0.48 |
−0.59 |
16 |
3.3.1 Adsorption and electronic properties of C7H8 onto Au-WTe2. After optimization C7H8, it is evident that gas molecules predominantly favor adsorption on Au-WTe2 via C atoms. Gas molecules adsorbed parallel to the surface of Au-WTe2 with adsorption energy (Eads) amounting to −0.55 eV as illustrated in Fig. 3(a). These shift results are significantly higher than C7H8/WTe2, as seen in Table 1. Post-optimization, the bond distance between Au and gas atoms is 3.90 Å which indicates weak interaction because the distance is greater than 3 Å.52
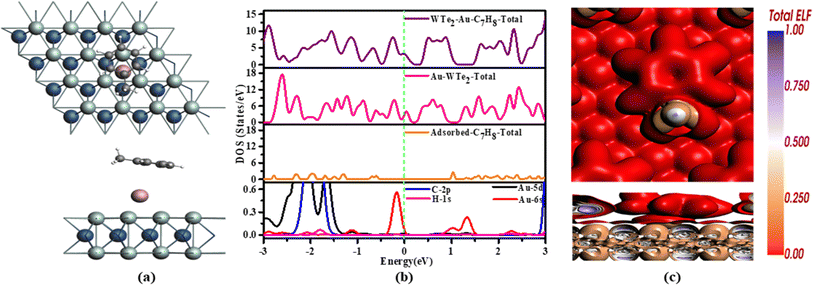 |
| Fig. 3 (a) Configuration of C7H8 adsorption (b) TDOS and PDOS (c) ELF of C7H8. | |
The electronic properties of Au-WTe2 during C7H8 adsorption are illustrated in Fig. 3(b). TDOS analysis shows that adsorbed gas does not affect the material's metallic behavior, indicating that Au plays a more significant role in determining metallic qualities than the influence of gas. PDOS also reveals that C-2p and H-1s orbitals overlap with Au-5d, and 6s orbitals at −0.3 eV and 1.3 eV also strong hybridization is seen in the valence band.
The results of the Hirshfeld charge analysis, shown in Fig. 10, indicate that C7H8 acts as an acceptor during the adsorption process. The white and brown hues observed on C7H8 indicate localized electron regions, highlighting the presence of covalent bonds between carbon and hydrogen atoms within C7H8, as illustrated in Fig. 3(c). On Au-WTe2, the red color reflects areas of elevated electron density or localization, implying a robust interaction between Au atoms and WTe2. This suggests a mix of covalent bonding within C7H8 and the possibility of metallic bonding between Au and WTe2 in adsorbed configuration.
3.3.2 Adsorption and electronic properties of CH2O onto Au-WTe2. Adsorption of CH2O on Au-WTe2 monolayer is depicted in Fig. 4(a), revealing a notable distance of 2.694 Å between Au and a carbon (C) atom showcasing a parallel tilted positioning. Despite the improvement in (Eads) of CH2O/Au-WTe2 is −0.24 eV compared to CH2O/WTe2 with a calculated Eads of −0.01 eV, it is inferred that the presence of Au decoration minimally impacts the adsorption performance of WTe2 monolayer concerning CH2O molecules.
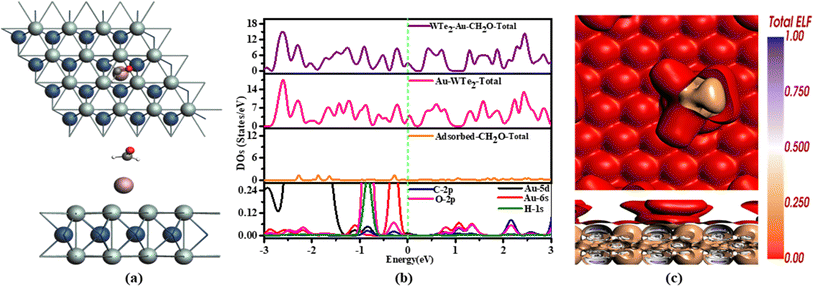 |
| Fig. 4 (a) Adsorption configuration of CH2O (b) TDOS and PDOS (c) ELF of CH2O. | |
After adsorption, Au-WTe2 material acts as a donor while CH2O functions as an acceptor. It is noticeable that the oxygen atom closest to the Au atom shows a subtle interaction with Au-WTe2, while the carbon atom displays some extent of charge transfer with an oxygen atom, acting as a donor, as depicted in Fig. 10.
Investigation of CH2O adsorption on an Au-WTe2 entails DOS examination. PDOS interaction between Au-5d, 6s and C-2p, O-2p, and H-1s orbitals overlap at −0.3 eV and 2.1 eV and strong hybridization can be seen at −0.3 to −1.2 eV while (TDOS) overlap with Au and CH2O/Au-WTe2 at 2.4 eV is illustrated in Fig. 4(b). However, peaks of adsorbed CH2O near the Fermi level indicate weak adsorption, contrasting with Au robust interactions. While CH2O/Au-WTe2 displays metallic behavior, the adsorbed gas lacks this trait, suggesting Au predominantly drives the material's metallic properties rather than the adsorbed gas contribution.
Color-coded representation provided by ELF highlights specific electron densities, with brown shading on CH2O indicating the presence of pi-electron density associated with its pi-system. This observation suggests a potential for pi-interactions with Au-WTe2. Additionally, red coloring observed on Au-WTe2 points to electron localization resulting from gas interaction, implying a chemical interaction and electron redistribution at the surface is illustrated in Fig. 4(c).
3.3.3 Adsorption and electronic properties of C3H6O onto Au-WTe2. After optimization of C3H6O on the surface of Au-WTe2, the C3H6O appeared as almost parallel and the bond distance between Au–H atoms increased from 2.927 to 3.029 Å indicating elevated physisorption16,52 illustrated in Fig. 5(a). This arrangement leads to an acetone adsorption energy of −0.08 eV, which is strong compared to C3H6O/WTe2, which can be obtained using eqn (2).
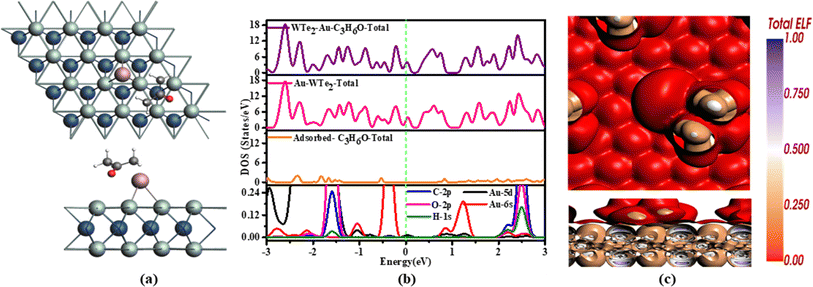 |
| Fig. 5 (a) Configuration of C3H6O adsorption (b) TDOS and PDOS (c) ELF of C3H6O. | |
Fig. 5(b) indicates the DOS distribution of C3H6O. We can affirm limited contact between C3H6O and Au-WTe2 signifying minimal charge transfer during adsorption and showing metallic behavior due to less contribution of gas molecules. Furthermore, PDOS orbital overlap very strongly at 2.2 eV and hybridization at 2.1 to 2.7 eV especially Au-5d overlap at the Fermi level. Only a discernible change in TDOS at 2.2 eV for C3H6O/Au-WTe2 compared to Au-WTe2, slight elevation of peaks, and noticeable hybridization at −0.3 to −2.4 eV in PDOS orbitals.
Hirshfeld charge analysis indicates gas molecules exhibit an electron-accepting behavior, while Au-WTe2 serves as a donor. In the C3H6O system, Au-WTe2 demonstrates a robust electron-donating propensity upon gas adsorption, thereby affirming its exceptional chemical activity and reactivity in gas interaction scenarios.
Fig. 5(c) illustrates ELF Within C3H6O, white regions signify areas of lower electron densities, whereas brown regions denote specific electron densities like pi-electron, localized electron distributions, or distinctive bonding features within C3H6O molecule. The red hue on the Au-WTe2 sheet indicates particular electron distributions or localized densities on this surface. This suggests electron localization stemming from interactions between surface Au and absorbed acetone molecule respectively.
3.3.4 Adsorption and electronic properties of H2O onto Au-WTe2. Before optimization oxygen atom is sited vertically at Au but after adsorption H2O is inclined to the Au-WTe2 surface as illustrated in Fig. 6(a). Corresponding Au with H-atom bond length before and after are 763, and 2.387 Å respectively. This decrease in bond length suggests that the optimized configuration is more stable, with potentially stronger interactions between H2O/Au-WTe2 due to elevated physisorption this arrangement leads to an H2O adsorption energy of −0.28 eV, which is strong as compared to H2O/WTe2.
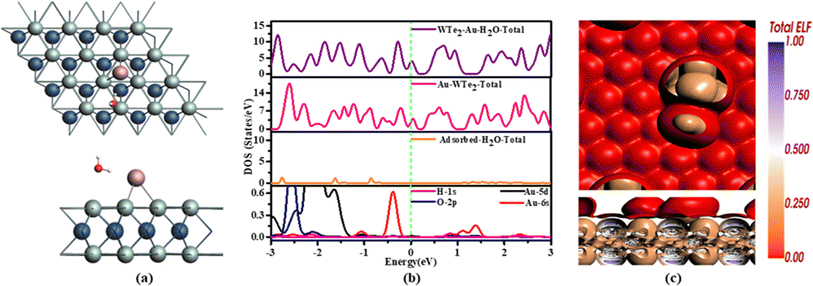 |
| Fig. 6 (a) Configuration of H2O adsorption (b) TDOS and PDOS (c) ELF of H2O. | |
Au-WTe2 demonstrates electron transfer behavior, while H2O acts as an acceptor by withdrawing electrons from a substrate, indicating a strong accepting property. It becomes evident that the oxygen atom exhibits a weak interaction with Au-WTe2, while the hydrogen atom displays some degree of charge transfer with an oxygen atom, behaving as a donor. WTe2 suggests minimal charge transfer from a surface to adsorbed gas.
DOS distribution in Fig. 6(b) demonstrates electronic changes in the Au-WTe2 system upon H2O adsorption. In H2O, the peak at the Fermi level weakens, while new peaks emerge around −0.3 eV and −0.9 eV. A significant decrease in the PDOS peak of Au-5d at the Fermi level provides compelling evidence compared to the isolated Au-WTe2 system. Moreover, the emergence of a novel peak in PDOS around 1.5 eV due to H2O suggests hybridization with Au-5d.
ELF analysis suggests strong electron localization on both Au-WTe2 sheets and adsorbed water molecules. ELF values on Au-WTe2 sheets indicate strong electron localization in these regions. Brown coloring on hydrogen and oxygen atoms signifies high ELF values, indicating localized electron density around these atoms which is illustrated in Fig. 6(c).
3.3.5 Adsorption and electronic properties of CO2 onto Au-WTe2. After the optimization configuration of CO2 indicating physisorption with Eads −0.14 eV, and bond length 2.45 Å that is related to the previous report.41 These observed changes provide compelling evidence for the activation of both CO2 and Au-WTe2 during the adsorption process which is illustrated in Fig. 7(a).
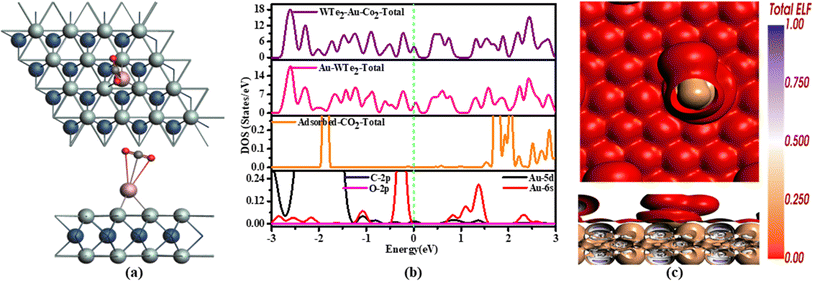 |
| Fig. 7 (a) Configuration of CO2 adsorption (b) TDOS and PDOS (c) ELF of CO2. | |
The DOS analysis of CO2 emphasizes the pivotal role of Au-5d orbitals in electron transfer, increasing electron density and surface electronegativity seen in Fig. 7(b). These peaks consistently align at 0 eV, indicating substantial electron transfer. Conversely, Au-6s orbitals show minimal change, maintaining their position at −1.2 eV suggesting limited involvement in electron transfer during the adsorption mechanism indicating that both Au and the gas contribute to electron transfer, playing a key role in imparting metallic properties to the material.
Hirshfeld charge analysis indicates that during the adsorption process of CO2, functions as an acceptor in this particular arrangement from Au-WTe2 and Au-decorating WTe2 emphasizing notable electron-donating characteristics. At the same time, the carbon atom donates a charge of 0.266e to an oxygen atom.
ELF in CO2 that is illustrated in Fig. 7(c) red color on Au might designate a durable interaction between gold and WTe2 and lead to a stronger interaction compared to purely metallic bonding. Analysis shows brown hues on CO2, indicating specific electron densities associated with their pi-electron systems.
3.3.6 Adsorption and electronic properties of CH4O onto Au-WTe2. The CH4O adsorbs on Au-WTe2, with a preference parallel to the Au site. This configuration shows physisorption with Eads −0.11 eV and 2.387 Å bond length with Au–H, indicating activation of both VOC molecules and Au-WTe2 as shown in Fig. 8(a).
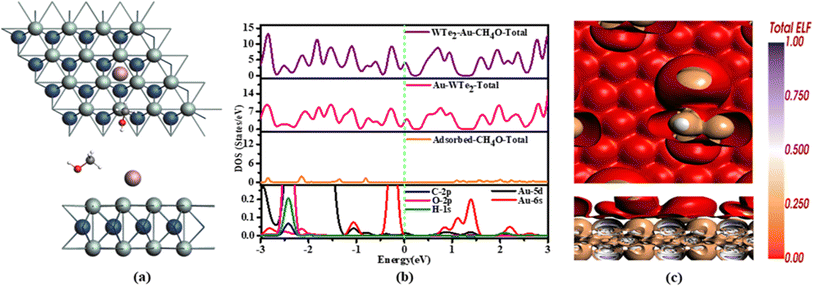 |
| Fig. 8 (a) Configuration of CH4O adsorption (b) TDOS and PDOS (c) ELF of CH4O. | |
TDOS configuration of Au-WTe2 is changed after gas adsorption. Au-WTe2 peak shift to the higher region nearly close to Fermi level but after a gas adsorbed shift at Fermi level with same height and analysis show that adsorbed gas has no effect on material's metallic behavior, indicating that Au plays a more significant role in determining material's metallic qualities and PDOS interaction between Au-5d, 6s and C-2p, O-2p, H-1s orbitals overlapped at −2.4 eV and hybridized at 0.6 to 1.5 eV and −0.3 to −1.2 eV is illustrated in Fig. 8(b).
Hirshfeld charge analysis suggests that during the adsorption process, CH4O acts as an acceptor within this specific arrangement involving Au-decorated WTe2, highlighting its significant electron-donating properties. Simultaneously, the carbon atom exhibits an electron-withdrawing effect on hydrogen and oxygen atoms within the molecule.
ELF of CH4O shows metallic interaction between gold atoms, possibly forming metal–metal bonds within the gold. Additionally, there might be some sort of interaction between the gas atoms and the material, possibly involving charge transfer or electronic transitions between the gas atoms and the material's orbitals illustrated in Fig. 8(c).
3.3.7 Adsorption and electronic properties of C2H5OH onto Au-WTe2. Fig. 9(a) depicts the adsorption arrangement of the C2H5OH system on Au-WTe2. The C2H5OH molecule optimized on Au-WTe2 in tilt form and slightly perpendicular to interact with the Au atom after optimization. This orientation is preferred due to its enhanced stability and stronger interactions, as indicated by the decrease in bond lengths between Au and the H-atom of the gas from 2.375 Å to 2.371 Å post-optimization. This decrease indicates a more stable configuration and suggests potentially stronger interactions between the molecule and the surface.25 Strong interaction between the gas molecule and the adsorbent surface induces significant deformation in the geometric structure of Au-WTe2, resulting in a high Eads of −0.57 eV, stronger than other gases and C2H5OH/WTe2 interactions,29,53 the Au-WTe2 system demonstrates superior interaction strength and also changes electronic configuration that is roughly related to the previous report.54 This enhanced adsorption energy suggests that the Au decoration significantly improves the material's sensitivity and effectiveness for detecting C2H5OH, making it a more promising candidate for sensor applications.
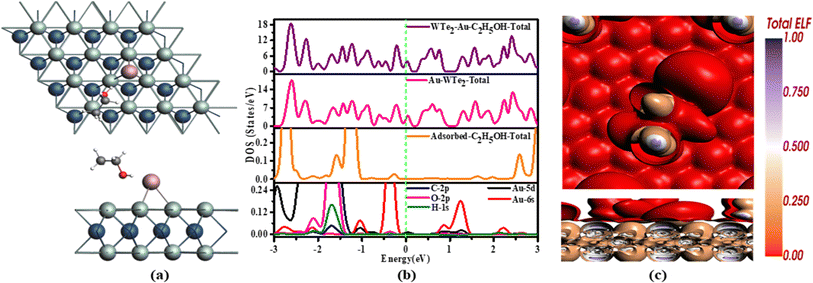 |
| Fig. 9 (a) Configuration of C2H5O adsorption (b) TDOS and PDOS (c) ELF of C2H5OH. | |
C2H5OH demonstrates an accepting nature, while the Au-WTe2 material serves as a donating behavior. Au-decorated WTe2 exhibits a strong capability for electron donation upon the adsorption of C2H5OH. This emphasizes its notable chemical activity and reactivity in gas interaction scenarios as in Fig. 10.
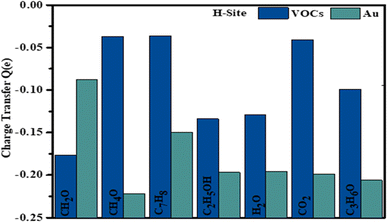 |
| Fig. 10 Charge transfer of system. | |
TDOS, giving rise to innovative peaks in DOS of C2H5OH structure. This deformation leads to the disintegration of electronic states around the Fermi level into quite a few smaller states around 2.1 eV and at −0.3 eV. From PDOS we can see the hybridization of C-2p, O-2p, H-1s, and Au 5d, 6s orbitals in the range from −2.3 eV to 1.4 eV as depicted in Fig. 9(b). Similar to observations in the system, it is evident that TDOS of the C2H5OH system has an activated peak at the Fermi level that indicates metallic behavior of C2H5OH/Au-WTe2 similar to Au-decorated WTe2. This analysis highlights the profound impact of Au-WTe2 interaction on electronic structure, with implications for the adsorption characteristics of C2H5OH on the surface.
ELF, a computational tool, unveils electron distributions, facilitating the comprehension of chemical bonds and interactions by displaying varied colors. Brown hues on C2H5OH indicate specific electron densities related to their pi-electron systems, hinting at potential pi-interactions with the Au-decorated WTe2 surface. Conversely, red coloring on Au-WTe2 denotes electron localization due to interactions with the adsorbed gas, suggesting the emergence of chemical interactions illustrated in Fig. 9(c).
4. Gas-sensing interpretation
4.1 Sensitivity and selectivity
In assessing the effectiveness of Au-decorated WTe2 as a gas sensor for CH2O, C7H8, CH4O, C2H5OH, CO2, C3H6O, and H2O molecules, attention must be paid to both their adsorption characteristics and electrical conductivity. The sensor's sensitivity relies on detecting changes in conductivity resulting from variations in the bandgap. Specifically, a reduction in bandgap leads to heightened electrical conductivity. To gauge sensor performance, key metrics such as sensitivity (S) and electrical conductivity (σ) are computed, indicating the material's ability to detect gases effectively calculated by using eqn (4) and (5).25,55 After adsorbing VOCs, bandgap values of Au-WTe2 remain at 0 eV, indicating a potential enhancement in conductivity. This phenomenon aligns with observations in other resistance-type sensors, where increased conductivity is often noted.25,56,57 Another aspect of device performance to consider is selectivity. Previous research indicated that selective detection among multiple species could be achieved by observing changes in either bandgap or conductivity.57 Certain studies have utilized sensitivity ratios of specific molecules to measure selectivity. Even when adsorption energy and bandgap values are comparable across various adsorption systems, certain sensors have demonstrated remarkable selectivity.38,58
With a bandgap of 0 eV across all VOCs, adsorbed systems exhibit a sensitivity of 100% for each gas which can be seen in Table 2. This implies that high sensitivity and selectivity are anticipated for qualitatively detecting VOCs through conductivity and selectivity measurements.
Table 2 VOCs sensing performance on Au-decorated WTe2
VOCs molecule |
Adsorption energy (eV) |
Band gap (Eg) |
Bond length (Å) |
Work function (eV) |
Work function sensitivity (%) |
Charge Q(e) |
Sensitivity (%) |
H2O |
−0.28 |
0 |
2.38 |
3.9475 |
−0.49 |
−0.129 |
100 |
CO2 |
−0.14 |
0 |
2.45 |
4.0149 |
1.20 |
−0.041 |
100 |
CH2O |
−0.24 |
0 |
2.69 |
4.2326 |
6.69 |
−0.177 |
100 |
CH4O |
−0.11 |
0 |
2.38 |
3.7956 |
−4.30 |
−0.037 |
100 |
C7H8 |
−0.55 |
0 |
3.90 |
3.8839 |
−2.09 |
−0.036 |
100 |
C3H6O |
−0.08 |
0 |
3.02 |
3.8215 |
−3.66 |
−0.099 |
100 |
C2H5OH |
−0.57 |
0 |
2.37 |
3.9354 |
−0.79 |
−0.134 |
100 |
4.2 Recovery time
The recovery time (τ) is crucial for gas sensor effectiveness, as it indicates the detachment of gas molecules post-detection. A shorter τ indicates superior reversibility, highlighting the efficacy of gas sensors in detecting and releasing gas molecules.39,59,60 The study investigated Au-WTe2 desorption at 298 K, 398 K, and 498 K, analyzing potential barrier (Eads) and recovery times as shown in Table 3. High Eads in C2H5OH leads to a notable energy barrier for desorption, resulting in prolonged recovery times, like 7.97 × 10−3 s at 298 K. Conversely, C3H6O, the smallest Eads, desorbs easily from the Au-WTe2, with desorption times of 2.4 × 10−11 s at 298 K, 1.04 × 10−11 s at 398 K, and 6.4 × 10−12 s at 498 K. As the working temperature increases, the recovery time of Au-WTe2 towards VOCs decreases, especially evident in C2H5OH desorption, where time reduces to 5.89 × 10−7 s at 498 K.
Table 3 Recovery time (τ) for mentioned VOCs at different temperatures
System |
τ (s) 298 K |
τ (s) 398 K |
τ (s) 498 K |
C2H5OH |
7.97 × 10−3 |
1.90 × 10−5 |
5.89 × 10−7 |
C7H8 |
3.85 × 10−3 |
1.06 × 10−5 |
1.15 × 10−7 |
H2O |
7.31 × 10−8 |
3.77 × 10−9 |
6.83 × 10−10 |
Co2 |
2.27 × 10−10 |
6.14 × 10−11 |
2.61 × 10−11 |
CH2O |
1.47 × 10−8 |
1.16 × 10−9 |
2.68 × 10−10 |
CH4O |
8.14 × 10−11 |
2.54 × 10−11 |
1.29 × 10−11 |
C3H6O |
2.4 × 10−11 |
1.04 × 10−11 |
6.4 × 10−12 |
We assert that Au-WTe2 shows promising material for the detection of C2H5OH. This is due to its consistent adsorption performance at ambient temperature and rapid recovery time at high temperatures, facilitating its repeated and efficient use. However, the remarkably short recovery time observed for the C3H6O desorption system at 298 K and 498 K indicates that Au-WTe2 is unsuitable for use as a sensor for C3H6O. The adsorption and desorption performance of Au-WTe2 for H2O molecules at ambient temperature indicates its suitability as a water sensor under simple operating conditions.55
4.3 Work function
Adsorption of VOCs typically alters a material's work function, which is a crucial parameter in gas detection applications.61,62 The work function represents the energy required to move an electron from the Fermi level to the vacuum and is calculated by eqn (7). Alterations in the work function of a system significantly impact its electrical conductivity. The intrinsic work function of pure WTe2 is 3.42 eV, while Au-WTe2 has 3.96 eV. Upon adsorption of CO2, H2O, CH4O·C2H5OH, CH2O, C3H6O, C7H8 the work function changes. Work functions (WFs) of CH2O and CO2 systems increase to 4.23 and 4.01 eV. Consequently, CH4O adsorption leads to a significant decrease in work function compared to other gases.63,64 It's important to note that DFT calculations can reasonably predict the changes in work function induced by physisorbed gases.63 Experimentally, the change in work function due to gas adsorption is estimated using the Scanning Kelvin Probe, enabling the development of work function-based gas sensors. The work functions of pristine WTe2 and Au-WTe2 are 3.42 and 3.96 eV, respectively, suggesting weaker electron affinity compared to graphene and Ni-doped WTe2, and heightened potential for vacuum-level electron release.40,65–67 The formula to calculate the sensitivity of the work function of Au-WTe2 with gases is.68–70
The equation can be utilized to determine the sensitivity of the work function. Where Φgas and ΦAu-WTe2 represent the work function of systems with gases adsorbed respectively. These findings show varied trends in the work function of CH2O and CO2 systems. It increases to 4.23 and 4.01 eV with the sensitivity of work-function 6.69% and 1.20% respectively as shown in Fig. 11.
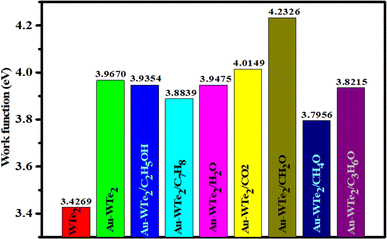 |
| Fig. 11 Comparative analysis of work function for WTe2 and Au-decorated WTe2 before and after exposure to volatile organic compounds (VOCs). | |
In contrast, other gases such as C3H6O, H2O, C7H8, C2H5OH, and CH4O exhibit decreases in WF of −3.66, −0.49, −2.09, −0.79 and −4.3%, respectively than Au-WTe2. The work function-based Au-WTe2 sensor shows high sensitivity towards CH2O compared to other gases. This is attributed to the higher Fermi energy of CH2O compared to gases, resulting in a higher work function. As a result, CH2O exhibits greater sensitivity as it requires less energy for electrons to escape from the Fermi level to infinity than other gases.
5. Conclusion
Nanosensors offer a cost-effective and non-invasive approach to early lung cancer detection. A key challenge is designing breath sensors that selectively detect specific biomarkers. In this study, the sensing capability of Au-decorated WTe2 for volatile organic compounds like acetone, ethanol, methanol, formaldehyde, and toluene commonly associated with lung cancer biomarkers, was assessed using DFT calculations. Additionally, we studied interfering gases commonly present in exhaled breath, specifically carbon dioxide and water. Our calculations indicate that the pristine WTe2 monolayer exhibits limited adsorption of the target molecules rendering it unsuitable for lung cancer detection. The Au-decoration increases the adsorption strength of VOC molecules on the WTe2 monolayer surface, extending their recovery periods to the detectable range. After accounting for the adsorption distances and charge transfers of the related VOCs, the adsorption onto the Au-WTe2 surface is primarily physisorption. Furthermore, we analyzed desorption patterns and sensing mechanisms of Au-WTe2 concerning VOCs. Au adsorbed onto WTe2 via H-sites, and electronic properties of Au-WTe2 remain unchanged following interaction with gases. Au-WTe2 demonstrates promising potential as a sensing material for VOC molecules due to its effective adsorption and desorption capabilities under specific conditions. The work function-based Au-WTe2 sensor shows high sensitivity towards CH2O compared to other gases with 6.69% respectively. However, it excels in detecting ethanol vapor molecules, making it a promising option for accurately assessing operational efficiency. We expect that the findings of this study will contribute to the development of effective Au-WTe2-based nanosensors for early lung cancer detection by targeting specific VOCs.
Data availability
All data generated or analyzed during this study are included in this published article.
Conflicts of interest
The authors declare no conflict of interest.
Acknowledgements
Authors express their appreciation to the Deanship of Scientific Research at King Khalid University, Saudi Arabia, for funding this work through a research group program under grant number RGP 2/538/45 to Saleh S Alarfaj.
References
- A. W. Boots, J. J. van Berkel, J. W. Dallinga, A. Smolinska, E. F. Wouters and F. J. van Schooten, The versatile use of exhaled volatile organic compounds in human health and disease, J. Breath Res., 2012, 6(2), 027108 CrossRef PubMed.
- Y. Y. Broza and H. Haick, Nanomaterial-based sensors for detection of disease by volatile organic compounds, Nanomedicine, 2013, 8(5), 785–806 CrossRef CAS PubMed.
- S. Sun, T. Hussain, W. Zhang and A. Karton, Blue phosphorene monolayers as potential nano sensors for volatile organic compounds under point defects, Appl. Surf. Sci., 2019, 486, 52–57 CrossRef CAS.
- B. de Lacy Costello, A. Amann, H. Al-Kateb, C. Flynn, W. Filipiak, T. Khalid, D. Osborne and N. M. Ratcliffe, A review of the volatiles from the healthy human body, J. Breath Res., 2014, 8(1), 014001 CrossRef CAS PubMed.
- X. Sun, K. Shao and T. Wang, Detection of volatile organic compounds (VOCs) from exhaled breath as noninvasive methods for cancer diagnosis, Anal. Bioanal. Chem., 2016, 408, 2759–2780 CrossRef CAS PubMed.
- T. Hibbard and A. J. Killard, Breath ammonia analysis: clinical application and measurement, Crit. Rev. Anal. Chem., 2011, 41(1), 21–35 CrossRef CAS.
- S. Das and M. Pal, Non-invasive monitoring of human health by exhaled breath analysis: a comprehensive review, J. Electrochem. Soc., 2020, 167(3), 037562 CrossRef CAS.
- Z. Cheng, B. Li, W. Yu, H. Wang, T. Zhang, J. Xiong and Z. Bu, Risk assessment of inhalation exposure to VOCs in dwellings in Chongqing, China, Toxicol. Res., 2018, 7(1), 59–72 CrossRef CAS PubMed.
- D. Zhang, Q. Mi, D. Wang and T. Li, MXene/Co3O4 composite based formaldehyde sensor driven by ZnO/MXene nanowire arrays piezoelectric nanogenerator, Sens. Actuators, B, 2021, 339, 129923 CrossRef CAS.
- X. Shao, D. Zhang, M. Tang, H. Zhang, Z. Wang, P. Jia and J. Zhai, Amorphous Ag catalytic layer-SnO2 sensitive layer-graphite carbon nitride electron supply layer synergy-enhanced hydrogen gas sensor, Chem. Eng. J., 2024, 153676 CrossRef CAS.
- H. Zhang, D. Zhang, R. Mao, L. Zhou, C. Yang, Y. Wu, Y. Liu and Y. Ji, MoS2-based charge trapping layer enabled triboelectric nanogenerator with assistance of CNN-GRU model for intelligent perception, Nano Energy, 2024, 127, 109753 CrossRef CAS.
- H. Zhang, D.-Z. Zhang, D.-Y. Wang, Z.-Y. Xu, Y. Yang and B. Zhang, Flexible single-electrode triboelectric nanogenerator with MWCNT/PDMS composite film for environmental energy harvesting and human motion monitoring, Rare Met., 2022, 41(9), 3117–3128 CrossRef CAS.
- S. Wang, J. Wang, Y. Zhu, J. Yang and F. Yang, A new device for liver cancer biomarker detection with high accuracy, Sens. Bio-Sens. Res, 2015, 4, 40–45 CrossRef.
- N. Peled, O. Barash, U. Tisch, R. Ionescu, Y. Y. Broza, M. Ilouze, J. Mattei, P. A. Bunn Jr, F. R. Hirsch and H. Haick, Volatile fingerprints of cancer specific genetic mutations, Nanomed. Nanotechnol. Biol. Med., 2013, 9(6), 758–766 CrossRef CAS PubMed.
- N. Nasiri and C. Clarke, Nanostructured chemiresistive gas sensors for medical applications, Sensors, 2019, 19(3), 462 CrossRef PubMed.
- R. P. Reji, S. K. C. Balaji, Y. Sivalingam, Y. Kawazoe and S. Velappa Jayaraman, First-principles density functional theory calculations on the potential of Sc2CO2 MXene nanosheets as a dual-mode sensor for detection of volatile organic compounds in exhaled human breath, ACS Appl. Nano Mater., 2023, 6(7), 5345–5356 CrossRef CAS.
- K. Timsina and S. Lepcha, MSc thesis, Sikkim Manipal Institute of Technology, 2022.
- J. Gusakova, X. Wang, L. L. Shiau, A. Krivosheeva, V. Shaposhnikov, V. Borisenko, V. Gusakov and B. K. Tay, Electronic properties of bulk and monolayer TMDs: theoretical study within DFT framework (GVJ-2e method), Phys. Status Solidi A, 2017, 214(12), 1700218 CrossRef.
- X. Huang, Z. Zeng and H. Zhang, Metal dichalcogenide nanosheets: preparation, properties and applications, Chem. Soc. Rev., 2013, 42(5), 1934–1946 RSC.
- L. Margulis, G. Salitra, R. Tenne and M. Talianker, Nested fullerene-like structures, Nature, 1993, 365(6442), 113–114 CrossRef CAS.
- R. Frindt and A. Yoffe, Physical properties of layer structures: optical properties and photoconductivity of thin crystals of molybdenum disulphide, Proc. R. Soc. Lond., Ser. A, Math. Phys. Sci., 1963, 273(1352), 69–83 Search PubMed.
- M. I. Khan, A. Saeed, M. Shakil, G. Saira, A. Ahmad, F. Imam and S. S. Alarfaji, Computational exploration of high-capacity hydrogen storage in alkali metal-decorated MgB2 material, J. Power Sources, 2024, 613, 234881 CrossRef.
- A. Kononov, M. Endres, G. Abulizi, K. Qu, J. Yan, D. G. Mandrus, K. Watanabe, T. Taniguchi and C. Schönenberger, Superconductivity in type-II Weyl-semimetal WTe2 induced by a normal metal contact, J. Appl. Phys., 2021, 129, 113903 CrossRef CAS.
- B. Amin, T. P. Kaloni and U. Schwingenschlögl, Strain engineering of WS2, WSe2, and WTe2, RSC Adv., 2014, 4(65), 34561–34565 RSC.
- Y. Liu, L. Gao, S. Fu, S. Cheng, N. Gao and H. Li, Highly efficient VOC gas sensors based on Li-doped diamane, Appl. Surf. Sci., 2023, 611, 155694 CrossRef CAS.
- V. Nagarajan and R. Chandiramouli, MoSe2 nanosheets for detection of methanol and ethanol vapors: a DFT study, J. Mol. Graphics Modell., 2018, 81, 97–105 CrossRef CAS PubMed.
- F. Li, F. Chen, H. Cui and X. Jiang, Pristine and Ni-doped WTe2 monolayer for adsorption and sensing of C2H2 and C2H4 in oil-immersed transformers: a DFT study, Comput. Theor. Chem., 2023, 1226, 114187 CrossRef CAS.
- Z. Xu, H. Cui and G. Zhang, Pd-decorated WTe2 monolayer as a favorable sensing material toward SF6 decomposed species: a DFT study, ACS Omega, 2023, 8(4), 4244–4250 CrossRef CAS PubMed.
- F. Huang, T.-Y. Sang, X. Hu, Z. Wang and W. Chen, Adsorption behaviors and electronic properties of Pd-doped MoTe2 monolayer for hazardous gases detecting and scavenging, Mater. Sci. Semicond. Process., 2024, 170, 107920 CrossRef CAS.
- L. Li, Z.-W. Tian, W.-H. Zhao, Q.-C. Zheng and R. Jia, Sniff lung cancer biomarkers in breath using N-doped monolayer WS2: a theoretical feasibility, Appl. Surf. Sci., 2023, 614, 156257 CrossRef CAS.
- G. t. Te Velde, F. M. Bickelhaupt, E. J. Baerends, C. Fonseca Guerra, S. J. van Gisbergen, J. G. Snijders and T. Ziegler, Chemistry with ADF, J. Comput. Chem., 2001, 22(9), 931–967 CrossRef CAS.
- M. Güell, J. M. Luis, M. Sola and M. Swart, Importance of the basis set for the spin-state energetics of iron complexes, J. Phys. Chem. A, 2008, 112(28), 6384–6391 CrossRef PubMed.
- E. Van Lenthe and E. J. Baerends, Optimized Slater-type basis sets for the elements 1–118, J. Comput. Chem., 2003, 24(9), 1142–1156 CrossRef CAS PubMed.
- J. P. Perdew, K. Burke and M. Ernzerhof, Generalized gradient approximation made simple, Phys. Rev. Lett., 1996, 77(18), 3865 CrossRef CAS PubMed.
- S. Grimme, Semiempirical GGA-type density functional constructed with a long-range dispersion correction, J. Comput. Chem., 2006, 27(15), 1787–1799 CrossRef CAS PubMed.
- M. Franchini, P. H. T. Philipsen and L. Visscher, The Becke fuzzy cells integration scheme in the Amsterdam Density Functional program suite, J. Comput. Chem., 2013, 34(21), 1819–1827 CrossRef CAS PubMed.
- R. Li, J. Zhang, S. Hou, Z. Qian, Z. Shen, X. Zhao and Z. Xue, A corrected NEGF + DFT approach for calculating electronic transport through molecular devices: filling bound states and patching the non-equilibrium integration, Chem. Phys., 2007, 336(2–3), 127–135 CrossRef CAS.
- S. Aghaei, A. Aasi, S. Farhangdoust and B. Panchapakesan, Graphene-like BC6N nanosheets are potential candidates for detection of volatile organic compounds (VOCs) in human breath: a DFT study, Appl. Surf. Sci., 2021, 536, 147756 CrossRef CAS.
- S. Peng, K. Cho, P. Qi and H. Dai, Ab initio study of CNT NO2 gas sensor, Chem. Phys. Lett., 2004, 387(4–6), 271–276 CrossRef CAS.
- F. Li, F. Chen, H. Cui and X. Jiang, Pristine and Ni-doped WTe2 monolayer for adsorption and sensing of C2H2 and C2H4 in oil-immersed transformers: a DFT study, Comput. Theor. Chem., 2023, 114187 CrossRef CAS.
- S. Ma, L. Su, L. Jin, J. Su and Y. Jin, A first-principles insight into Pd-doped MoSe2 monolayer: a toxic gas scavenger, Phys. Lett. A, 2019, 383(30), 125868 CrossRef CAS.
- T. Hussain, P. Panigrahi and R. Ahuja, Enriching physisorption of H2S and NH3 gases on a graphane sheet by doping with Li adatoms, Phys. Chem. Chem. Phys., 2014, 16(17), 8100–8105 RSC.
- Z. Xu, B. Luo, M. Chen, W. Xie, Y. Hu and X. Xiao, Enhanced photogalvanic effects in the two-dimensional WTe2 monolayer by vacancy-and substitution-doping, Appl. Surf. Sci., 2021, 548, 148751 CrossRef CAS.
- J. B. Barot, S. K. Gupta and P. N. Gajjar, Optical properties of WTe2-a layered topological insulator: a DFT study, Mater. Today: Proc., 2023 DOI:10.1016/j.matpr.2023.01.191.
- P. Cai, J. Hu, L. He, J. Pan, X. Hong, Z. Zhang, J. Zhang, J. Wei, Z. Mao and S. Li, Drastic pressure effect on the extremely large magnetoresistance in WTe2: quantum oscillation study, Phys. Rev. Lett., 2015, 115(5), 057202 CrossRef CAS PubMed.
- N. Shehzad, I. Shahid, S. Yao, S. Ahmad, A. Ali, L. Zhang and Z. Zhou, A first-principles study of electronic structure and photocatalytic performance of two-dimensional van der Waals MTe2–As (M= Mo, W) heterostructures, Int. J. Hydrogen Energy, 2020, 45(51), 27089–27097 CrossRef CAS.
- X.-Q. Tian, L. Liu, X.-R. Wang, Y.-D. Wei, J. Gu, Y. Du and B. I. Yakobson, Engineering of the interactions of volatile organic compounds with MoS2, J. Mater. Chem. C, 2017, 5(6), 1463–1470 RSC.
- L. Wang, Z. Lin, Y. Du, H. Guo, K. Zheng, J. Yu, X. Chen and L. Lang, Properties-enhanced gas sensor based on Cu-doped tellurene monolayer to detect acetone molecule: a first-principles study, Mol. Phys., 2021, 119(7), e1864490 CrossRef.
- P. Ou, P. Song, X. Liu and J. Song, Superior sensing properties of black phosphorus as gas sensors: a case study on the volatile organic compounds, Adv. Theory Simul., 2019, 2(1), 1800103 CrossRef.
- C.-H. Yeh, Computational study of Janus transition metal dichalcogenide monolayers for acetone gas sensing, ACS Omega, 2020, 5(48), 31398–31406 CrossRef CAS PubMed.
- U. Nosheen, A. Jalil, S. Z. Ilyas, A. Illahi, S. A. Khan and A. Hassan, First-Principles Insight into a B4C3 Monolayer as a Promising Biosensor for Exhaled Breath Analysis, J. Electron. Mater., 2022, 51(11), 6568–6578 CrossRef CAS PubMed.
- S. S. Dindorkar, N. Sinha and A. Yadav, Comparative study on adsorption of volatile organic compounds on graphene, boron nitride and boron carbon nitride nanosheets, Solid State Commun., 2023, 359, 115021 CrossRef CAS.
- Y. Yan, Y. Luo, Y. Li, Y. Zhang, P. Wu, J. Tang, X. Zhang and S. Xiao, Transition metal (Au, Ag, Pt, Pd, Ni) doped MoS2 as gas sensing materials for C4F7N leakage detection: a comparative study, Surf. Interfaces, 2024, 44, 103625 CrossRef CAS.
- Y. Yang, K. Hu, J. Zhang, T. He, Y. Jiang, Y. Zhang and H. Liu, Adsorption properties of noble-metal (Ag, Rh, or Au)-doped CeO2 (1 1 0) to CO: a DFT + U study, Comput. Mater. Sci., 2024, 231, 112543 CrossRef CAS.
- H. Cui, X. Zhang, G. Zhang and J. Tang, Pd-doped MoS2 monolayer: a promising candidate for DGA in transformer oil based on DFT method, Appl. Surf. Sci., 2019, 470, 1035–1042 CrossRef CAS.
- Q. Wan, X. Chen and Y. Gui, First-principles insight into a Ru-doped SnS2 monolayer as a promising biosensor for exhale gas analysis, ACS Omega, 2020, 5(15), 8919–8926 CrossRef CAS PubMed.
- H. Cui, P. Jia and X. Peng, Adsorption of SO2 and NO2 molecule on intrinsic and Pd-doped HfSe2 monolayer: a first-principles study, Appl. Surf. Sci., 2020, 513, 145863 CrossRef CAS.
- A. Aasi, S. M. Aghaei and B. Panchapakesan, Pt-decorated phosphorene as a propitious room temperature VOC gas sensor for sensitive and selective detection of alcohols, J. Mater. Chem. C, 2021, 9(29), 9242–9250 RSC.
- X. Tang, A. Du and L. Kou, Gas sensing and capturing based on two-dimensional layered materials: overview from theoretical perspective, Wiley Interdiscip. Rev.: Comput. Mol. Sci., 2018, 8(4), e1361 Search PubMed.
- T. Liu, Z. Cui, X. Li, H. Cui and Y. Liu, Al-doped MoSe2 monolayer as a promising biosensor for exhaled breath analysis: a DFT study, ACS Omega, 2020, 6(1), 988–995 CrossRef PubMed.
- T. Hussain, D. Singh, S. K. Gupta, A. Karton, Y. Sonvane and R. Ahuja, Efficient and selective sensing of nitrogen-containing gases by Si2BN nanosheets under pristine and pre-oxidized conditions, Appl. Surf. Sci., 2019, 469, 775–780 CrossRef CAS.
- D. Singh, P. K. Panda, Y. K. Mishra and R. Ahuja, van der Waals induced molecular recognition of canonical DNA nucleobases on a 2D GaS monolayer, Phys. Chem. Chem. Phys., 2020, 22(12), 6706–6715 RSC.
- M.-L. Bocquet, A. Rappe and H.-L. Dai*, A density functional theory study of adsorbate-induced work function change and binding energy: olefins on Ag (111), Mol. Phys., 2005, 103(6–8), 883–890 CrossRef CAS.
- M. Gussoni, M. Rui and G. Zerbi, Electronic and relaxation contribution to linear molecular polarizability. An analysis of the experimental values, J. Mol. Struct., 1998, 447(3), 163–215 CrossRef CAS.
- Z. Jiang, Q. Pan, M. Li, T. Yan and T. Fang, Density functional theory study on direct catalytic decomposition of ammonia on Pd (1 1 1) surface, Appl. Surf. Sci., 2014, 292, 494–499 CrossRef CAS.
- F. Li, X. Gao, R. Wang, T. Zhang and G. Lu, Study on TiO2-SnO2 core-shell heterostructure nanofibers with different work function and its application in gas sensor, Sens. Actuators, B, 2017, 248, 812–819 CrossRef CAS.
- D. Singh and R. Ahuja, Highly sensitive gas sensing material for environmentally toxic gases based on janus NbSeTe monolayer, Nanomaterials, 2020, 10(12), 2554 CrossRef CAS PubMed.
- D. Chidambaram, R. Kalidoss, K. Pushparaj, V. J. Surya and Y. Sivalingam, Post-deposition annealing influences of gas adsorption on semi-vertical β-FeOOH nanorods at
room temperature: a scanning kelvin probe analysis, Mater. Sci. Eng., B, 2022, 280, 115694 CrossRef CAS.
- Y. Sivalingam, P. Elumalai, S. V. Yuvaraj, G. Magna, V. J. Sowmya, R. Paolesse, K.-W. Chi, Y. Kawazoe and C. Di Natale, Interaction of VOCs with pyrene tetratopic ligands layered on ZnO nanorods under visible light, J. Photochem. Photobiol., A, 2016, 324, 62–69 CrossRef CAS.
- R. P. Reji, G. Marappan, Y. Sivalingam and V. J. Surya, VOCs adsorption induced surface potential changes on phthalocyanines: a combined experimental and theoretical approach towards food freshness monitoring, Mater. Lett., 2022, 306, 130945 CrossRef CAS.
|
This journal is © The Royal Society of Chemistry 2024 |