DOI:
10.1039/D4RA02572E
(Paper)
RSC Adv., 2024,
14, 24516-24533
An industrially potent rhamnolipid-like biosurfactant produced from a novel oil-degrading bacterium, Bacillus velezensis S2†
Received
5th April 2024
, Accepted 28th June 2024
First published on 5th August 2024
Abstract
Surfactants can reduce the interfacial surface tension between two immiscible liquids making them a desirable component for various industrial applications. However, the toxic nature of chemical surfactants brought immense attention towards biosurfactants. Being biodegradable, biosurfactants are eco-friendly and considered safer for different commercial uses. This study focused on the production of biosurfactant from an oil-degrading bacteria and its functional efficacy for prospective industrial applications. Here, a promising oil-tolerant strain, Bacillus velezensis S2 was isolated from oil contaminated sites which showed >50% degradation of convoluted crude oil within 28 days in comparison to a control. The isolate was then found to produce an excellent surface-active compound with an emulsification index of 67.30 ± 0.8% and could reduce the surface tension up to 36.86 ± 0.36 mN m−1. It also manifested a critical micelle concentration of 45 mg L−1 while reducing the surface tension from 72 to 30 mN m−1. When extracting biosurfactant from isolated bacteria, ethyl acetate extraction showed 1.5 times greater efficacy than chloroform
:
methanol extraction. The purified biosurfactant was characterized using TLC, 1H NMR, 13C NMR, FTIR, elemental analyses and spectrophotometric techniques leading to its identification as a rhamnolipid. The stability of produced biosurfactant at higher temperature (up to 180 °C) was determined by thermal analysis, endorsing its application in high temperature reservoir conditions. Additionally, the extracted biosurfactant showed excellent foaming efficacy with insignificant antibacterial and cytotoxic responses, which indicates their potential application in cleaning and cosmetics industries. Thus, the present study outlines a bi-functional novel isolate Bacillus velezensis S2 which could play a significant role in oil remediation from the environment as well as serve as a potential source of non-toxic and eco-friendly biosurfactants for various industrial applications.
1. Introduction
Environmental pollution is a burning problem confronting the current world which occurs due to the high accumulation of hydrocarbons, chemicals, solvents, heavy metals etc. These pollutants have serious harmful effects on living organisms and also can hamper the economic growth in developing countries especially by polluting the aquatic environment.1 Crude oil being one of these hazardous pollutants, shows toxic and hydrophobic properties that hamper the diversified growth of the ecosystem.2 Moreover, crude oil spills in the environment undergo physico-chemical changes of properties resulting in the exposure of toxic polycyclic aromatic hydrocarbons (PAHs).3 Some lipophilic PAHs tend to spread through the food web and get bioaccumulated3–5 affecting the biodiversity of nature.2 A report in 2019 (ref. 6) stated that leakage of around 10
000 liters of heavy fuel oil into the Karnaphuli River of Bangladesh could spread over 16 kilometers and posed a threat to diversified aquatic lives including dolphins around the exposure site. However, chemical remediation of such crude oil contaminants from the surfaces is impeded by high cost and secondary pollution problems.7 Therefore, the progress in sustainable innovations has directed the quest for biological approaches to remediate crude oil from contaminated sites. In this regard, several microbial species such as Pseudomonas, Bacillus and Streptomyces have been reported for their capability to grow in oil contaminated stressful areas.8–11 These microbes have been reported to degrade heavy hydrocarbon moieties present in oil by producing active surfactants.8,12 The referred to biologically produced surfactants are known as biosurfactants which has led to the theory of eco-friendly surfactant production from natural sources.
Biosurfactants are amphipathic compounds having both hydrophilic and hydrophobic ends produced by living organisms.13 Due to such structural property, it can interact at the interface between aqueous and non-aqueous systems showing active surface activity. Glycolipids, lipopeptides or lipoproteins, neutral lipids, phospholipids, substituted fatty acids and lipopolysaccharides etc. are different forms of biosurfactant.14,15 The increased popularity held in the concept of biosurfactants is because of its numerous advantages over chemical surfactants. For instance, their low-toxicity, eco-friendly nature, higher biodegradability, natural stability at extreme conditions like pH, salinity and high or low temperature make them more valuable than the synthetic counterparts.16,17 As a consequence, these biomolecules have promising applications in multi-functional purposes such as oil recovery, cleaning, food processing, soil remediation, textiles industry, cosmetics, personal care industry and so on.18,19 Additionally, some biosurfactants conjugated with nano-particles (like Ag, Cu) or specialized amino acid have shown potential antibacterial activity against pathogens, proving their worth in this antibiotic retrospect era.20,21 Therefore, the microbes that can remove long-chained hydrocarbon containing waste oil from the environment and produce value added surfactants like biomolecules parallelly, are of great interest for sustainable ecosystem.
It is well known that oil-contaminated sites contain high volume of organic pollutants and theoretically the microbial diversity present there must degrade the organic contaminants to get energy for living. However, biosurfactant production has been proved as a major strategy of the microbes to break down the organic pollutants.22,23 The current study reports about such an efficient oil-degrading bacterial strain isolated from crude oil contaminated site which is also a source of potential biosurfactant compounds having prospective industrial uses.
2. Materials & methods
2.1 Materials
All media, chemicals, reagents and solvents used in the current study were of analytical grade; including: isooctane (C8H18, ≥ 99%, Sigma-Aldrich, USA), agar base (Himedia, India), nutrient agar (NA) (Scharlau, Spain), nutrient broth (Merck, USA), n-hexane (C6H8, ≥95%, Sigma-Aldrich, USA), acetone (C3H6O, ≥99%, Sigma-Aldrich, USA), chloroform (CHCl3, ≥99%, Sigma-Aldrich, USA), methanol (CH3OH, ≥99.8%, Sigma-Aldrich, USA), sulfuric acid (H2SO4, 95–97%, Scharlau, Spain), ethanol (C2H5OH, 99.8%, Sigma-Aldrich, USA), Mueller–Hinton agar (Himedia, India), agarose gel (Genedire, USA), dimethyl sulfoxide (DMSO, 99.99%, Sigma-Aldrich, USA), sodium chloride (NaCl, 99.9%, Sigma-Aldrich, USA), potassium chloride (KCl, Scharlau, Spain), potassium biphosphate (KH2PO4, Scharlau, Spain), disodium phosphate (Na2HPO4, Scharlau, Spain), dipotassium phosphate (K2HPO4, Scharlau, Spain), magnesium sulfate heptahydrate (MgSO4·7H2O, Sigma-Aldrich, USA), calcium chloride (CaCl2, ≥97%, Sigma-Aldrich, USA), NaOH (Sigma-Aldrich, USA), tween-20 (Scharlau, Spain), tween-80 (Pure Pharma, GmbH, Germany), and sodium dodecyl sulfate (SDS, Pure Pharma, GmbH, Germany).
2.2 Sample collection and screening of crude oil degrading bacteria
Three types of samples (40 years old oil contaminated soil, 15 years old oil contaminated soil and waste oil) were collected from MEGHNA Fatullah Depot, Narayanganj (23′33′–23′57′N and 90′26′–90′45′ E), Bangladesh. The samples were serially diluted (up to 10−7) in phosphate-buffered saline (137 mmol per L NaCl, 10 mmol per L H3PO4, and 2.70 mmol per L KCl, pH 7.4). Then 0.1 mL from 10−3, 10−4 and 10−5 dilutions were spread on to Nutrient Agar (NA) and the plates were incubated at 37 °C for 24–48 h. After that, a single distinct colony was surface streaked on the same culture media under the previous conditions, to obtain isolated colonies.
Bacterial tolerance to petroleum crude oil was examined by incubating the isolated colonies in minimal salt medium (MSM) broth constituting of 2.94 mM KH2PO4, 7.01 mM K2HPO4, 0.81 mM MgSO4·7H2O, 0.18 mM CaCl2, and 1.71 mM NaCl24 and supplemented with 1% crude oil for 7 days, at 180 rpm and 30 °C. Each distinct oil tolerant cultures was further investigated for their oil degradability and survivability by determining their growth on MSM supplemented with crude oil at different concentrations (2%, 5%, 10%, 20%, 40%, 50%, 75%, and 100%; v/v).25 1 mL of culture solution (OD600 = 0.32) was transferred to each treatment. After incubating them under the same condition, the viable bacterial count was determined on nutrient agar media through spread plate technique on the following day.
2.3 Isolate characterization and identification
2.3.1 Phenotypic and biochemical characterization. As a potential oil degrader, the target isolate (ODB-2) was initially characterized based on colony morphology on NA media and microscopic appearance (Zeiss Primo Star Microscope, Germany). The biochemical characterization was performed according to the procedure described in bergey's manual of determinative bacteriology.26
2.3.2 Phylogenetic analysis by 16S rRNA gene sequences. Total genomic DNA was extracted from selected isolates to identify based on 16S rRNA gene sequence analysis using boil template method27 (ESI File†).
2.4 Degradation of crude oil by the most potent isolate
The potential oil degrading isolate was then tested for its oil degrading capability employing gravimetric analysis, spectrophotometric analysis, and gas chromatography (GC) analysis. For this, the isolate was inoculated in 100 mL MSM contained with 10% of (v/v) (∼81 g) crude oil as sole carbon source, followed by the incubation at 30 °C and 120 rpm for 28 days. Un-inoculated flask having the identical system was prepared as controls, so that the abiotic losses of crude oil, particularly for evaporation could be determined by the un-inoculated control. The records for each treatment were taken in triplicates and calculated in reference to un-inoculated controls.
2.4.1 Gravimetric analysis of crude oil biodegradation. The quantitative degradation of crude oil was initially determined by gravimetric method28 (described in ESI File†).
2.4.2 Spectrophotometric method. The quantitative analysis of oil biodegradation by UV spectrophotometry was determined according to the standard protocol described by Liu et al.29 with few modifications (described in ESI File†).
2.4.3 Oil degradation analysis by gas chromatography (GC). Residual crude oil samples extracted after 7 days of incubation was analyzed by GC to confirm the degradation efficacy of the strain in reference to control condition30 (described in ESI File†).
2.5 Determination of biosurfactant producing ability
The qualitative studies that are frequently used as quick and simple experiments to check the presence of any surface-active compound in a solution were performed in this study which includes oil spreading test, drops collapse assay, BATH test, emulsification index, and most importantly, surface tension measurement.31,32
2.5.1 Oil spreading assay (OSA). OSA test was performed according to the method described by ref. 32 to determine the presence of biosurfactant in culture solution (described in ESI File†).
2.5.2 Drop collapse test (DCT). DCT is a qualitative test for screening of biosurfactant production and this test was performed according to Bodour and Miller-Maier31 (described in ESI File†).
2.5.3 Emulsification index (E24). The emulsification index (E24) test was performed to evaluate the emulsifying ability of culture supernatant as reported by ref. 33 (described in ESI File†).
2.5.4 Bacterial adhesion to hydrocarbon (BATH) assay. The relative hydrophobicity of bacterial cells was measured by a BATH assay described by ref. 34 (described in ESI File†).
2.5.5 Surface tension (ST) determination. The ST of the biosurfactant-containing CFS was determined according to the Wilhelmy plate method35 using Ossila Contact Angle Goniometer (L2004A1, Netherland) equipped with a high-resolution video-camera system and dedicated computer software (described in ESI File†).
2.6 Biosurfactant extraction
Crude biosurfactant was extracted following the acid precipitation and liquid–liquid solvent extraction method explained by ref. 36 (described in ESI File†).
2.6.1 Dry weight measurement of biosurfactants. Two sterile Petri plates were weighed and the unpurified biosurfactant produce from chloroform
:
methanol liquid–liquid extraction and ethyl acetate mediated extraction were poured onto the plates separately. After drying them for 2 hours at 80 °C on the hot air oven, the plates were weighed again.37 The dry weight of the biosurfactants was calculated as follows:
Dry weight of biosurfactants = (weight of the dried biosurfactant containing plate − weight of the empty plate) |
2.6.2 Microscopic analysis. To examine the microstructure of the extracted biosurfactant, the product was gently stirred to ensure a homogenous sample. A loop-full biosurfactant was spread on a glass slide of 15 × 20 mm2, previously cleaned with 70% ethanol and dried properly. A 0.17 mm thick coverslip was placed on top to the spread sample for ensuring zero air gaps between the sample and the coverslip. An optic microscopy was carried out using a four-objective binocular microscope (Zeiss Primo Star Microscope, Germany). The sample was observed under 10×, 40× and 100× lens at room temperature (24 °C).
2.7 Purification of biosurfactant and thin layer chromatography
To analyze the compound structure, crude biosurfactant was purified using the modified method of ref. 38 and 39 where the precipitate or biosurfactant was allowed to run through open column chromatography using a laboratory burette (ESI File†).
2.8 Structural characterization of obtained biosurfactant product
2.8.1 Nuclear magnetic resonance (NMR) analysis. Isolated pure compounds were studied under nuclear magnetic resonance (NMR) spectrometer (Bruker 400 NMR spectrometer) for further structural elucidation with a unity of 400 MHz. During the measurement of 1H spectra, the instrumental conditions were recorded as; frequency (399.73 MHz), spectral width (6410.3 Hz), acquisition time (2.556 s), relaxation delay (1 s), pulse angle flip (90°) and number of transients.40 The instrumental conditions for carbon spectra were: frequency (100.51 MHz), spectral width (25
510.2 Hz), acquisition time (1.285 s), relaxation delay (7 s), pulse angle flip (90°) and number of transients (1000). Here, deuterated chloroform, CDCl3 was used to dissolve the compounds. The structures of the compounds were elucidated by spectroscopic analysis as well as by comparing with previously published NMR data. The chemical shifts are expressed in ppm.
2.8.2 Fourier transform infrared spectroscopy (FTIR) analysis. Fourier-transform infrared (FTIR) spectra of the purified biosurfactant were recorded to identify the functional groups and chemical nature of the product. For this, the sample was dried and converted in powdered form. The powdered sample was mixed with KBr and FT-IR spectrum was recorded over the range 450–4000 cm−1 using FTIR Spectrometer (Shimadzu, FTIR-8900/8400S Japan). Attenuated Total Reflection (ATR)-FTIR spectra (Model-IR Prestige 21, Shimadzu, JAPAN) of the sample were also taken with the diamond crystal to get complete information of all functional groups of the sample. The spectrum was recorded in the range of 4000 cm−1 to 650 cm−1 and the entire scans were 30 in number, with a spectral resolution of 4 cm−1.41 Before analysis of each sample, the instrument was standardized, and the ATR diamond crystal was cleaned using 2-propanol (70%).
2.8.3 Rhamnose detection. The UV-spectrophotometer (UV-2600, SHIMADZU, Japan) was used to determine the presence of carbohydrate groups in the biosurfactant molecule by rhamnose test using the method of ref. 42 (ESI File†).
2.8.4 Elemental analysis. The elemental contents (C, H, O, N, and S) of the biosurfactant samples (before and after purification) were measured by an elemental analyzer (CHNS analyzer vario MICRO cube Elementar, Germany).
2.8.5 UV. The ultraviolet-visible (UV-vis) absorption spectra of purified biosurfactant sample were taken using an UV-vis-NIR (near-infrared) spectrophotometer (Shimadzu UV 2600 ISR Plus) between 200 and 800 nm wavelength region to study the optical response of sample.
2.8.6 X-ray diffraction (XRD) analysis. Extracted biosurfactant was studied with X-ray diffraction (XRD) experiments (SmartLab SE, Rigaku, Japan), with the aim to describe their structural molecular organization at few Angstrom resolutions. The data had been recorded as a function of temperature by fixing scanning range, 2θ = 5° to 90° and step width of 0.01°, Here, a copper tube made of ceramic (CuKα, λ = 1.54060 Å) was chosen as the X-ray source with a step scan of 15° min−1 and the voltage and current were fixed at 40 kV and 50 mA, respectively.
2.8.7 Thermal properties analyses. The thermal properties of the extracted biosurfactant were performed by a simultaneous thermal analyzer (Model: STA449F3, NETZSCH, Weimar, Germany) (described in ESI File†).
2.8.8 Determination of foaming property. The purified biosurfactant was tested for its foaming abilities following the method described by ref. 43 (ESI File†).
2.8.9 Determination of critical micelle concentration (CMC). In this study, the CMC of purified biosurfactant was determined by measuring its surface tensions for a series of concentrations (0–100 mg L−1).44 In general, the ST of two immiscible surfaces will drop down with the increased addition of surfactant up to a certain concentration. However, once the saturation point is reached, the ST would become independent of surfactant amount and will remain unchanged. The surfactant concentration of this saturation point is known as CMC beyond which the molecules get aggregated and start to form micelles. Once the micelles formation is achieved, topping up the surfactant amount in the interface would be worthless.36
2.8.10 Antibacterial test. To determine if the produced biosurfactant had any antibacterial property, Staphylococcus aureus (ATCC 14458) and Escherichia coli O157:H17 (ATCC 43895) were chosen as Gram-positive and Gram-negative representative. For this, agar well diffusion assay was performed according to the technique recommended by ref. 45 and 46 (ESI File†).
2.8.11 Cell cytotoxicity. The cytotoxic response of purified biosurfactant was investigated using the Trypan Blue Exclusion method47 (described in ESI File†).
2.9 Statistical analysis of experiments
Around all the experiments, particularly the data for bacterial growth, biochemical parameters, and functional screening like oil displacement, emulsification, surface tension, foam test was performed in three replicates. The statistical package SPSS (version 26.00, Chicago, IL, USA) was used for data processing with a two-way ANOVA analysis of variance. Duncan's multiple range examination was applied at a 5% probability level to determine significant disparities in the mean values.
3. Results and discussion
3.1 Isolation and screening for oil degrading bacteria
Due to the intriguing and eco-friendly properties of biosurfactants, an emerging interest and active research are extending the concept to incorporate such compounds in potential industrial applications.48–51 Moreover, biosurfactants derived from oil degrading bacteria are gaining strong status for both restoring environmental conditions as well as prospective commercial uses.52–55 This study made use of potential oil degrading bacteria (ODB), isolated from oil contaminated soil samples collected from oil contaminated soil (15 years and 40 years old) and waste oil samples collected from MEGHNA Fatullah Depot, Narayanganj (23′33′–23′57′N and 90′26′–90′45′E), Bangladesh (Fig. 1A) for their application in petroleum remediation capability and production of industrially valuable biosurfactant. Initially, a total of 16 distinct bacterial colonies were isolated among which, 7 isolates could grow in presence of 1% crude oil containing MSM media. The isolates were labelled as oil degrading bacteria (ODB) 1 to 7. To harness the possible oil degradation ability of the 7 isolates, they were tested for scoring their oil tolerance capability, most of them were found to be tolerant to oil concentrated from 2% to 40% while only one isolate could tolerate up to 90% of crude oil contained in batch culture system (Fig. 1B). This isolate (ODB-2) was selected as a potential oil degrader for further study.
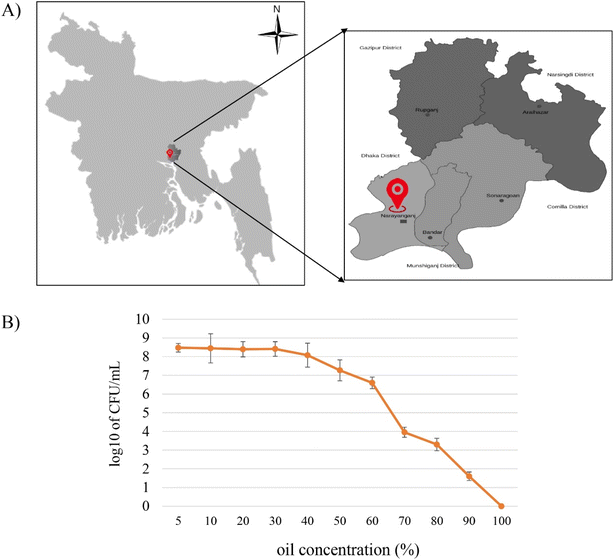 |
| Fig. 1 A representative illustration of Bangladesh map with a magnified view of sampling site (A); a line graph showing the oil tolerance capability of isolate ODB-2 at different crude oil concentration based on its viability upon 24 hours of incubation (B). The experiment was done in triplicate where the bars represent standard deviation (±SD). | |
3.1.1 Identification of potential oil degrader. Morphological, microscopic, and biochemical characteristics of the isolate ODB-2 showed its resemblance with Bacillus forming an irregular, moderate sized, white, skin-like pellicles with mucoid texture and a ridged surface when grown in NA (Fig. 2A). When cultured at 37 °C for 24 h in NA medium, the culture was found as Gram-positive, rod shaped, spore containing strain under microscope. The biochemical analysis also revealed the isolate as Bacillus strain with positive catalase test, Methyl red and Voges Proskauer (MR–VP) test, different sugar test, oxidase test, and negative hydrogen sulfide and nitrate reduction test (Fig. 2B). The isolate was then identified as Bacillus velezensis S2 based on 16 s rRNA partial gene sequence that showed 99.86% similarity to B. velezensis strains CBMB205 (GenBank accession number is NR_116240.1).
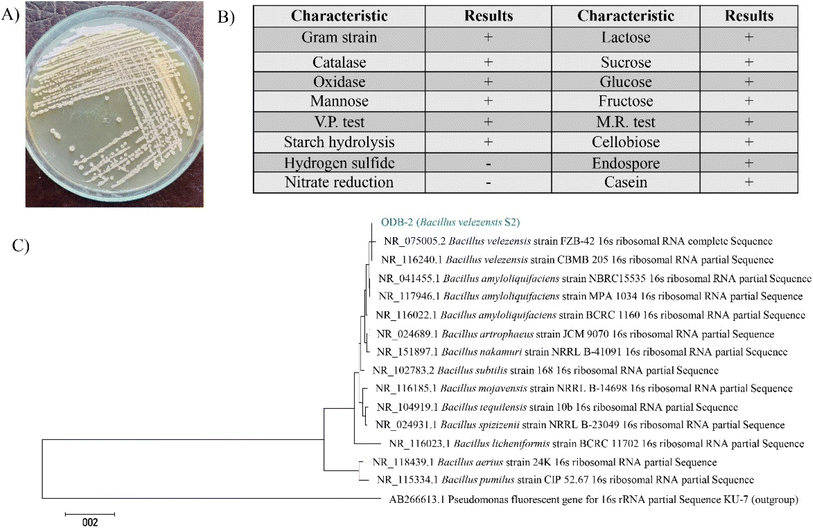 |
| Fig. 2 Characterization and identification of potential oil biodegrader, ODB-2 as Bacillus velezensis S2. Colony morphology on NA plate (A), biochemical characteristics, where “+” indicates positive response; “−” indicates negative response (B), phylogenetic tree of 16s rRNA of ODB-2 showing its relation to the available sequence on the NCBI database (C). | |
The 16S rRNA gene partial sequence of strain S2 was submitted to the NCBI GenBank database under accession no. gb |OR337901|. The phylogenetic tree of the 16S rDNA of S2 strain and its relation to the available sequences on the NCBI GenBank database is illustrated in Fig. 2C.
Interestingly, different B. velezensis isolates were reported to contain the genes responsible for the degradation of lignocellulosic, cellulosic and hemicellulosic materials.56 In other studies, B. velezensis strains were also investigated for their ability to utilize various types of agro-industrial byproducts in biofuel production.57 Some experiments also reported it as a probiotic, bile tolerant, riboflavin producer and exopolysaccharide (EPS) synthesizer58 while other reported this spp as lipid producer.59 Meena et al., on the other hand, found a Bacillus velezensis strain showing both surfactant producing and crude oil degrading ability.60 Overall, this spp appears to be a great interest of study while thinking of industrially valuable biocompounds.
3.2 Crude oil biodegradation
When the potential isolate S2 was tested for its oil degradation efficiency, the gravimetric analysis showed more than 50% degradation of crude oil in reference to control (un-inoculated) following 28 days of incubation period (Fig. 3A). Similar pattern of oil degradation rates was proved by the spectrophotometric experimental method, where the degradation rates were found as 19.13%, 33.09%, 40.47% and 51.87% at the incubation intervals of 7, 14, 21 and 28 days respectively (Fig. 3A).
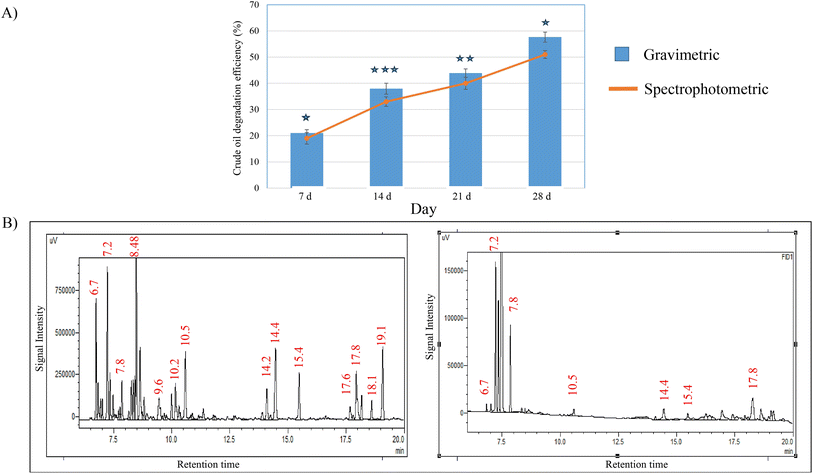 |
| Fig. 3 An outline describing the oil biodegradation ability of B. velezensis S2. The bar diagram depicts the quantitative analysis of crude oil degradation by gravimetric method while the line graph demonstrates the same by spectrophotometric assay (A). The GC chromatogram (left side) represents the alkane fractions present in the untreated crude oil (control), while the right-side image clearly shows the reduced alkane fraction of crude oil treated with S2 after 1 week of incubation (B). The statistical analysis for the gravimetric and spectrophotometric analysis was performed using SPSS statistical package (version22) for the one-way ANOVA, where the values were found as significant shown by asterisk (*, p < 0.05; **, p < 0.01; and ***, p < 0.001). | |
The GC-ECD chromatogram of oil biodegradation was recorded after 1 week of the culture incubation (treated) and was compared to that of the abiotic control sample (untreated crude oil containing MSM) (Fig. 3B). The signal intensities for various crude oil fractions in S2 treated culture were disappeared or significantly reduced by more than 5 folds indicating the efficiency of this strain in biodegradation and/or biotransformation of crude oil. This experiment was repeated for 3 times which demonstrated almost similar reduction of crude oil fraction.
An alike study showed that a consortium of six Gram-negative oil-tolerant bacteria could degrade about 42% of crude oil in 36 days which continued to the reduction of 83.70% of the oil in 75 days.28 Another study signified the efficiency of oil-tolerant bacterial consortium where it observed 50.63–55.43% of crude oil removal in 7 days supporting the use of consortium in facilitating remediation of oil-contaminated sites.61 Therefore, alone or with other, the isolate from this study may prove to be significant in biodegradation of crude oil.
3.3 Determination of biosurfactant producing ability
While considering different experiments to determine the producing capability of biosurfactant by the isolate, we chose the most common, rapid, and sensitive methods to be carried out. Among them, the OSA is considered as a simple and rapid biosurfactant detection method requiring a small volume of sample.37 In our OSA experiment, 1 drop of the culture CFS could produce 3.93 (±0.15) mm of clear zone (in diameter) by reducing the interfacial surface tension between water and crude oil. Similarly, the findings of Nayarisseri et al.62 reported two biosurfactant producers, where Staphylococcus and Bacillus showed positive response with a clearance zone of 1.8 mm and 2.5 mm respectively. As several studies proved that oil spreading area in this technique is directly related to the surface-active agent present in the solution,63 this finding proves the production of biosurfactant by S2 isolate.
Another sensitive test, the drop collapse assay endorsed the findings of OSA producing a flat/collapsed drop 58 s upon the addition of bacterial CFS on the top of equilibrated crude oil surface in microtiter plate. This occurred due to the surface tension reduction in the interface of two immiscible liquids.64 The record showed similarities with a separate study where biosurfactant producing Bacillus spp could collapse the drop within 58 s whereas Staphylococcus spp could do the same within 1 min 56 s.62
The emulsification index assay is an effective way to determine the presence of biosurfactant. It works on a concept that a culture grown in a media containing hydrophobic natured hydrocarbons (such as crude oil) would emulsify the present hydrocarbons40 if the culture can produce any surfactant like compound. In this study, the CFS from S2 culture showed an emulsification index of 67.30 ± 0.8%, whereas it is reported that E24 greater than 30% can be indicated as higher activity of surfactant.62 Accordingly, when Pseudomonas aeruginosa showed an emulsification activity of (55.56–60)% in a study,40 it was reported as a biosurfactant producer. A likely report also exhibited that P. guguanensis strain extracted product showed an E24% of 52 ± 0.33% (ref. 65) and was proved to be a biosurfactant producer.
Another technique to screen out biosurfactant producing bacteria is BATH test which can determine surfactant producer by identifying their cell hydrophobicity in present of crude oil.62 Here, cell hydrophobicity can be indicated by the adherence or affinity of bacterial cells to the crude oil portion in the batch culture system. In this experiment, the cell adherence for S2 strain was found as (92.44 ± 0.65)% which can be regarded as a positive biosurfactant producer following a similar study conducted by.62
The reduction in surface tension is another strong indication of the presence of biosurfactant in a solution.66 In this experiment, the surface tension measurement of CFS showed a gradual reduction in surface tension with the increasing cell number. As shown in Fig. 4B, B. velezensis S2 decreased the surface tension by (36.86 ± 0.36) mN m−1 having an OD600 value of 0.98 comparing to the control indicating the existence of surfactant like compound in the culture. Similarly, other reports on P. aeruginosa IITR48,67 Staphylococcus and Bacillus spp;62 which were found as biosurfactant producer, could reduce the surface tension by 29 N m−1, 42 mN m−1 and 38 mN m−1 respectively. Reports claim that an efficient biosurfactant can reduce the surface tension of pure water from 72 mN m−1 to 30 mN m−1.54 For instance, 10 μM surfactin could reduce the surface tension of water (72 mN m−1)to 27 mN m−1 (ref. 68) while rhamnolipids, sophorolipids, and trehalose were reported to reduce the surface tension to <30–33 mN m−1.69–71
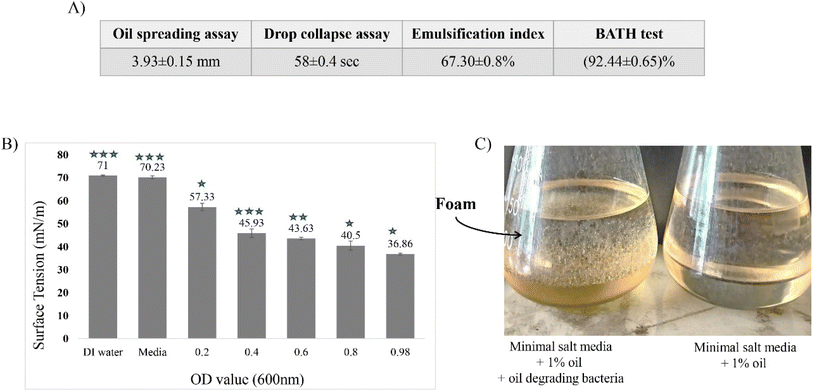 |
| Fig. 4 A diagram exhibiting the experiment outcomes of biosurfactant production by S2 strain. Here, the table shows positive indications of biosurfactant production in terms of OSA, drop collapse assay, emulsification index and BATH test (A); a gradual reduction of surface tension in S2 treated media with the increasing cell density compared to untreated conditions (DI water and only media) (B); a picture showing the crude oil containing media appearances in terms of treated (with S2) and untreated (without S2) conditions (C). The statistical analysis was performed using SPSS statistical package (version22) for the one-way ANOVA, where the values were found as significant shown by asterisk (*, p < 0.05; **, p < 0.01; and ***, p < 0.001). | |
As we have used MSM media for the batch culture system, no surface-active compound could be present in the CFS unless they were produced by the culture. In this study, a direct correlation is visible in OSA, drop collapse, emulsification index, BATH test and surface tension assays. Similar correlation was reported by Bodour and Miller-Maier31 between drop collapse method and surface tension while they were determining the production of biosurfactant.
3.4 Crude biosurfactant extraction
In this study, ethyl acetate was found as a good recovery solvent for biosurfactant extraction compared to chloroform
:
methanol (2
:
1) giving the yield quantity of 3.12 ± 0.11 g L−1 and 2.15 ± 0.09 g L−1 respectively. Hence, the dry weight of biosurfactant produced from ethyl acetate extraction weighed almost 1.5 times higher compared to the chloroform
:
methanol extraction. The impurified biosurfactant was brown in color with thick consistency. These results are in concordance with those found in previous works for biosurfactant reported by Patowary and his colleagues where ethyl acetate extracted biosurfactant from P. aeruginosa, resulted in 2.26 g L−1 honey brown crude biosurfactant.72 In opposed to that, the recovery outcome from this study is contradictory to the study of ref. 73 and 74 where ethyl acetate could recover least biosurfactant upon extraction. However, the European Union (EU) regulation recommends the use of ethyl acetate for solvent extraction process74 in the case of food applications and other human consumables. The microscopic analysis of biosurfactant showed oily droplet like composition under the lens (Fig. 5).
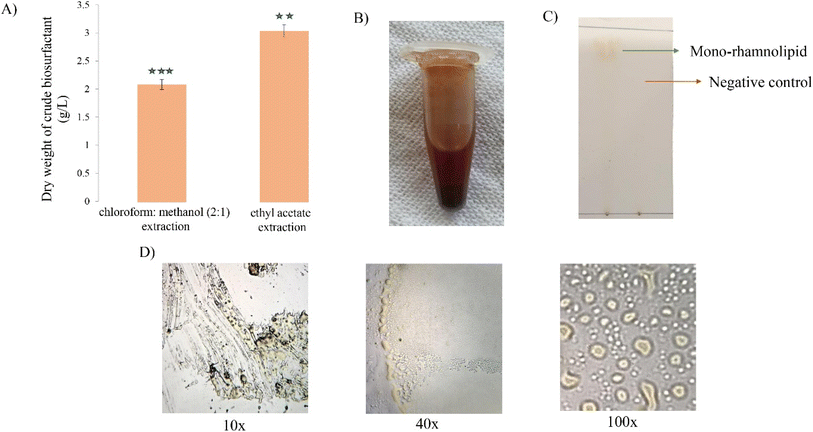 |
| Fig. 5 Comparison of biosurfactant amount (g L−1) extracted from chloroform : methanol (1 : 1) and ethyl acetate treatment (A); crude biosurfactant (B); TLC performed on purified biosurfactant where the left lane contains sample along with ethyl acetate and the right lane contains ethyl acetate only (C); biosurfactant molecules under compound microscope at 10×, 40× and 100× resolution (D). The statistical analysis was performed using SPSS statistical package (version22) for the one-way ANOVA, where the values were recorded as significant shown by asterisk (**, p < 0.01; and ***, p < 0.001). | |
3.5 Structural characterization of obtained biosurfactant product
3.5.1 Biosurfactant purification and TLC. Following biosurfactant purification, the fraction eluted after column chromatography using ethyl acetate (100%) showed only one spot on the TLC plates with Rf value 0.81 (Fig. 5C). Ghazi et al. reported the production of monorhamnolipid by Pseudomonas guguanensis with an Rf value of 0.83.65 A likely findings were revealed by Sun and his colleagues where a glycolipid produced from P. aeruginosa formed two spots of di and monorhamnolipid with Rf values 0.53 and 0.85, respectively on TLC analysis.75
3.5.2 NMR. The 1H and 13C NMR spectra of purified biosurfactant are illustrated in Fig. 6 showing some characteristic chemical shifts clearly indicative for rhamnolipid like biosurfactant.76 The long aliphatic chain and sugar ring were confirmed by the significant signals in the region of 0.86–1.5 ppm in 1H NMR analysis. Particularly the methyl rhamnosyl moiety is attributed to the doublet chemical shifts at around 1.3 ppm. The resonance peaks between 2.5 and 2.9 ppm indicated the probable occurrence of –COO–CH2– whereas the peak at 4.043 ppm denotes the existence of –CH2O– due to the β hydroxyl fatty acid chain in the sample. The doublet chemical shift of 1H NMR at 5.051 ppm confirmed the presence of –CH–O–C moiety in the sugar ring. Similarly, 13C NMR spectrum shows significant signals at 14.11 ppm and from 21.04 to 36.53 ppm denoting –CH3 and the presence of lipid in the sample respectively. Also, the signal for –CH2O– was generated at 60.41 ppm whereas the ester and ketonic group (C
O) peaks were shifted at 171.16 and 173.39 ppm respectively. The absence of any resonance peak for aromatic or amine group and the occurrence of long aliphatic chain with ketone and ester groups suggests the sample having chemical structure of mono-lipid rhamnolipid.77–79
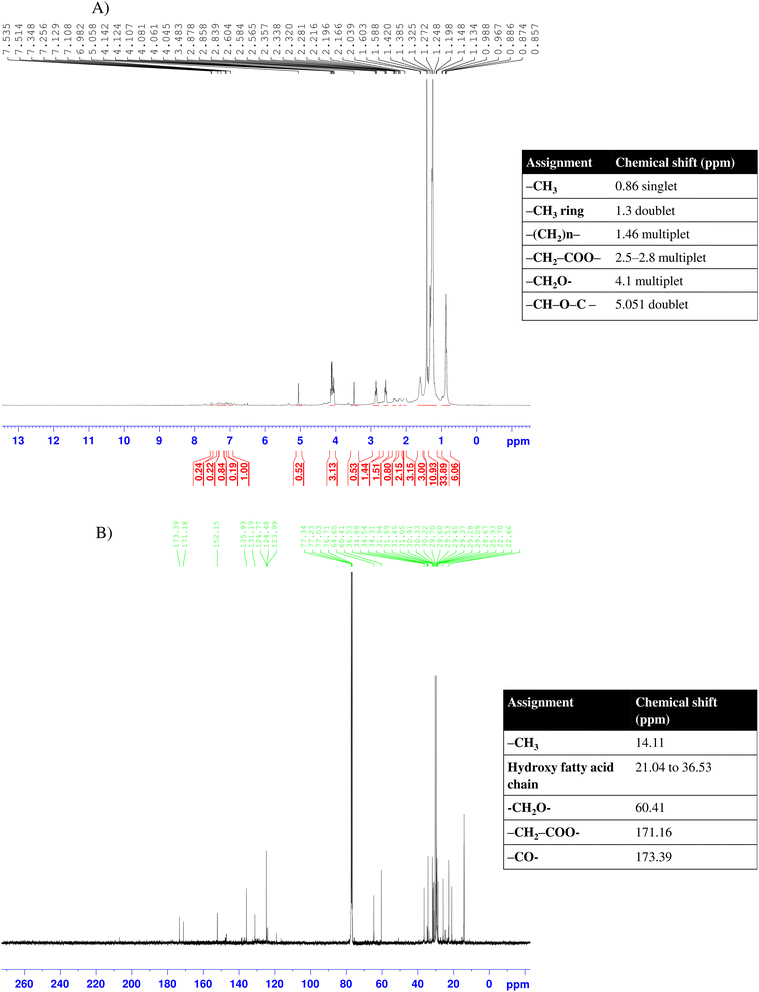 |
| Fig. 6 1H NMR spectrum (A), and 13C NMR spectrum (B) of purified biosurfactant extracted from B. velezensis. | |
3.5.3 FT-IR analysis. Fourier transform infrared (FT-IR) spectra of purified biosurfactant demonstrating maximum absorption at distinct wavenumber positions due to the presence of specific functional groups are shown in Fig. 7. Both KBr and ATR-FTIR spectrum showed several significant peaks at almost same range of wavenumber. The characteristic peak noticed at 3358 cm−1 in ATR-FTIR and 3406 cm−1 in KBr-FTIR confirms the stretching vibration of hydroxyl (–OH) functional group.80,81 The two consecutive peaks at the positions of 2927 and 2855 cm−1 (ATR-FTIR) are attributed to the stretching bands of aliphatic chains (–CH2– and –CH3 groups). The ketonic (C
O) group was identified by the sharply pointed band at wavenumber 1719 cm−1 and 1729 cm−1 in ATR and KBr spectrum respectively.82 The ester carbonyl group (COOR–) and the epoxy group (C–O–C) were confirmed by the stretching bands originated at 1552 and 1163 cm−1 respectively (ATR spectrum). In the fingerprint region (by the bands below 1500 cm−1 to 500 cm−1), the absorption bands between 1485 and 1330 cm−1 indicates the deformation vibrations of alkyl groups according to. All these peaks obtained from FT-IR analysis suggest the structure of biosurfactant as rhamnolipid homologue following the findings consistent with the other reported works.83
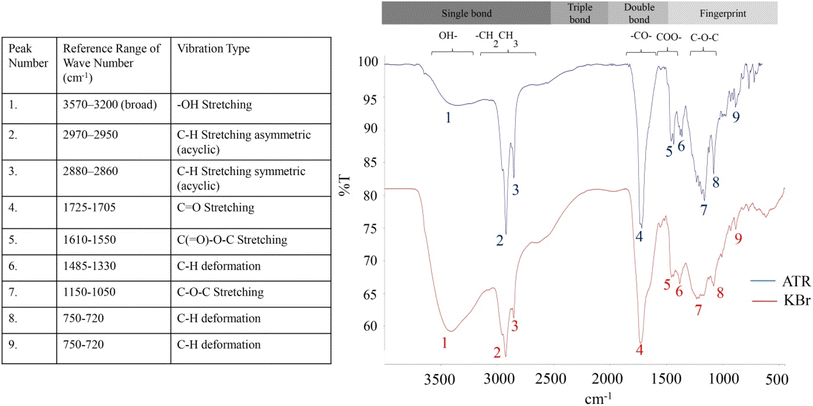 |
| Fig. 7 FT-IR spectrum of purified biosurfactant. | |
3.5.4 Rhamnose test. Following the data derived from NMR and FTIR, a UV-vis spectrum based rhamnose test was performed for the purified biosurfactant and the results was found as positive (Fig. 8A) exhibiting a significant peak at 490 nm. This indicates the glucose moiety in the biosurfactant to be a rhamnose sugar.
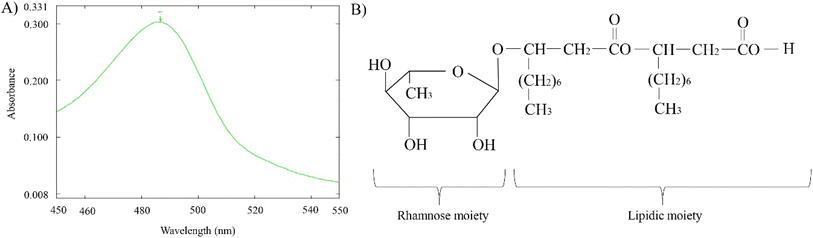 |
| Fig. 8 A diagram showing the presence of rhamnose moiety in the extracted biosurfactant structure; spectrophotometric analysis exhibiting the rhamnose positive test (A); the structure of monorhamnolipid indicating its rhamnose and lipid moiety in the structure (B). | |
All the findings of NMR, FTIR and rhamnose test suggested a likely structure of the compound having rhamnose and lipidic group. Compared to previous reports, the extracted biosurfactant which were confirmed as rhamnolipid showed similar experiment outcome.76,77 To date, Pseudomonas spp have been majorly reported to produce rhamnolipid like biosurfactant while Bacillus spp have been found as the potential secreter of surfactin-type biosurfactant.84 In fact, Bacillus velezensis have also been globally recorded as surfactin producer so far.60,85 To our best knowledge, this is the first time the isolated B. velezensis S2 strain from this study has been proved to synthesize rhamnolipid-like biosurfactant.
Rhamnolipid belongs to amphiphilic glycolipid group consisting of one or two rhamnose units (mono or di-rhamno) and attached to one or two lipid chains (mono or di-lipid). The variation in sugar moiety and the fatty acid chain quantity generates quite a number of rhamnolipid homologue in nature.76,77 Interestingly, the di lipid-rhamnolipids form an ester bond linking two fatty acid groups of the lipid chains. This ester group can transform to its stereoselective forms like ether, amide, hydrocarbon or ketone; opening a broad spectrum of biological functions compared to other biomolecules.77–79 This may explain the reason why some rhamnolipids are well known for their anti-bacterial or anticancer activity, while the others do not show such properties (e.g. the rhamnolipid purified in this study).
3.5.5 Elemental analysis. Following the outcome of FTIR, 1H NMR and 13C NMR of the extracted biosurfactant, the identity of this compound was then confirmed by elemental analysis where the elemental composition of both crude and purified biosurfactant was investigated (Table 1). Here, the carbon percentage in the crude biosurfactant (before column chromatography) was found as 50.10%, which improved to 61.90% after purification. Concurrently, the proportion of Hydrogen molecule rose 1.3 times higher after purification (9.59%). Meanwhile, in crude sample, the presence of nitrogen and sulphur molecule was evident which was not found after refinement of the product. This suggests the native formation of untreated organic sample.
Table 1 Results of elemental analysis of crude and purified biosurfactant
Material |
C (%) |
O (%) |
H (%) |
S (%) |
N (%) |
Crude biosurfactant |
50.10 |
34.64 |
6.96 |
5.788 |
2.51 |
Purified biosurfactant |
61.90 |
28.57 |
9.59 |
— |
— |
If the synthesized compound is rhamnolipid, (C 26 H 48 O 9, mass = 504.7), then using its molecular mass, we can prove the molecular formula of purified biosurfactant as a monorhamnolipid following to the empirical formula calculation86 (Table 2).
Table 2 Empirical formula analysis
Component |
C (%) |
O (%) |
H (%) |
% |
61.90 |
28.57 |
9.59 |
÷Atomic mass |
61.90/12 |
28.57/16 |
9.59/1.0078 |
÷Smallest value |
5.16/1.79 |
1.79/1.79 |
9.523/1.79 |
Ratio |
2.88 |
1 |
5.32 |
Mass of C2.88H5.32O1 = 55.92 |
Ratio of mass (504.7/55.92) = 9.02 |
So the empirical formula, (C2.88H5.32O1)×9.02 = ∼C26H48O9 |
3.5.6 The ultraviolet-visible (UV-vis) analysis. Fig. 9A shows the optical characteristics of the purified biosurfactant recorded at 200–800 wavelength of UV-vis-NIR. The spectral curve represented a strong absorption edge at wavelength ∼265.01 nm with the occurrence of other absorption peaks at 322.9 and 368.14 nm. These findings coincide with the reports of Schenk and his group where they found similar optical feature exhibited by the extracted rhamnolipid like biosurfactant from Pseudomonas aeroginosa.87
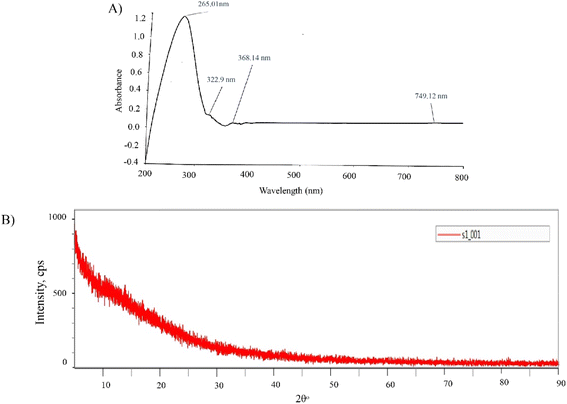 |
| Fig. 9 The optical characteristics of purified biosurfactant exhibiting its UV absorbance at 265.01 nm (A), amorphic structure identified by XRD (B). | |
3.5.7 XRD. X-ray diffraction of the purified biosurfactant exhibited no significant peak except a wide diffracted background (Fig. 9B). This type of appearance suggests the core structure of rhamnolipid as amorphic in nature.88
3.5.8 Thermal analysis. The thermostability of the purified biosurfactant is a crucial criterion for its industrial application. From the thermal analysis profiles (Fig. 10), it is clearly evitable that the thermolysis of the sample followed 3 major steps of weight loss between the temperature range of 25 °C and 600 °C. Initially, a gradual loss of 3.7% occurred up to 180 °C due to the release of physically bounded water from the compound, denoting insignificant presence of moisture of the product in that temperature.89 A sharp fall in the TG curve from 193 °C to 347 °C revealed the major degradation of the product (approximately 80%), which may associate with the breakdown of rhamnose moiety of rhamnolipid. Abbasi and his colleagues shared a similar observation about rhamnolipid produced by Pseudomonas aeruginosa,90 where around 80% of the total biosurfactant weight was lost at 300 °C. In the following stage, a decrement of almost 13.4% weight occurred from 374 °C to 596 °C corresponding to the possible decomposition of hydrocarbon chain in the lipid portion. This finding is accompanied by the DTG curve demonstrating maximum weight degradation rate as 360 mg min−1 and 334 mg min−1 acquired at 223 °C and 344.1 °C respectively. The appearance of a sharp endothermic effect in the DTA curve was evitable from the beginning of the thermolysis process with an extreme at 191 °C attributing to the rhamnose ring degradation. All findings from the thermos-analysis are analogous to the remarks of other studies corresponding to rhamnolipid.82,91
 |
| Fig. 10 A graphical illustration showing the thermogravimetric (TG), difference thermo-gravimetric (DTG), and differential thermal analysis (DTA) performed on the extracted biosurfactant produced from S2 strain. | |
As the surfactant isolated in this study was found to be thermally stable up to 180 °C, it can be considered for its commercial application at high temperature reservoir conditions including cleaning.
3.6 Determination of foaming properties
Fig. 11A exhibits the remarkable foaming and emulsifying abilities of rhamnolipid biosurfactant (1 g L−1) compared to the pure commercial products (tween-20 and tween-80). While tween-20 could only produce foam and tween-80 significantly emulsified the solution; rhamnolipid from this study, showed both characteristics. Several reports on rhamnolipid suggest its notable foaming abilities92 while others added up to its emulsification property.93,94 Desai et al. confirmed that hexose containing fatty acid esters produced by microorganisms are better surfactant than the anionic chemical surfactant as sodium bis(2-ethylhexyl) sulfosuccinate.49 Calvo et al. reported the significance of rhamnolipid as a bioemulsifier in oil bio-remediation.95 In other study, the emulsification property of rhamnolipid was suggested to be used in food industry as bio – preservative.96 Therefore, both the emulsification and foaming properties of the isolated rhamnolipid suggests its wider application in different industrial sections.
 |
| Fig. 11 An observation of foaming and emulsification stability of the isolated rhamnolid in presence of pure tween-20 (commercial surfactant) and tween-80 (commercial emulsifier) (A); the CMC of purified biosurfactant determined by plotting the surface tension against the respective concentrations of the surfactant (B). Here, error bars represent the standard deviations (n = 3). | |
3.7 CMC measurement
As per Fig. 11B, the purified biosurfactant exhibited a CMC of 45 mg L−1 showing effective surface activity as it decreased the ST of water from 72 to 30 mN m−1. The determination of CMC is quite significant for the industrial use of a surfactant because it denotes the efficacy of the surfactant molecule. As the ST cannot be reduced once the CMC is reached, meaningful use of the surfactant up to a certain amount can be specified.
3.8 Antibacterial test
In general, rhamnolipids and other biosurfactants are well known for their antibacterial activity.97,98 It has been found that rhamnolipid has the permeability to cell membrane, which is why they can inhibit bacterial cell growth to a varying degree.99 Several reports have also mentioned their biofilm-disrupting and antiadhesive properties which make them good candidates to be considered as antibacterial agents.100,101 In this experiment, antibacterial assay of isolated rhamnolipid was carried out against S. aureus and E. coli bacterial strains. Based on the inhibition response analysis,102 the biosurfactant was found ineffective against E. coli while it showed weak activity against S. aureus (Fig. 12A and B). Interestingly, the radius of inhibition zones did not extend constantly with the increasing amount of biosurfactant concentrations (25, 50, 100 μg mL−1), rather remained same (Fig. 12C). However, Ferreira et al.103 reported that the antibacterial efficacy of rhamnolipid is pH dependent. The group explained that the Gram-positive pathogens (S. aureus) were more sensitive to rhamnolipid at acidic environment while the Gram-negative pathogens (E. coli) were resistant to it at all pH studied (pH 5–8). Other reports also suggested mono-rhamnolipid having better antimicrobial applications in agriculture as potential agricultural pathogens, such as Pantoea agglomerans, Alternaria alternata and Cladosporium sp showed significant sensitivity towards it.104 It is visible that, the commercial surfactant, tween-20 also showed no activity against the pathogens (Fig. 12A and B). However, there was an opalescent zone around the well containing tween-20 in S. aureus lawned plate. This happened due to the lipase production by the bacteria which hydrolyzed tween-20 and produced water insoluble fatty acid precipitation around the well.105
 |
| Fig. 12 Antibacterial response of rhamnolipid biosurfactant. Antibacterial activity of the biosurfactant in presence of commercial surfactant (tween-20) and negative control (PBS) (labelled as ‘bio’, ‘T20’ and ‘Neg’ respectively) against E. coli (A) and S. aureus (B) poor inhibition responses were recorded for different concentration of biosurfactants against S. aureus (C and D). | |
3.9 Cytotoxicy
Fig. 13A–E depicts the microscopic images of the Vero cells exposed to different concentration of biosurfactant (100, 250, 500, 1000 μg mL−1) under controlled conditions, and Fig. 13F shows the corresponding cell viability in comparison to the control. Notably, the viability rate of Vero cells gradually decreased with the increased concentration of biosurfactant treatment but not in a significant way (p = 0.10). In comparison to the control (94.63%), the viability rate was found as 93%, 92.33%, 91.20% and 88.70% while treating with 100, 250, 500 and 1000 μg mL−1 biosurfactant respectively.
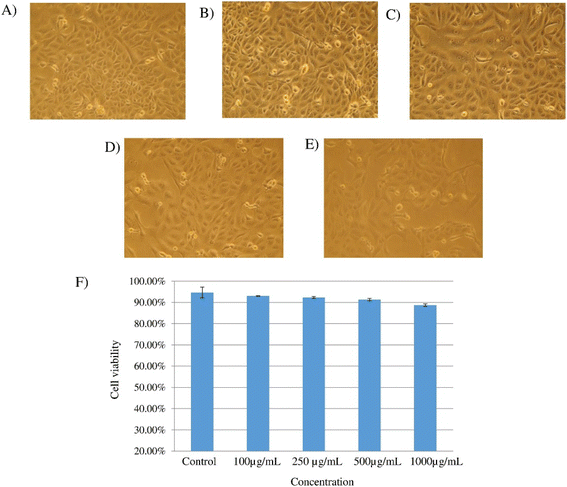 |
| Fig. 13 (A–E) microscopic images of the Vero cells – control treatment (A); biosurfactant = 100 μg mL−1 (B); biosurfactant = 250 μg mL−1 (C); biosurfactant = 500 μg mL−1 (D) and biosurfactant = 1000 μg mL−1 (E). A bar diagram representing viability rate of Vero cells after treating with biosurfactant in comparison to the control (DMSO) (F). The statistical analysis was performed using SPSS statistical package (version22) for the one-way ANOVA. | |
As the isolated biosurfactant was found non-toxic for the epithelial cells (Vero cell line) and showed insignificant antibacterial activity, therefore, both observations suggest the extracted biosurfactant as a safe biomaterial for commercial use as cleaning agent and emulsifier.
4. Limitations
The major limitations of this study include the missing of determining cost-effective conditions for large scale biosurfactant production. Also, a broad molecular analysis of the isolate could widen its scope in different industrial use including enzyme and other bio-active compound production. Furthermore, in this study the detailed applications of extracted biosurfactant were not evaluated. All these shortcomings would be considered as prospects for future investigations of the study.
5. Conclusion
Rhamnolipid is one of the renowned biosurfactants for being biodegradable and eco-friendly. This makes them better choice for a broad range of applications in food preservation, oil recovery, detergents, cosmetics, and pharmaceuticals. In this present study, a rhamnolipid producer Bacillus velezensis S2, isolated from oil-contaminated soil showed remarkable crude oil degradation capacity. When the strain was tested for its biosurfactant producing ability, it confirmed the secretion of a biosurfactant having extensive surface tension reducing and high emulsifying activity. Upon extraction and purification, the biosurfactant product was confirmed as rhamnolipid via TLC, NMR, FT-IR and spectrophotometric analysis. The foam test, emulsification index, antibacterial test, cytotoxic analysis, and thermal stability confirmed its safe and wide use in various applications, particularly in cleaning and cosmetics industry. However, the yield amount is considerably poor in proportion to the extraction and purification expenses which largely inhibits its industrial use. A well-defined large-scale fermentation method for an optimum amount of biosurfactant production with minimal down-stream cost demands elaborate studies.
Data availability
As the corresponding author of this study, I certify that all data underlying the findings are available as the part of the manuscript and no additional source of data is required.
Author contributions
Shahnaz Sultana: conceptualization, data curation, analysis, methodology, writing original draft, editing. Rokaia Sultana: conceptualization, analysis, methodology, writing review. Md. Abdullah Al-Mansur: data curation, analysis, supervision. Md. Ahedul Akbor: data curation, analysis. Nasrin Akter Bhuiyan: analysis, methodology, writing editing. Shamim Ahmed: investigation, resources. Sabina Yasmin: data analysis. A. H. M. Shofiul Islam Molla Jamal: data analysis.
Conflicts of interest
The authors declare no conflict of interest.
Acknowledgements
The authors are grateful to the Bangladesh Council of Scientific and Industrial Research (BCSIR) for financial support (R&D approved by fiscal year 2020–21, ref. no. 39.02.0000.011.14.128.2020/636, date 29.12.2020) and facilities. We are also thankful to M. A. Gafur, PSO; Syed Farid Uddin Farhad, PSO; Suriya Sharmin, SSO and Md. Ashraful Alam, SO for their help in thermal analysis, surface tension, cellular cytotoxity test and XRD respectively.
References
- J. Zhang, Q. Xue, X. Pan, Y. Jin, W. Lu, D. Ding and Q. Guo, Graphene oxide/polyacrylonitrile fiber hierarchical-structured membrane for ultra-fast microfiltration of oil-water emulsion, Chem. Eng. J., 2017, 307, 643–649 CrossRef CAS
. - B. E. Finch and W. A. Stubblefield, Phototoxicity assessments of field sites in Barataria Bay, Louisiana, USA, and heavily weathered Macondo crude oil: 4 years after the Deepwater Horizon oil spill, Environ. Toxicol. Chem., 2019, 38(8), 1811–1819 CrossRef CAS PubMed
. - S. Kottuparambil and S. Agusti, Cell-by-cell estimation of PAH sorption and subsequent toxicity in marine phytoplankton, Chemosphere, 2020, 259, 127487 CrossRef CAS PubMed
. - M. I. Cerezo and S. Agusti, Polycyclic aromatic hydrocarbons alter the structure of oceanic and oligotrophic microbial food webs, Mar. Pollut. Bull., 2015, 101(2), 726–735 CrossRef CAS PubMed
. - J. R. Polli, B. R. Rushing, L. Lish, L. Lewis, M. I. Selim and X. Pan, Quantitative analysis of PAH compounds in DWH crude oil and their effects on Caenorhabditis elegans germ cell apoptosis, associated with CYP450s upregulation, Sci. Total Environ., 2020, 745, 140639 CrossRef CAS PubMed
. - The third pole, The report 2019, available from: https://www.thethirdpole.net/en/pollution/dolphins-threatened-by-bangladesh-oil-spill/.
- R. D. C. F. Silva, D. G. Almeida, R. D. Rufino, J. M. Luna, V. A. Santos and L. A. Sarubbo, Applications of biosurfactants in the petroleum industry and the remediation of oil spills, Int. J. Mol. Sci., 2014, 15(7), 12523–12542 CrossRef PubMed
. - S. Patel, A. Homaei, S. Patil and A. Daverey, Microbial biosurfactants for oil spill remediation: pitfalls and potentials, Appl. Microbiol. Biotechnol., 2019, 103(1), 27–37 CrossRef CAS PubMed
. - M. Adam, Biodegradation of marine crude oil pollution using a salt-tolerant bacterial consortium isolated from Bohai Bay, China, Mar. Pollut. Bull., 2016, 105(1), 43–50 CrossRef PubMed
. - X. Tang, L. He, X. Tao, Z. Dang, C. Guo, G. Lu and X. Yi, Construction of an artificial microalgal-bacterial consortium that efficiently degrades crude oil, J. Hazard. Mater., 2010, 181(1–3), 1158–1162 CrossRef CAS PubMed
. - C. Dechsakulwatana, A. Rungsihiranrut, C. Muangchinda, R. Ningthoujam, P. Klankeo and O. Pinyakong, Biodegradation of petroleum oil using a constructed nonpathogenic and heavy metal-tolerant bacterial consortium isolated from marine sponges, J. Environ. Chem. Eng., 2022, 10(6), 108752 CrossRef CAS
. - M. Marti, W. Colonna, P. Patra, H. Zhang, C. Green and G. Reznik, et al., Production and characterization of microbial biosurfactants for potential use in oil-spill remediation, Enzyme Microb. Technol., 2014, 55, 31–39 CrossRef CAS PubMed
. - H. Hajfarajollah, S. Mehvari, M. Habibian, B. Mokhtarani and K. A. Noghabi, Rhamnolipid biosurfactant adsorption on a plasma-treated polypropylene surface to induce antimicrobial and antiadhesive properties, RSC Adv., 2015, 5(42), 33089–33097 RSC
. - P. S. Kumar and P. T. Ngueagni, A review on new aspects of lipopeptide biosurfactant: Types, production, properties and its application in the bioremediation process, J. Hazard. Mater., 2021, 407, 124827 CrossRef PubMed
. - S. Shekhar, A. Sundaramanickam and T. Balasubramanian, Biosurfactant producing microbes and their potential applications: a review, Crit. Rev. Environ. Sci. Technol., 2015, 45(14), 1522–1554 CrossRef CAS
. - I. O. Olasanmi and R. W. Thring, The role of biosurfactants in the continued drive for environmental sustainability, Sustainability, 2018, 10(12), 4817 CrossRef
. - S. S. Cameotra, R. S. Makkar, J. Kaur and S. K. Mehta, Synthesis of biosurfactants and their advantages to microorganisms and mankind, Biosurfactants, 2010, 261–280 CAS
. - R. S. Makkar, S. S. Cameotra and I. M. Banat, Advances in utilization of renewable substrates for biosurfactant production, AMB Express, 2011, 1, 1–19 CrossRef PubMed
. - T. G. Ambaye, M. Vaccari, S. Prasad and S. Rtimi, Preparation, characterization and application of biosurfactant in various industries: A critical review on progress, challenges and perspectives, Environ. Technol. Innovation, 2021, 24, 102090 CrossRef CAS
. - B. C. P. Sanches, C. A. Rocha, J. G. Martin Bedoya, V. Ld Silva, P. Bd Silva and A. M. Fusco-Almeida, et al., Rhamnolipid-based liposomes as promising nano-carriers for enhancing the antibacterial activity of peptides derived from bacterial toxin-antitoxin systems, Int. J. Nanomed., 2021, 925–939 CrossRef PubMed
. - A. R. da Silva, M. Á. Manresa, A. Pinazo, M. T. García and L. Pérez, Rhamnolipids functionalized with basic amino acids: Synthesis, aggregation behavior, antibacterial activity and biodegradation studies, Colloids Surf., B, 2019, 181, 234–243 CrossRef PubMed
. - S. Sharma, R. Verma and L. M. Pandey, Crude oil degradation and biosurfactant production abilities of isolated Agrobacterium fabrum SLAJ731, Biocatal. Agric. Biotechnol., 2019, 21, 101322 CrossRef
. - O. Marchut-Mikolajczyk, P. Drożdżyński, D. Pietrzyk and T. Antczak, Biosurfactant production and hydrocarbon degradation activity of endophytic bacteria isolated from Chelidonium majus L, Microb. Cell Fact., 2018, 17(1), 1–9 CrossRef PubMed
. - S.-J. Yuan, W.-W. Li, Y.-Y. Cheng, H. He, J.-J. Chen and Z.-H. Tong, et al., A plate-based electrochromic approach for the high-throughput detection of electrochemically active bacteria, Nat. Protoc., 2014, 9(1), 112–119 CrossRef CAS PubMed
. - A. Reyes-César, A. E. Absalon, F. J. Fernández, J. M. González and D. V. Cortés-Espinosa, Biodegradation of a mixture of PAHs by non-ligninolytic fungal strains isolated from crude oil-contaminated soil, World J. Microbiol. Biotechnol., 2014, 30, 999–1009 CrossRef PubMed
. - D. H. Bergey, Bergey's Manual of Determinative Bacteriology, Lippincott Williams & Wilkins, 1994 Search PubMed
. - B. C. Millar, X. Jiru, J. E. Moore and J. A. Earle, A simple and sensitive method to extract bacterial, yeast and fungal DNA from blood culture material, J. Microbiol. Methods, 2000, 42(2), 139–147 CrossRef CAS PubMed
. - S. J. Varjani, D. P. Rana, A. K. Jain, S. Bateja and V. N. Upasani, Synergistic ex-situ biodegradation of crude oil by halotolerant bacterial consortium of indigenous strains isolated from on shore sites of Gujarat, India, Int. Biodeterior. Biodegrad., 2015, 103, 116–124 CrossRef CAS
. - H. Liu, G. Yang, H. Jia and B. Sun, Crude oil degradation by a novel strain Pseudomonas aeruginosa AQNU-1 isolated from an oil-contaminated lake wetland, Processes, 2022, 10(2), 307 CrossRef CAS
. - K. Patowary, R. Patowary, M. C. Kalita and S. Deka, Development of an efficient bacterial consortium for the potential remediation of hydrocarbons from contaminated sites, Front. Microbiol., 2016, 7, 1092 Search PubMed
. - A. A. Bodour and R. M. Miller-Maier, Application of a modified drop-collapse technique for surfactant quantitation and screening of biosurfactant-producing microorganisms, J. Microbiol. Methods, 1998, 32(3), 273–280 CrossRef CAS
. - M. Morikawa, Y. Hirata and T. Imanaka, A study on the structure–function relationship of lipopeptide biosurfactants, Biochim. Biophys. Acta, Mol. Cell Biol. Lipids, 2000, 1488(3), 211–218 CrossRef CAS PubMed
. - D. G. Cooper and B. G. Goldenberg, Surface-active agents from two Bacillus species, Appl. Environ. Microbiol., 1987, 53(2), 224–229 CrossRef CAS PubMed
. - M. Rosenberg, D. Gutnick and E. Rosenberg, Adherence of bacteria to hydrocarbons: a simple method for measuring cell-surface hydrophobicity, FEMS Microbiol. Lett., 1980, 9(1), 29–33 CrossRef CAS
. - E. Ramé, The interpretation of dynamic contact angles measured by the Wilhelmy plate method, J. Colloid Interface Sci., 1997, 185(1), 245–251 CrossRef PubMed
. - Screening and Characterization of Biosurfactant Produced by Bacterial Consortium in Degrading Polycyclic Aromatic Hydrocarbon Compound. AIP Conference Proceedings, ed. A. Sumiardi, E. S. Soetarto and D. Susilaningsih, AIP Publishing, 2018 Search PubMed
. - B. Anandaraj and P. Thivakaran, Isolation and production of
biosurfactant producing organism from oil spilled soil, J. Biosci. Technol., 2010, 1(3), 120–126 Search PubMed
. - S. O. Adebajo, A. K. Akintokun, A. E. Ojo, D. M. Egbagbe, P. O. Akintokun and L. O. Adebajo, Biosurfactant Production by Rhizospheric Bacteria Isolated from Biochar Amended Soil Using Different Extraction Solvents, Appl. Environ. Res., 2019, 41(3), 72–82 Search PubMed
. - N. Qiao and Z. Shao, Isolation and characterization of a novel biosurfactant produced by hydrocarbon-degrading bacterium Alcanivorax dieselolei B-5, J. Appl. Microbiol., 2010, 108(4), 1207–1216 CrossRef CAS PubMed
. - H. Wasoh, S. Baharun, M. Halim, A. F. Lajis, A. Ariff and O.-M. Lai, Production of rhamnolipids by locally isolated Pseudomonas aeruginosa using sunflower oil as carbon source, Biorem. Sci. Technol. Res., 2017, 5(1), 1–6 CrossRef
. - M. Ueland, J. M. Howes, S. L. Forbes and B. H. Stuart, Degradation patterns of natural and synthetic textiles on a soil surface during summer and winter seasons studied using ATR-FTIR spectroscopy, Spectrochim. Acta, Part A, 2017, 185, 69–76 CrossRef CAS PubMed
. - M. DuBois, K. A. Gilles, J. K. Hamilton, P. Rebers and F. Smith, Colorimetric method for determination of sugars and related substances, Anal. Chem., 1956, 28(3), 350–356 CrossRef CAS
. - M. Abouseoud, R. Maachi, A. Amrane, S. Boudergua and A. Nabi, Evaluation of different carbon and nitrogen sources in production of biosurfactant by Pseudomonas fluorescens, Desalination, 2008, 223(1–3), 143–151 CrossRef CAS
. - M. Nitschke, C. Ferraz and G. M. Pastore, Selection of microorganisms for biosurfactant production using agroindustrial wastes, Braz. J. Microbiol., 2004, 35, 81–85 CrossRef
. - N. F. Paoni and Jr D. E. Koshland, Permeabilization of cells for studies on the biochemistry of bacterial chemotaxis, Proc. Natl. Acad. Sci. U. S. A., 1979, 76(8), 3693–3697 CrossRef CAS PubMed
. - C. S. Compaoré, D. S. Nielsen, L. I. Ouoba, T. S. Berner, K. F. Nielsen and H. Sawadogo-Lingani, et al., Co-production of surfactin and a novel bacteriocin by Bacillus subtilis subsp. subtilis H4 isolated from Bikalga, an African alkaline Hibiscus sabdariffa seed fermented condiment, Int. J. Food Microbiol., 2013, 162(3), 297–307 CrossRef PubMed
. - N. Khan, F. Afroz, M. Begum, S. Rony, S. Sharmin and F. Moni, et al., Endophytic Fusarium solani: a rich source of cytotoxic and antimicrobial napthaquinone and aza-anthraquinone derivatives, Toxicol. Rep., 2018, 5, 970–976 CrossRef CAS PubMed
. - S. S. Cameotra and R. S. Makkar, Recent applications of biosurfactants as biological and immunological molecules, Curr. Opin. Microbiol., 2004, 7(3), 262–266 CrossRef CAS PubMed
. - J. D. Desai and I. M. Banat, Microbial production of surfactants and their commercial potential, Microbiol. Mol. Biol. Rev., 1997, 61(1), 47–64 CAS
. - N. Lang, P. Delichere and A. Tuel, Post-synthesis introduction of transition metals in surfactant-containing MCM-41 materials, Microporous Mesoporous Mater., 2002, 56(2), 203–217 CrossRef CAS
. - L. Rodrigues, I. M. Banat, H. Van der Mei, J. Teixeira and R. Oliveira, Interference in adhesion of bacteria and yeasts isolated from explanted voice prostheses to silicone rubber by rhamnolipid biosurfactants, J. Appl. Microbiol., 2006, 100(3), 470–480 CrossRef CAS PubMed
. - A. F. Almansoory, H. A. Hasan, S. R. S. Abdullah, M. Idris, N. Anuar and W. M. Al-Adiwish, Biosurfactant produced by the hydrocarbon-degrading bacteria: Characterization, activity and applications in removing TPH from contaminated soil, Environ. Technol. Innovation, 2019, 14, 100347 CrossRef
. - D. Yalaoui-Guellal, S. Fella-Temzi, S. Djafri-Dib, S. K. Sahu, V. U. Irorere, I. M. Banat and K. Madani, The petroleum-degrading bacteria Alcaligenes aquatilis strain YGD 2906 as a potential source of lipopeptide biosurfactant, Fuel, 2021, 285, 119112 CrossRef CAS
. - L. Fracchia, M. Cavallo, M. G. Martinotti and I. M. Banat, Biosurfactants and bioemulsifiers biomedical and related applications–present status and future potentials, Biomed. Sci., Eng. Technol., 2012, 14(1), 1–49 Search PubMed
. - M. E. Stanghellini and R. M. Miller, Biosurfactants: their identity and potential efficacy in the biological control of zoosporic plant pathogens, Plant Dis., 1997, 81(1), 4–12 CrossRef CAS PubMed
. - C. Wang, D. Dong, H. Wang, K. Müller, Y. Qin, H. Wang and W. Wu, Metagenomic analysis of microbial consortia enriched from compost: new insights into the role of Actinobacteria in lignocellulose decomposition, Biotechnol. Biofuels, 2016, 9, 1–17 CrossRef CAS PubMed
. - Y. Li, L. Lei, L. Zheng, X. Xiao, H. Tang and C. Luo, Genome sequencing of gut symbiotic Bacillus velezensis LC1 for bioethanol production from bamboo shoots, Biotechnol. Biofuels, 2020, 13, 1–12 CrossRef PubMed
. - N. T. Quach, T. H. N. Vu, N. A. Nguyen, T. L. Bui, S. C. Ky and T. L. Le, et al., Phenotypic features and analysis of genes supporting probiotic action unravel underlying perspectives of Bacillus velezensis VTX9 as a potential feed additive for swine, Ann. Microbiol., 2021, 71(1), 1–14 CrossRef
. - A. S. Nair, S. Al-Bahry and N. Sivakumar, Co-production of microbial lipids and biosurfactant from waste office paper hydrolysate using a novel strain Bacillus velezensis ASN1, Biomass Convers. Biorefin., 2020, 10, 383–391 CrossRef CAS
. - K. R. Meena, R. Dhiman, K. Singh, S. Kumar, A. Sharma and S. S. Kanwar, et al., Purification and identification of a surfactin biosurfactant and engine oil degradation by Bacillus velezensis KLP2016, Microb. Cell Factories, 2021, 20, 1–12 CrossRef PubMed
. - F. Chaillan, A. Le Flèche, E. Bury, Y.-H. Phantavong, P. Grimont, A. Saliot and J. Oudot, Identification and biodegradation potential of tropical aerobic hydrocarbon-degrading microorganisms, Res. Microbiol., 2004, 155(7), 587–595 CrossRef CAS PubMed
. - A. Nayarisseri, P. Singh and S. K. Singh, Screening, isolation and characterization of biosurfactant producing Bacillus subtilis strain ANSKLAB03, Bioinformation, 2018, 14(6), 304 CrossRef PubMed
. - P. Bharmoria, S. Hisamitsu, H. Nagatomi, T. Ogawa, M.-A. Morikawa, N. Yanai and N. Kimizuka, Simple and versatile platform for air-tolerant photon upconverting hydrogels by biopolymer–surfactant–chromophore co-assembly, J. Am. Chem. Soc., 2018, 140(34), 10848–10855 CrossRef CAS PubMed
. - V. B. Fainerman, E. V. Aksenenko, A. V. Makievski, M. V. Nikolenko, A. Javadi, E. Schneck and R. Miller, Particular behavior of surface tension at the interface between aqueous solution of surfactant and alkane, Langmuir, 2019, 35(47), 15214–15220 CrossRef CAS PubMed
. - F. Z. Ghazi, M. Sallal Mahdi and K. H. Alobaidi, Optimization and Chemical Characterization of Biosurfactant Produced from a Novel Pseudomonas guguanensis Strain Iraqi ZG. KM, Int. J. Microbiol., 2023, 2023(1), 1571991 Search PubMed
. - A. Saimmai, O. Rukadee, T. Onlamool, V. Sobhon and S. Maneerat, Isolation and functional characterization of a biosurfactant produced by a new and promising strain of Oleomonas sagaranensis AT18, World J. Microbiol. Biotechnol., 2012, 28, 2973–2986 CrossRef CAS PubMed
. - V. Tripathi, V. K. Gaur, N. Dhiman, K. Gautam and N. Manickam, Characterization and properties of the biosurfactant produced by PAH-degrading bacteria isolated from contaminated oily sludge environment, Environ. Sci. Pollut. Res., 2020, 27, 27268–27278 CrossRef CAS PubMed
. - G. Seydlová and J. Svobodová, Development of membrane lipids in the surfactin producer Bacillus subtilis, Folia Microbiol., 2008, 53, 303–307 CrossRef PubMed
. - K.-I. Hisatsuka, T. Nakahara, N. Sano and K. Yamada, Formation of rhamnolipid by Pseudomonas aeruginosa and its function in hydrocarbon fermentation, Agric. Biol. Chem., 1971, 35(5), 686–692 CrossRef
. - A. M. Abdel-Mawgoud, F. Lépine and E. Déziel, Rhamnolipids: diversity of structures, microbial origins and roles, Appl. Microbiol. Biotechnol., 2010, 86, 1323–1336 CrossRef CAS PubMed
. - J. Arutchelvi and M. Doble, Characterization of glycolipid biosurfactant from Pseudomonas aeruginosa CPCL isolated from petroleum-contaminated soil, Lett. Appl. Microbiol., 2010, 51(1), 75–82 CAS
. - K. Patowary, R. Patowary, M. C. Kalita and S. Deka, Characterization of biosurfactant produced during degradation of hydrocarbons using crude oil as sole source of carbon, Front. Microbiol., 2017, 8, 279 Search PubMed
. - S. Adebajo, P. Akintokun, A. Ojo, A. Akintokun and O. Badmos, Recovery of biosurfactant using different extraction solvent by rhizospheric bacteria isolated from rice-husk and poultry waste biochar amended soil, Egypt. J. Basic Appl. Sci., 2020, 7(1), 252–266 Search PubMed
. - A. López-Prieto, A. B. Moldes, J. M. Cruz and C. B. Perez, Towards more ecofriendly pesticides: Use of biosurfactants obtained from the corn milling industry as solubilizing agent of copper oxychloride, J. Surfactants Deterg., 2020, 23(6), 1055–1066 CrossRef
. - W. Sun, W. Cao, M. Jiang, G. Saren, J. Liu and J. Cao, et al., Isolation and characterization of biosurfactant-producing and diesel oil degrading Pseudomonas sp. CQ2 from Changqing oil field, China, RSC Adv., 2018, 8(69), 39710–39720 RSC
. - T. Moussa, M. Mohamed and N. Samak, Production and characterization of di-rhamnolipid produced by Pseudomonas aeruginosa TMN, Braz. J. Chem. Eng., 2014, 31, 867–880 CrossRef
. - T. B. Lotfabad, H. Abassi, R. Ahmadkhaniha, R. Roostaazad, F. Masoomi and H. S. Zahiri, et al., Structural characterization of a rhamnolipid-type biosurfactant produced by Pseudomonas aeruginosa MR01: enhancement of di-rhamnolipid proportion using gamma irradiation, Colloids Surf., B, 2010, 81(2), 397–405 CrossRef CAS PubMed
. - N. Christova, B. Tuleva, A. Kril, M. Georgieva, S. Konstantinov and I. Terziyski, et al., Chemical structure and in vitro antitumor activity of rhamnolipids from Pseudomonas aeruginosa BN10, Appl. Biochem. Biotechnol., 2013, 170, 676–689 CrossRef CAS PubMed
. - K. V. Ramana and N. Karanth, Factors affecting biosurfactant production using Pseudomonas aeruginosa CFTR-6 under submerged conditions, J. Chem. Technol. Biotechnol., 1989, 45(4), 249–257 CrossRef CAS
. - N. Singh, S. C. Pemmaraju, P. A. Pruthi, S. S. Cameotra and V. Pruthi, Candida biofilm disrupting ability of di-rhamnolipid (RL-2) produced from Pseudomonas aeruginosa DSVP20, Appl. Biochem. Biotechnol., 2013, 169, 2374–2391 CrossRef CAS PubMed
. - K. Deepika, M. Raghuram and P. Bramhachari, Rhamnolipid biosurfactant production by Pseudomonas aeruginosa strain KVD-HR42 isolated from oil contaminated mangrove sediments, Afr. J. Microbiol. Res., 2017, 11(6), 218–231 CrossRef CAS
. - R. Khademolhosseini, A. Jafari, S. M. Mousavi, H. Hajfarajollah, K. A. Noghabi and M. Manteghian, Physicochemical characterization and optimization of glycolipid biosurfactant production by a native strain of Pseudomonas aeruginosa HAK01 and its performance evaluation for the MEOR process, RSC Adv., 2019, 9(14), 7932–7947 RSC
. - J. Coates, Interpretation of Infrared Spectra, a Practical Approach, 2000 Search PubMed
. - O. Pornsunthorntawee, P. Wongpanit and R. Rujiravanit, Rhamnolipid biosurfactants: production and their potential in environmental biotechnology, Biosurfactants, 2010, 211–221 CrossRef CAS PubMed
. - P. Jakinala, N. Lingampally, A. Kyama and B. Hameeda, Enhancement of atrazine biodegradation by marine isolate Bacillus velezensis MHNK1 in presence of surfactin lipopeptide, Ecotoxicol. Environ. Saf., 2019, 182, 109372 CrossRef CAS PubMed
. - E. Brand, L. J. Saidel, W. H. Goldwater, B. Kassell and F. J. Ryan, The Empirical Formula of β-Lactoglobulin1, J. Am. Chem. Soc., 1945, 67(9), 1524–1532 CrossRef CAS
. - T. Schenk, I. Schuphan and B. Schmidt, High-performance liquid chromatographic determination of the rhamnolipids produced by Pseudomonas aeruginosa, J. Chromatogr. A, 1995, 693(1), 7–13 CrossRef CAS PubMed
. - S. A. Haeri, S. Abbasi and S. Sajjadifar, Bio-dispersive liquid liquid microextraction based on nano rhaminolipid aggregates combined with magnetic solid phase extraction using Fe3O4@ PPy magnetic nanoparticles for the determination of methamphetamine in human urine, J. Chromatogr. B, 2017, 1063, 101–106 CrossRef CAS PubMed
. - A. J. Habish, S. Lazarević, I. Janković-Častvan, B. Jokić, J. Kovač and J. Rogan, et al., Nanoscale zerovalent iron (nZVI) supported by natural and acid-activated sepiolites: The effect of the nZVI/support ratio on the composite properties and Cd 2+ adsorption, Environ. Sci. Pollut. Res., 2017, 24, 628–643 CrossRef CAS PubMed
. - H. Khoshdast, H. Abbasi, A. Sam and K. A. Noghabi, Frothability and surface behavior of a rhamnolipid biosurfactant produced by Pseudomonas aeruginosa MA01, Biochem. Eng. J., 2012, 60, 127–134 CrossRef CAS
. - P. Singh and B. N. Tiwary, Isolation and characterization of glycolipid biosurfactant produced by a Pseudomonas otitidis strain isolated from Chirimiri coal mines, India, Bioresour. Bioprocess., 2016, 3, 1–16 CrossRef
. - R. Hajimohammadi and S. Johari-Ahar, Synergistic effect of saponin and rhamnolipid biosurfactants systems on foam behavior, Tenside, Surfactants, Deterg., 2018, 55(2), 121–126 CAS
. - E. Z. Ron and E. Rosenberg, Natural roles of biosurfactants: Minireview, Environ. Microbiol., 2001, 3(4), 229–236 CrossRef CAS PubMed
. - B. A. McKew, F. Coulon, M. M. Yakimov, R. Denaro, M. Genovese and C. J. Smith, et al., Efficacy of intervention strategies for bioremediation of crude oil in marine systems and effects on indigenous hydrocarbonoclastic bacteria, Environ. Microbiol., 2007, 9(6), 1562–1571 CrossRef CAS PubMed
. - C. Calvo, M. Manzanera, G. Silva-Castro, I. Uad and J. González-López, Application of bioemulsifiers in soil oil bioremediation processes. Future prospects, Sci. Total Environ., 2009, 407(12), 3634–3640 CrossRef CAS PubMed
. - C. S. Chander, T. Lohitnath, D. M. Kumar and P. Kalaichelvan, Production and characterization of biosurfactant from Bacillus subtilis MTCC441 and its evaluation to use as bioemulsifier for food bio-preservative, Adv. Appl. Sci. Res., 2012, 3(3), 1827–1831 Search PubMed
. - J. N. Sleiman, S. A. Kohlhoff, P. M. Roblin, S. Wallner, R. Gross and M. R. Hammerschlag, et al., Sophorolipids as antibacterial agents, Ann. Clin. Lab. Sci., 2009, 39(1), 60–63 CAS
. - L. Magalhães and M. Nitschke, Antimicrobial activity of rhamnolipids against Listeria monocytogenes and their synergistic interaction with nisin, Food Control, 2013, 29(1), 138–142 CrossRef
. - K. J. Kim, Y. B. Kim, B. S. Lee, D. H. Shin and E. K. Kim, Characteristics of sophorolipid as an antimicrobial agent, J. Microbiol. Biotechnol., 2002, 12(2), 235–241 CAS
. - A. De Giani, J. Zampolli and P. Di Gennaro, Recent trends on biosurfactants with antimicrobial activity produced by bacteria associated with human health: different perspectives on their properties, challenges, and potential applications, Front. Microbiol., 2021, 12, 655150 CrossRef PubMed
. - S. K. Satpute, N. S. Mone, P. Das, I. M. Banat and A. G. Banpurkar, Inhibition of pathogenic bacterial biofilms on PDMS based implants by L. acidophilus derived biosurfactant, BMC Microbiol., 2019, 19(1), 1–15 CrossRef PubMed
. - T. Parnomo, Effect of Arabica Coffee Bean Extract (Coffea arabica) as a Growth Inhibitor of Enterococcus faecalis ATCC 29212, J. Drug Delivery Ther., 2021, 11(3), 89–96 CrossRef CAS
. - J. de Freitas Ferreira, E. A. Vieira and M. Nitschke, The antibacterial activity of rhamnolipid biosurfactant is pH dependent, Food Res. Int., 2019, 116, 737–744 CrossRef CAS PubMed
. - J. Crouzet, A. Arguelles-Arias, S. Dhondt-Cordelier, S. Cordelier, J. Pršić and G. Hoff, et al., Biosurfactants in plant protection against diseases: Rhamnolipids
and lipopeptides case study, Front. Bioeng. Biotechnol., 2020, 8, 1014 CrossRef PubMed
. - D. Nercessian, L. Di Meglio, R. De Castro and R. Paggi, Exploring the multiple biotechnological potential of halophilic microorganisms isolated from two Argentinean salterns, Extremophiles, 2015, 19, 1133–1143 CrossRef CAS PubMed
.
|
This journal is © The Royal Society of Chemistry 2024 |
Click here to see how this site uses Cookies. View our privacy policy here.