DOI:
10.1039/D4RA00555D
(Review Article)
RSC Adv., 2024,
14, 10331-10347
Electrochemical sensing of B-complex vitamins: current challenges and future prospects with microfluidic integration
Received
22nd January 2024
, Accepted 9th March 2024
First published on 28th March 2024
Abstract
Vitamins are crucial micronutrients found in limited quantities in food, living organisms, and soil. Since most vitamins are not produced within the human body, a lack of these essential nutrients can result in various physiological disorders. Analyzing vitamins typically involves costly, time-consuming methods, requiring skilled personnel, automated equipment, and dedicated laboratory setups. The pressing need is for the development of efficient, portable, and user-friendly detection techniques that are cost-effective, addressing the challenges associated with traditional analytical approaches. In recent years, electrochemical sensors and electrochemical microfluidic devices have garnered prominence owing to their remarkable sensitivity, quick analysis, cost-effectiveness, and facile fabrication procedures. Electrochemical sensing and microfluidics are two distinct fields that are often integrated to create powerful and versatile sensing devices. The connection between them leverages the advantages of both fields to create highly efficient, miniaturized, and portable analytical systems. This interdisciplinary approach has led to the development of innovative devices with broad applications in various scientific, medical, and environmental domains. This review begins by outlining the importance of vitamins in human nutrition and health and emphasizing the need for precise and reliable sensing techniques. Owing to the limited literature available on electrochemical detection of vitamin B complexes, this review offers an in-depth analysis of modern electrochemical sensing of water-soluble vitamins, focusing on B1, B2, B6, B9, and B12. The challenges faced by researchers are addressed, including selectivity, sensitivity, interference, matrix effects, and calibration, while also exploring promising prospects such as nanomaterial integration, miniaturization, microfluidics-based IoTs, and innovative sensor designs.
1 Introduction
The study of nutritional requirements produced notable findings at the turn of the 20th century. Vital amines, sometimes called “vitamins” at the time, have been described.1 Vitamins are a substantial class of organic compounds that are present in natural foods and are required in sufficient amounts in the diet due to their wide range of biochemical functions.2 They are crucial for many vital bodily functions, such as metabolism, maintaining blood sugar levels, and cell growth control. Vitamins are also useful in activating enzymes, which enables them to carry out a variety of enzyme-catalyzed biochemical reactions in the human body.3 Vitamin deficiencies in people frequently result in common illnesses like Parkinson's and Alzheimer's disease, leukemia, hypoglycemia, and cutaneous (skin rashes, alopecia, and conjunctivitis) conditions.4
Vitamin sensing can serve as an essential component for purposes including nutritional evaluation, the diagnosis and management of diseases, personalized nutritional interventions, large-scale public health initiatives, and facilitating research and development efforts. The instability and complexity of the substrates in which they are typically found make it very challenging to determine the presence of water-soluble vitamins. Various methods, as mentioned in Table 2, have been utilized to measure vitamins in various samples but face a number of limitations, including extensive analysis times, complex sample preparation, expensive equipment, and skilled personnel. Hence, in recent years, there has been a lot of interest in their quick and precise identification in various samples, as can be seen in Table 3.
Considerable efforts have been made for the development of vitamin sensing methods; researchers encounter numerous challenges in their pursuit. These difficulties include the sample matrix's complexities, the choice of suitable electrode materials, fouling problems, issues about the sensitivity and repeatability of developed techniques, and the simultaneous detection of the vitamins. It is imperative that these obstacles are carefully addressed to advance the field of vitamin sensing.
Nanotechnology has led to substantial advancements in the domains of electrochemical sensing and microfluidics, ushering in groundbreaking possibilities for precise and sensitive detection. This progress is primarily attributed to the widespread integration of nanomaterials such as carbon nanotubes, graphene, and metal nanoparticles into electrochemical sensors and microfluidic devices. Because these nanomaterials provide an increased surface area, they consequently greatly improve electrode modification and increase electrocatalytic activity. This, in turn, empowers the detection of analytes at even lower concentrations.5 The combination of electrochemical sensors with microfluidics is particularly beneficial for point-of-care applications. The resulting devices can be used for rapid and on-site detection of various analytes, ranging from medical diagnostics to environmental monitoring and food safety. Hence, integrating electrochemical sensors directly onto microfluidic chips eliminates the need for external instrumentation, making the entire system more compact and portable.
Though an additional significant challenge that has been overlooked or devalued involves the responsible integration of innovative nanomaterials and assemblies into sensor devices, they generate data that must be processed and decoded, making it a dynamic part of research in the sensing field. In order to provide the final assessment to the end user in real time, the data must be analyzed and converted by means of tools like deep learning, artificial intelligence, etc. to cope with big data. Their integration should be accessible for the manufacturing of commercial devices; otherwise, their use will be restricted to lab-scale experiments with very narrow socio-economic impacts.6
1.1 An overview of vitamins
The structure, chemistry, biological characteristics, and solubility of vitamins vary. The primary categorization of vitamins is based on solubility, as vitamins A, D, E, and K are soluble in fat while vitamins B and C are soluble in water.7 The types of vitamins and their sources are specified in Fig. 1, and their 3D structural representations are given in Fig. 2.
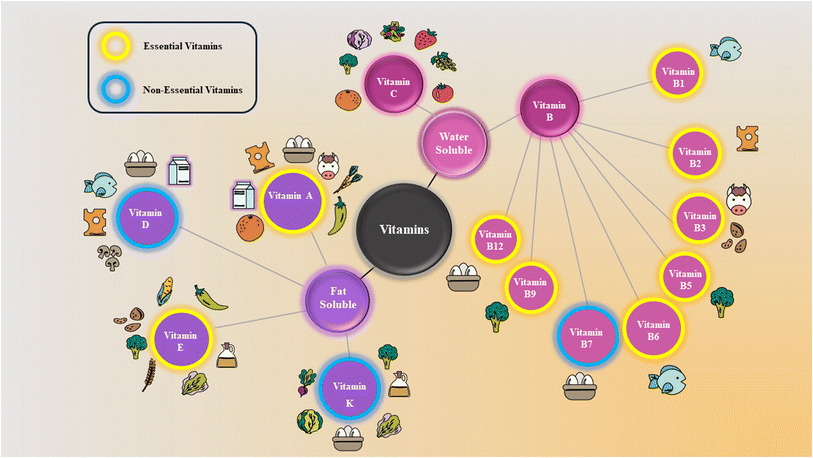 |
| Fig. 1 Types of vitamins and their sources. | |
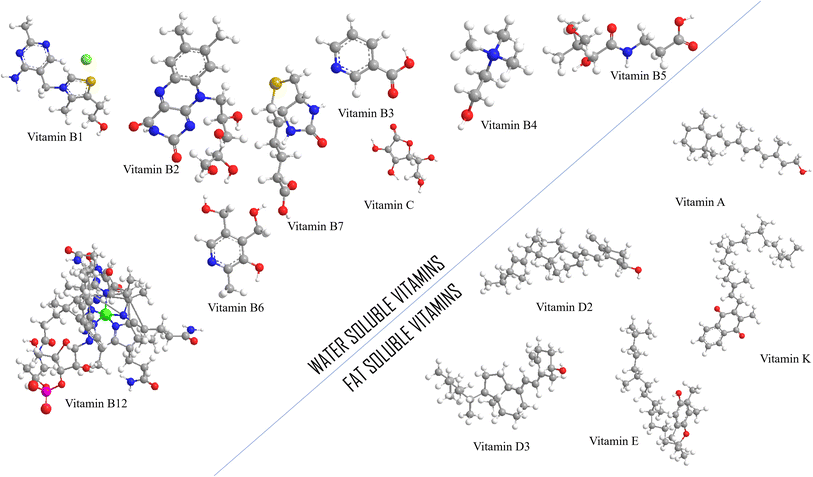 |
| Fig. 2 3D structural representations of vitamins (white = hydrogen, gray = carbon, red = oxygen, blue = nitrogen, brown = sulfur, green = cobalt). | |
The water-soluble vitamins that are the subject of this review include thiamine, riboflavin, pyridoxine, folic acid, and cobalamin. A well-known water-soluble vitamin of the B complex family, thiamine, also known as vitamin B1, is produced by a variety of plants and microbes. Only a small amount of it is produced in mammals by the gut bacterial flora.8 Thiamine, with a short half-life ranging from 1 to 12 hours and limited body stores, underscores the necessity for a consistent dietary intake to maintain thiamine levels in tissues. Whole grains, yeasts, meats, legumes, and nuts stand out as some of the richest food sources of thiamine.9 The methylene bridge joins an aminopyrimidine and a thiazolium ring to form the hydrophilic and biosafe amino acid thiamine. Thiamine demonstrates significant coordination with nanoparticles (NPs) in the presence of sulfur moieties.10 Riboflavin (vitamin B2) is a biochemically active vitamin that is a member of the enormous flavin family and is a crucial cofactor in enzymatic reactions, including the Krebs cycle. The fluorescence and redox capabilities of riboflavin (RF) are due to the isoalloxazine ring group's presence in its chemical structure. It is a crucial substance for overall human health.11 It performs several essential tasks in biological systems, including the metabolism of lipids, carbohydrates, and proteins. It also contributes significantly to the human diet.12 Examples of natural sources of RF include milk, eggs, and leafy vegetables. According to the Food and Nutrition Board, the recommended daily allowance of RF for both women and men, assuming good health, is between 1.1 and 1.3 mg.13
Among the B-group vitamins, pyridoxine (Py), also known as vitamin B6, is essential for both physical and mental health. Py is a crucial vitamin that promotes the growth of healthy red blood cells and supports the most crucial physiological metabolism.14 Numerous studies suggest that the root cause of various diseases lies in a deficiency of vitamin B6. Since vitamin B6 cannot be synthesized within the human body, it needs to be supplied through foods rich in vitamin B2, such as fish, milk, certain fruits, and green vegetables.15 Water-soluble vitamin B9, also known as folic acid (FA), belongs to the B complex family. Its molecular structure consists of three moieties: glutamic acid (Glu) residues, 6-methyl pterin residues (Mep), and p-amino benzoic acid residues (PABA).16 FA is not naturally synthesized in the human body and can only be obtained through fortified foods and snacks, such as yeast, green vegetables, and certain citrus fruits.17 This vitamin has lately attracted a lot of consideration due to its apparent biological and antioxidant properties.18
Vitamin B12, also known as cobalamin or cyanocobalamin, is a vital organic chemical with a complex structure that has a corrin ring with a central Co(III) atom covalently bonded to nitrogen from corrin, allowing Co3+ to be reduced to Co2+.19 The human body produces blood cells and needs vitamin B12 to maintain brain processes. Primary sources of vitamin B12 include meat, eggs, dairy products, etc. Consequently, vegetarians are at a heightened risk of vitamin B12 deficiency.20 Thus, its absence results in numerous serious and irreversible harms, particularly to the neurological system and brain.20 Table 1 (references given refer to daily requirements) represents daily requirements and imbalances related to vitamin B complex.
Table 1 Requirements and related imbalances of vitamin B complex
Vitamins |
Daily requirement (μg d−1) |
Consequences |
References |
Deficiencies |
Excessive intake |
Thiamine (vitamin B1) |
1300 |
Metabolic, neurological, and developmental problems |
Allergic reactions, urticaria, angioedema, diaphoresis, cyanosis |
21 |
Riboflavin (vitamin B2) |
1300 |
Digestive problems, burning of the eyes |
DNA and liver oxidative injury |
22 |
Pyridoxine (vitamin B6) |
1500 |
Hyperemia, edema, skin problems, neurological diseases |
Malabsorption, impaired nerve function |
23 |
Folic acid (vitamin B9) |
400 |
Megaloblastic anemia, cardiovascular and birth defects |
Impede the absorption of VB12 and zinc |
17 |
Cobalamin (vitamin B12) |
2.4 |
Cognitive, cardiovascular, hematological and neuropsychiatric disorders |
Liver dysfunction, neurotoxicity, and kidney failure |
24 |
1.2 Non-electrochemical detection strategies for vitamins analysis
Numerous techniques have been devised to detect vitamins, including fluorescence25 ultra-performance liquid chromatography-electrospray ionization multiple reaction monitoring/mass spectrometry (UPLC-ESIMRM/MS)26 surface-enhanced Raman spectroscopy (SERS),27 surface plasmon resonance (SPR),28 capillary electrophoresis,29 and ultraviolet-visible spectroscopy.30 Various studies were done that used novel approaches, like Chen et al. developed a novel cyanostilbene macrocycle (CSM)-based organic fluorescence sensor. By using a straightforward Schiff-base “1 + 1” condensation and a crown-ether cyanostilbene macrocycle, a significant 78% yield was obtained, which showed high turn-on green fluorescence for vitamin B1 among other guests in aqueous media. CSM shows a limit of detection (LOD) of 7.8 × 10−7 M.31
A method for simultaneous quantification of vitamins B1, B6, and B12 in multivitamin tablets was established using high-performance liquid chromatography (HPLC) by Markopoulou. Gradient elution and a Hypersil-BDS C18 reversed phase column are used in the procedure. Triethylamine was 0.015% of the aqueous mobile phase; pH was adjusted to 2.7 using 1 N sulfuric acid and acetonitrile. By linearly altering the system's proportion with time-schedule software, separation and quantitation were made possible. A dual-beam ultraviolet (UV) detector tuned at 280 and 350 nm was employed for the detection process. Pyridoxine HCl was detected up to 0.005 g mL−1, thiamine HCl to 0.014 g mL−1, and hydroxocobalamin-Cl equal to 0.006 g mL−1 in the concentration range of 50–300 g mL−1.32
HPLC stands out as a widely favored technique for separating vitamins in diverse products because of its exceptional selectivity and sensitivity. However, this approach often necessitates a larger sample volume, increased solvent usage, labor-intensive sample preparation, and prolonged analysis times,33 hence, having a question mark on following green chemistry principles. A comparative study between conventional and non-conventional methods for vitamin detection, along with their sensitivities, has been summarized in Table 2.
Table 2 Comparing novel and conventional methods for vitamin sensing
Sr. no. |
Technique |
Vitamin |
Limit of detection (ng mL−1) |
References |
1 |
Colorimetric technique |
Vitamin B1 |
7.0 × 103 |
34 |
2 |
Surface plasmon resonance sensor |
Vitamin B2 |
13.5 × 10−4 |
35 |
3 |
Ultraviolet-visible spectroscopy |
Vitamin B2 |
6.0 × 106 |
36 |
4 |
Albumin-stabilized fluorescent copper nanocluster |
Vitamin B6 |
1.83 × 108 |
37 |
5 |
Surface plasmon resonance sensor |
Vitamin B9 |
2.5 × 10−4 |
35 |
6 |
High-performance liquid chromatography-photodiode array |
Vitamin B12 |
2.85 |
38 |
7 |
Fluorescence based sensor |
Vitamin B12 |
6.9 × 104 |
39 |
8 |
Surface plasmon resonance sensor |
Vitamin B12 |
2.5 × 10−4 |
35 |
Even though these methods offer notable advantages, including high selectivity and sensitivity, they also have drawbacks such as lengthy analysis times, complex sample preparation, large samples, expensive equipment, skilled personnel, and specialized labs. To address related issues effectively, there is a need to develop portable, user-friendly, and cost-effective approaches.40 Recent developments in electronics and sensor technology have established themselves as an affordable, quick, highly sensitive, and straightforward tool that makes point-of-care (POC) monitoring and the identification of medical conditions easier than with conventional diagnostic methods.41
1.3 Electrochemical approaches for sensing
Sensors have great importance due to their vast applications in automobiles, agriculture, medicine, water treatment, and many other fields. There are many types of sensors, like chemical sensors, immunosensors, voltammetric sensors, biosensors, and electrochemical sensors. The electrochemical technique represents a potent analytical tool capable of detecting electrical currents produced during redox reactions. Electrochemical detection happens when a working electrode (WE) interfaces with a target analyte and a potential is applied to it relative to the reference electrode (RE), with the simultaneous measurement of the resultant current.42 An illustration of the electrochemical setup and examples of respective electrode materials are given in Fig. 3.
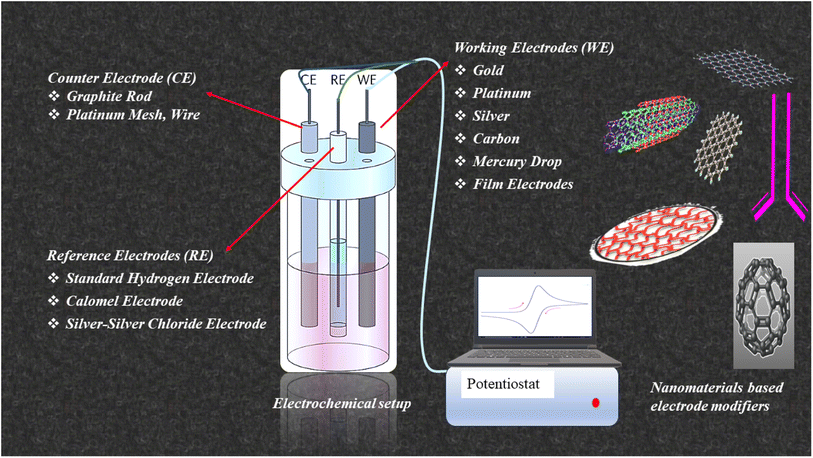 |
| Fig. 3 Schematic representation of electrochemical setup and examples of respective electrode materials. | |
1.4 Synergy between nanotechnology and electrochemical sensing
The combination of nanotechnology and electrochemical sensing has demonstrated impressive collaboration, yielding improved functionality across a wide range of applications. The integration of nanoscale materials, including nanoparticles, nanowires, and nanocomposites, into electrochemical sensors has yielded several benefits. The resulting advantages include increased surface area, improved electron transfer rates, and increased sensitivity, which together result in more accurate and efficient target analyte detection.43
Within the field of biosensing, gold and silver nanoparticles have been widely used to immobilize biomolecules, which makes it easier to identify individual analytes with precision. This collaborative approach has culminated in the creation of highly sensitive biosensors, which hold significant promise for utilization in healthcare, environmental monitoring, and ensuring food safety.44
1.4.1 Nanozyme-based electrochemical sensor. A number of enzyme-based electrochemical sensors for vitamins have been studied, and excellent substrate specificity and sensitivity have been reported.45,46 Unfortunately, their high price and poor stability, which caused them to denature under usual circumstances, limited their practical applicability.Non-enzymatic electrochemical sensors have been introduced in place of these drawbacks as a possible improvement.47 Researchers have successfully fabricated a selection of nanomaterial-based artificial enzymes (nanozymes) for the sensing of B complex vitamins. Yan et al. developed a promising non-enzymatic sensing platform based on zinc porphyrin MOF nanosheets for vitamin C with an LOD of 3.61 μM.48 Nanozymes derived from graphene exhibit intrinsic nanomaterial characteristics that not only serve as a straightforward alternative to enzymes but also provide a versatile platform capable of sensing analytes in intricate biochemical surroundings.49 MoS2/MWCNT porous nanohybrid structured nanozymes possessed oxidase-like properties and were used for the detection of carbendazim.50 Apart from nanozyme-based sensors, there are various types of electrochemical sensors. These sensors identify and quantify chemical or biological substances by utilizing the electrical response generated during a chemical reaction. Additional categories of electrochemical sensors include ion-selective electrodes (ISEs),51 biosensors,52 voltammetric sensors,53 potentiometric sensors,54 amperometric sensors,55 and field-effect transistors (FETs).56 Each type of electrochemical sensor has its advantages and limitations, making them suitable for specific applications. The choice of sensor depends on factors such as sensitivity, selectivity, cost, and the nature of the target analyte.
1.5 Various transducing matrixes for vitamins sensing
For electrochemical analysis, researchers have employed a wide variety of transducing matrices and electrodes. Electrochemical transducers play a crucial role in converting chemical information into electrical signals, finding applications in diverse fields. They are prized for their exceptional sensitivity, selectivity, and ability to operate in harsh environments. These transducers possess properties such as rapid response, wide dynamic range, and low detection limits, making them valuable for precise analyte detection. Furthermore, their compactness and ease of integration into portable devices enable on-site monitoring and diagnostics, enhancing their significance.57 Some of the transducing platforms are discussed here. Furthermore, this comparative overview paves the way for various researchers to choose a more selective and sensitive transducer platform for future investigations.
1.5.1 Carbon based electrodes. Recently, there has been progress in creating electrochemical sensors that are responsible for detecting specific substances with high sensitivity. Enhancing these sensors responses includes altering carbon-based electrodes through the incorporation of diverse materials. This section discusses recent developments in electrochemical detection of B complex vitamins using modified carbon-based electrodes, including carbon paste electrodes (CPEs), glassy carbon electrodes (GCEs), and screen-printed electrodes (SPEs).
1.5.1.1 Glassy carbon electrode (GCE). GCEs are among the most popular electrodes because of their lustrous surface. Glassy carbon, a non-graphitic carbon variety derived from the pyrolysis of specific polymeric precursors, offers exceptional properties. GCEs are widely employed in electrochemistry due to their attributes, which include high-temperature resistance, hardness, low density, and excellent electrical and chemical resistance when combined with other composite materials.58 This electrode is now frequently utilized in electrochemical sensing due to its effective sensing capabilities.Electroanalysis offers a powerful tool for the detection and quantification of redox-active vitamins in biological samples. The method for detecting vitamin B1 in a neutral solution via differential pulse voltammetry (DPV) was developed using a GCE by Putra et al. The deprotonation of thiamine hydrochloride was studied with an electro-generated base directly on the working electrode surface. An in situ method was suggested to do both: generate the base (with the applied negative potential) and detect the analyte (with the applied positive potential). The interference effects and real applications are investigated. In the obtained data, an oxidation peak for thiamine was observed at a potential of 0.03 V versus Ag/AgCl. The linear range extended from 0.6 mM to 1.6 mM, with a high correlation coefficient (R2 = 0.9809), and the method exhibited a detection limit of 5.14 × 10−4 M, a quantitation limit of 1.71 × 10−3 M, and a precision (% RSD) of 4.46%.59 However, without modification, bare GCE could not attain a much more sensitive range as compared to modified GCEs, as explained in subsequent examples.
The synthesis of cobalt-doped dysprosium oxide nanocubes (Co–Dy2O3 NCs) and their modification with GCE was first explained by Sangavi et al. for the selective and sensitive detection of vitamin B2. In that work, the electrochemical behavior of RF on the 10 wt% Co–Dy2O3/GCE significantly improved due to the high cobalt concentration, acting as a promoter to enhance the electrochemical reaction and improve electron transfer in RF, where the electrocatalytic reaction involved a redox process with the transfer of two protons and two electrons, shown in Fig. 4A. DPV and cyclic voltammetry (CV) were carried out using a three-electrode system to evaluate the samples. The modified electrode has two linear concentration ranges (0.5–50, 50–300 μM) with a low LOD of 0.094 μM and a high sensitivity of 3.67 μA μM−1 cm−2.60
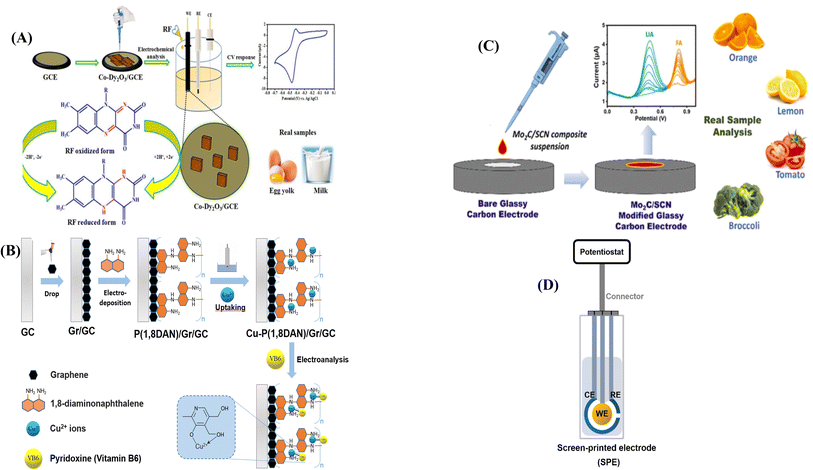 |
| Fig. 4 GCEs modification processes for various vitamins detection. (A) Reproduced from ref. 60 with permission from Wiley-VCH, copyright 2024 and (B) reproduced from ref. 61 with permission from Wiley-VCH, copyright 2022 (C) reproduced from ref. 62 with permission from Elsevier, copyright 2023 (D) representation of SPE potentiostat adopted from ref. 64 permission under the terms of the CC-BY Creative Commons Attribution 4.0. | |
Vitamin B6 was measured using square wave voltammetry (SWV) at a copper–poly(1,8-diaminonaphthalene)/graphene-modified GCE by Vu et al.61 In their study (Fig. 4B), a novel approach was employed to construct a copper–poly(1,8-diaminonaphthalene)/graphene composite film with the purpose of electrochemically detecting VB6. The polymer poly(1,8-diaminonaphthalene) exhibits chelating complexation properties with Cu2+ ions through the amine and secondary amino groups present on its chain. As a result of Cu2+ ions adhering to the polymer surface, a monolayer copper catalyst was formed, and the proposed catalyst facilitated the electrocatalytic oxidation of VB6. The integration of graphene into the composite film is aimed at improving its electrochemical properties, presenting a potential avenue for enhanced effectiveness and significance in the detection process. The peak current in SWV of pyridoxine exhibited a linear correlation with its concentration within the range of 0.58 to 24.51 μM, boasting the LOD of 0.3 μM in a 0.1 M phosphate buffer solution (pH 7.4) with better repeatability and stability.
Mo2C/SCN nanocatalyst was used to modify GCE for the purpose of sensing FA, as reported.62 Bare glassy carbon modification with Mo2C/SCN nanocomposites has been presented in Fig. 4C. The Mo2C/SCN nanocatalyst produced in that study underwent through characterization techniques such as scanning electron microscopy (SEM), including elemental mapping and X-ray diffraction (XRD). Exploration of the SEM images revealed a homogeneous attachment of the porous SCN network onto Mo2C, leading to a reduction in crystallinity evident in the diffractogram. Elemental mapping of Mo2C/SCN displayed distinct peaks corresponding to nitrogen (32.54%), carbon (41.47%), molybdenum (24.62%), and sulfur (1.37%), with no observable impurity peaks. The outcome indicated the effective synthesis of the nanocatalyst without additional contaminants. The designed Mo2C/SCN/GCE, exhibiting an enhanced electron transport rate, demonstrated the ability to electrochemically detect FA in a binary mixture. Using the DPV method, the LOD for FA was determined to be 14.7 nM (linear range = 0.09–167.25 μM) at Mo2C/SCN/GCE.62
Another important study was reported that was based on combining methylene blue and zinc oxide nanoparticles on a GCE (PMb/ZnO NPs/GCE) to assess VB12 in commercially available supplements. The improved DPV response for VB12 in the material could be attributed to the formation of a VB12–Zn complex. The complex involved PMb/ZnO NPs serving as an electrocatalyst, facilitating VB12 reduction. The polymer has a dual role, aiding electron transfer and increasing the electrode's surface area, enhancing sensitivity to VB12 in an aqueous solution. Surface coverage with PMB further improves electrode properties, such as electroconductivity and biocompatibility. These collective enhancements result in an increased response and sensitivity during the VB12 measurement. The linear relationship Ip = 0.0673x + 0.3449, r = 0.9942, and the redox peak current for the Co(II/I) pair at −0.8 V vs. Ag/AgCl under optimal circumstances revealed a quantification range for vitamin B12 concentrations of 0.099–69.51 μM with a detection threshold of 0.0104 μM.63
1.5.1.2 Carbon paste electrode (CPE). CPE is a heterogeneous hybrid made from graphite powder and a pasting fluid.65 The CPE's easy modification, renewable surface, low background current, wide potential range, small ohmic resistance, and affordability have made it a popular choice for working electrodes in the detection of biologically active substances.66In a study conducted by Tesfaye et al., a CPE was modified with poly(glutamic acid)/zinc oxide nanoparticles to create a sensor that exhibited reliability, repeatability, stability, and selectivity for measuring the amount of vitamin B2 in milk samples and nonalcoholic drinks. Under optimized conditions, the sensor demonstrated substantial electrocatalytic activity towards VB2 (Fig. 5A). During polymerization, an anodic peak at 1.54 V indicated the formation of monomer radicals, which initiated the process. A conductive polymer coating on ZnO NPs–CPE may have developed as a result of the increased oxidation peak current with cycles. Initially, oxidation of the glutamic acid monomer occurred at a higher positive potential, resulting in the formation of α-amino free radicals. The carbon electrode surface developed carbon–nitrogen bonds as a result of these radicals, which subsequently caused the formation of poly(glutamic acid) films. The redox peak currents at poly(glutamic acid)/ZnO NPs–CPE significantly increased, attributed to the synergistic effect of ZnO NPs and poly(glutamic acid). Because of its increased conductivity, large effective surface area, presence of functional groups, and strong accumulation capability, the suggested synergy featured high sensitivity and a low detection limit. The polymer film and VB2 engaged in hydrogen bonding and π–π interactions, which together increased the VB2's electron transfer rate and promoted its accumulation. The proposed sensor exhibited a linear response within the concentration range of 0.005–10 μM, with an LOD of 0.0007 μM and a high sensitivity of 21.53 μA μM−1 with acceptable recovery rates.67
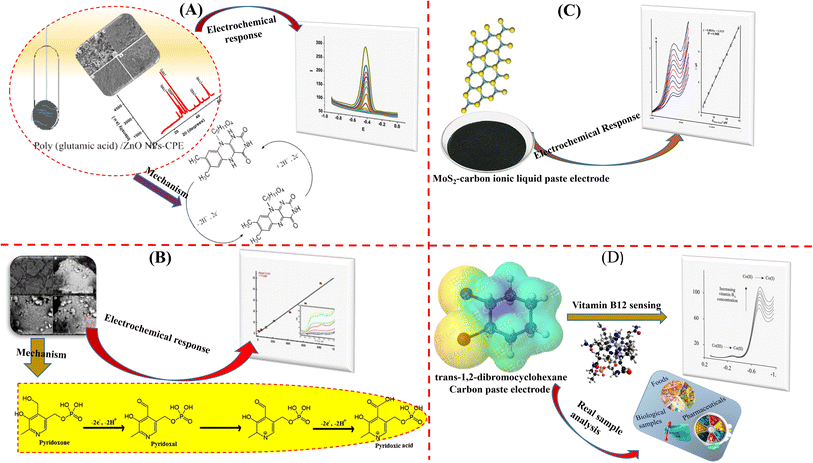 |
| Fig. 5 CPEs modification processes for various vitamins detection. Adapted figures (A–C) from the ref. 67–69 permission from Springer under the terms of the CC-BY Creative Commons Attribution 4.0. (D) Adapted from ref. 71 with permission from American Chemical Society, copyright 2004. | |
In an investigation carried out by Moustafa et al., a straightforward electrochemical sensor for transformed CPE with iron oxide nanoparticles was presented (Fig. 5B). The electro-oxidation mechanism of pyridoxine involves the generation of the aldehyde form (pyridoxal) and its subsequent oxidation to pyridoxic acid. The method tested vitamin B6 in phosphate buffer with a pH of 5.0–8.0, detection limits of 9.06 μM, and concentration ranges of 8.88–1000.0 μM, resulting in positive results in real samples.68
Vitamin B9 sensor was successfully fabricated by Nejad et al. by modifying a carbon ionic liquid paste electrode (CILPE) with two-dimensional transition metal dichalcogenide MoS2. The electrochemical properties of the produced electrode were investigated using chronoamperometry, DPV, and CV. The oxidation peak current for DPV was proportional to the concentration of B9 in the range of 5.0 M to 100.0 μM. The mechanism for the oxidation of FA on MoS2–CILPE was proposed based on the correlation between the oxidation potential and the pH of the supporting electrolyte (Fig. 5C). The optimized pH, corresponding to the highest peak current, was determined to be 7.0. This suggests the involvement of protons in the FA oxidation reaction, indicating a process that includes 2 electrons and 2 protons with an estimated LOD of 1.0 μM and a limit of quantification of 5.0 μM.69 In spite of having reasonable outcomes, using an ionic liquid-based modifier may be a limitation based on economic analysis.
Tomčik et al. introduced an innovative CPE for the detection of vitamin B12. CPE utilized trans-1,2-dibromocyclohexane (DBCH), serving both as a binding agent and a reactive component, enhancing the voltammetric signal (SWV) by reducing Co(III) or Co(II) to Co(I). Co(I) subsequently engaged in electrocatalytic reactions with DBCH, leading to the regeneration of Co(II). The proposed sensor reflected an outstanding sensitivity (LOD = 8.5 × 10−10 mol dm−3) based on the selected strategy of using trans-1,2-dibromocyclohexane as a binder as well as amplifying material (Fig. 5D).70
1.5.2 Screen printed electrode (SPE). Screen Printed Electrode (SPEs) are shaped through the application of diverse conductive inks onto plastic or ceramic substrates. Typically, polyester screens are employed for printing, allowing analysts to create patterns tailored to their specific analytical objectives. The selectivity and sensitivity needed for each study are determined by the different ink compositions when they are printed on electrodes.71 This technology has many advantages, including adaptability, affordability, excellent miniaturization capability, acceptable reproducibility, capacity for complicated structures, and commercial viability. The screen-printed carbon electrode SPCE, which has a primary electrochemical background and can typically be adjusted with a variety of modifiers, has been reported to have a broad range of applications in the fields of clinical, biological, pharmaceutical, environmental, and food analysis.17 SPE-potiostat setup is shown in Fig. 4D.64Papavasileiou et al. enhanced vitamin B2 with the use of silver nanoparticles coated onto SPES using controlled-energy sparking discharges. RF sensing was shown to work best with electrical discharges that had dissipated energies of 0.85 mJ, transferred charges of 1.5 C, and time constants of roughly 2 s per sparking site. The enhanced electrocatalytic characteristics of the silver nanoparticle (AgNP)-modified SPEs were demonstrated in the superior electroreduction of RF. The electroactive behavior of riboflavin includes a redox transition involving the transfer of 2 electrons and 2 protons (2e−/2H+). The detection range for DPV was 1.9 (LOQ) to 100 nM (R2 = 0.997) under ideal conditions, and an LOD of 0.56 nM was attained in real media.72
Jahani et al. employed zinc ferrite nanoparticles (ZnFe2O4) to modify a screen-printed graphite electrode (SPGE) to voltametrically determine vitamin B6 levels in real samples. Their findings indicated that on this modified electrode, the oxidation of vitamin B6 occurred at a potential approximately 150 mV less positive compared to an unchanged SPGE. The sensor demonstrated a strong linear response within the concentration range of 0.8–585.0 μM, boasting a detection limit of 0.17 μM for vitamin B6. Furthermore, the modified electrode effectively resolved the overlapping voltammetric peaks of vitamin B6 and vitamin C by nearly 530 mV.73
An electrochemical sensor for the detection of vitamin B9 was created by Mohammadi et al. using a Ni–BTC (BTC = benzene-1,3,5-tricarboxylic acid) metal–organic framework (MOF) modified SPE. The detection limit for this electrochemical sensor was determined to be 0.030 μM, and its linear response range was from 0.08 to 635.0 μM. In addition, the conventional addition method was used to assess the viability of the Ni–BTC MOF/SPE sensor's ability to detect B9 in real samples.74
Abid et al. suggested a 2-D MoS2 nanosheet with applied gold nanoparticles at the SPCE (AuNPs–MoS2/SPCE)-based electrochemical sensor for detecting vitamin B9. The AuNPs–MoS2/SPCE sensor showed a good sensitivity of 20.636 μA μM−1 cm−2, in the linear concentration range of 0–20 μM with an LOD of 1.04 μM.75 Although the reported sensor has good sensitivity, its cost-effectiveness might be compromised to some extent.
Microfluidic electrochemical devices enable cost-effective, sensitive, selective, and multiplexed vitamin analysis in diverse matrices and facilitate the development of portable and user-friendly systems. Fig. 6 represents some examples of integrating electrochemical sensing with microfluidics.76,77
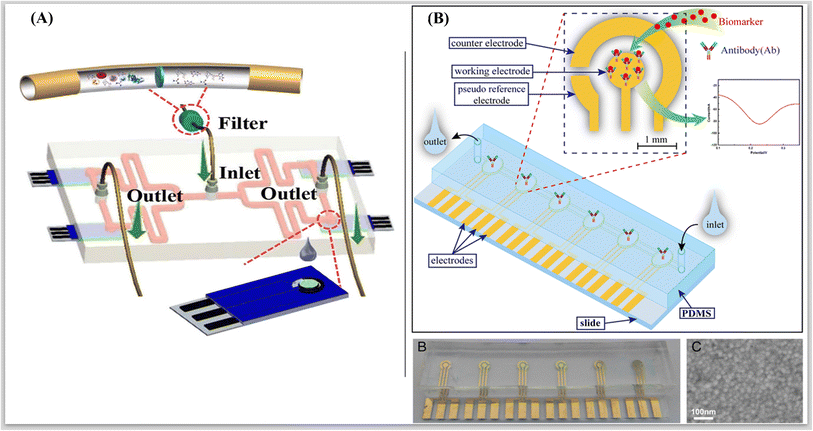 |
| Fig. 6 Microfluidics setup integration with electrochemical sensing platforms. (A) Reproduced from ref. 76 with permission from American Chemical Society, copyright 2020 (B) reproduced from ref. 77 with permission from Springer under the terms of the CC-BY Creative Commons Attribution 4.0. | |
2 Challenges in electrochemical sensing of vitamins
Electrochemical detection has become a versatile and efficient method for assessing vitamin concentrations due to its high sensitivity, rapid response, and cost-effectiveness. Despite significant progress in refining electrochemical methodologies for vitamin quantification, challenges persist in addressing issues such as sample matrix complexity, electrode fouling, calibration accuracy, and sensitivity and specificity limitations (Fig. 7). The full potential of electrochemical sensors for precise and reliable vitamin analysis can be realized through sustained research and innovative efforts focused on improving electrode materials, surface enhancements, and detection approaches. Overcoming these obstacles is crucial to unlocking the complete capabilities of electrochemical detection in the field of vitamin analysis.
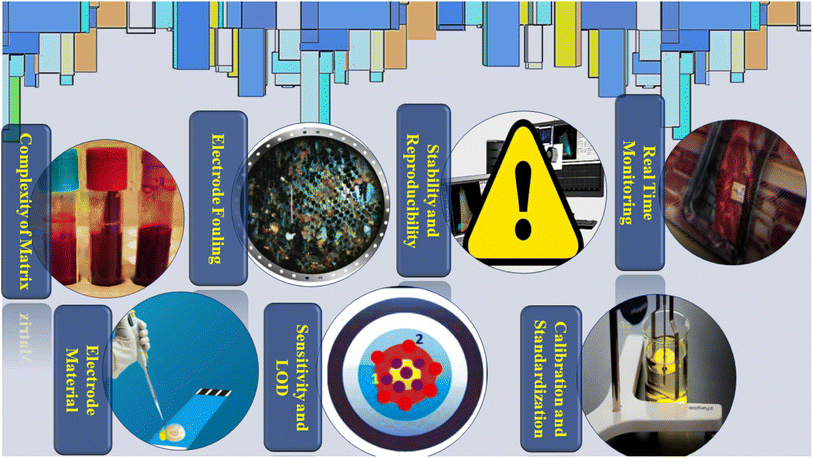 |
| Fig. 7 Challenges in electrochemical sensing of vitamins. | |
2.1 Pretreatment of complex vitamin matrixes
Vitamins are frequently found in complex matrices like pharmaceuticals, food, and biological fluids, requiring tailored electrochemical methods to accurately distinguish different vitamin derivatives based on their redox properties.78 Despite their potential, several challenges, like pretreatment (which converts the complex matrix into a simpler, more suitable form), exist in the electrochemical detection of vitamins that need to be addressed for reliable and accurate analyses. The concentration of analytes in real samples, for instance, in food, biological fluids (up to nmol), and pharmaceutical formulations (up to mg g−1), is low, and the molecular weight of analytes is small compared to coexisting foulants in matrices.79,80
The presence of interfering species can adversely affect selectivity and sensitivity, and the capture of small-molecule analytes in complex food matrices and the signal transduction of sensing surface changes are challenging for sensors.81 Many real samples contain complex matrices that can interfere with the electrochemical measurements of vitamins. Strategies such as sample pretreatment,82 electrode surface modifications,83 and the use of suitable mediators84,85 have been explored to mitigate interference effects.
Developing sensors that can selectively detect vitamins in complex matrices remains a significant challenge. Also, there is not much literature reported on electrochemical sensors for the simultaneous detection of both fat-soluble and water-soluble vitamins; therefore, it is necessary to explore such dynamic electroactive platforms that have the ability to work on all the vitamins. In this way, a complete picture of the vitamin profile of the required sample (human, animal, plant, etc.) using a single platform can be attained.
2.2 Electrode material selection
A critical challenge in the electrochemical detection of vitamins is the appropriate selection of electrode materials. New electrode materials and improved sensor designs are needed to enhance the selectivity of electrochemical sensors towards the target vitamins while minimizing interference from coexisting compounds.86
The performance of a sensor depends on the choice of material, which should possess excellent electrocatalytic properties, a high surface area, and stability. Researchers have investigated various materials, such as carbon-based materials,87,88 metal nanoparticles and composites,89,90 and conducting polymers,91–93 to enhance the sensitivity and selectivity of sensors. However, finding an ideal electrode material that provides both stability and sensitivity to different vitamins remains an ongoing challenge.
2.3 Electrode fouling in real complex media
Electrode fouling remains a persistent issue during vitamin detection and leads to a decline in sensitivity and accuracy over time.94 The formation of biofilms, oxidation products, or adsorbed species on the electrode surface can obstruct the electron transfer process, resulting in a degraded analytical performance.81 Matrix components might lead to fouling of the electrode surface, thus passivating them, masking the target analytes, or altering the electrochemical behavior.36 Scientists need to explore novel surface modification techniques and electrode materials to mitigate fouling effects and extend the operational lifetime of electrochemical sensors. Various antifouling materials have been used, like modified multiwalled carbon nanotubes,95 zwitterionic polymers,96 mesoporous silica film,97 poly(glutamic acid),98 cellulose,99 and hybrid materials.100–102 However, exploring active anti-fouling materials from green and synthetic sources is an ongoing issue on which current research is focused.
2.4 Sensitivity and limit of detection (LOD)
The accurate detection of vitamins at trace levels is crucial for various applications, including dietary assessments and clinical diagnostics. However, achieving ultralow detection limits for some vitamins, particularly those required in minute quantities, can be challenging.103 Researchers have employed various signal amplification strategies, such as nanomaterial modification using AuNP, CuS, ZnFe2O4 (ref. 73, 104 and 105) enzymatic amplification, i.e., NAD(P)H-dependent quinone reductase,106 and signal enhancement techniques, i.e., amplification by employing materials like MoS2@ErGO, CoNPs/CuNPs,103,107 to improve sensitivity and LOD.
Although electrochemical techniques offer good sensitivity, achieving ultralow detection limits for certain vitamins remains a challenge. Particularly for water-soluble vitamins, such as vitamin B complex and vitamin C, enhancing sensitivity beyond current levels is vital to address nutritional deficiencies and monitor health conditions effectively.108,109 Exploring advanced signal amplification strategies and utilizing nanomaterial-based sensors is a potential avenue for enhancing sensitivity. However, further improvements are required to achieve the desired sensitivity levels.
2.5 Stability and reproducibility
The stability of electrochemical sensors for vitamin detection pertains to the ability of the sensor to maintain uniform performance over time and under varying conditions. Electrode surface modifications, interactions with the sample matrix, and electrochemical reactions can all contribute to sensor instability. For instance, the degradation of electrode materials over time can lead to decreased sensitivity and altered response characteristics. To combat this issue, researchers have explored novel electrode materials and protective coatings.110,111
Reproducibility in electrochemical vitamin detection refers to the ability to obtain consistent results when repeating experiments under similar conditions. Inconsistencies can arise from variations in electrode fabrication, sample preparation, and measurement conditions. These variations can stem from factors such as electrode surface roughness, deposition techniques, and operational parameters. Achieving high reproducibility requires standardized protocols and rigorous quality control measures.111–113 To ensure reliable results, electrochemical sensors for vitamin detection should demonstrate long-term stability and reproducibility. Electrode fouling, degradation, and drift can occur over time, leading to decreased sensor performance. Developing robust electrode surface modifications and optimizing the experimental conditions is essential to overcome these challenges and maintain sensor stability and reproducibility over extended periods.
2.6 Robust and on-spot real-time monitoring
In various applications, real-time monitoring of vitamin levels is crucial to promptly respond to changes or deficiencies. However, the time required for electrochemical analysis, including sample preparation and analysis, is a limitation. The time required for the sensor to reach equilibrium, respond to changes in the analyte concentration, and stabilize can limit its ability to capture rapid changes in vitamin levels.114 Therefore, researchers must develop rapid and portable electrochemical devices capable of providing on-site, real-time measurements of vitamins.
2.7 Calibration and standardization
Establishing accurate calibration curves and standardizing electrochemical methods for vitamin detection is crucial for obtaining reliable results. Factors such as electrode preparation, solution composition, and potential scan rate can influence the electrochemical response. The creation of standardized protocols and reference materials will aid in the comparison and reproducibility of results across different laboratories.
3 Microfluidic systems in vitamins detection
Microfluidics has emerged as a high-performance platform in recent years capable of liquid handling at the micro- and nanoscale. It refers to a channel or device size in which one characteristic length is less than a millimeter. Due to the small size of the channel, microfluidic devices are more affordable, automated, integrated, portable, and offer point-of-care testing. These devices are divided into various types of liquid handling platforms, such as channel-based microfluidics (continuous flow), paper-based microfluidics (porous media), and digital microfluidics (droplets) which rely on electrowetting.115,116
Fabrication of microfluidic devices is based on either photolithography based chemical etching techniques, or by physical ablation or cutting such as laser machine and micromachining represented in Fig. 8. This is termed as the top-down approach. Additive manufacturing such as 3D printers have also emerged recently as a viable fabrication method using the bottom-up approach. Once the platform is fabricated, it is sealed and connected to flow control electronics to allow movement of fluid at the micron level. There are different materials used for microfluidic devices among which silicon is the most common element. This was later replaced by the more affordable elastomer, PDMS, still requiring a cleanroom facility for its fabrication. Other materials include thermoplastics, paper, and thin films.117
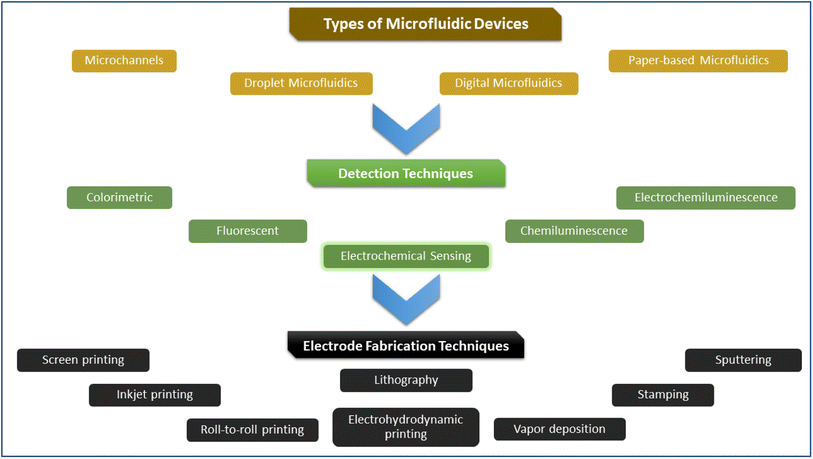 |
| Fig. 8 Representation of types, detection methods, and electrode modification processes involved in microfluidics devices. | |
These devices are applied in food safety testing, environmental detection of elements, and health diagnostics. Specifically, the food sensing application relies extensively on electrochemical detection, primarily because the original test uses the same technology. These electrochemical sensing electrodes have various options to be deposited onto micro-electromechanical systems (MEMS). These electrodes can be deposited using photolithography, soft lithography, screen printing, inkjet printing, roll-to-roll printing, electrohydrodynamic printing, stamping, vapor deposition, sputtering, dip pen, polymer-assisted metal deposition, and ion-exchange technology.118
Using these techniques, vitamin detection platforms have been developed in recent years, combining electrochemistry with MEMS technology. Examples include the detection of water-soluble vitamins using carbon nanotubes deposited using screen printing to enhance the resolution by a factor of 2.119 Paper-based devices have also been developed to detect vitamin C on a low-cost, disposable platform using an electrochemical sensor.120 The device used a polyaniline (PANI)-modified carbon electrode as the working electrode and two simple screen-printed carbon electrodes as the reference and counter electrodes. The results showed a limit of detection of 30 ± 3 μM using a 30–270 μM concentration.
As perceived from Table 3, there are still opportunities where electrochemical methods have not been explored for various vitamins or their functionality testing in functional environments such as organ-on-chip.126 Therefore, there is a need to focus more on such platforms for direct diagnosis and analysis.
Table 3 Microfluidic platforms and detection methods used for the vitamin B complex
Vitamin |
Platform material |
Detection method |
LOD (nM) |
References |
Thiamine (vitamin B1) |
Transparency sheet |
Colorimetric sensing |
8.88 |
121 |
Riboflavin (vitamin B2) |
Microchannel |
SERS and electrochemical detection |
1 and 100 |
122 |
Pyridoxine (vitamin B6) |
Carbon fiber paper |
Electrochemical detection |
10 |
123 |
Folic acid (vitamin B9) |
Self-assembly of graphene oxide/Ag nanoparticle hybrids |
SERS |
9 |
124 |
Cobalamin (vitamin B12) |
Microchannel |
Chemiluminescence |
|
125 |
3.1 Wearable devices
There have been significant advancements in wearable or portable devices that can be easily attached to the human body or carried with ease, so that vital signal detection can be performed at any time and place. For instance, a vitamin C sensor developed by researchers from UC-Berkeley uses the three-electrode system on planar polyethylene terephthalate substrate.127 It is further functionalized using Au dendrites, PEDOT/LiClO4 deposition, and enzyme immobilization surfaces. The flexible sensor maintains consistent current output and measures vitamin C levels using sweat. Another wearable biosensor used polyaniline film modified with organic acids for vitamin C detection.127 Wagih et al. demonstrated the use of graphene electrodes functionalized with redox-active nanoparticles and combined with microfluidics and iontophoresis to detect various amino acids and vitamins.128 Fig. 9 demonstrates recent developments and future opportunities in MEMS.32,116,129–131,133
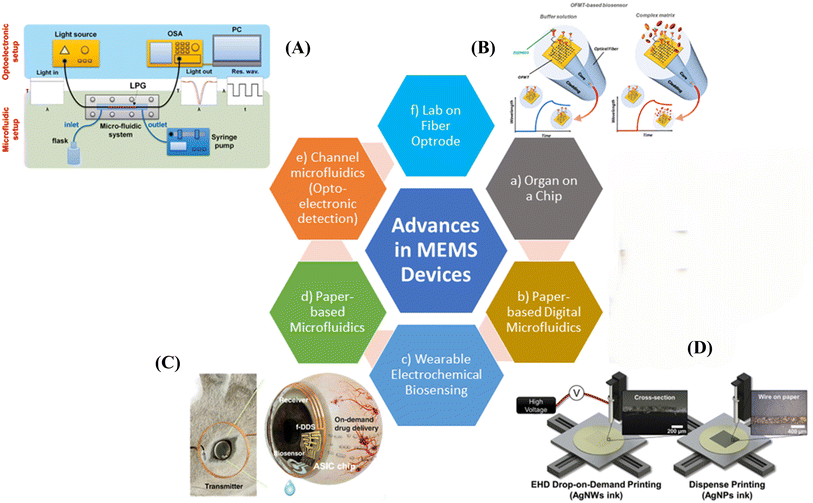 |
| Fig. 9 Advances in MEMS (micro-electromechanical system) and their prospects. Image (A) adopted from ref. 133 with permission from Elsevier, copyright 2015. Image (B) reproduced from ref. 131 with permission from Elsevier copyright under the Creative Commons CC-BY. Image (C) reproduced from ref. 129 with permission from AAAS copyright under the terms of the CC-BY Creative Commons attribution 4.0. Image (D) adapted from ref. 116 with permission from American Chemical Society, copyright 2020. | |
3.2 Challenges in device fabrication
As the diversity of food choices expands, chemical usage proliferates, and our standards of living continue to rise in tandem with the pursuit of healthier lifestyles. Herein, the detection of vitamin levels has garnered considerable interest. In a society where healthcare and food safety are fundamental priorities, this attention is well-placed, however, the sensing platforms have a significant challenge in the following areas:
(1) Affordable
(2) Reliable
(3) Energy utilization
(4) Sustainable manufacturing
(5) Ease of manufacturing
(6) Biodegradable
(7) Biocompatible
Some challenges have already been addressed, such as paper substrates providing an affordable and biodegradable substrate that uses no energy for fluid flow. Similarly, polymer materials have been designed for microfluidics for flexible and improved surface modification properties. However, both have challenges in terms of high volumes of production and reliability issues in the long run. Another challenge is the multidetector system since sensing only a single analyte will not be enough for a complete health analysis. Therefore, the MEMS technology (denoted in Fig. 6) needs significant improvement to achieve real-time multi-component detection with high sensitivity and selectivity.126,132
4 Future aspects
Electrochemical sensing has a bright future ahead of it, with constant progress and inventions that could completely transform a number of sectors. Electrochemical sensors are predicted to become essential in areas including food safety, environmental monitoring, and healthcare with continued research and development. These sensors are expected to become smaller and more sensitive, allowing for more accurate real-time on-site detection of a variety of analytes. Integration with cutting-edge technology, such as microfluidics, artificial intelligence, and the Internet of Things (IoT), could result in the creation of smart sensing systems that offer data analysis and seamless connections. Additionally, the exploration of new materials and fabrication techniques is likely to enhance the performance, durability, and versatility of electrochemical sensors, opening doors to novel applications and expanding their impact on monitoring and control systems in the future (Fig. 10).
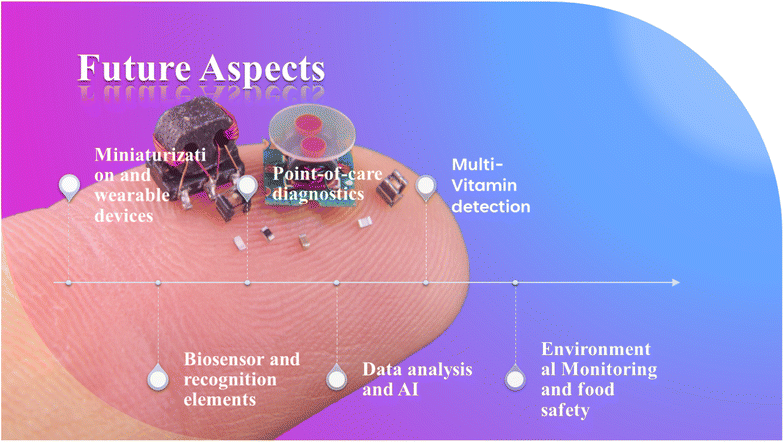 |
| Fig. 10 Future aspects of electrochemical sensors. | |
4.1 Miniaturization and wearable devices
The electrochemical vitamin sensors are likely to extend into wearable technologies and “electrochemical microfluidics” or “electrochemical lab-on-a-chip” devices. These compact devices could facilitate real-time monitoring of vitamin levels in individuals, offering valuable insights into personalized health and wellness.
4.2 Biosensors and recognition element
Integrating biomolecules like enzymes, antibodies, or aptamers into electrochemical microfluidic sensors has the potential to amplify precision and exclusivity when detecting specific vitamins. Progress in the field of bioconjugation methods and bio-recognition components could substantially improve the precision of vitamin detection.
4.3 Point-of-care (POC) diagnostics
Electrochemical vitamin detectors prove to be highly suitable for POC medical assessments owing to their portable nature and user-friendly operation. Subsequent advancements might concentrate on designing approachable devices capable of being operated by individuals without specialized training, thereby delivering swift and precise insights into vitamin deficiencies or excesses.
4.4 Data analysis and artificial intelligence
With the rising intricacy and abundance of data produced by electrochemical sensors, the incorporation of artificial intelligence (AI) and machine learning has the potential to support real-time analysis of data, the identification of patterns, and the creation of predictive models. This could ultimately elevate the precision and dependability of vitamin detection. Sensors produce data, a key but sometimes overlooked aspect of sensor technology. This data requires processing, often involving artificial intelligence and deep learning, to provide real-time feedback to users. Handling the growing volume of diverse data from various sources is a significant challenge in this field.6
4.5 Simultaneous detection of various vitamins
Revolutionizing the realm of vitamin analysis, the development of electrochemical microfluidics sensors capable of simultaneously detecting numerous vitamins at a time holds the potential to streamline analytical procedures and offer a holistic comprehension of nutritional well-being. This innovative research direction has the power to transform the landscape of vitamin analysis.
4.6 Environmental monitoring and food safety
Electrochemical vitamin sensors have the potential to be utilized for tracking vitamin levels in food items and evaluating the nutritional composition of crops in agriculture. This technological advancement could play a vital role in safeguarding both food safety and quality standards.
5 Conclusions
In summary, vitamins are essential micronutrients vital for human health and well-being, playing a central role in various physiological processes. Insufficient intake of these nutrients can result in a spectrum of health-related issues, underscoring the imperative for accurate and dependable sensing methods to monitor their presence. While multiple analytical techniques exist for vitamin detection, they often entail significant drawbacks, including high costs, lengthy analysis times, and resource-intensive processes. In response to these limitations, electrochemical sensors have gained prominence as a viable solution. These sensors offer notable advantages, including exceptional sensitivity, rapid analysis capabilities, cost-effectiveness, and straightforward fabrication procedures. This review underscores the increasing significance of electrochemical sensing, particularly in the context of water-soluble vitamins like B1, B2, B6, B9, and B12.
Nevertheless, the path toward effective vitamin sensing presents its share of challenges. Researchers grapple with issues pertaining to selectivity, sensitivity, interference, matrix effects, sample preparation, and calibration. These hurdles constitute critical areas for ongoing exploration and innovation. Looking forward, the integration of nanomaterials, sensor miniaturization, electrochemical microfluidics, and the development of inventive sensor designs hold significant promise for the field. These advancements offer a route to the creation of portable, user-friendly, and highly cost-efficient methodologies for the detection of essential vitamins. By addressing these challenges and embracing emerging technologies, we can enhance our capacity to monitor and safeguard human health through precise vitamin detection methods.
Data availability
The raw/processed data required to reproduce these findings cannot be shared at this time as the data also forms part of an ongoing study.
Conflicts of interest
The authors declare that they have no known competing financial interests or personal relationships that could have appeared to influence the work reported in this paper.
References
- M. Hrubša, T. Siatka, I. Nejmanová, M. Vopršalová, L. Kujovská Krčmová, K. Matoušová, L. Javorská, K. Macáková, L. Mercolini and F. Remião, Nutrients, 2022, 14, 484 CrossRef PubMed
. - A. Podkowa, A. Kryczyk-Poprawa, W. Opoka and B. Muszyńska, Eur. Food Res. Technol., 2021, 247, 513–533 CrossRef CAS
. - M. Alagawany, S. S. Elnesr, M. R. Farag, R. Tiwari, M. I. Yatoo, K. Karthik, I. Michalak and K. Dhama, Vet. Q., 2021, 41, 1–29 CrossRef CAS PubMed
. - I. Darnton-Hill, Curr. Dev. Nutr., 2019, 3, nzz075 CrossRef PubMed
. - E. F. Marondedze, L. O. Olasunkanmi, A. Singh and P. P. Govender, Emerging Nanomaterials and Their Impact on Society in the 21st Century, 2023, vol. 135, pp. 284–303 Search PubMed
. - E. Comini, Front. Sens., 2021, 1, 607063 CrossRef
. - J. Santos, J. A. Mendiola, M. B. Oliveira, E. Ibáñez and M. Herrero, J. Chromatogr. A, 2012, 1261, 179–188 CrossRef CAS PubMed
. - R. Mancinelli and M. Ceccanti, Alcohol Alcohol., 2009, 44, 177–182 CrossRef CAS PubMed
. - K. C. Whitfield, M. W. Bourassa, B. Adamolekun, G. Bergeron, L. Bettendorff, K. H. Brown, L. Cox, A. Fattal-Valevski, P. R. Fischer and E. L. Frank, Thiamine Deficiency Disorders: Diagnosis, Prevalence, and a Roadmap for Global Control Programs, Report 0077-8923, Wiley Online Library, 2018 Search PubMed.
- A. Saeed, M. Akhtar, S. Zulfiqar, F. Hanif, I. A. Alsafari, P. O. Agboola, S. Haider, M. F. Warsi and I. Shakir, Chem. Pap., 2022, 1–11 Search PubMed
. - A. Sharma, A. Khosla and S. Arya, Microchem. J., 2020, 156, 104858 CrossRef CAS
. - R. Madhuvilakku, S. Alagar, R. Mariappan and S. Piraman, Sens. Actuators, B, 2017, 253, 879–892 CrossRef CAS
. - S. K. Schwechheimer, E. Y. Park, J. L. Revuelta, J. Becker and C. Wittmann, Appl. Microbiol. Biotechnol., 2016, 100, 2107–2119 CrossRef CAS PubMed
. - D. R. Kumar, D. Manoj, J. Santhanalakshmi and J.-J. Shim, Electrochim. Acta, 2015, 176, 514–522 CrossRef CAS
. - T. T. Calam, Microchem. J., 2021, 169, 106557 CrossRef
. - M. L. Dántola, M. P. Denofrio, B. Zurbano, C. S. Gimenez, P. R. Ogilby, C. Lorente and A. H. Thomas, Photochem. Photobiol. Sci., 2010, 9, 1604–1612 CrossRef PubMed
. - M. Alizadeh, F. Garkani Nejad, Z. Dourandish, S. Tajik, F. Karimi, P. Mohammadzadeh Jahani, A. Aghaei Afshar, R. Zaimbashi, I. Sheikhshoaie and H. Beitollahi, J. Food Meas. Charact., 2022, 16, 3423–3437 CrossRef
. - F. Chekin, F. Teodorescu, Y. Coffinier, G.-H. Pan, A. Barras, R. Boukherroub and S. Szunerits, Biosens. Bioelectron., 2016, 85, 807–813 CrossRef CAS PubMed
. - D. M. Stanković, D. Kuzmanović, D. Manojlović, K. Kalcher and G. Roglić, J. Electrochem. Soc., 2016, 163, B348 CrossRef
. - S. Antherjanam, B. Saraswathyamma, R. G. Krishnan and G. M. Gopakumar, Chem. Pap., 2021, 75, 2981–2995 CrossRef CAS
. - J. Oni, P. Westbroek and T. Nyokong, Electroanalysis, 2002, 14, 1165–1168 CrossRef CAS
. - B. R. A. Junior, F. L. F. Soares, J. A. Ardila, L. G. C. Durango, M. R. Forim and R. L. Carneiro, Spectrochim. Acta, Part A, 2018, 188, 589–595 CrossRef CAS PubMed
. - R. Rejithamol and S. Beena, Chem. Pap., 2020, 74, 2011–2020 CrossRef CAS
. - G. Tsiminis, E. P. Schartner, J. L. Brooks and M. R. Hutchinson, Appl. Spectrosc. Rev., 2017, 52, 439–455 CrossRef
. - A. Paudics, D. Hessz, M. Bojtár, I. Bitter, V. Horváth, M. Kállay and M. Kubinyi, Sens. Actuators, B, 2022, 369, 132364 CrossRef CAS
. - A. El-Hawiet, F. M. Elessawy, M. El Demellawy and A. F. El-Yazbi, Microchem. J., 2022, 181, 107625 CrossRef CAS
. - M. Jafari, M. Mousavi, K. Shirzad, M.-A. Hosseini, A. Badiei, P. Pourhakkak and J. B. Ghasemi, Microchem. J., 2022, 181, 107813 CrossRef CAS
. - V. Prakashan, G. Gejo, M. Sanu, M. Sajna, T. Subin, P. Biju, J. Cyriac and N. Unnikrishnan, Appl. Surf. Sci., 2019, 484, 219–227 CrossRef CAS
. - F. Tezcan and F. B. Erim, Beverages, 2018, 4, 86 CrossRef CAS
. - T. B. V. da Silva, A. de Oliveira, T. F. M. Moreira, K. C. da Silva, R. C. Zanin, E. Bona, O. H. Gonçalves, M. A. Shirai, A. P. Peron and F. V. Leimann, Food Chem., 2021, 360, 129979 CrossRef CAS PubMed
. - J. Chen, X. Huang, H. Tang, H. Guo and F. Yang, Dyes Pigm., 2022, 207, 110705 CrossRef CAS
. - C. K. Markopoulou, K. Kagkadis and J. Koundourellis, J. Pharm. Biomed. Anal., 2002, 30, 1403–1410 CrossRef CAS PubMed
. - X. Wang, K. Li, L. Yao, C. Wang and A. Van Schepdael, J. Pharm. Biomed. Anal., 2018, 147, 278–287 CrossRef CAS PubMed
. - B. R. Khalkho, R. Kurrey, M. K. Deb, K. Shrivas, S. S. Thakur, S. Pervez and V. K. Jain, Heliyon, 2020, 6, e03423 CrossRef PubMed
. - D. Çimen and A. Denizli, Photonic Sens., 2020, 10, 316–332 CrossRef
. - T. Zhou, H. Li, M. Shang, D. Sun, C. Liu and G. Che, TrAC, Trends Anal. Chem., 2021, 143, 116412 CrossRef CAS
. - C. Chauhan, V. Bhardwaj and S. K. Sahoo, Microchem. J., 2021, 170, 106778 CrossRef CAS
. - H. İ. Ulusoy, S. Gülle, E. Yilmaz and M. Soylak, Anal. Methods, 2019, 11, 5108–5117 RSC
. - X. Ji, S. Wang, Y. Luo, X. Yuan, Y. Wei, Q. Zhang, K. Qin and Y. Tu, Dyes Pigm., 2021, 184, 108818 CrossRef CAS
. - S. Wadhwa, A. T. John, S. Nagabooshanam, A. Mathur and J. Narang, Appl. Surf. Sci., 2020, 521, 146427 CrossRef CAS
. - Y. Uludag, F. Narter, E. Sağlam, G. Köktürk, M. Y. Gök, M. Akgün, S. Barut and S. Budak, Anal. Bioanal. Chem., 2016, 408, 7775–7783 CrossRef CAS PubMed
. - Ö. B. Ünlüer, F. Ghorbani-Bidkorbeh, R. Keçili and C. M. Hussain, in Handbook on Miniaturization in Anal. Chem., Elsevier, 2020, pp. 277–296 Search PubMed
. - A. D. Ambaye, K. K. Kefeni, S. B. Mishra, E. N. Nxumalo and B. Ntsendwana, Talanta, 2021, 225, 121951 CrossRef CAS PubMed
. - A. V. Police Patil, Y.-S. Chuang, C. Li and C.-C. Wu, Biosensors, 2023, 13, 125 CrossRef CAS PubMed
. - J. R. Sempionatto, A. A. Khorshed, A. Ahmed, A. N. De Loyola e Silva, A. Barfidokht, L. Yin, K. Y. Goud, M. A. Mohamed, E. Bailey and J. May, ACS Sens., 2020, 5, 1804–1813 CrossRef CAS PubMed
. - J. R. Sempionatto, V. R.-V. Montiel, E. Vargas, H. Teymourian and J. Wang, ACS Sens., 2021, 6, 1745–1760 CrossRef CAS PubMed
. - M. Awan, M. Nasir, S. Iqbal, K. Manshad, A. Hayat and H. Ajab, Synth. Met., 2023, 292, 117240 CrossRef CAS
. - T. Yan, G. Zhang, K. Yu, H. Chai, M. Tian, L. Qu, H. Dong and X. Zhang, Chem. Eng. J., 2023, 455, 140779 CrossRef CAS
. - L. A. Dinu and S. Kurbanoglu, Nanoscale, 2023, 15, 16514–16538 RSC
. - X. Zhu, P. Liu, Y. Ge, R. Wu, T. Xue, Y. Sheng, S. Ai, K. Tang and Y. Wen, J. Electroanal. Chem., 2020, 862, 113940 CrossRef CAS
. - I. M. Shakir, Baghdad Sci. J., 2016, 13, 0458 CrossRef
. - Y. Gao, F. Guo, S. Gokavi, A. Chow, Q. Sheng and M. Guo, Food Chem., 2008, 110, 769–776 CrossRef CAS
. - A. Baghizadeh, H. Karimi-Maleh, Z. Khoshnama, A. Hassankhani and M. Abbasghorbani, Food Anal. Methods, 2015, 8, 549–557 CrossRef
. - A. R. Pires, A. N. Araújo, M. C. B. Montenegro, P. Chocholous and P. Solich, J. Pharm. Biomed. Anal., 2008, 46, 683–691 CrossRef CAS PubMed
. - B. Su, Y. Chen, X. Yang, J. Han, H. Jia, P. Jing and Y. Wang, Int. J. Electrochem. Sci., 2017, 12, 6417–6427 CrossRef CAS
. - V. Volotovsky and N. Kim, Anal. Chim. Acta, 1998, 359, 143–148 CrossRef CAS
. - X. Huang, Y. Zhu and E. Kianfar, J. Mater. Res. Technol., 2021, 12, 1649–1672 CrossRef CAS
. - Y. Yi, G. Weinberg, M. Prenzel, M. Greiner, S. Heumann, S. Becker and R. Schlögl, Catal. Today, 2017, 295, 32–40 CrossRef CAS
. - B. R. Putra, V. P. Rahmawati, E. Rohaeti and W. T. Wahyuni, AIP Conf. Proc., 2022, 2638(1), 050002 CrossRef CAS
. - R. Sangavi, M. Keerthana and T. Pushpa Malini, ChemistrySelect, 2022, 7, e202201661 CrossRef CAS
. - T. V. Vu, M. T. Nguyen, T. T. Do, H. L. Nguyen, V. A. Nguyen and D. T. Nguyen, Electroanalysis, 2022, 34, 1478–1486 CrossRef CAS
. - P.-S. Ganesh, M. Govindasamy, S.-Y. Kim, D.-S. Choi, H.-U. Ko, R. A. Alshgari and C.-H. Huang, Ecotoxicol. Environ. Saf., 2023, 253, 114694 CrossRef CAS PubMed
. - N. Abd-Alazize and A. Mohammedtayeb, Egypt. J. Chem., 2023, 66, 375–384 Search PubMed
. - S. Damiati and B. Schuster, Sensors, 2020, 20, 1721 CrossRef CAS PubMed
. - I. Svancara, K. Kalcher, A. Walcarius and K. Vytras, Electroanalysis with Carbon Paste Electrodes, CRC Press, 2012 Search PubMed
. - G. Tesfaye, N. Negash, T. Hailu and M. Tessema, Electroanalysis, 2022, 34, 1054–1064 CrossRef CAS
. - G. Tesfaye, N. Negash and M. Tessema, BMC Chem., 2022, 16, 1–17 CrossRef PubMed
. - A. Moustafa, R. S. El-Kamel, S. Abdelgawad, A. Fekry and M. Shehata, Ionics, 2022, 28, 4471–4484 CrossRef CAS
. - H. S. Nejad, F. G. Nejad and H. Beitollahi, ADMET DMPK, 2023, 1823 Search PubMed
. - P. Tomčik, C. E. Banks, T. J. Davies and R. G. Compton, Anal. Chem., 2004, 76, 161–165 CrossRef PubMed
. - O. D. Renedo, M. Alonso-Lomillo and M. A. Martinez, Talanta, 2007, 73, 202–219 CrossRef CAS PubMed
. - A. V. Papavasileiou, T. Hoder, T. Medek, M. I. Prodromidis and J. Hrbac, Talanta, 2023, 258, 124409 CrossRef CAS PubMed
. - P. M. Jahani, M. Jafari and S. A. Ahmadi, ADMET DMPK, 2023, 11, 251–261 Search PubMed
. - S. Z. Mohammadi, F. Mousazadeh and M. Mohammadhasani-Pour, J. Electrochem. Sci. Eng., 2022, 12, 1111–1120 CAS
. - K. Abid, R. Zribi, R. Maalej, A. Foti, A. Khaskhoussi, P. G. Gucciardi and G. Neri, FlatChem, 2022, 36, 100433 CrossRef CAS
. - L. Zhu, X. Liu, J. Yang, Y. He and Y. Li, Anal. Chem., 2020, 92, 11981–11986 CrossRef CAS PubMed
. - Y. Xie, X. Zhi, H. Su, K. Wang, Z. Yan, N. He, J. Zhang, D. Chen and D. Cui, Nanoscale Res. Lett., 2015, 10, 1–9 CrossRef CAS PubMed
. - J. Kumar, R. A. Soomro, R. R. Neiber, N. Ahmed, S. S. Medany, M. D. Albaqami and A. Nafady, Biosensors, 2022, 12, 231 CrossRef CAS PubMed
. - H. Zhang, X. Ren, Z. Yang and J. Lai, Nutrients, 2022, 14, 4844 CrossRef CAS PubMed
. - M. Pereira, A. Dantas Damascena, L. M. Galvão Azevedo, T. de Almeida Oliveira and J. da Mota Santana, Crit. Rev. Food Sci. Nutr., 2022, 62, 1308–1316 CrossRef CAS PubMed
. - Y. Gu, Y. Li, Q. Wu, Z. Wu, L. Sun, Y. Shang, Y. Zhuang, X. Fan, L. Yi and S. Wang, Compr. Rev. Food Sci. Food Saf., 2023, 22(5), 4074–4106 CrossRef CAS PubMed
. - P. Sampathkumar, S. Sudalaimani, K. Giribabu and C. Suresh, J. Electrochem. Soc., 2021, 168, 087507 CrossRef CAS
. - Y. Yang, N. Hu, J. Deng and J. Yang, Chemosensors, 2022, 10, 494 CrossRef CAS
. - T. Anusha, K. S. Bhavani, J. S. Kumar, P. K. Brahman and R. Y. Hassan, Bioelectrochemistry, 2022, 143, 107935 CrossRef CAS PubMed
. - N. Sardar, U. B. Tariq, S. A. Khan, M. Haris and A. Rasheed, Vitamin D Detection Using Electrochemical Biosensors a comprehensive overview, IntechOpen, 2023 Search PubMed
. - A. Sanati, Y. Esmaeili, E. Bidram, L. Shariati, M. Rafienia, S. Mahshid and O. Parlak, Appl. Mater. Today, 2022, 26, 101350 CrossRef
. - A. Avan and H. Filik, Russ. J. Electrochem., 2021, 57, 858–871 CrossRef CAS
. - N. S. Prinith, J. G. Manjunatha, G. Tigari, Z. A. ALOthman, A. M. Alanazi and A. Pandith, ChemistrySelect, 2021, 6, 10746–10757 CrossRef CAS
. - C. Tortolini, F. Tasca, M. A. Venneri, C. Marchese and R. Antiochia, Chemosensors, 2021, 9, 229 CrossRef CAS
. - J. Ganesamurthi, R. Shanmugam and S.-M. Chen, J. Electrochem. Soc., 2022, 169, 096505 CrossRef CAS
. - R. Rajeev, L. Benny, M. Roy, A. T. Mathew, K. Akshaya, A. Varghese and G. Hegde, New J. Chem., 2022, 46, 4114–4125 RSC
. - T. Xu, W. Ji, Y. Zhang, X. Wang, N. Gao, L. Mao and M. Zhang, Angew. Chem., 2022, 134, e202204344 CrossRef
. - A. Abo-bakr, M. Abd-Elsabour and M. Abou-Krisha, Electroanalysis, 2021, 33, 2476–2489 CrossRef CAS
. - Y. Li, Z. Luo, G. Li, T. Belwal, L. Li, Y. Xu, B. Su and X. Lin, ACS Sens., 2021, 6, 1604–1612 CrossRef CAS PubMed
. - X. Liao, X. Luo, Y. Li, Y. Zhou, Q. Liang, K. Feng, M. B. Camarada and J. Xiong, Microchem. J., 2023, 190, 108671 CrossRef CAS
. - Q. Du, W. Wang, X. Zeng and X. Luo, Anal. Chim. Acta, 2023, 1239, 340674 CrossRef CAS PubMed
. - J. Guo, X. Liu, A. Wang, X. Yu and L. Ding, Microchem. J., 2022, 183, 107964 CrossRef CAS
. - A. Meng, X. Hong, Y. Zhang, J. Yin, L. Sheng and Z. Li, Microchem. J., 2021, 168, 106365 CrossRef CAS
. - H. Ajab, A. Yaqub, M. S. Nazir, M. Z. H. Rozaini and M. A. Abdullah, BioResources, 2020, 15, 6273–6281 CAS
. - A. M. Mahmoud, B. A. Alyami, M. H. Mahnashi, F. Alshareef, Y. S. Alqahtan and M. M. El-Wekil, Microchem. J., 2023, 187, 108419 CrossRef CAS
. - S. Lokesh Kumar, S. Kumar and K. K. Tetala, Microchim. Acta, 2023, 190, 345 CrossRef CAS PubMed
. - D. D. Khandagale and S.-F. Wang, New J. Chem., 2023, 47, 14933–14942 RSC
. - L. Huang, S. Tian, W. Zhao, K. Liu and J. Guo, Talanta, 2021, 222, 121645 CrossRef CAS PubMed
. - M. Cheelenahally, M. P. Sham Aan and S. M. Ujwal, J. Nanostruct., 2021, 11, 628–637 Search PubMed
. - P. Arul, N. Gowthaman, E. Narayanamoorthi, S. A. John and S.-T. Huang, J. Electroanal. Chem., 2021, 887, 115143 CrossRef CAS
. - M. Khalife, D. Stankovic, V. Stankovic, J. Danicka, F. Rizzotto, V. Costanche, A. Slama-Schwok and P. Gaudu, Food Chem., 2024, 433, 137316 CrossRef CAS PubMed
. - Z.-F. Zhou, X.-Z. Feng, T. Zhan, G.-C. Han, Z. Chen and H.-B. Kraatz, Anal. Bioanal. Chem., 2022, 414, 6791–6800 CrossRef CAS PubMed
. - D. F. Pereira, E. R. Santana and A. Spinelli, Microchem. J., 2022, 179, 107588 CrossRef CAS
. - V. R.-V. Montiel, J. R. Sempionatto, E. Vargas, E. Bailey, J. May, A. Bulbarello, A. Düsterloh, N. Matusheski and J. Wang, Biosens. Bioelectron., 2021, 194, 113590 CrossRef PubMed
. - J. Ahmed, M. Faisal, F. A. Harraz, M. Jalalah and S. A. Alsareii, J. Taiwan Inst. Chem. Eng., 2021, 125, 360–371 CrossRef CAS
. - T. Dodevska, D. Hadzhiev and I. Shterev, Micromachines, 2022, 14, 41 CrossRef PubMed
. - F. Bettazzi, C. Ingrosso, P. S. Sfragano, V. Pifferi, L. Falciola, M. L. Curri and I. Palchetti, Food Chem., 2021, 344, 128692 CrossRef CAS PubMed
. - J. Niranjana, H. Koyakutty, A. A. Thomas and M. J. Bushiri, Mater. Sci. Eng., B, 2023, 290, 116283 CrossRef CAS
. - M. Zayed and E. Y. Frag, Food Chem., 2023, 421, 136197 CrossRef PubMed
. - H. Lim, A. T. Jafry and J. Lee, Molecules, 2019, 24, 2869 CrossRef CAS PubMed
. - A. T. Jafry, H. Lee, A. P. Tenggara, H. Lim, Y. Moon, S.-H. Kim, Y. Lee, S.-M. Kim, S. Park and D. Byun, Sens. Actuators, B, 2019, 282, 831–837 CrossRef CAS
. - S. Nishat, A. T. Jafry, A. W. Martinez and F. R. Awan, Sens. Actuators, B, 2021, 336, 129681 CrossRef CAS
. - Q. Li, J. Zhang, Q. Li, G. Li, X. Tian, Z. Luo, F. Qiao, X. Wu and J. Zhang, Front. Mater., 2019, 5, 77 CrossRef
. - Y. Xing and P. S. Dittrich, Sensors, 2018, 18, 134 CrossRef PubMed
. - W. Kit-Anan, A. Olarnwanich, C. Sriprachuabwong, C. Karuwan, A. Tuantranont, A. Wisitsoraat, W. Srituravanich and A. Pimpin, J. Electroanal. Chem., 2012, 685, 72–78 CrossRef CAS
. - P. Duenchay, K. Kaewjua, O. Chailapakul and W. Siangproh, New J. Chem., 2020, 44, 9223–9229 RSC
. - M. R. Bailey, A. M. Pentecost, A. Selimovic, R. S. Martin and Z. D. Schultz, Anal. Chem., 2015, 87, 4347–4355 CrossRef CAS PubMed
. - A. R. Cherian, L. Benny, A. Varghese, N. S. John and G. Hegde, J. Electrochem. Soc., 2021, 168, 077512 CrossRef CAS
. - W. Ren, Y. Fang and E. Wang, ACS Nano, 2011, 5, 6425–6433 CrossRef CAS PubMed
. - H. Shen, Q. Li, W. Song and X. Jiang, Lab Chip, 2023, 23, 341–348 RSC
. - S. Battat, D. A. Weitz and G. M. Whitesides, Lab Chip, 2022, 22, 530–536 RSC
. - C. Zhu, Y. Bing, Q. Chen, B. Pang, J. Li and T. Zhang, ACS Appl. Mater. Interfaces, 2023, 15, 19384–19392 CrossRef CAS PubMed
. - M. Wagih, L. Balocchi, F. Benassi, N. B. Carvalho, J.-C. Chiao, R. Correia, A. Costanzo, Y. Cui, D. Georgiadou and C. Gouveia, IEEE J. Microw., 2022, 3, 193–226 Search PubMed
. - D. H. Keum, S.-K. Kim, J. Koo, G.-H. Lee, C. Jeon, J. W. Mok, B. H. Mun, K. J. Lee, E. Kamrani and C.-K. Joo, Sci. Adv., 2020, 6, eaba3252 CrossRef CAS PubMed
. - J. Theobald, A. Ghanem, P. Wallisch, A. A. Banaeiyan, M. A. Andrade-Navarro, K. Taskova, M. Haltmeier, A. Kurtz, H. Becker and S. Reuter, ACS Biomater. Sci. Eng., 2018, 4, 78–89 CrossRef CAS PubMed
. - A. Cusano, G. Quero, P. Vaiano, P. Cicatiello, M. Principe, A. Micco, M. Ruvo, M. Consales and A. Cusano, Biosens. Bioelectron., 2023, 242, 115717 CrossRef CAS PubMed
. - C.-S. Lee, Front. Sens., 2020, 1, 583035 CrossRef
. - F. Esposito, L. Sansone, A. Srivastava, A. M. Cusano, S. Campopiano, M. Giordano and A. Iadicicco, Sens. Actuators, B, 2021, 347, 130637 CrossRef CAS
.
|
This journal is © The Royal Society of Chemistry 2024 |