DOI:
10.1039/D4RA02152E
(Paper)
RSC Adv., 2024,
14, 16194-16206
Evaluation of multi-target iridium(III)-based metallodrugs in combating antimicrobial resistance and infections caused by Staphylococcus aureus†
Received
21st March 2024
, Accepted 28th April 2024
First published on 20th May 2024
Abstract
The rapid emergence and spread of multidrug-resistant bacteria pose a serious challenge to human life and health, necessitating the development of novel antibacterial agents. Herein, to address this challenge, three iridium-based antibacterial agents were prepared and their antimicrobial activity were explored. Importantly, the three complexes all showed robust potency against S. aureus with MIC values in the range of 1.9–7.9 μg mL−1. Notably, the most active complex Ir3 also exhibited relative stability in mammalian fluids and a significant antibacterial effect on clinically isolated drug-resistant bacteria. Mechanism studies further demonstrated that the complex Ir3 can kill S. aureus by disrupting the integrity of the bacterial membrane and inducing ROS production. This multi-target advantage allows Ir3 to not only effectively combat bacterial resistance but also efficiently clear the bacterial biofilm. In addition, when used together, complex Ir3 could enhance the antibacterial potency of some clinical antibiotics against S. aureus. Moreover, both G. mellonella wax worms and mouse infection model demonstrated that Ir3 has low toxicity and robust anti-infective efficacy in vivo. Overall, complex Ir3 can serve as a new antibacterial agent for combating Gram-positive bacterial infections.
1 Introduction
Multidrug-resistant bacterial infections have posed a growing global health threat. The resulting death toll is anticipated to exceed that of AIDS and cancer combined by the year 2050.1–3 As the most common Gram-positive pathogen, Staphylococcus aureus (S. aureus) colonizes in one-third of the world population and is one of the major pathogens to cause fatal infections.4 Furthermore, S. aureus has evolved to achieve antibiotic resistance owing to the abuse of antibiotics, which makes the problem worse. It was reported that the evolution of methicillin-resistant S. aureus (MRSA), which is also known as a “superbug”, has resulted in nearly 20
000 deaths in the United States each year.5 Worse yet, few drugs are available to fight against this notorious pathogen after it developed resistance. Therefore, it is urgent to develop novel drugs for combating this so-called superbug.
In recent decades, metal-based agents as potential anti-tumor drugs have received extensive attention and research.6 In addition, due to the unique photophysical and electrochemical properties, they were commonly used in biocatalysts and cell imaging to treat diseases, with a huge advantage to improve the accuracy of diagnosis and treatment.7–9 In fact, metal-based antibacterial agents have also received extensive attention and possess huge potential to address drug-resistant bacterial infections.10–14 It has been reported that iridium(III) complexes bearing polypyridine moieties showed obvious antibacterial activity against Gram-positive bacteria such as S. aureus.15–18 Meanwhile, ruthenium-based complexes, which could disrupt the integrity of the bacterial membrane, not only exhibited robust antibacterial efficacy against Gram-positive bacteria but also effectively blocked the generation of bacterial resistance.19
In this work, three iridium-based antibacterial agents capable of bacterial membrane destruction and reactive oxygen species induction were designed and prepared, aiming to screen a better antibacterial metallodrug to combat multidrug-resistant bacteria. Their antibacterial potencies against S. aureus were evaluated through minimum inhibitory concentration. Furthermore, the antibacterial mechanism was confirmed using scanning electron microscopy, bacterial staining assay, and the leakage of cytoplasmic materials analyses. Next, a series of assays, including the hemolytic toxin secretion, bacterial biofilm formation and clearance, checkerboard assay and drug resistance development assay, were further performed to confirm the advantage of complex Ir3 as a candidate metal-based antibiotic. At last, the G. mellonella wax worms and mouse infection model were employed to verify its toxicity and anti-infective efficacy in vivo.
2 Results and discussion
2.1 Synthesis, characterization and in vitro antibacterial activity against S. aureus
The synthesis routes of the three complexes are shown in Scheme 1.20–22 Three iridium complexes were isolated with hexafluorophosphate as the counter anion. The structures of all complexes were characterized by HRMS spectrometry, 1H-NMR, and 13C-NMR. For the HRMS spectra, all found signals of [M-PF6]+ were exactly the same as the theoretical values. In addition, the purity of the three complexes was confirmed by HPLC (ESI †).
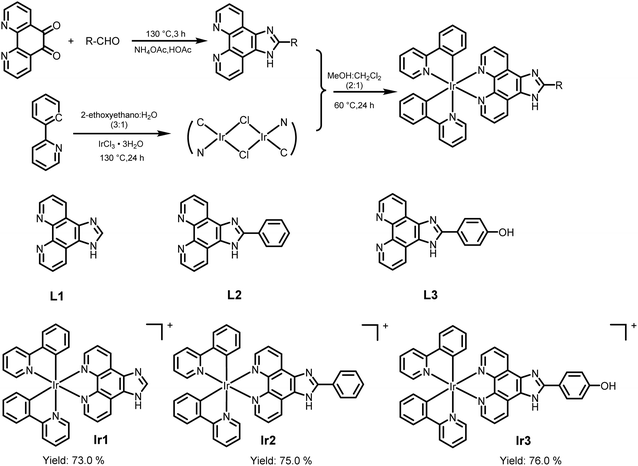 |
| Scheme 1 The synthetic route to the iridium-based complexes and the structures of three iridium complexes. | |
Firstly, the minimum inhibitory concentrations (MIC) of the three iridium-based agents against S. aureus were assessed using a broth microdilution assay. The MIC values of Ir1, Ir2 and Ir3 against S. aureus were 7.8 μg mL−1, 3.9 μg mL−1 and 1.9 μg mL−1, respectively (Fig. 1). Notably, the antimicrobial potency of complex Ir3, which works best, is comparable to that of vancomycin, a glycopeptide antibiotic that is used as the first-line drug for drug-resistant S. aureus. In addition, complex Ir3 exhibited robust effectiveness against drug-resistant S. aureus (MRSA) isolated from the clinic, with the MIC value at only 3.9 μg mL−1 (Table S1†).
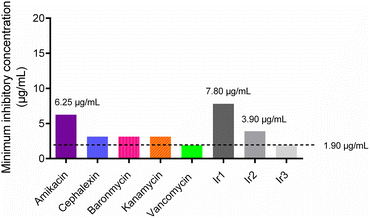 |
| Fig. 1 Minimum inhibitory concentration (MIC) values of some antibiotics and the three iridium complexes against the S. aureus Newman strain. | |
After entering the bloodstream, metal-based agents are easily decomposed by some enzymes, which limits their clinical applications to a great extent. Therefore, the stability of the three iridium complexes was evaluated after being incubated in plasma, serum and blood at different times.23,24 As showed in Fig. 2, the MIC values of Ir3 remained constant after being incubated in 50% serum even for 8 h. Furthermore, the MIC values of Ir3 only increased to 4 and 8 μg mL−1 after being incubated in blood or plasma, respectively. In contrast, the MIC values of Ir1 and Ir2 increased to 125 and 30 μg mL−1, respectively, under the same conditions. This result indicated that Ir3 provided the best antibacterial activity and biological stability, making it a suitable antibiotic candidate.
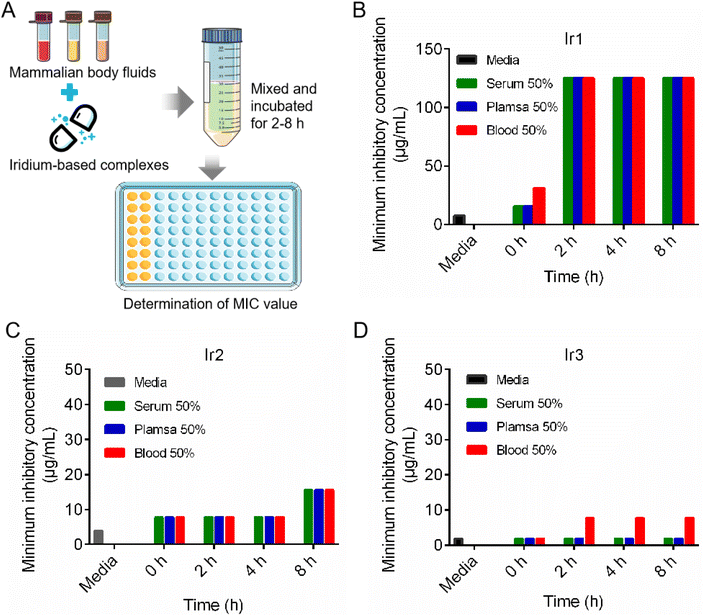 |
| Fig. 2 The scheme illustration of the stability evaluation of three iridium-based complexes in mammalian body fluids (A); the MIC changes of Ir1 (B), Ir2 (C) and Ir3 (D) after co-incubated with mammalian body fluids. | |
2.2 Antibacterial mechanism studies of Ir3
Encouraged by the excellent potency of Ir3 against S. aureus in vitro, we further investigated its antibacterial mechanisms using a series of assays. At first, two fluorescence probes, PI and DAPI, were employed to investigate the damage effect on the bacterial membrane. Generally, DAPI can stain all cells blue, while PI only stained damaged cells red.25 As shown in Fig. 3, after co-incubation with Ir3 or vancomycin at 1 × MIC concentration for 4 h, both red and blue fluorescence signals were observed, indicating that the membrane integrity of the bacteria was compromised. In contrast, only blue fluorescence was observed in the PBS-treated group. These results indicated that Ir3 might effectively destroy the integrity of the bacterial cell membrane.
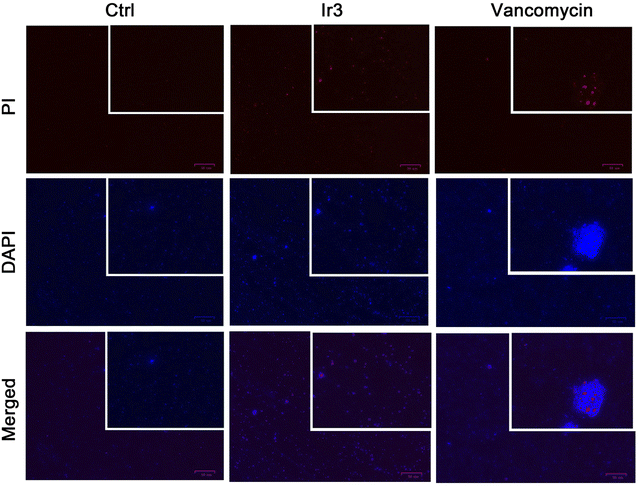 |
| Fig. 3 Fluorescence micrographs of S. aureus cells stained with DAPI/PI after co-incubation with Ir3 or vancomycin. | |
In general, physical destruction of the cell membrane would result in the leakage of intracellular proteins and nucleic acids. Next, the membrane-disruptive effect of complex Ir3 on S. aureus was further verified by testing the leakage of cytoplasmic materials (e.g., nucleic acid with absorption at 260 nm). As shown in Fig. 4A, the obvious release of nucleic acids from S. aureus was observed after co-incubation with complex Ir3. Notably, Ir3 caused more nucleic acid leakage than polymyxin B, a small lipopeptide membrane destabilizer. Similarly, complex Ir3 also resulted in the leakage of ortho-nitrophenyl-β-galactoside (ONPG), an intracellular enzyme which can produce O-nitrophenol with absorbance at 415 nm,26,27 under the same condition (Fig. 4B). These results further showed that Ir3 could significantly damage the S. aureus cytoplasmic membrane, leading to the leakage of cytoplasmic material. At last, the scanning electron microscope (SEM) assay was employed to directly monitor the morphological changes of the bacteria. As shown in Fig. 4C, the morphology of the bacterial cells in the untreated group exhibits a smooth and intact membrane. However, the bacterial membrane was obviously damaged and distorted into sharp angles after co-incubation with Ir3. Taken together, these results suggested that complex Ir3 can destroy the bacterial membrane, leading to bacterial death.
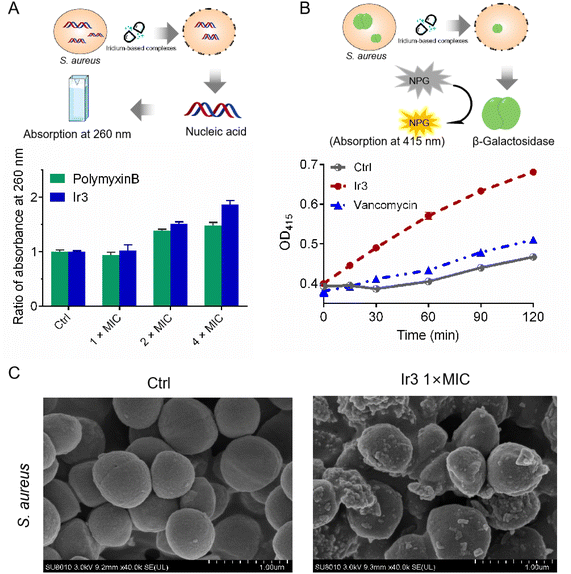 |
| Fig. 4 (A) Ratio of the absorbance at 260 nm of the supernatant in S. aureus suspensions after treatment with Ir3 or polymyxin B for 2 h at different concentrations. (B) The cytoplasmic membrane permeabilization of S. aureus cells treated with Ir3 or vancomycin. (C) SEM images of S. aureus treated with Ir3 at 1 × MIC. | |
As is well known, many metal-based agents can induce the production of reactive oxygen species (ROS), which then kill bacteria or tumor cells. For example, some ruthenium complexes can efficiently kill pathogens by generating ROS.28 Here, in order to verify whether Ir3 also can induce ROS generation in S. aureus, we monitored the intracellular ROS levels using DCFH-DA, a green fluorescent probe which specifically recognizes ROS in vivo. As shown in Fig. 5A, in the presence of Ir3, large amounts of ROS were detected in S. aureus, as indicated by the obvious green fluorescence. Next, we further investigated the MIC value changes of Ir3 in the presence of N-acetyl-L-cysteine (NAC), a commonly used ROS scavenger,29 aiming to confirm the important role of ROS in Ir3-killed S. aureus. As shown in Fig. 5B, the antibacterial potency of Ir3 against S. aureus was reduced by 16 times, with the MIC values increased from 1.95 to 31.25 μg mL−1, in the presence of NAC. This result suggested that Ir3 could cause S. aureus to produce ROS, which then helps Ir3 kill them. Overall, the above series of mechanism research experiments clearly showed that Ir3 has a multi-target antibacterial mechanism, killing S. aureus by disrupting the integrity of the bacterial membrane and inducing ROS production.
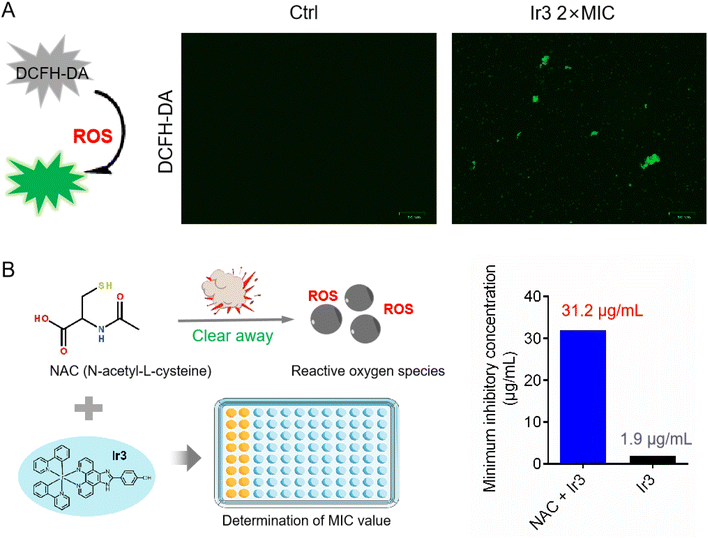 |
| Fig. 5 (A) Minimum inhibitory concentration of Ir3 against S. aureus in the presence or absence of NAC. (B) Intracellular ROS production was detected by 2,7-dichlorofluorescein diacetate (DCFH-DA), and then photographed by fluorescence microscope. | |
2.3 Ir3 inhibited biofilm formation and eradicated the established biofilm
In vitro antibacterial activity and mechanism studies showed that complex Ir3 has robust potency and a multi-target antibacterial mechanism. We believe this advantage must be extremely beneficial in fighting bacterial infections. As is well known, the bacterial biofilm is an important protective barrier for them against antibiotics.30 At first, in order to identify whether Ir3 can efficiently inhibit the formation of the S. aureus biofilm, a crystal violet staining assay was employed. Briefly, S. aureus bacteria were co-incubated with Ir3 (0.25, 0.5 or 0.75 × MIC) at 37 °C for 48 h, then the biofilm was stained and quantified. As shown in Fig. 6A, the ability of the bacteria to produce biofilms was significantly disrupted in the presence of Ir3. The biofilm formation was significantly reduced by 17.4, 35.3 and 65.4% after exposure to 0.5, 1.0 and 1.5 μg mL−1 of Ir3, respectively. Once the biofilm forms, bacteria can hide in it and then evade antibiotic attacks. Therefore, the ability of an antibacterial agent to eradicate the established biofilms is very important in its complete removal of bacteria from the site of infection.31 Subsequently, the biofilm eradication potency of Ir3 was investigated by monitoring the number of viable bacteria in matured biofilms after being co-incubated with it. As shown in Fig. 6B, only at 2 × MIC, Ir3 reduced the number of viable bacteria, those hiding within the matured biofilm, by 22.2%. When the concentration of Ir3 reached 4 × MIC, an 83.3% decrease of viable bacteria in the biofilm was observed. Taken together, these results indicated that complex Ir3 could not only inhibit biofilm formation of S. aureus, but also eradicated the established biofilm.
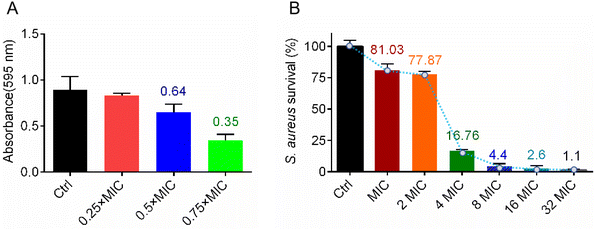 |
| Fig. 6 (A) The formation of a S. aureus biofilm in the presence of Ir3; the biofilm was stained with crystal violet and quantified by monitoring the absorbance at 595 nm. (B) The number of viable bacteria in a matured biofilm after co-incubation with Ir3; all data are shown as mean ± sd. | |
2.4 Bacterial resistance study
One of the main drawbacks of traditional clinical antibiotics is that bacteria can easily develop resistance to them after repeated use, which poses a major challenge for the treatment of infectious diseases.32 Since complex Ir3 has the advantage of a multi-target mechanism and clearing matured biofilm, this should be helpful to combat bacterial resistance. Subsequently, in order to confirm this hypothesis, the repeated use of Ir3 against S. aureus was performed to induce bacterial resistance development. As showed in Fig. 7, complex Ir3 did not induce any resistance in S. aureus even after 20 passages, while the MIC value of ampicillin, one of the most commonly used antibiotics in the clinic, increased by 1024 times under the same conditions. This result undoubtedly confirmed the ability of Ir3 in fighting against the rapid development of resistance in bacteria.
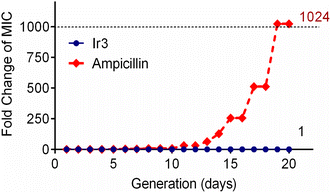 |
| Fig. 7 Drug-resistant development profile of S. aureus exposed to Ir3 and the clinically used antibiotic ampicillin. | |
2.5 Ir3 increases the susceptibility of S. aureus to antibiotics
As an alternative approach, in order to mitigate antibiotic resistance development, the use of antimicrobial adjuvants to increase antibiotic potency and reverse drug resistance has received much attention in recent years.33 Next, a checkerboard assay was performed,28 aiming to investigate whether Ir3 can enhance the potency of the clinically used antibiotic. Here, we selected five different classes of antibiotics, including aminoglycoside antibiotic (gentamicin), macrolide antibiotics (tetracycline), polypeptide antibiotics (polymyxin B), glycopeptide antibiotics (vancomycin) and cephalosporins antibiotics (cefalexin). At first, the heat plots of the five checkerboard assays were drawn (Fig. 8A). Next, the fractional inhibitory concentration index (FICI), a key data to evaluate synergism, was calculated and an isobologram analysis of the synergistic effects was plotted (Fig. 8F and G). Generally, FICI ≤ 0.5 clearly suggests synergism. Our results confirmed that Ir3 could efficiently improve the antibacterial potency of gentamicin (FICI = 0.3125), tetracycline (FICI = 0.5) and polymyxin B (FICI = 0.3125) against S. aureus. This result indicated that Ir3 had the potential as an antimicrobial adjuvant.
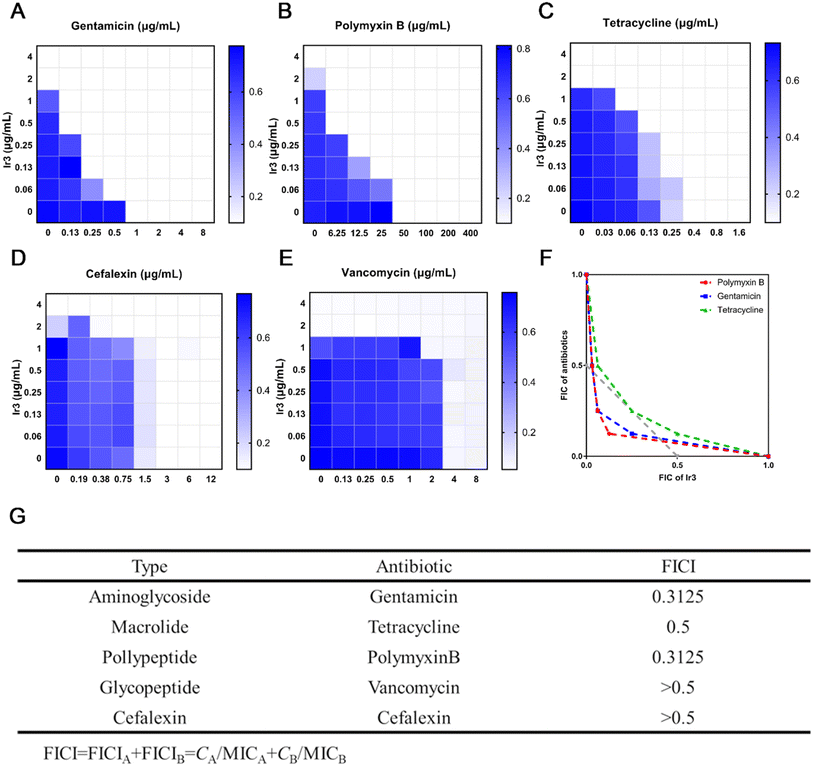 |
| Fig. 8 Heat plots of checkerboard assays for Ir3 after combined use with some antibiotics against S. aureus (A–E). An isobologram analysis of the synergistic effects of Ir3 with gentamicin, tetracycline and polymyxin B (F). Fractional inhibitory concentration indices (FICI) of antibiotics when used in combination with Ir3 against S. aureus (G). | |
2.6 Ir3 can inhibit the production of hemolytic toxin of S. aureus
As an important bacterial exotoxin, the α-hemolysin of S. aureus plays an important role in its ability to cause serious infections.34 Therefore, preventing the secretion of the hemolytic toxin from S. aureus is very beneficial to speed up the cure of infectious diseases. Here, to identify whether Ir3 could inhibit the secretion of α-hemolysin from S. aureus, the amount of α-hemolysin was quantitatively analyzed by a rabbit erythrocyte lysis test. As shown in Fig. 9, upon co-incubation with 0.5 or 1 μg mL−1 of Ir3, the amount of α-hemolysin secretion in the bacterial culture supernatant significantly decreased by 25.4% and 99.9%, respectively. Thus, our results presented here clearly suggested that Ir3 could efficiently inhibit the toxin production. Especially at 0.5 × MIC, the secretion of α-toxins is almost completely suppressed.
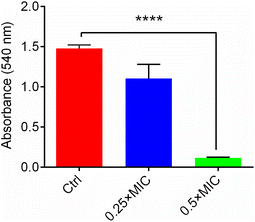 |
| Fig. 9 The α-hemolysin secretion in S. aureus; the results are shown as mean ± sd. | |
2.7 The toxicity of complex Ir3
A potential antibacterial drug should not only have robust potency, but also exhibit low toxicity to mammalian cells. This is a key prerequisite for entering clinical research. Therefore, we further explored the toxicity of complex Ir3 in vitro and in vivo. Firstly, rabbit erythrocytes were used to evaluate the biocompatibility of Ir3 in vitro. In brief, different concentrations of Ir3 were incubated with rabbit erythrocytes at 37 °C. The PBS and 0.1% Triton-X treated samples were used as the negative and positive controls, respectively. At 2 μg mL−1 (1 × MIC), Ir3 did not damage any red blood cells. Even at 400 μg mL−1 (100 × MIC), the percentage of ruptured red blood cells was only 45.0% in the presence of Ir3, indicating that complex Ir3 has good blood biocompatibility (Fig. S1†). Next, to further explore the toxicity of Ir3 in vivo, G. mellonella, an insect widely used for toxicity screening due to its significant similarity of the physiology and immune systems to those of mammals, was employed. As shown in Fig. 10, Ir3 exhibited very low toxicity to G. mellonella at doses of 2 to 256 mg kg−1. Even at a high dose (256 mg kg−1), Ir3 did not cause more deaths than the PBS-treated group. Taken together, our results indicated that Ir3 has low cytotoxicity and good biocompatibility.
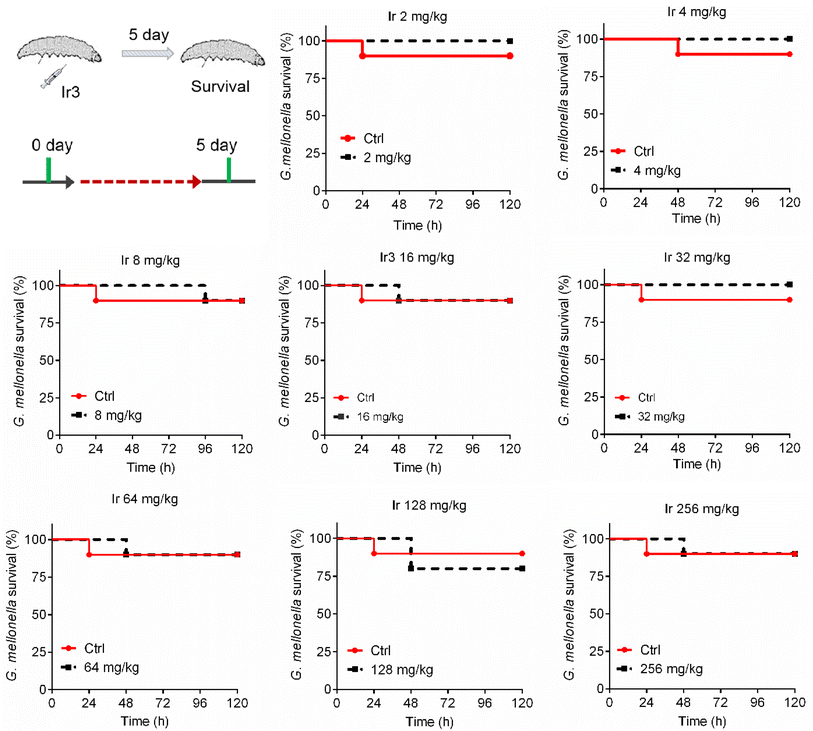 |
| Fig. 10 The survival rate of G. mellonella wax worms after treatment with Ir3 at a dose range of 8 to 256 mg kg−1. | |
2.8 The therapy efficacy of Ir3 against S. aureus-induced infection
Due to Ir3 presenting excellent antibacterial activity against S. aureus in vitro and its low toxicity, we further established two animal infection models in order to evaluate its antibacterial efficacy in vivo. At first, G. mellonella was used again. In brief, a bacterial suspension was injected into the right front gastropoda of G. mellonella larva. After 1 hour, Ir3 or a commonly used clinical antibiotic (ampicillin) was injected into the left front gastropoda of the larva, and then their therapeutic effects were evaluated by recording the survival rate of the infected G. mellonella within 5 days. As shown in Fig. 11, the infected G. mellonella all survived after treatment with 40 mg kg−1 Ir3 or ampicillin. Meanwhile, at a dose of 20 mg kg−1, the survival rate of the infected G. mellonella treated with Ir3 was obviously higher than that with ampicillin, indicating that Ir3 exhibited a better therapy efficacy than ampicillin, at least in the case of 20 mg kg−1 dose.
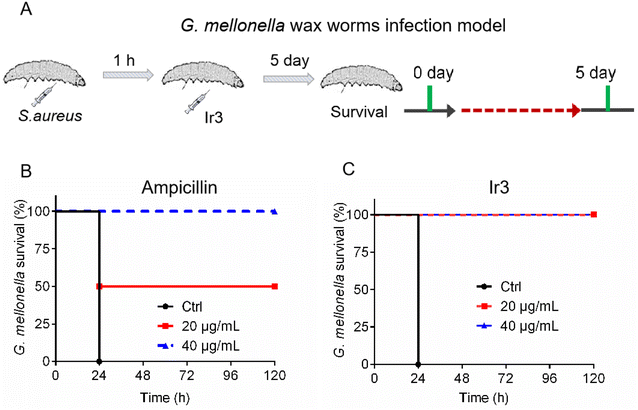 |
| Fig. 11 (A) Schematic illustration of the experimental protocol for the G. mellonella larva infection model. The survival rate of S. aureus-infected G. mellonella wax worms after treatment with Ir3 (B) or ampicillin (C). | |
Next, a model of S. aureus-infected dermal wound was established on a mouse model to further investigate Ir3's in vivo potency and infected-wound healing application. Briefly, the wound-infected mouse was randomly divided into 4 groups (n = 5), and then the S. aureus-infected dermal wounds were treated with a sterile cream containing Ir3 or ampicillin. During the therapy course, the wound healing size of the mice was calculated and the mice were weighed. At last, the number of viable bacteria in the infected-wound was monitored. As shown in Fig. 12, after being treated for 10 days, marked differences in the cutaneous wound size were observed between the Ir3, ampicillin and PBS groups. Both Ir3 and ampicillin significantly accelerated the healing of the wound, and the smaller wound resulting from Ir3 suggested it has a better therapeutic effect than ampicillin at the same dose. In addition, at the dose of 150 μg mL−1, the number of viable bacteria in the infected-wound of the mouse model in the Ir3 group is less than that in the ampicillin group. It is worth noting that the weight of all of the mice did not change significantly during the treatment, again suggesting the low toxicity of Ir3. All in all, these results suggested that Ir3 has excellent therapy efficacy against S. aureus-induced infections as well.
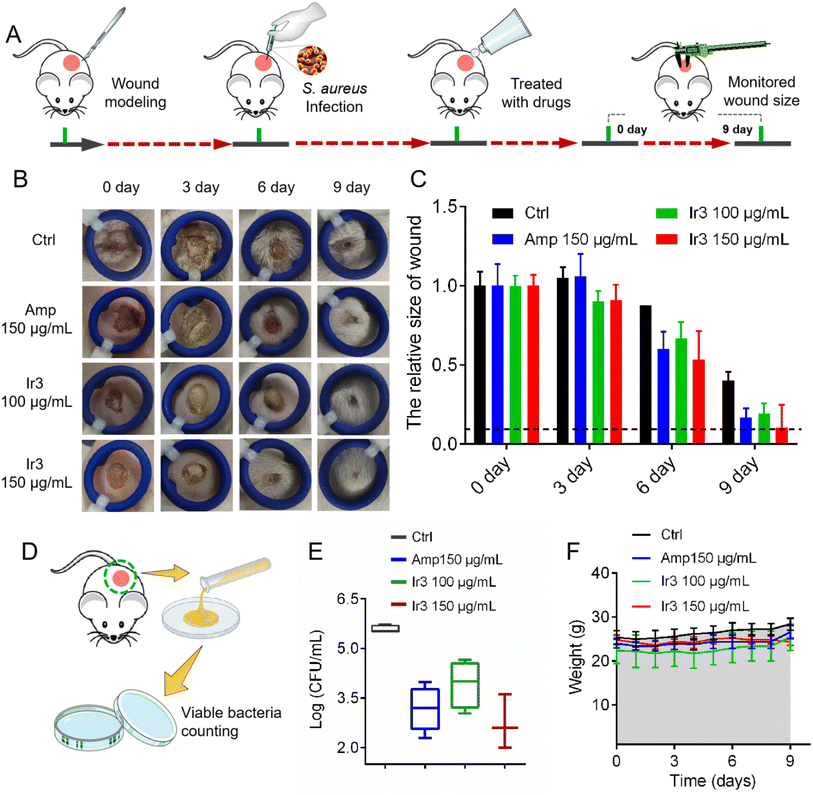 |
| Fig. 12 (A) Schematic illustration of the experimental protocol for the mouse S. aureus-infected dermal wound model. (B) Representative photographs of cutaneous abscesses after treatment with Ir3 or an ampicillin-containing ointment. (C) The relative size changes of skin ulcers over 9 days. (D) Schematic illustration of the experimental protocol for monitoring the viable bacteria in the mouse wound. (E) The number of viable bacteria in the mouse wound. (F) The relative weight changes of the mouse model over 9 days. The data are shown as mean ± sd. | |
3 Conclusion
In the face of a severe drug resistance situation, the development of new multi-target drugs with excellent activity that can hinder bacterial resistance is urgently needed. In this work, a series of iridium-based antibacterial agents were synthesized and evaluated. Importantly, complex Ir3 was identified to be the most active one, which not only showed robust potency against S. aureus with a MIC value that only lies at 1.9 μg mL−1, but also exhibited relative stability in mammalian fluids. Furthermore, using a series of assays, we demonstrated that Ir3 has multiple targets in killing S. aureus. It killed bacteria mainly through disrupting the integrity of the bacterial membrane and inducing ROS production. Due to its multi-target mechanism, Ir3 could efficiently avoid the development of bacterial resistance. The MIC values of Ir3 against S. aureus did not change after being used for 20 passages. It is worth noting that Ir3 also inhibited the biofilm formation of S. aureus, eradicated the established biofilm, efficiently suppressed the secretion of α-toxins, and showed potential as an antimicrobial adjuvant as it can improve the antibacterial potency of clinical antibiotics. This series of advantages over traditional antibiotics make it a potential new metal-based antibacterial drug. In addition, using G. mellonella wax worms and mouse infection model, we have demonstrated that complex Ir3 showed low toxicity and had excellent therapy efficacy against S. aureus-induced infection as well. Overall, our work presented here clearly suggested that iridium-based antibacterial agents possessed excellent antibacterial activity, and can serve as a novel antibacterial agents for combating Gram-positive bacterial infections.
4 Experimental section
4.1 Minimum inhibitory concentration (MIC)
Briefly, S. aureus cells were diluted to 1 × 106 CFU mL−1 with fresh TSB medium, and then transferred to a 96-well micro plate. Then, different concentrations of tested drugs were added, and the plate was incubated at 37 °C for 20 h to determinate the MIC values.
4.2 Drug resistance develop assay
The minimum inhibitory concentration (MIC) was employed to evaluate the development of bacterial resistance after exposure to Ir3 or ampicillin. Bacterial cells under 0.5 × MIC of the tested compound were employed to make a new S. aureus suspension for the next assay. Every passage was incubated for 20 hours with shaking at 220 rpm. The process was repeated for 20 passages and the MIC values were recorded.
4.3 Hemolytic activity study
S. aureus suspensions were diluted to 1 × 106 CFU mL−1, and then different concentrations of Ir3 were added. After being incubated with shaking at 37 °C for 10 h, the supernatant was obtained through centrifugation. The fresh sterile rabbit blood was centrifuged at 2000 rpm for 2 min, and washed with PBS 3 times to obtain pure red blood cells. The bacterial supernatant (200 μL) and red blood cells (50 μL) were added into 1 mL PBS buffer. The mixture was incubated at 37 °C for 30 min, then centrifuged at 2000 rpm for 2 min. At last, the hemolytic activity was determined through the absorption at 540 nm.
4.4 Bacterial biofilm inhibition assay
A 2 mL volume of 1 × 106 CFU mL−1 S. aureus suspension was added to a 24-well polyphenylene plate, followed by different concentrations of Ir3. After being incubated at 37 °C for 48 h, the planktonic bacteria were removed and then washed with PBS 3 times. The plate was dried out at room temperature. Then, 0.1% crystal violet solution was used to stain the bacterial biofilm for 15 min, and washed with PBS 3 times. Afterwards, acetic acid (50%) was used to dissolve the crystal violet attached to the biofilm. Finally, the absorbance values at 595 nm were detected.
4.5 Bacterial biofilm disruption assay
A 200 μL volume of 1 × 106 CFU mL−1 S. aureus suspension was added into a 96-well micro plate. After being incubated at 37 °C for 48 h, the planktonic bacteria were removed and then washed with PBS 3 times. Different concentrations of Ir3 were added, and then the plate was incubated at 37 °C for 4 h. Next, the biofilm was washed with PBS 3 times, and then 200 μL PBS was added into the 96-well micro plate. Afterwards, ultrasonication was used to treat the 96-well micro plate with the attached biofilm, making it fall off. Subsequently, 50 μL suspensions were plated on a TSB agar plate to quantity the number of viable bacteria.
4.6 Checkerboard assay
S. aureus cells were diluted to 1 × 106 CFU mL−1 with TSB medium, and transferred into a 96-well micro plate. After adding the diluted bacterial suspension in the presence of the mixtures of Ir3 and the antibiotics (Ir3 and antibiotics were continuously diluted by 2-fold dilution method), the plate containing the mixtures was incubated at 37 °C for 20 h to determine the MIC of Ir3 and the antibiotics.
4.7 DAPI/PI fluorescence staining
The S. aureus suspension was centrifuged at 5000 rpm for 2 min, and washed with PBS 3 times. The bacterial cell concentration was further diluted to OD600 = 0.4 with PBS, and then Ir3 was added. The suspension was incubated with shaking at 37 °C for 4 h. Afterwards, 30 μL DAPI (10 μM) or 30 μL PI (10 μM) were added into the bacterial suspension, and then further incubated at room temperature in the dark for 30 min. Finally, the fluorescence of the bacteria was monitored using a fluorescence microscope.
4.8 Intracellular ROS assay
Bacterial suspensions with OD600 = 0.4 were prepared. Ir3 was added into the bacterial suspensions with shaking at 220 rpm at 37 °C for 2 h. Afterwards, with a constant volume of 5 mL with PBS, the oxygen fluorescent probe 2,7-dichlorodihydrofluorescein diacetate (DCFH-DA) was used to identify the bacteria ROS level. In brief, DCFH-DA (10 μL, 30 μM) was added into the bacterial suspension for 30 min under dark conditions. Then, the fluorescence of the bacteria was monitored using a fluorescence microscope.
4.9 Membrane permeability assay
S. aureus cells were diluted with M9 minimal medium, and then incubated at 37 °C for 8 h with lactose as the sole carbon source. Next, S. aureus cells were centrifuged at 5000 rpm for 2 min and washed with PBS 3 times. The bacterial cell concentration was diluted to OD600 = 0.3 with PBS, and 6 mL of the bacterial suspension was added into a test tube, followed by the addition of 300 μL ONPG (1.2 mM) to each well. Then, 10 μL of Ir3 and vancomycin were added to the test tubes and incubated with gentle shaking at 37 °C for 2 h. The hydrolysis of ONPG to O-nitrophenol over time was monitored at 415 nm with a micro plate reader.
4.10 Scanning electron microscope
S. aureus suspensions were centrifuged at 5000 rpm for 2 min and washed with PBS 3 times. Bacterial cells were diluted to OD600 = 0.4 with PBS. Then, Ir3 was added and further incubated with shaking at 37 °C for 2 h. After being centrifuged for 2 min at 5000 rpm, the cells were washed with PBS 3 times. Next, bacterial cells were fixed with 2.5% glutaraldehyde overnight and dehydrated with tertbutanol. Afterwards, the surface morphology of the bacterial cell membranes was observed.
4.11 In vivo toxicity of the Ir3
Rabbit erythrocytes were used to evaluate the bio compatibility of Ir3 in vitro. In brief, different concentrations of Ir3 were added into each well containing rabbit erythrocytes. The PBS and 0.1% Triton-X treated rabbit erythrocytes were used as the negative and positive controls, respectively. The hemolytic activity was determined through the absorbance value at 540 nm. Next, to further explore the in vivo toxicity of Ir3, G. mellonella was used. Prior to injection, the site was sterilized with ethanol. Then, 10 μL Ir3 was injected into the right front gastropoda of the larva. Afterwards, the survival rate of G. mellonella was observed within 5 days.
4.12Mouse Infection Model
All mouse infection models were purchased from Nanjing Kerusi Animal Co., LTD. The mouse infection models were divided into four groups (5 mice in each group), then wounds were generated on the backs of the mice. Next, S. aureus suspensions were diluted to OD600 = 1 with PBS, and the wounds on the mice were injected with the S. aureus suspension. After 24 hours of infection, the ointments containing Ir3 or ampicillin were softly smeared on the abscess, and the size of the wounds was measured over times. Thereafter, the mice were weighed. Nine days later, the tissues were collects and the viable bacteria were counted by the surface plating method.
4.13G. mellonella Experiment
S. aureus suspensions were centrifuged at 5000 rpm for 2 min and washed with PBS 3 times. Next, bacterial cells were diluted to the absorbance of OD600 = 0.3 with PBS. Prior to injection, G. mellonella was sterilized with ethanol. A 5 μL volume of S. aureus bacterial suspension was injected into the right front gastropoda of the larva. After 1 hour, a different dose of Ir3 or ampicillin was injected into the left front gastropoda of the larva. At last, the survival rate of G. mellonella was observed within 5 days.
Ethical statement
The animal study was reviewed and approved by the Animal Care and Use Committee of Jiangxi Science&Technology Normal University, and complied with the academic guidelines for the care and use of laboratory animals.
Author contributions
Shijie Lin: data curation, investigation, writing. Yushou Chen: formal analysis, investigation, writing. Yajuan Sun: investigation. Guangying Yu: formal analysis, methodology. Xiangwen Liao: funding acquisition, project administration, supervision. Qiang Yang: funding acquisition, project administration, supervision.
Conflicts of interest
The authors have declared that there is no conflict of interest.
Acknowledgements
We gratefully acknowledge the generous support provided by the Health Research Project of Hainan Province (Grant No. 22A200010) and Jiangxi Science & Technology Normal University (2021QNBJRC001).
References
- Q. Kong, G. Li, F. Zhang, T. Yu, X. Chen, Q. Jiang and Y. Wang, J. Med. Chem., 2022, 65, 11309–11321 CrossRef CAS PubMed.
- A. R. Collaborators, Lancet, 2022, 399, 629–655 CrossRef PubMed.
- H. Fongang, A. T. Mbaveng and V. Kuete, Adv. Bot. Res., 2023, 106, 1–20 CAS.
- Y. Jiang, M. Han, Y. Bo, Y. Feng, W. Li, J. R. Wu, Z. Song, Z. Zhao, Z. Tan, Y. Chen, T. Xue, Z. Fu, S. H. Kuo, G. W. Lau, E. Luijten and J. Cheng, ACS Cent. Sci., 2020, 6, 2267–2276 CrossRef CAS PubMed.
- H. Y. Chow, K. H. L. Po, P. Gao, P. Blasco, X. Wang, C. Li, L. Ye, K. Jin, K. Chen, E. W. C. Chan, X. You, R. Yi Tsun Kao, S. Chen and X. Li, J. Med. Chem., 2020, 63, 3161–3171 CrossRef CAS PubMed.
- C. Licona, J. B. Delhorme, G. Riegel, V. Vidimar, R. Cerón-Camacho, B. Boff, A. Venkatasamy, C. Tomasetto, P. Gomes, D. Rognan, J. N. Freund, R. Lagadec, M. Pfeffer, I. Gross, G. Mellitzer and C. Gaiddon, Inorg. Chem. Front., 2020, 7, 678–688 RSC.
- E. Baggaley, J. A. Weinstein and J. A. G. Williams, Coord. Chem. Rev., 2012, 256, 1762–1785 CrossRef CAS.
- A. Pothig and A. Casini, Theranostics, 2019, 9, 3150–3169 CrossRef PubMed.
- B. M. Zeglis, V. C. Pierre and J. K. Barton, Chem. Commun., 2007, 44, 4565–4579 RSC.
- E. A. Kukushkina, S. I. Hossain, M. C. Sportelli, N. Ditaranto, R. A. Picca and N. Cioffi, Nanomaterials, 2021, 11, 1687 CrossRef CAS PubMed.
- A. Pathak, V. Blair, R. Ferrero, L. Kedzierski and P. Andrews, J. Inorg. Biochem., 2017, 177, 266–275 CrossRef CAS PubMed.
- P. Arthi, M. Dharmasivam, B. Kaya and A. K. Rahiman, Chem.-Biol. Interact., 2023, 373, 110349 CrossRef CAS PubMed.
- A. Gupta, P. Prasad, S. Gupta and P. K. Sasmal, ACS Appl. Mater. Interfaces, 2020, 12, 35967–35976 CrossRef CAS PubMed.
- A. Frei, A. Verderosa, A. Elliott, J. Zuegg and M. Blaskovich, Nat. Rev. Chem, 2023, 7, 202–224 CrossRef CAS PubMed.
- C. M. Bernier, C. M. DuChane, J. S. Martinez, J. O. Falkinham and J. S. Merola, Organometallics, 2021, 40, 670–1681 CrossRef.
- B. F. Hohlfeld, B. Gitter, C. J. Kingsbury, K. J. Flanagan, D. Steen, G. D. Wieland, N. Kulak, M. O. Senge and A. Wiehe, Chemistry, 2021, 27, 6440–6459 CrossRef CAS PubMed.
- N. Kavitha, V. Thamilarasan and N. Sengottuvelan, J. Organomet. Chem., 2021, 952, 122032 CrossRef CAS.
- L. Lu, L. Liu, W. Chao, H. Zhong, M. Wang, X. Chen, J. Lu, R. Li, D. Ma and C. Leung, Sci. Rep., 2015, 5, 14544 CrossRef CAS PubMed.
- L. Wang, L. Liu, X. Wang, Y. Tan, X. Duan, C. Zhang, J. Cheng, Y. Xiong, G. Jiang, J. Wang and X. Liao, Eur. J. Med. Chem., 2022, 238, 114485 CrossRef CAS PubMed.
- W. Zhang, F. Du, M. He, L. Bai, Y. Gu, L. Yang and Y. Liu, Eur. J. Med. Chem., 2019, 178, 390–400 CrossRef CAS PubMed.
- J. Wang, X. Hou, H. Bo and Q. Chen, Inorg. Chem. Commun., 2015, 61, 31–34 CrossRef CAS.
- L. He, M. F. Zhang, Z. Pan, K. Wang, Z. Zhao, Y. Li and Z. Mao, Chem. Commun., 2019, 55, 10472–10475 RSC.
- H. Li, Y. Li, Y. Wang, L. Liu, H. Dong and T. Satoh, Acta Biomater., 2022, 142, 136–148 CrossRef CAS PubMed.
- H. Li, S. Fu, L. Liu, X. Yuan, Y. Wang, C. Zhang, H. Dong and T. Satoh, Eur. J. Med. Chem., 2022, 228, 113977 CrossRef CAS PubMed.
- Y. Guo, E. Hou, T. Wen, X. Yan, M. Han, L. P. Bai, X. Fu, J. Liu and S. Qin, J. Med. Chem., 2021, 64, 12903–12916 CrossRef CAS PubMed.
- L. Chopra, G. Singh, K. Kumar Jena and D. K. Sahoo, Sci. Rep., 2015, 5, 13412 CrossRef CAS PubMed.
- R. Kuppala, M. Govindarajan, R. Tambat, N. Patel, H. Nandanwar, K. Bhutani and K. Kartha, RSC Adv., 2016, 6, 3700–3713 RSC.
- C. Zhang, R. Yu, L. Wang, H. Huang, J. Wang, X. Liao, X. Duan and Y. Xiong, Eur. J. Med. Chem., 2022, 240, 114562 CrossRef CAS PubMed.
- A. I. Rodriguez-Rosado, E. Y. Valencia, A. Rodriguez-Rojas, C. Costas, R. S. Galhardo, J. Rodriguez-Beltran and J. Blazquez, J. Antimicrob. Chemother., 2019, 74, 2188–2196 CrossRef CAS PubMed.
- F. Chen, J. Moat, D. McFeely, G. Clarkson, I. J. Hands-Portman, J. P. Furner-Pardoe, F. Harrison, C. G. Dowson and P. J. Sadler, J. Med. Chem., 2018, 61, 7330–7344 CrossRef CAS PubMed.
- W. Chang, J. Liu, M. Zhang, H. Shi, S. Zheng, X. Jin, Y. Gao, S. Wang, A. Ji and H. Lou, Nat. Commun., 2018, 9, 5102 CrossRef PubMed.
- K. O'Connell, J. Hodgkinson, H. Sore, M. Welch, G. Salmond and D. Spring, Angew. Chem., Int. Ed., 2013, 52, 10706–10733 CrossRef PubMed.
- X. Ding, C. Yang, W. Moreira, P. Yuan, B. Periaswamy, P. de Sessions, H. Zhao, J. Tan, A. Lee, K. X. Ong, N. Park, Z. C. Liang, J. Hedrick and Y. Yang, Adv. Sci., 2020, 7, 2001374 CrossRef CAS PubMed.
- A. Steinhuber, R. Landmann, C. Goerke, C. Wolz and U. Fluckiger, Int. J. Med. Microbiol., 2008, 298, 599–605 CrossRef CAS PubMed.
|
This journal is © The Royal Society of Chemistry 2024 |