DOI:
10.1039/D3RA08055B
(Paper)
RSC Adv., 2024,
14, 4315-4323
Detoxification of corn stover prehydrolysate by different biochars and its effect on lactic acid fermentation
Received
24th November 2023
, Accepted 18th January 2024
First published on 31st January 2024
Abstract
During the utilization of lignocellulosic biomass such as corn stover, many by-products are produced in the pretreatment process that can severely inhibit the activity of microbes in the fermentation step. To achieve efficient biomass conversion, detoxification is usually required before microbial fermentation. In this study, the prehydrolysate from dilute acid pretreatment of corn stover was used as a lactic acid fermentation substrate. Biochars made from corn stover (CSB), cow manure (CMB), and a mixture of corn stover and cow manure (MB) were applied for the detoxification of the prehydrolysate. All three types of biochar had a porous structure with a specific surface area ranging from 4.08 m2 g−1 (CMB) to 7.03 m2 g−1 (MB). After detoxification, both the numbers of inhibitors and their concentrations in the prehydrolysate decreased, indicating that the biochars prepared in this study were effective in inhibitor removal. The concentration of lactic acid obtained from the prehydrolysate without detoxification was only 12.43 g L−1 after fermentation for 96 h with a productivity of 0.13 g (L h)−1. Although the specific area of CMB was the lowest among the three biochars, the CMB-treated prehydrolysate resulted in the highest lactic acid concentration of 39.25 g L−1 at 96 h with a productivity of 0.41 g (L h)−1. The lactic acid bacteria in the CMB-treated prehydrolysate grew faster than the other two biochars, reaching an OD value of 8.12 at 48 h. The results showed promise for the use of agricultural wastes to make biochar to increase the yield of lactic acid fermentation through the detoxification process.
Introduction
Lignocellulose is the most valuable carbon-based carrier available to replace fossil fuel materials. Lignocellulosic biomass, composed of 30–45% cellulose, 20–35% hemicellulose, and 10–20% lignin,1 is an excellent feedstock for lactic acid (LA), itself widely used in the agricultural, pharmaceutical, food, feed, and chemical industries, with a market value approximately 1.5 times that of ethanol.2 The use of sugars produced by the hydrolysis of corn stover for subsequent LA fermentation has advantages in terms of sustainability and availability. However, the complex structure of lignocellulose and the high structural stability resulting from the combination of the three components make it effective in resisting the hydrolysis of chemicals such as acids and alkalis as well as biological enzymes.3 Also, the high degree of polymerization and crystallinity of cellulose increases its resistance.4 Therefore, to obtain monosaccharides that can be used by microorganisms, lignocellulose must first be pretreated. Dilute acid pretreatment is one of the most widely used methods for pretreatment of lignocellulosic material with economic advantages.5 Dilute sulfuric acid pretreatment can dissolve most of the hemicellulose and a small fraction of the lignin and reduce the degree of polymerization and crystallinity of the solid fraction of cellulose, thus improving biomass utilization in subsequent processing.6 However, the dilute acid pretreatment of lignocellulose also produces a variety of degradation products, such as aldehydes, weak acids, aromatic compounds, etc., which can inhibit cell growth and metabolism of related enzymes and products.7 Therefore, studies on the detoxification of prehydrolysates are necessary.
Activated carbon (AC) is commonly used in waste water treatment to remove organic substances. It is also used in toxic compound removal in various area. The most common raw materials for commercial AC are coal, asphalt, wood, coconut shell, etc., of which coal is the most commonly used.8–10 However, coal is a non-renewable resource, so the development of other renewable adsorbents can alleviate the pressure on coal resources. Also, the preparation of AC usually goes through two steps: carbonization and activation. In industry, AC is generally treated by steam activation or chemical activation. The gas activation method needs to be carried out at a high pyrolysis temperature above 800 °C, which will consume a lot of energy.11 The use of chemical reagents in the chemical activation method not only increases the cost but also produces a large amount of waste liquid that needs to be disposed of. Biochar is very similar to AC in physical properties and applications. Compared to AC, biochar is an environmentally friendly adsorbent because it is produced by one step thermal decomposition process of renewable biomass and can be used for the removal of toxins from pre-treatment liquids due to its high specific surface area, rich pore structure, large number of surface functional groups, and high adsorption capacity.12 In short, both the raw material cost and the processing cost of biochar are much lower than AC. In recent years, biochar has been widely studied and used in various ways, such as in soil improvement, organic matter adsorption, and heavy metal adsorption because of its low cost, ease to obtain, high efficiency, and environmental protection.13–15 Biochar adsorption is a simple and effective method of detoxifying the prehydrolysates, as it is highly effective in removing inhibitors. This method has the advantage of being simple to operate and no other chemicals are needed.16 Activated biochar made from coconut shells was used for the detoxification of sugarcane bagasse pretreatment liquor, where it was highly selective for the removal of phenols and furan aldehydes.17 Flax shive biochar could adsorb the fermentation inhibitors furfural and hydroxymethylfurfural (HMF) from switchgrass acid hydrolysis and significantly reduce the lag phase of ethanol fermentation.18 Ethanol fermentation can be carried out after spruce charcoal detoxification of spruce wood pretreatment liquor.19 However, the application of biochar in the detoxification of prehydrolysate for the production of LA was rarely studied.
In this study, biochar made from corn stover (corn stover biochar: CSB), cow manure (cow manure biochar: CMB), and a mixture of corn stover and cow manure (mixed biochar: MB) were used for the detoxification of prehydrolysates obtained from pretreatment of corn stover by dilute sulfuric acid. Their performance was compared with commercial activated charcoal. The prehydrolysates were measured for compositional changes before and after the biochar detoxification, and their fermentation capacity was tested by lactic acid bacteria. The goal of this study is to investigate the feasibility of using biochar to improve the fermentability of biomass prehydrolysate for LA production. The behavior of biochar produced from different types of agro-waste was also compared in this work.
Materials and methods
Chemicals and microorganisms
Sulfuric acid, sodium hydroxide, and calcium carbonate were purchased from Sinopharm Chemical Reagent Company (China); MRS cultural medium, glucose, and yeast extracts were purchased from Sigma-Aldrich (China); activated carbon was purchased from Shanghai Aladdin Biochemical Technology Company (China).
The strain used for the LA fermentation was Lactobacillus pentosus (ATCC 8041). The Lactobacillus MRS broth was used as the medium for the seed culture, which contains 20 g per L glucose, 10 g per L peptone, 4 g per L yeast extract, 1.0 mL sorbitan mono-oleate, 2.0 g per L ammonium citrate tribasic, 5.0 g per L CH3COONa·3H2O, 0.2 g per L MgSO4·7H2O, 0.05 g per L MnSO4·4H2O, and 2.0 g per L K2HPO4. Lactic acid bacteria were incubated at 37 °C until the OD600 reached approximately 1.5 and then used as a seed culture. Although the ATCC 8041 strain can consume both glucose and xylose, the conversion of glucose to LA was the dominant metabolism for this strain. In this study, only the LA from glucose was quantified to determine the LA yield and prehydrolysate toxicity.
Biochar preparation
Corn stover was collected from the Shangzhuang Experimental Station of the China Agricultural University, and cow manure was obtained from the Jinyindao Ranch in Beijing, China. Corn stover was air-dried, and cow manure was oven-dried at 105 °C. Thereafter, they were crushed using a high-speed grinder (RT-34, Taiwan RongCong Precision Technology Company, China) to pass through a 20-mesh sieve, and stored in self-sealing bags at room temperature, respectively. Equal masses of corn stover and cow manure were mixed to prepare the substrate for mixed biochar. Three raw materials, corn stover, cow manure, and their mixture, were thermally treated at 500 °C for 1 h under a nitrogen flow of 100 mL min−1 at a heating rate of 10 °C min−1. Finally, these samples were cooled to room temperature under N2 flow. Biochar made from corn stover was named corn stover biochar (CSB); biochar made from cow manure was named cow manure biochar (CMB); biochar made from the mixture of corn stover and cow manure was named mixed biochar (MB).
Dilute acid pretreatment of corn stover
Corn stover with particle size between 20 and 40 mesh was collected for dilute sulfuric acid pretreatment. The corn stover was mixed with 1.0% (w/w) sulfuric acid solution at a solid-to-liquid ratio of 1
:
2.5 (w/v) in pressure-resistant bottles. These pressure-resistant bottles were then placed in an autoclave (Sanyo MLS-350, Japan) at 121 °C to carry out the acid pretreatment for 1 h. After the pretreatment, the bottles were cooled to room temperature, and the pretreated corn stover was then separated from the prehydrolysate by vacuum filtration. The pretreated corn stover was washed with DI water until near neutral pH, it was then dried and kept in sealed bags for future use. The prehydrolysate was collected and stored at 4 °C. The pretreatment solution was measured to contain 5.84 ± 0.30 g per L glucose and 64.16 ± 0.20 g per L xylose following the National Renewable Energy Laboratory (NREL, USA) analytical procedure.20 The sugars in oligomers form were determined according to the NREL analytical procedures and measured glucose oligosaccharides at 4.86 ± 0.58 g L−1 and xylo-oligosaccharides at 0.71 ± 0.22 g L−1.21
Detoxification of prehydrolysate
Detoxification of the prehydrolysate was carried out in flasks. The prehydrolysate was added to the flasks with 5% (w/v) CSB, CMB, and MB, respectively, and kept at room temperature and 200 rpm for 2 h. The biochar was separated by vacuum filtration and was dried in an oven at 60 °C. The detoxified liquid was adjusted to pH 6.0 using NaOH and the precipitate was removed by centrifugation, and the supernatant was taken for LA fermentation. The raw prehydrolysate solution without detoxification was used as a control group for LA fermentation and is denoted as UT (untreated) group.
Lactic acid fermentation
Fermentation was carried out in 50 mL serum bottles at 37 °C and 150 rpm for 120 h. Glucose and yeast extract were added to the prehydrolysate to the final concentration of 50 g L−1 and 15 g L−1, respectively. CaCO3 was supplemented at a dosage of 0.56 g per g-glucose to control the pH during fermentation. A pure glucose solution of 50 g L−1 with 15 g per L yeast extract was used as a control group and is denoted as G (glucose) group. The fermentation bottles were steam-sterilized at 121 °C for 15 min. After they were cooled to room temperature, the fermentation broth was added to it through a 0.22 μm sterile filter membrane. Each bottle containing fermentation broth was sealed with sterilized rubber stoppers and aluminum caps to maintain airtight conditions during the fermentation. A 10% (v/v) seed inoculum was then transferred into the medium. The bottles were kept sealed throughout the fermentation process, and samples were collected by syringe every 12 hours in the first 72 hours, and then every 24 hours to determine the sugar and LA content and the OD value.
HPLC and LCMS analysis
The concentrations of sugar and LA in the fermentation samples were quantified by HPLC (Waters e2695) with an RI detector (Waters 2414) equipped with an Aminex HPX-87H column (Bio-Rad, USA). The column temperature was 55 °C, the mobile phase was 5 mM sulfuric acid solution, and the flow rate was 0.6 mL min−1. The qualitative and quantitative analyses of inhibitors presented in the prehydrolysate were carried out by HPLC and LCMS and the detailed methods have been described earlier.22 The LA yield is calculated as follows:
Scanning electron microscopy (SEM) analysis
The surface morphology of biochar was analyzed using a SU-3500 scanning electron microscope (SEM; Hitachi, Japan). A suitable amount of sample was fixed on a carrier table with black conductive adhesive and sprayed with Au for 60 seconds. The accelerating voltage of the instrument was 15 kV during the test.
FTIR analysis
The functional groups in biochars before and after detoxification were detected using a Fourier transform infrared spectroscope (Spectrum 400; PerkinElmer; USA). Samples and spectroscopic KBr were mixed at a mass ratio of 1
:
100 and thoroughly ground in an agate mortar, then pressed using a tableting machine from a transparent sheet, which was placed onto the stage for scanning. Scanning wave numbers ranged from 400 cm−1 to 14
000 cm−1 with a resolution of 4 cm−1 and 64 scans. Each sample was measured in duplicates.
Biochar pore structure characterization
The thermal nitrogen desorption method was used for determining the specific surface area and pore sizes with the automatic analyzer (Nova 1000e, Quantachrome Instruments, America).23
Results and discussion
Biochar characterization before and after prehydrolysate detoxification
The biochar characteristic results are presented in Table 1. Overall, the specific surface area, pore size, and pore volume of biochars produced in this study were much smaller than that of activated carbon (AC). The specific surface areas of all biochars are smaller than 10 m2 g−1 while AC has a specific surface area of over 180 m2 g−1. The production of AC requires an activation step at elevated temperatures which gives it a high surface area. These results were in accordance with literature values. The surface area of corn stover biochar made by Chen et al. under 500 °C for 60 min is 4.56 m2 g−1, which is similar to the result in this study.24 Zhang et al. produced biochar from cow manure at 500 °C which has a surface area of 1.77 m2 g−1.25 Among the three biochar samples produced in this study, MB has the largest surface area of 7.03 m2 g−1, followed by CSB (5.18 m2 g−1), and CMB (4.08 m2 g−1). Differences in the raw material of biochar led to differences in pore characteristics after pyrolysis.26 The decomposition temperature of hemicellulose, cellulose, and lignin ranges from 200 °C to 500 °C,27 and the partially decomposed vacancies form pores. The crude fiber content of corn stover is higher than that of manure, so the specific surface area of CSB is larger. Studies have shown that co-pyrolysis may increase the specific surface area and the total pore volume of biochar compared with mixed biochar after separate pyrolysis.28 Vyas et al. found that the specific surface area of biochar obtained by co-pyrolysis of Pennsylvania coal and feed corn stover was larger than that obtained by separate pyrolysis of the two materials.29 This may be because there is a chemical interaction during the pyrolysis of the two materials, and the synergy in the mixture causes it to vaporize more easily.30,31 The pore sizes of all three biochars are very close to each other at around 3 nm and so are the pore volumes.
Table 1 Textural properties of the biochars before and after prehydrolysate detoxification
|
SBET (m2 g−1) |
Pore diameter (nm) |
Pore volume (cm3 g−1) |
Before |
After |
Before |
After |
Before |
After |
CSB |
5.18 |
1.49 |
3.63 |
1.20 |
0.013 |
0.007 |
CMB |
4.08 |
1.82 |
3.23 |
1.19 |
0.017 |
0.011 |
MB |
7.03 |
1.67 |
3.63 |
1.19 |
0.015 |
0.011 |
AC |
187.68 |
16.10 |
1.23 |
1.18 |
0.064 |
0.016 |
During the detoxification process, various inhibitor compounds were adsorbed onto the surface of biochar. So, the specific surface area, pore diameter, and pore volume of the biochar decreased remarkably after the detoxification. The specific surface areas of three biochars after detoxification decreased to smaller than 2.0 m2 g−1 with reductions of over 70%. The drop in AC's surface area was more significant indicating better inhibitor adsorption. The pore size and pore volume for biochars and AC showed similar trends after detoxification as shown in Table 1 with one exception, the pore diameter of AC almost did not change after detoxification. We do not have a clear explanation for this right now. One possible reason might be that the lower limit of detectable pore diameter in our case was around 1.00 nm. The pore diameter of AC was already as low as 1.23 nm, so the drop after adsorption was not clear.
The SEM of CSB, CMB, and MB before and after adsorption is shown in Fig. 1. The surface of CSB is flat and the pores are relatively uniform in size and orderly arranged. The surface of CMB is uneven and the shape of the stomata is disorderly. Many irregular pores can be seen in the particles, showing a porous or tubular structure with obvious delamination. This is due to the decomposition of organic matter in the pyrolysis process, which leaves many pores in the cellulose and lignin framework structure, causing the biomass to have a porous structure.32 After detoxification, there were clear precipitations on the surface of the biochar, indicating that inhibitors in the prehydrolysate adhered to the surface and pores of the biochar during detoxification treatment.
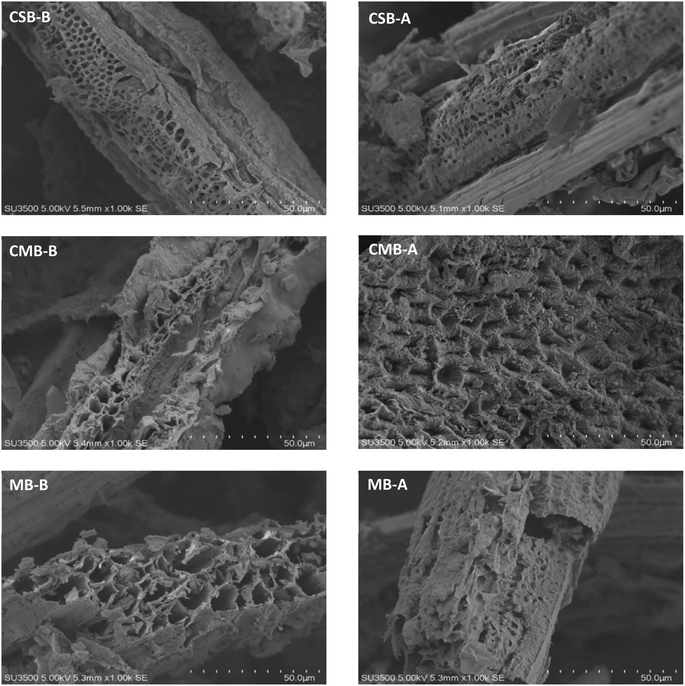 |
| Fig. 1 SEM images of biochars before (B) and after (A) adsorption. | |
The changes in the functional groups of biochar prepared from different raw materials before and after detoxification were analyzed by FTIR (Fig. 2). The absence of absorption peaks in the stretching vibration band of –OH (3500–3200 cm−1) and the stretching vibration of C–H in the aliphatic group (2930–2850 cm−1) indicates that the bound water and aliphatic components of biochars were decomposed at 500 °C.33 The bandwidth in the 1740–1700 cm−1 band is the C
O stretching vibration of carboxyl groups, aldehydes, ketones, and esters.34 The absorption peaks in this band of the three biochars were significantly shifted after adsorption as shown in Fig. 2, based on the principle of infrared spectroscopy testing, where a blue shift of the peak to a high wave number indicates an increase in the force constant of the chemical bond, and a redshift of the peak to a low wave number indicates a decrease in the force constant of the chemical bond. This indicates that there is a more stable bonding relationship between the adsorbent and the biochar functional groups. The stretching vibration for aromatic C
C and C
O (1598 cm−1) of CSB increased after adsorption, indicating that the adsorption efficiency of CSB for aromatic compounds was higher than that of the other two biochars.33,35 Peaks of CMB and MB at 798 and 750 cm−1 (the out-of-plane oscillating vibration of the C–H bond in the aromatic ring) weakened after absorption, indicating that biochar adsorbed polycyclic aromatic carbon rings during the detoxification process.36,37
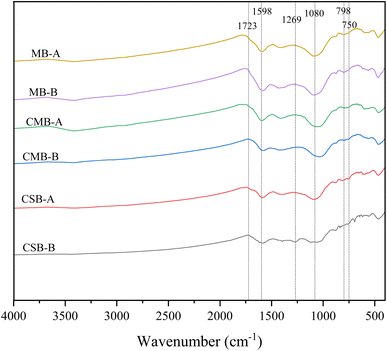 |
| Fig. 2 FTIR spectra of biochar (B-before; A-after). | |
Effect of biochar detoxification on prehydrolysates
The concentrations of sugars and four typical inhibitors (HMF, furfural, acetic acid, and vanillin) in the prehydrolysate were measured before and after detoxification (Table 2). Furfural, HMF, acetic acid, and vanillin are the most representative inhibitors in the dilute-acid prehydrolysate. They have been chosen as model inhibitory compounds in various literature.38–40 The ability to remove a single inhibitor by three biochars was almost the same (76–80% of HMF remained, 12–28% of furfural remained, and 82–95% of acetic acid remained) except for the CMB for Vanillin. The CMB removed 98% of vanillin in the prehydrolysate, while MB and CSB just reduced 6% and 44% of the vanillin in the prehydrolysate. The reduction of vanillin by CMB was even higher than AC. This might be one of the reasons why CMB showed the best improvement in the fermentability of prehydrolysate since vanillin was reported to be the most toxic of the four.41 Moreover, all three biochars had a lower acetic acid removal rate, which was no more than 20%. AC, as a widely used inhibitor removal agent, just eliminated 22% of acetic acid. It was reported that for lactic acid bacteria, acetic acid was less toxic than furfural and HMF.2 On the other hand, the biochar did not reduce the sugar concentration after detoxification, and the fermentable substrates were retained, which was beneficial for the subsequent LA fermentation. Lee and Do found that activated carbon could result in 8.9% and 24% sugar loss while efficiently removing inhibitors such as furfural and HMF.16,42 The specific surface area of AC is much larger than that of CMB, but the adsorption of biochar is not only related to the specific surface area and pore volume but also related to surface functional groups, surface minerals, surface electrical properties, etc., resulting in the best removal effect of CMB on vanillin, which is superior to AC.43 The AC, on the other hand, had a better physical adsorption effect due to its higher specific surface area and pore volume, making it more effective for the removal of all four inhibitors. In addition, the adsorption on the straw biochar is a single molecular layer chemisorbed,44 and the adsorption on the surface of the cow manure biochar is multi-layer heterogeneous adsorption rather than a single layer,25 which leads to the difference in the detoxification effect of the biochar.
Table 2 Relative concentrations of substances in prehydrolysates after detoxificationa
|
Glucose |
Xylose |
HMF |
Furfural |
Acetic acid |
Vanillin |
Assuming a concentration of 100 in the untreated solution. |
UT |
100 |
100 |
100 |
100 |
100 |
100 |
CSB |
100 |
100 |
76 |
12 |
82 |
56 |
CMB |
102 |
100 |
77 |
28 |
95 |
2 |
MB |
102 |
100 |
80 |
13 |
89 |
94 |
AC |
99 |
99 |
19 |
0 |
78 |
4 |
The potential inhibitors in the prehydrolysate were also determined by LC/MS as shown in Fig. 3. Both CMB and AC can effectively remove the inhibitors in the hydrolysate. Not only the numbers of inhibitors but also their concentrations in the prehydrolysate decreased, indicating that biochars prepared in this study were effective in inhibitor removal.
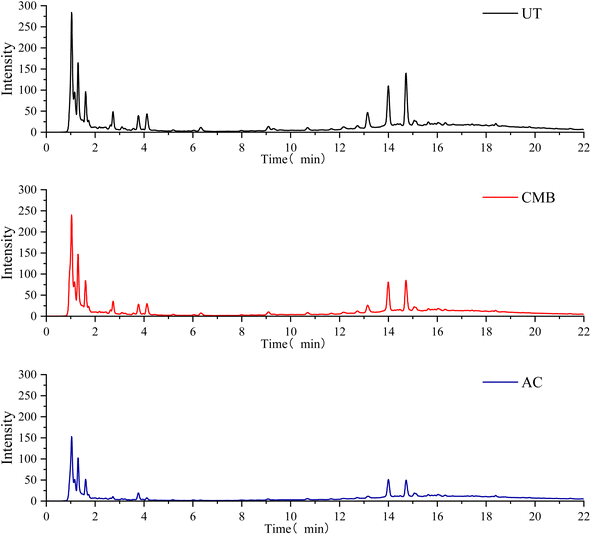 |
| Fig. 3 LC spectrum of prehydrolysate before and after detoxification. | |
Effect of different biochar detoxification on lactic acid fermentation
The fermentability of detoxified prehydrolysate was tested by carrying out LA fermentation. The growth and metabolism of the lactic acid bacteria were significantly inhibited in the non-detoxified prehydrolysate (denoted as UT: untreated) compared to the control group of glucose solution (denoted as G: glucose). The glucose in the control group was completely consumed by the lactic acid bacteria within 48 h (Fig. 4). However for the UT group, only 16% of the glucose was used by the lactic acid bacteria at 48 h, and the LA concentration was only 8.31 g L−1. After biochar detoxification, the fermentation performance of the prehydrolysate was significantly improved. The use of three biochar increased the LA concentration at 120 h from 18.69 g L−1 (UT) to 43.15 g L−1 (CMB), 40.68 g L−1 (MB), and 23.24 g L−1 (CSB), respectively. The fermentation result of AC after detoxification was better than that of biochar, which reached 45.88 g L−1 in 72 h. Among the 3 biochars, CMB showed the best detoxification effect, which gave an LA concentration of 32.31 g L−1 at 72 h and 43.15 g L−1 at 120 h (0.45 g (L h)−1 and 0.36 g (L h)−1). This fermentation result is in agreement with the data in Table 2, better inhibitor removal resulted in better fermentation. The CMB had the best overall inhibitor removal rate compared to CSB and MB, especially in the case of vanillin. Studies have shown that vanillin is one of the most toxic inhibitors for microbes.41 The prehydrolysate after MB treatment showed the 2nd best performance with a LA concentration of 40.68 g L−1 at 120 h of fermentation. Although the final LA concentration from MB detoxification was close to the CMB group, CMB detoxification surpassed the MB group at the early-stage fermentation up to 72 h (Fig. 4 and 5). The fermentability of CSB-treated prehydrolysate was much lower than expected. The removal rate of CSB on the inhibitors was higher than that of MB for all four inhibitors (Table 2), but the LA production was almost halved. After 120 h of fermentation, the CSB group only had 23.24 g L−1 LA. The inferior performance of CSB detoxification might be attributed to undetected inhibitors which were not included in Table 2. MB may have stronger adsorption effect on undetected inhibitors. AC and CMB treatments produce the most LA in the second stage, accounting for 53.37% and 34.95% of the final LA concentration, MB in the fourth stage, and CMB and UT in the last stage (Fig. 4). It can be seen that the LA production rate changes significantly during the fermentation process in all different experimental groups (Fig. 4 and 5). Even in the AC treatment group, the fermentation rate was low in the first 12 h, yet increased significantly after. The LA productivity was low until 96 h. The increase in productivity in the case of UT and CSB group after 96 h of fermentation may be because the microorganisms were slowly adapted to the toxic environment.45 In addition, when biochar is mixed with pre-hydrolysate, biochar not only absorbs substances in the liquid, but also releases other substances, such as minerals and metal ions.46,47 The concentration of minerals and metal ions leaching from the CMB in the liquid is higher,48 so the prehydrolysate treated with CMB and MB will also have more minerals, which is beneficial for the growth of microorganisms. The detoxification performance of biochar prepared in this study is compared with literature results in Table 3. Most literature studies on the biochar or AC detoxication of prehydrolysate were for ethanol or butanol production, thus a direct comparison between this work and the literature results is hard to perform. But the overall effect of detoxification on microbes are similar.
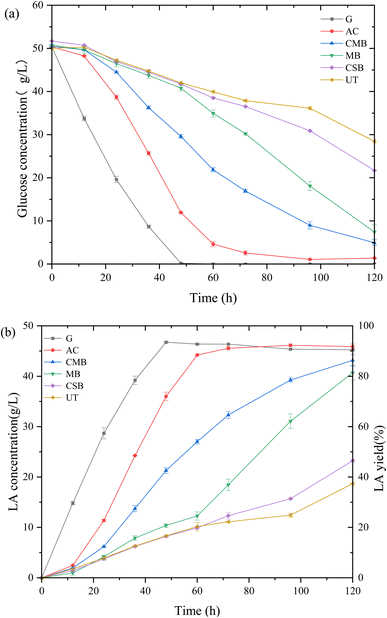 |
| Fig. 4 LA fermentation results of prehydrolysate with different biochar detoxification. (a) Glucose consumption rate changes with time. (b) Lactic acid concentration and yield change with time. | |
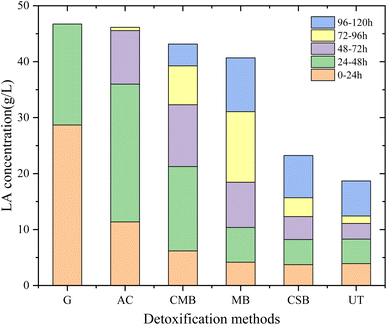 |
| Fig. 5 Changes of LA concentration in different periods after detoxification with different biochar. | |
Table 3 Studies on detoxification of prehydrolysate by biochar
Adsorbent |
Inhibitor removal |
Fermentation product |
Fermentation result |
Reference |
Wood charcoals |
100% furfural, HMF, vanillin |
Ethanol |
Ethanol concentration increased from 0 to about 9 g L−1 |
19 |
Flax shive activated biochar |
90% furfural, 60% HMF |
Ethanol |
Ethanol concentration increased from 1 g L−1 to 31 g L−1 |
18 |
Acai berry biochar |
53% furfural, 100% HMF, 40% acetic acid |
Ethanol |
Cell concentration increased from 23.57 g L−1 to 28.07 g L−1 |
16 |
Activated charcoal |
— |
Ethanol |
Ethanol concentration increased from 3.46 g L−1 to 7.43 g L−1 |
49 |
Charcoal |
93% furfural, 99% HMF, 52% acetic acid |
ABE |
Product concentration increased from 0 to 7.83 g L−1 |
50 |
Corn stover biochar |
Up to 88% furfural, 24% HMF, 18% acetic acid, 98% vanillin |
Lactic acid |
Lactic acid concentration increased from 18.69 g L−1 to 23.24 g L−1, 43.15 g L−1, and 40.68 g L−1, respectively |
This study |
Cow manure biochar |
Mixed biochar |
The measures of OD confirmed the effect of biochar detoxification on prehydrolysate suitability for LA fermentation (Fig. 6). The OD value of the glucose control group rapidly reached 6.07 at 12 h and increased to 9.43 at 60 h. The OD of bacteria in AC-treated prehydrolysate exceeded that of glucose at 36 h and reached its maximum value of 10.41 at 48 h. However, a clear lag period could be observed for the AC group in the first 12 h, indicating that the activated carbon detoxification did not completely remove the inhibitory effect of the substances in the prehydrolysates. The OD value of the CMB group reached its maximum of 8.12 at 48 h which was similar to the glucose control. But the OD value of the CSB and MB groups did not reach the maximum after 72 h, and the maximum value of the MB group was slightly higher than that of the CSB group. The trend of OD value over time was consistent with that of LA concentration, which was AC > CMB > MB > CSB. Even though biochar is less efficient than activated carbon in removing pretreatment byproducts, they have the advantage of being less costly because they do not require an activation step.39
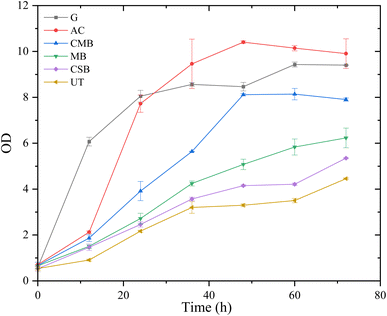 |
| Fig. 6 OD values during lactic acid fermentation. | |
Conclusion
Biochars made from agro-waste were proven to be effective in the detoxification of the prehydrolysate generated from dilute-acid pretreatment of corn stover. Three types of biochar prepared in this study could efficiently remove furfural from the prehydrolysate but have limited adsorption on HMF and acetic acid. Cow manure biochar showed the best inhibitor removal rate, especially for vanillin, and the highest LA yield among the three biochars. Although the removal of major inhibitors in the prehydrolysate was not as good as expected, the detoxification with biochars improved the fermentability of prehydrolysate. As a result, biochar can be used as a low-cost adsorbent for prehydrolysate detoxification. The detailed mechanism of the different performances of three types of biochar could be further studied. To improve the economic competitiveness of biochar, further research on the reuse of biochar is needed.
Author contributions
Chun Wang and Yu Shan performed the experiments, analyzed the data, and prepared the first draft of this manuscript. Yuli Shen and Weng Fu helped in method development and manuscript writing. David Blersch edited and revised the manuscript. Wei Wu was in charge of validation. Suan Shi and Lujia Han were the supervisors for this research project.
Conflicts of interest
There are no conflicts to declare.
Acknowledgements
This study was supported by the National Key R&D Program of China (2022YFD2002103, 2022YFD1300602); the Innovative Research Team in University of Education and the Ministry of China (IRT_17R105); the Earmarked Fund for CARS36; and the Chinese Universities Scientific Fund (2023TC082).
References
- A. Malinowski and D. Wardzińska, Chemik, 2012, 66(9), 982–990 CAS.
- X. Ma, M. Gao, S. Liu, Y. Li, X. Sun and Q. Wang, Bioresour. Technol., 2022, 352, 127108 CrossRef CAS PubMed.
- M. J. Taherzadeh and K. Karimi, Int. J. Mol. Sci., 2008, 9, 1621–1651 CrossRef CAS PubMed.
- M. Nathan, W. Charles, D. Bruce, E. Richard, Y. Y. Lee, H. Mark and L. Michael, Bioresour. Technol., 2005, 96, 673–686 CrossRef PubMed.
- M. Brienzo, Y. Abud, S. Ferreira, R. C. N. R. Corrales, V. S. Ferreira-Leitao, W. de Souza and C. Sant'Anna, Ind. Crops Prod., 2016, 84, 305–313 CrossRef CAS.
- V. B. Agbor, N. Cicek, R. Sparling, A. Berlin and D. B. Levin, Biotechnol. Adv., 2011, 29, 675–685 CrossRef CAS PubMed.
- L. Zhang, X. Li, Q. Yong, S.-T. Yang, J. Ouyang and S. Yu, Bioresour. Technol., 2016, 203, 173–180 CrossRef CAS PubMed.
- J. Zhou, S. Hao, L. Gao and Y. Zhang, Ann. Nucl. Energy, 2014, 72, 237–241 CrossRef CAS.
- S. Liu, Y. Zhang, M. Xue, X. Cui, B. Li and J. Li, Environ. Eng., 2021, 39, 79–89 Search PubMed.
- L. Zhong, Y. Zhang, Y. Ji, P. Norris and W.-P. Pan, J. Therm. Anal. Calorim., 2016, 123, 851–860 CrossRef CAS.
- J. Jiang, Y. Liu, Z. Zhou, J. Duan and D. Sun, Chem. Ind. For. Prod., 2017, 37, 1–10 Search PubMed.
- A. Shaaban, S. M. Se, N. M. M. Mitan and M. F. Dimin, Procedia Eng., 2013, 68, 365–371 CrossRef CAS.
- M. Li, Z. Zhang, Z. Li and H. Wu, Ecol. Eng., 2020, 149, 105792 CrossRef.
- Y. Dai, N. Zhang, C. Xing, Q. Cui and Q. Sun, Chemosphere, 2019, 223, 12–27 CrossRef CAS PubMed.
- N. You, J.-Y. Li, H.-T. Fan and H. Shen, J. Adv. Res., 2019, 15, 77–86 CrossRef CAS PubMed.
- B. F. do Nascimento, C. M. Bezerra de Araujo, A. C. do Nascimento, F. L. Honorato da Silva, D. J. Nobrega de Melo, E. F. Jaguaribe, J. V. F. L. Cavalcanti and M. A. d. M. Sobrinho, J. Hazard. Mater., 2021, 409, 124494 CrossRef CAS PubMed.
- J. V. Freitas, F. G. E. Nogueira and C. S. Farinas, Ind. Crops Prod., 2019, 137, 16–23 CrossRef CAS.
- K. T. Klasson, B. S. Dien and R. E. Hector, Ind. Crops Prod., 2013, 49, 292–298 CrossRef CAS.
- H. Miyafuji, H. Danner, M. Neureiter, C. Thomasser, J. Bvochora, O. Szolar and R. Braun, Enzyme Microb. Technol., 2003, 32, 396–400 CrossRef CAS.
- A. Sluiter, B. Hames, R. O. Ruiz, C. Scarlata and D. Templeton, Enzyme Microb. Technol., 2004, 396–400 Search PubMed.
- A. Sluiter, B. Hames, R. Ruiz, C. Scarlata, J. Sluiter and D. Templeton, Determination of sugars, byproducts, and degradation products in liquid fraction process samples, Golden: National Renewable Energy Laboratory, 2006, vol. 11, pp. 65–71 Search PubMed.
- P. Persson, J. Andersson, L. Gorton, S. Larsson, N. O. Nilvebrant and L. J. Jönsson, J. Agric. Food Chem., 2002, 50, 5318–5325 CrossRef CAS PubMed.
- O. Cheremisina, T. Litvinova, V. Sergeev, M. Ponomareva and J. Mashukova, Water, 2021, 13, 3101 CrossRef CAS.
- F. Chen, Y. Sun, C. Liang, T. Yang, S. Mi, Y. Dai, M. Yu and Q. Yao, Sci. Rep., 2022, 12, 17714 CrossRef CAS PubMed.
- P. Zhang, Y. Li, Y. Cao and L. Han, Bioresour. Technol., 2019, 285, 121348 CrossRef CAS PubMed.
- Y. Xin, H. Cao, D. Wang, Q. Yuan and Y. Yang, Trans. Chin. Soc. Agric. Mach., 2015, 46, 190–195 CAS.
- T. Xie, K. R. Reddy, C. Wang, E. Yargicoglu and K. Spokas, Crit. Rev. Environ. Sci. Technol., 2015, 45, 939–969 CrossRef CAS.
- Y. Gao, Z. Jiang, J. Li, W. Xie, Q. Jiang, M. Bi and Y. Zhang, Environ. Res., 2019, 172, 561–568 CrossRef CAS PubMed.
- A. Vyas, T. Chellappa and J. L. Goldfarb, J. Anal. Appl. Pyrolysis, 2017, 124, 79–88 CrossRef CAS.
- P. Yangali, A. M. Celaya and J. L. Goldfarb, J. Anal. Appl. Pyrolysis, 2014, 108, 203–211 CrossRef CAS.
- A. O. Aboyade, J. F. Goergens, M. Carrier, E. L. Meyer and J. H. Knoetze, Fuel Process. Technol., 2013, 106, 310–320 CrossRef CAS.
- A. Janus, A. Pelfrene, S. Heymans, C. Deboffe, F. Douay and C. Waterlot, J. Environ. Manage., 2015, 162, 275–289 CrossRef CAS PubMed.
- A. R. A. Usman, A. Abduljabbar, M. Vithanage, Y. S. Ok, M. Ahmad, M. Ahmad, J. Elfaki, S. S. Abdulazeem and M. I. Al-Wabel, J. Anal. Appl. Pyrolysis, 2015, 115, 392–400 CrossRef CAS.
- Y. Wang, Y. Hu, X. Zhao, S. Wang and G. Xing, Energy Fuels, 2013, 27, 5890–5899 CrossRef CAS.
- X. Gai, H. Wang, J. Liu, L. Zhai, S. Liu, T. Ren and H. Liu, PLoS One, 2014, 9, e113888 CrossRef PubMed.
- Q.-x. Lin, C.-h. Zhang, X.-h. Wang, B.-g. Cheng, N. Mai and J.-l. Ren, Catal. Today, 2019, 319, 31–40 CrossRef CAS.
- J. R. Kastner, J. Miller, D. P. Geller, J. Locklin, L. H. Keith and T. Johnson, Catal. Today, 2012, 190, 122–132 CrossRef CAS.
- Z. Qiang, Z. Li and A. B. Thomsen, Nongye Jixie Xuebao, 2012, 43, 108–111 Search PubMed.
- F. Monlau, C. Sambusiti, N. Antoniou, A. Zabaniotou, A. Solhy and A. Barakat, Bioresour. Technol., 2015, 187, 379–386 CrossRef CAS PubMed.
- J. V. Freitas, L. A. M. Ruotolo and C. S. Farinas, Fuel, 2019, 251, 1–9 CrossRef CAS.
- J. Li, C. Zhu, M. Tu, P. Han and Y. Wu, Appl. Biochem. Biotechnol., 2015, 175, 3657–3672 CrossRef CAS PubMed.
- J. M. Lee, R. A. Venditti, H. Jameel and W. R. Kenealy, Biomass Bioenergy, 2011, 35, 626–636 CrossRef CAS.
- W. Song and M. Guo, J. Anal. Appl. Pyrolysis, 2012, 94, 138–145 CrossRef CAS.
- J. Xu, Y. Ma, G. Yao, Y. Zhang, L. Yang, N. Zhou and S. Fan, Environ. Sci., 2022, 43, 5635–5646 Search PubMed.
- H. Gu, J. Zhang and J. Bao, Bioresour. Technol., 2014, 157, 6–13 CrossRef CAS PubMed.
- X. Sun, H. K. Atiyeh, Y. A. Adesanya, H. Zhang and T. Ezeji, Bioresour. Technol., 2019, 6–13 CAS.
- W.-t. Wang, L.-c. Dai, B. Wu, B.-f. Qi, T.-f. Huang, G.-q. Hu and M.-x. He, Biotechnol. Biofuels, 2020, 13, 28 CrossRef CAS PubMed.
- X. Sun, H. K. Atiyeh, Y. Adesanya, C. Okonkwo, H. Zhang, R. L. Huhnke and T. Ezeji, Bioresour. Technol., 2020, 298, 122569 CrossRef CAS PubMed.
- A. K. Chandel, R. K. Kapoor, A. Singh and R. C. Kuhad, Bioresour. Technol., 2007, 98, 1947–1950 CrossRef CAS PubMed.
- N. K. N. Al-Shorgani, A. I. Al-Tabib and M. S. Kalil, Bioresources, 2017, 12, 8505–8518 CAS.
|
This journal is © The Royal Society of Chemistry 2024 |
Click here to see how this site uses Cookies. View our privacy policy here.