DOI:
10.1039/D3RA07607E
(Paper)
RSC Adv., 2024,
14, 4518-4532
SiO2@AuAg/PDA hybrid nanospheres with photo-thermally enhanced synergistic antibacterial and catalytic activity†
Received
7th November 2023
, Accepted 3rd January 2024
First published on 2nd February 2024
Abstract
Wastewater discharged from industrial, agricultural and livestock production contains a large number of harmful bacteria and organic pollutants, which usually cause serious harm to human health. Therefore, it is urgent to find a “one-stone-two-birds” strategy with good antimicrobial and pollutant degradation activity for treating waste water. In this paper, SiO2@AuAg/Polydopamine (SiO2@AuAg/PDA) core/shell nanospheres, which possessed synergistic “Ag+-release–photothermal” antibacterial and catalytic behaviors, have been successfully prepared via a simple in situ redox polymerization method. The SiO2@AuAg/PDA nanospheres showed good catalytic activity in reducing 4-nitrophenol to 4-aminophenol (0.576 min−1 mg−1). Since the AuAg nanoclusters contain both gold and silver elements, they provided a high photothermal conversion efficiency (48.1%). Under NIR irradiation (808 nm, 2.5 W−2), the catalytic kinetics were improved by 2.2 times. Besides the intrinsic Ag+-release, the photothermal behavior originating from the AuAg bimetallic nanoclusters and the PDA component of SiO2@AuAg/PDA also critically improved the antibacterial performance. Both E. coli and S. aureus could be basically killed by SiO2@AuAg/PDA nanospheres at a concentration of 90 μg mL−1 under NIR irradiation. This “Ag+-release–photothermal” coupled sterilization offers a straightforward and effective approach to antimicrobial therapy, and further exhibits high potential in nanomedicine for combating bacterial contamination in environmental treatment and biological fields.
1. Introduction
Over the past decade, the water pollution caused by the effects of industrial, agricultural and livestock production and urban residential water use has become increasingly prominent, and the accumulation of harmful bacteria and pollutants in wastewater poses a serious threat to people’s lives.1–4 To treat the bacteria, antibiotics are often overused; hence, the emergence and spread of super-resistant bacteria have caused a series of therapeutic problems.5–7 In recent years, nanoplatforms have been widely investigated and demonstrated to be effective in the fields of nanocatalysis and antimicrobials. Among them, noble-metal nanocrystals (Pd, Au, Ag, Pt and Ce) have attracted more and more attention due to their simple synthesis, excellent photothermal conversion capability, good catalytic activity, and significant antimicrobial properties.8–11 Due to their large surface area, noble-metal bimetallic nanocrystals have been widely used in the degradation of organic pollutants.12–15 Therefore, nanoplatforms integrated with noble-metal nanocrystals possess great potential in both nanocatalysis and antimicrobials.
The organic pollutant 4-nitrophenol is carcinogenic, teratogenic, and mutagenic, and its reduction product (4-aminophenol) is widely used in a variety of applications, such as anticorrosive lubrication and corrosion inhibition, because of its low toxicity. Therefore, it is necessary to convert 4-nitrophenol to 4-aminophenol. Noble-metal nanoparticles (Au, Ag, Cu, Pt and Pd) have been commonly applied as nanocatalysts to address this problem. Liao et al. investigated the reduction of 4-nitrophenol by three efficient silver-based nanoparticle catalysts and found that smaller silver-nanoparticle sizes and sharper edge and corner contributed to an increase in the total surface area, which improved the catalytic performance.16 Deshmukh et al. investigated and compared the effect of gold nanoparticles (AuNPs) and gold nanoflowers (AuNFs) on the reduction of 4-nitrophenol. The results showed that the AuNFs’ catalytic activity was 1.57 times higher than that of AuNPs.17 Moreover, noble-metal nanoparticles exhibit significantly enhanced catalytic activity and selectivity due to synergistic effects as well as in situ formation of reactive species.18,19 It has been reported that a bimetallic structure facilitates the adsorption of reactants and the release of products, thus improving the catalytic performance.20 In addition, Ag ions are known to have broad-spectrum antimicrobial activity and they can bind to the catabolic metabolite control proteins of Staphylococcus aureus, inducing oligomerization as well as inhibiting the ability to bind to DNA.21 Therefore, Ag-doped bimetallic nanocrystals have broad application prospects in both antimicrobials and nanocatalysis.
Photothermal therapy (PTT) has received increasing attention as an effective, novel, non-invasive therapeutic technique that shows great potential in the treatment of drug-resistant bacteria and bacterial biofilms.22–24 It is well-known that Au-based nanocrystals have excellent photothermal conversion effects.25–27 In addition, noble-metal nanostructures have high photothermal conversion efficiency due to their good near-infrared absorption properties in the localized surface plasmon resonance region.28,29 As a result, various noble-metal nanoparticles with synergistic photothermal antimicrobial performance have been explored. Furthermore, a previous report indicated that bimetallic nanocrystals had stronger antimicrobial effects than monometallic ones.30 Kim found that Ag nanoparticles in cobalt–silver bimetallic nanocomposites exhibited strong photothermal conversion efficiency, which synergized with Ag and Co ions to achieve a significant antimicrobial effect.31 Although Ag+ is effective in killing bacteria as a broad-spectrum antimicrobial agent, the severe biotoxicity also limits its application. Therefore, a bio-safe nanoplatform that not only balances the biocompatibility and toxicity but also possesses controllable photothermal antibacterial behavior is highly required. Polydopamine (PDA) possesses extraordinary adhesive properties, good biocompatibility, and excellent photothermal conversion ability. During the past decades, PDA has been used to carry noble-metal nanoparticles and this method not only solves the agglomeration problem of nanocrystals but also effectively promotes the photothermal antimicrobial effect.32–35
Another strategy to the prevent agglomeration of noble-metal nanoparticles is to immobilize them on a carrier. It is worth noting that SiO2 nanospheres have the advantages of high dispersibility, good chemical stability, easy modification, and high temperature resistance, which make them a non-toxic and non-polluting green material. Due to its optical transparency and biocompatibility, SiO2 has a wide range of applications in the chemical industry, biomedicine and optoelectronic devices. SiO2@Au and Au@SiO2 nanoparticles have been reported to enhance the fluorescence of rhodamine B dye in the presence of AuNPs.36 Zhang et al. reported that SiO2 loaded with Pd–Cu bimetallic nanoparticles exhibited good activity and selectivity under mild conditions.37 Similarly, metal–organic frameworks (MOFs) are a class of porous crystalline hybrid materials, and these ordered crystal structures have highly desirable physicochemical properties, such as tunable pore size, high surface area, open metal sites and large void space.38,39 As far as applications are concerned, MOFs have a wide range of prospects in numerous fields, such as drug delivery and catalysis. To this end, the construction of bimetallic nanoplatforms based on SiO2 or MOF carriers and investigation of their relative catalytic and antibacterial performances have become attractive.
In this study, we report a simple synthesis method for a SiO2@AuAg/PDA nanocomposite in which the gold and silver bimetallic nanoparticles are sandwiched between SiO2 and PDA. The content of gold and silver, as well as the thickness of the shell layer, can be controlled by changing the experimental conditions. Such noble-metal nanoparticles have catalytic and antimicrobial properties. Taking 4-nitrophenol as an example, we performed kinetic analysis experiments on the nanospheres by catalyzing its conversion to 4-aminophenol and found that the reduction effect was more significant under near-infrared irradiation. In addition, the SiO2@AuAg/PDA composite was effective in the sterilization of Gram-negative (Escherichia coli, E. coli) and Gram-positive (Staphylococcus aureus, S. aureus) bacteria under NIR irradiation, and showed a typical “photothermal–Ag+-release” coupled antibacterial effect. This study provides a multifunctional composite material with both bactericidal and catalytic properties, which has promising applications in environmental treatment and biology.
2. Materials and methods
2.1 Materials
2-Methylimidazole (C4H6N2) was purchased from Hebei Bailing Wei Ultrafine Materials Co. 3-Aminopropyltriethoxysilane (APTES) and trisodium citrate dihydrate (C6H5Na3O7·2H2O) were supplied by China Aladdin Chemical Co. Cetyltrimethylammonium bromide (CTAB), zinc acetate dihydrate (C4H6O4Zn·2H2O), sodium borohydride (NaBH4), 4-nitrophenol (C6H5NO3), ammonia (NH3·H2O), tetraethyl orthosilicate (TEOS), gold(III) chloride (HAuCl4), and silver nitrate (AgNO3) were purchased from China National Pharmaceutical Chemical Reagent Co. No experimental reagents were further purified and ultrapure water was used for the experiments.
2.2 Synthesis of SiO2@AuAg/PDA core/shell nanospheres
Synthesis of SiO2 nanospheres. Silica nanospheres were prepared via a modified Stöber method.40 First, a round-bottom flask was filled with 50 mL ethanol, 4.9 mL distilled water and 9.3 mL ammonia, and the contents stirred at 30 °C for 30 min. Then, 5 mL TEOS was added and the reaction was carried out. 12 h later, the product was separated from the suspension, and washed with water and ethanol alternately three times to obtain SiO2 nanospheres. Finally, SiO2 nanosphere powder was obtained via vacuum drying.
Synthesis of SiO2@AuAg/PDA core/shell nanospheres. Firstly, SiO2 nanospheres (8.5 mg) were ultrasonically dispersed in 50 mL ethanol to obtain a homogeneous solution and a drop of APTES was added. Then, freshly prepared AgNO3 solution (0.02 g mL−1, 136 μL), HAuCl4 (0.1 g mL−1, 40 μL) and sodium citrate (15 mg) were added to form solution (1). Secondly, DA-HCl (30 mg) was added to 30 mL Tris (pH = 8.5) to form solution (2). Solution (2) was then mixed with solution (1) and the reaction was conducted under ultra-sonication. After 3 h, the product was washed with ethanol and purified water alternately three times, followed by vacuum drying at 45 °C for 12 h to obtain SiO2@AuAg/PDA nanospheres.
Synthesis of ZIF-8@AuAg/PDA nanospheres. First, 14.4 g of Zn(CH3COO)2·2H2O and 0.0384 g CTAB were dissolved in 240 mL of water, sealed and sonicated. Next, 140 mL of aqueous 2-methylimidazole (0.38 g mL−1) was added. The solution turned into a milky white liquid under heating conditions in a water bath at 25 °C for 2 hours, then it was centrifuged (9000 rpm, 5 min). Finally, the solution was washed with anhydrous ethanol more than 3 times and dried in a vacuum oven for 12 h to obtain ZIF-8 nanocubes. For the preparation of ZIF-8@AuAg/PDA, ZIF-8 (10 mg) was sonicated in 40 mL ethanol for 2 h. Then, 40 μL chloroauric acid, trisodium citrate and 136 μL of a ready-made Ag ion solution were added and sonicated. Then, 30 mL Tris buffer (pH = 8.5) mixed with 30 mg of DA was added and the reaction was carried out for 3 h. Finally, ZIF-8@AuAg/PDA could be obtained by centrifugation, washing with ethanol and vacuum drying at 45 °C for 12 h.
2.3 Photothermal effect of SiO2@AuAg/PDA
SiO2@AuAg/PDA nanospheres were dispersed in deionized water at a concentration of 1 mg mL−1 and diluted into a gradient of five concentrations (20, 40, 60, 80 and 100 μg mL−1). The respective absorbances of the diluted suspensions were then measured at 808 nm. Then, 1 mL SiO2@AuAg/PDA at different concentrations was taken in a centrifuge tube (1.5 mL) and continuously irradiated with a near-infrared laser (808 nm, 2.5 W cm−2) for 6 min, and the temperature at different time points was recorded with an infrared camera. In order to evaluate the light absorption capacity of the nanocatalysts at 808 nm, the extinction coefficient ε(λ) was determined according to the Lambert–Beer law:where A is the absorbance at λ (808 nm), L is the optical length (1 cm), and C is the concentration of the aqueous SiO2@AuAg/PDA solution (μg mL−1). The extinction coefficient ε was calculated by plotting the slope (L g−1 cm−1) of each linear fit versus wavelength. In addition, the photothermal conversion efficiency was calculated as follows:41 |
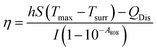 | (2) |
The value of hS is obtained using the formula m·Cp = hS·τS, where m is the volume of the solution, Cp is the specific heat of water and τS refers to a time constant of sample system. Tmax and Tsurr are the maximum and room temperatures, respectively, while QDis the heat dissipation of the light absorption of the sample itself. I is the laser power and A808 is the absorbance value of the sample at 808 nm.
2.4 Catalytic activity of SiO2@AuAg/PDA nanospheres for the reduction of 4-nitrophenol
First, 50 mL of aqueous 4-nitrophenol (10−4 M) and 50 mg NaBH4 were added to a beaker (100 mL), and then SiO2@AuAg/PDA nanospheres were added to the mixture. To monitor the reaction, 2 mL of the reaction solution was quickly removed with a syringe at regular intervals and the nanocatalysts were separated from the reaction solution by injecting them into a centrifuge tube through a pressurized syringe filter to stop the reaction. The conversion of 4-nitrophenol was then detected by UV spectrophotometry and the characteristic absorption at 400 nm was monitored. After the reaction, the nanocatalyst was separated from the reaction system by suction filtration and reused in subsequent catalytic reactions. We studied the reaction kinetics of the catalytic 4-nitrophenol degradation reaction. Typically, catalytic degradation reactions follow the Langmuir–Hinshelwood model and exhibit distinct first-order reaction kinetics (kapp). The apparent rate is determined using the following equation:42,43C0 and Ct are the initial concentration of the solution and the concentration at reaction time t, respectively.
2.5 Antibacterial activity testing for SiO2@AuAg/PDA
The antibacterial activity of SiO2@AuAg/PDA nanospheres against E. coli (Gram-negative bacteria) and S. aureus (Gram-positive bacteria) was conducted. First, an appropriate amount (50 μL) of E. coli or S. aureus preserved in glycerol was taken out and added to 50 mL of Lauria–Bertani culture broth, placed in a conical flask and oscillated for 12 h at 37 °C and 100 rpm. The cultured bacteria were then diluted to 1 × 106 CFU mL−1 with broth and different concentrations of samples were added. After mixing, the bacterial suspensions were treated with a NIR laser (808 nm, 2.5 W cm−2) and without a NIR laser, labeled as L+ and L−, respectively. The treatment time was 6 min, except for the control group. Each group was incubated in a constant-temperature incubator at 37 °C for 4 h. The diluted co-culture solution (100 μL) was inoculated on plates containing Luria–Bertani agar medium and incubated at 37 °C for 24 h. The total number of surviving bacteria was determined using the dilution method and planar colony counting.
2.6 Characterization
The morphology and nanostructure of the nanocomposites were analyzed via field emission transmission electron microscopy (FE-TEM) using a JEM-2100F and scanning electron microscopy (SEM) using a JEOL JSM-6700F. Energy-dispersive X-ray spectroscopy (EDS) was carried out using a JEM-2100F field emission transmission electron microscope. X-ray diffractometers (Bruker D8 Advance) were used to study the samples’ X-ray powder diffraction (XRD). The infrared spectra of the samples were obtained using an infrared spectrometer (TENSOR model 27) in the range of 4000 to 500 cm−1 wave numbers. The X-ray photoelectron spectroscopy (XPS) of all the samples was carried out using a photoelectron spectrometer (ESCALAB 250). Thermogravimetric analysis was carried out on a thermogravimetric apparatus (DTG-60H) under air conditions at a heating rate of 10 °C min−1 from room temperature to 700 °C. Temperature variations during the sample photothermal testing experiments were studied and recorded using an infrared camera (ImageIR 8325). Finally, the metal content in the composites was determined via inductively coupled plasma mass spectrometry (ICP-MS, PlasmaQuad 3).
3. Results and discussion
3.1 Synthesis and characterization of SiO2@AuAg/PDA core/shell nanospheres
In this paper, SiO2@AuAg/PDA nanospheres were successfully prepared via a simple in situ reduction–oxidation polymerization reaction.44 Scheme 1 shows the synthesis process of the SiO2@AuAg/PDA nanospheres. Firstly, SiO2 nanospheres were obtained via the well-known sol–gel method. Then, an appropriate amount of APTES was added into the system for surface functionalization modification and the subsequent amino-modified SiO2 nanospheres provided many anchor sites for the noble-metal nanoparticles, enhancing the interaction between the noble-metal nanoparticles and SiO2. Finally, through a one-step in situ redox polymerization reaction between chloroauric acid, silver nitrate solution and dopamine hydrochloride, the AuAg/PDA hybrid layer was successfully formed and it uniformly covered the surface of the SiO2 nanospheres, resulting in the formation of the SiO2@AuAg/PDA hybrid nanostructure.
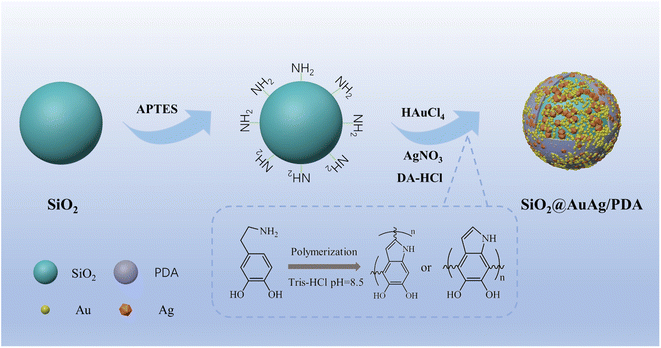 |
| Scheme 1 Schematic illustration for the preparation of SiO2@AuAg/PDA core/shell nanospheres. | |
Fig. 1a shows the SEM image of the SiO2@AuAg/PDA nanospheres. The final sample has a rough surface, which indicates that the AuAg nanoparticles are uniformly coated on the SiO2 nanospheres. As shown in the TEM images, the SiO2@AuAg/PDA nanospheres are relatively uniform in size and show a typical sandwich structure (Fig. 1b). In addition, the large magnification TEM image in Fig. 1c also demonstrates that the AuAg nanocrystals are located between the SiO2 nanosphere and PDA shell. The synthesized samples exhibit a spherical morphology and the average particle size of the final SiO2@AuAg/PDA is about 330 nm with a shell thickness of about 25 nm. The high magnification TEM image (Fig. 1c) shows that there is a large amount of black particles between the SiO2 nanospheres and the PDA shell layer. As a comparison, the SiO2/PDA nanospheres also could be obtained in the absence of AgNO3 and HAuCl4 (Fig. 1d–f). Different from the SiO2@AuAg/PDA, the surface of the nanosphere is smooth. However, an obvious thin shell with a bumpy appearance is found on the surface of the solid core, which indicates that the PDA layer has been well-coated on the SiO2 nanospheres. Fig. 1g–i are the TEM and SEM images of the pristine SiO2 nanospheres. It is found that SiO2 nanospheres are uniformly distributed and possess a spherical structure. Based on the above analysis, it can be concluded that hybrid nanoparticles with AuAg nanoparticles sandwiched between the SiO2 nanosphere and PDA shell are effectively obtained.
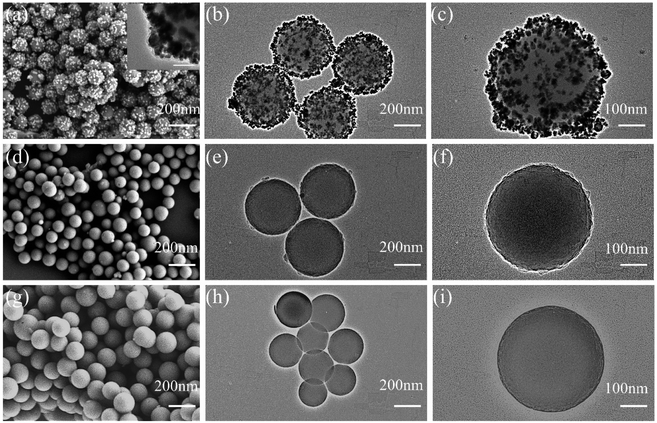 |
| Fig. 1 SEM (a) and TEM (b and c) images of SiO2@AuAg/PDA, SEM (d) and TEM (e and f) images of SiO2/PDA, and SEM (g) and TEM (h and i) images of SiO2; the scale bar of the TEM inset in (a) is 20 nm. | |
To further analyze the internal structure of the AuAg bimetallic nanocrystals in the sandwich-structured SiO2@AuAg/PDA nanospheres, high-angle annular dark-field scanning electron microscopy (HAADF-STEM) and energy-dispersive X-ray spectroscopy (EDX) elemental mapping studies were carried out. The average size of SiO2@AuAg/PDA is increased by about 50 nm compared to pristine SiO2, which originates from the AuAg/PDA coating (Fig. 2a). It is clear that the core/shell nanostructures are mainly composed of C, N, O, Si, Au and Ag (Fig. 2b). Fig. 2c–e show the dispersion of the N, O, and Si elemental composition, respectively. It is noteworthy that the Au and Ag elements are distributed in a hollow-appearing spherical shape, the sizes of which are both larger than the Si sphere (Fig. 2f and g), which indicates that the periphery of the SiO2 nanosphere is well-encapsulated by the AuAg bimetallic nanocrystals. Moreover, the diameters of Au/Ag element spheres are smaller than that of N, suggesting that the AuAg nanocrystals are sandwiched between the SiO2 core and the PDA layer, which is in accordance with the TEM analysis. In this work, it is found that the Au and Ag elements show the similar distribution; thus, they are defined as AuAg bimetallic nanocrystals. These bimetallic nanocrystals are not uniformly distributed on the surface of SiO2 core and most of them are assembled together to form cluster-like nanostructures. This result must be owing to the in situ reduction to form AuAg bimetallic nanocrystals, which will be further discussed in the following discussion. Furthermore, the EDX spectrum also matches the above analysis, which also evidences the successful preparation of SiO2@AuAg/PDA nanospheres (Fig. 2h).
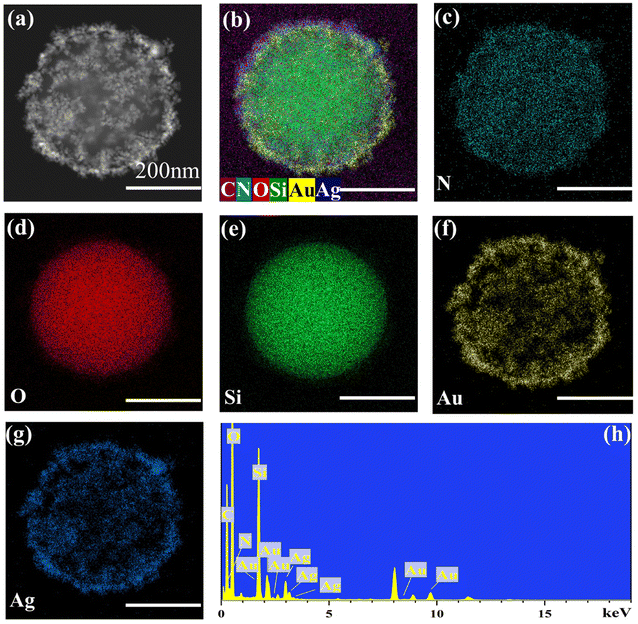 |
| Fig. 2 HAADF-STEM images and EDX elemental mapping of C, N, O, Si, Au and Ag (a–g), and EDX spectrum of SiO2@AuAg/PDA (h); scale bars are 200 nm. | |
In this work, the AuAg/PDA layers are simultaneously formed on the SiO2@AuAg/PDA nanospheres. The AuAg nanostructure and composition of SiO2@AuAg/PDA can be easily tuned by controlling the concentrations of the precursors. Fig. S1† shows the TEM images of SiO2@AuAg/PDA prepared using different contents of HAuCl4: 0 mM (a and b), 5.94 × 10−3 mM (c and d), 8.82 × 10−3 mM (e and f) and 1.17 × 10−2 mM (g and h). Shown in Fig. S1a and b† are the products obtained without the addition of HAuCl4. Clearly, most of the SiO2 nanospheres have tiny Ag nanoparticles attached to them and some of them are naked. Our experiments indicate that the Ag nanocrystals were difficult to immobilize onto the SiO2 nanospheres under the present conditions. When the HAuCl4 content was increased from 0 mM to 5.94 × 10−3 mM, the Au/PDA layer was wrapped around the SiO2 periphery and some AuAg nanoclusters appeared (Fig. S1c and d†). When the HAuCl4 content was 8.82 × 10−3 mM, the density of the Au nanocrystals increased and many monodisperse Au nanocrystals were presented in addition to the AuAg nanoclusters (Fig. S1e and f†). On continuing to increase the HAuCl4 content to 1.17 × 10−2 mM, the coverage of the SiO2 surface was further improved and the Au nanocrystals became well-distributed (Fig. S1g and h†). The high magnification TEM images (Fig. S1b, d, f and h†) also indicate that the agglomeration of the AuAg nanocrystals gradually weakened with the increase in the HAuCl4 content, which must be due to the quick reaction kinetics at high concentration and the good association between the AuAg nanocrystals and PDA polymer.
The above method can also be applied for coating of other nanocarriers due to its versatility. Here, ZIF-8@AuAg/PDA was successfully obtained by using ZIF-8 nanocubes as the template. Without using ZIF-8, only AuAg/PDA nanoaggregates were found in the product (Fig. 3a), in which the AuAg nanocrystals were encapsulated within a PDA shell. When the ZIF-8 was introduced into the reaction system composed of HAuCl4, AgNO3, and dopamine, ZIF-8@AuAg/PDA was fabricated via in situ oxidative polymerization. Fig. 3b shows the TEM image of ZIF-8, which indicates that all the products exhibit cube-like nanostructures with dimensions of around 70–130 nm. During the PDA coating, HAuCl4 and AgNO3 were simultaneously introduced into the ethanol suspension of ZIF-8. Ultrasonication was used to ensure that the HAuCl4 and AgNO3 on the ZIF-8 nanocubes were uniformly dispersed around the cubes. In addition, freshly prepared dopamine hydrochloride solution was added in Tris buffer (pH = 8.5) to start the coating process. After 3 h of sonication, ZIF-8@AuAg/PDA was successfully produced (Fig. 3c). As shown in Fig. 3d, all the ZIF-8 cubes underwent different degrees of self-etching, and there were uniform black particles between the cubes and the pale-white shells, which were obviously AuAg bimetallic nanocrystals. As a result, a ZIF-8@AuAg/PDA nanocomposite with a typical core/shell nanostructure was fabricated.
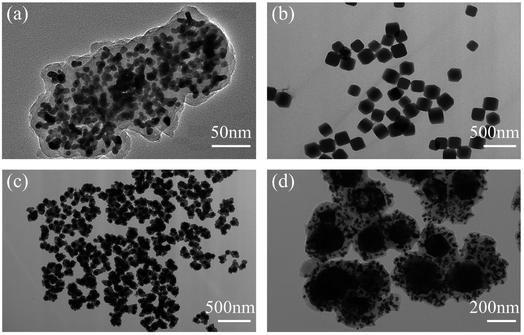 |
| Fig. 3 TEM images of AuAg/PDA (a), ZIF-8 (b), and ZIF-8@AuAg/PDA (c and d). | |
Fig. 4a shows the XRD patterns of SiO2, SiO2/PDA, SiO2@Au/PDA, SiO2@Ag/PDA and SiO2@AuAg/PDA nanospheres. The results show that the initial SiO2 has only one diffraction peak at 2θ = 22°, which is characteristic of the amorphous structure of SiO2.45 After the SiO2 nanospheres have been coated with an AuAg/PDA shell layer, diffraction peaks appeared at 38.2°, 44.22°, 64.52°, and 77.54°, which can be classified as the (111), (200), (220), and (311) Au lattice planes (JCPDS card no. 04-0784). Due to the Au and Ag having a similar structure, they cannot be distinguished. Here, the diffraction peaks must originate from both Au and Ag, and thus the nanocrystals in the product are defined as AuAg bimetallic nanoparticles. Moreover, the SiO2@AuAg/PDA, SiO2@Au/PDA and SiO2@Ag/PDA exhibit similar XRD patterns, which also agrees with the above analysis.
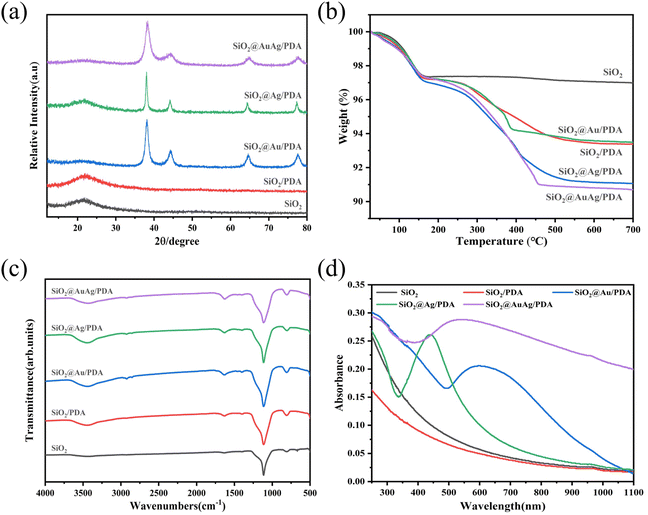 |
| Fig. 4 (a) XRD diffraction patterns, (b) TG curves, (c) FTIR spectra and (d) UV-vis absorption spectra of SiO2, SiO2/PDA, SiO2@Au/PDA, SiO2@Ag/PDA and SiO2@AuAg/PDA. | |
Thermogravimetric analysis (TGA) was further used to study the composition of the SiO2@AuAg/PDA nanospheres (Fig. 4b). The weight losses of SiO2, SiO2/PDA, SiO2@Au/PDA, SiO2@Ag/PDA and SiO2@AuAg/PDA are 3.03 wt%, 6.64 wt%, 8.95 wt%, 6.53 wt% and 9.33 wt%, respectively. PDA will decompose at high temperatures, so the weight loss of SiO2/PDA is larger than that of SiO2. On the other hand, the weight loss of SiO2/PDA is small, indicating that the PDA shell is thin, which agrees with the above TEM analysis. The weight loss of SiO2@AuAg/PDA is the largest, which may be related to the fact that AuAg bimetallic nanocrystals promote the polymerization of PDA.
Fig. 4c shows the FTIR patterns of SiO2, SiO2/PDA, SiO2@Au/PDA, SiO2@Ag/PDA, and SiO2@AuAg/PDA. For SiO2, a strong absorption peak at 1119 cm−1 appears, which can be assigned as the stretching vibration of Si–O–Si. For SiO2@AuAg/PDA, the absorption peaks at 3440 cm−1, 1623 cm−1 and 1403 cm−1 are attributed to the stretching vibrations of –OH/–NH2, –O–H and –C–O in PDA. The Au and Ag do not show characteristic peaks in the FTIR; thus, SiO2@Au/PDA, SiO2@Ag/PDA, and SiO2@AuAg/PDA have the same absorption spectra.
Fig. 4d shows the UV-vis absorption profiles of the different samples. As shown in the figure, the SiO2 and SiO2/PDA suspensions do not exhibit obvious characteristic peaks in the range of 250–1100 nm. In contrast, the characteristic peaks of SiO2@Au/PDA and SiO2@Ag/PDA are located at 650 nm and 450 nm, respectively, which indicates that the Au/PDA and Ag/PDA shell layers have been successfully immobilized on the SiO2. In addition, SiO2@AuAg/PDA has a full coverage of absorption peaks with higher absorbance than the other samples. The characteristic peak absorption is located at about 550 nm, indicating successful loading of AuAg bimetallic nanocrystals. Moreover, the absorption area is critically broadened to the near-infrared region, which demonstrates that the final product probably exhibits good photothermal behavior.
X-ray photoelectron spectroscopy (XPS) was used to analyze the surface chemical compositions and binding states of the SiO2@AuAg/PDA nanospheres. As shown in Fig. 5a, C 1s (284.8 eV), O 1s (532.3 eV), and Si 2p (99.8 eV) peaks were clearly observed for SiO2. The O 1s and Si 2p peaks were derived from SiO2, while the C 1s was derived from the residue ethanol or organic groups of TEOS in the product. After coating with PDA, a clear N 1s peak (399.9 eV) appeared, indicating the presence of PDA. With the AuAg/PDA shell coated on the SiO2, the Si 2p peak is significantly decreased because the detection depth of XPS is only 10 nm, which demonstrates that the SiO2 was well-wrapped by the AuAg/PDA shell layer. In the C 1s spectrum, the characteristic C–N peak at 286.5 eV occupies a considerable proportion (Fig. 5b). In addition, Ag 3d (367.8 eV) and Au 4f (83.7 eV) peaks were detected, which further proves that the Au and Ag bimetallic nanocrystals were encapsulated within the PDA shell (Fig. 5c and d). Moreover, the XPS of the SiO2@Au/PDA nanospheres and SiO2@Ag/PDA nanospheres also indicated the successful immobilization of Au or Ag nanocrystals in the product, respectively.
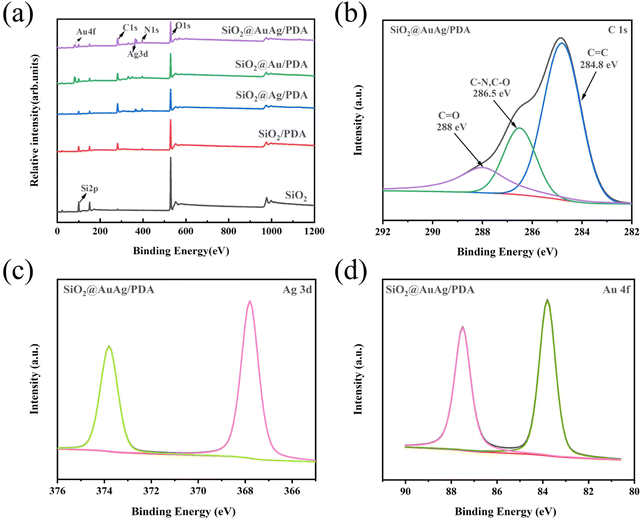 |
| Fig. 5 (a) XPS of SiO2, SiO2/PDA, SiO2@Ag/PDA, SiO2@Au/PDA and SiO2@AuAg/PDA; (b) C 1s, (c) Ag 3d and (d) Au 4f core-level spectra of SiO2@AuAg/PDA. | |
In this work, the surface modification is important for the preparation of the core/shell nanostructure. Here, SiO2@Au/PDA nanospheres synthesized with and without 3-aminopropyltriethoxysilane (APTES) modification were taken as an example. After the addition of dopamine hydrochloride buffer solution, under alkaline conditions, the HAuCl4 reacted rapidly with DA and the solution showed a brick-red color, indicating the formation of Au nanocrystals. As shown in Fig. S2a and c,† Au/PDA nanoclusters attached to SiO2 nanospheres were obtained, in which the Au nanocrystals formed clusters and were encapsulated within the PDA shell. The high magnification TEM image of an individual SiO2@Au/PDA nanosphere is shown in Fig. S2e.† Clearly, cluster-like Au/PDA core/shell nanoparticles were randomly immobilized around the surface of SiO2 and the average size of the cluster-like Au/PDA nanoparticles was 56–100 nm. In this case, APTES was used as a surface modifier for the SiO2 nanospheres, and the amino group was grafted onto the surface of the nanospheres via chemical grafting to obtain SiO2–NH2. After the APTES modification, the Au/PDA shell layer could be effectively wrapped around the surface of SiO2 to give the SiO2@Au/PDA nanosphere. As shown in Fig. S2b and d,† the Au/PDA shell layer was uniformly coated on the SiO2 and the shell thickness was about 20 nm. Fig. S2f† shows the high magnification TEM image of a SiO2@Au/PDA nanosphere. Both the Au nanocrystals and SiO2 nanosphere were well-encapsulated by the PDA, which indicated that the surface modification is important for the preparation.
3.2 Photothermal performance of the SiO2@AuAg/PDA nanospheres
Fig. 6a shows the UV-vis-NIR absorption spectra of the SiO2@AuAg/PDA nanospheres. With increasing concentration, the NIR absorption is relatively increased, which indicates the product possesses a potential photothermal behavior. Under NIR laser irradiation (808 nm, 2.5 W cm−2), the temperature of the SiO2@AuAg/PDA suspensions increased rapidly with increasing concentration and irradiation time. Fig. 6b shows the temperature changes of water, SiO2, SiO2/PDA, SiO2@Ag/PDA, SiO2@Au/PDA, and SiO2@AuAg/PDA after irradiation with a NIR laser (808 nm, 2.5 W cm−2) for 6 min to evaluate the photothermal conversion capability. The temperature changes of deionized water and the SiO2 suspension were small and negligible, and SiO2/PDA had a weak warming trend, which indicated that PDA had a low photothermal conversion effect. In contrast, the temperature changes of SiO2@Ag/PDA, SiO2@Au/PDA and SiO2@AuAg/PDA after 6 min of 808 nm laser irradiation were very large, among which SiO2@AuAg/PDA had the best photothermal effect of 65.1 °C. The photothermal effects of SiO2@Au/PDA and SiO2@Ag/PDA were almost the same and the temperatures were 47.1 °C and 40.8 °C, respectively. These results indicated that Au and Ag nanocrystals were the key factors for photothermal conversion. With increasing concentration, the temperature of SiO2@AuAg/PDA increased and it could reach as high as 73.4°C. As shown in Fig. 6c, when the concentration of the SiO2@AuAg/PDA nanosphere solution was increased from 20 μg mL−1 to 100 μg mL−1, the temperature of the sample solution increased from 49.8 °C to 73.4 °C after irradiation with NIR light for 6 min. To further evaluate the photothermal conversion performance of the SiO2@AuAg/PDA nanospheres, the test process profiles of heating and cooling were recorded (Fig. 6d). The photothermal conversion efficiency of SiO2@AuAg/PDA was calculated to be 48.1% (Fig. 6e), indicating a strong photothermal conversion capability. Fig. 6f shows the photothermal stability of the sample solution, evaluated by controlling the NIR irradiation (808 nm, 2.5 W cm−2) between on/off at room temperature. The temperature distributions of the sample solutions were similar over the 6 cycling cycles, and the results indicated that SiO2@AuAg/PDA possessed good photothermal stability. In addition, the photothermal conversion performance of the SiO2@AuAg/PDA nanospheres can be further directly visualized via IR thermography (Fig. 6g).
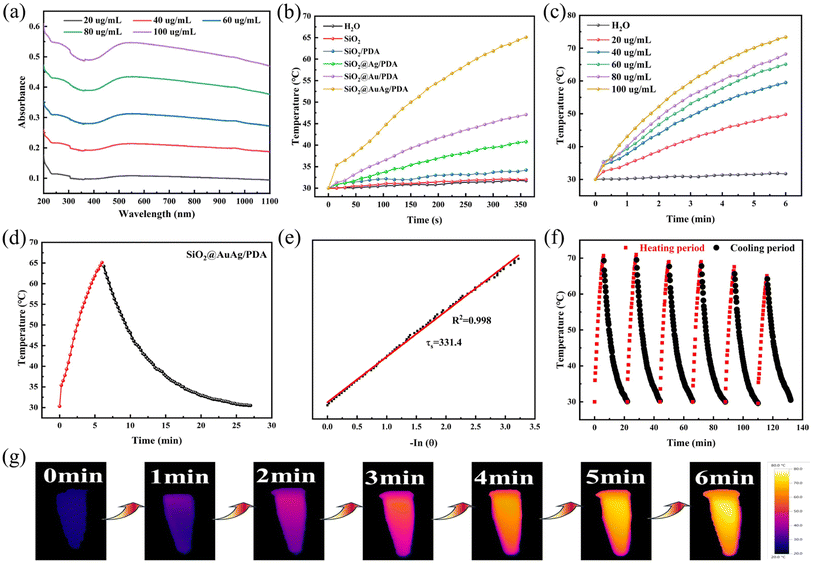 |
| Fig. 6 (a) UV-Vis-NIR absorption spectra of SiO2@AuAg/PDA nanospheres; (b) temperature elevation curves for DI water, SiO2, SiO2/PDA, SiO2@Ag/PDA, SiO2@Au/PDA and SiO2@AuAg/PDA (average concentration 60 μg mL−1), under NIR laser irradiation (808 nm, 2.5 W cm−2); (c) temperature elevation curves for SiO2@AuAg/PDA core–shell suspensions with various concentrations under NIR laser irradiation (808 nm, 2.5 W cm−2); (d) heating and cooling profiles of SiO2@AuAg/PDA nanosphere solution (60 μg mL−1); (e) a plot fitting of cooling time versus −ln(θ), where θ refers to a dimensionless driving force temperature; (f) temperature elevation curve of SiO2@AuAg/PDA core/shell structure solution (60 μg mL−1) with NIR laser on/off cycles; (g) infrared thermography of the SiO2@AuAg/PDA suspension under NIR laser irradiation. | |
3.3 Photothermally enhanced catalytic activity of SiO2@AuAg/PDA nanospheres for reducing 4-nitrophenol
Due to the presence of AuAg bimetallic nanocrystals, the SiO2@AuAg/PDA nanospheres have good catalytic properties. 4-Nitrophenol is characterized by difficult degradation and high stability, and it is an important pollutant in industrial wastewater. Therefore, the catalytic activity of SiO2@AuAg/PDA was evaluated by using 4-nitrophenol as a model.
As shown in Fig. 7a, 4-nitrophenol has a characteristic absorption peak at 317 nm, and the solution was light yellow in color. After the addition of NaBH4, 4-nitrophenol generates 4-nitrophenol ion and the absorption peak was shifted right to 400 nm. When the catalyst was further added, 4-aminophenol was obtained and the solution showed a transparent color with an increased absorption peak at 300 nm. The formation of the target product could be further confirmed by the infrared spectroscopic data from Kate et al.46 In our experiment, 2 mg SiO2@AuAg/PDA nanospheres were added in the reaction and the absorption peak at 400 nm gradually diminished, while at the same time the new absorption peak at 300 nm appeared, which corresponded to the conversion of 4-nitrophenol ions to 4-aminophenol. Eventually, the characteristic peak at 400 nm disappeared completely, while the absorption peak at 300 nm no longer increased, indicating the end of the reaction. The time required for the whole reaction process was 3.5 min, indicating quick reaction kinetics (Fig. 7b).
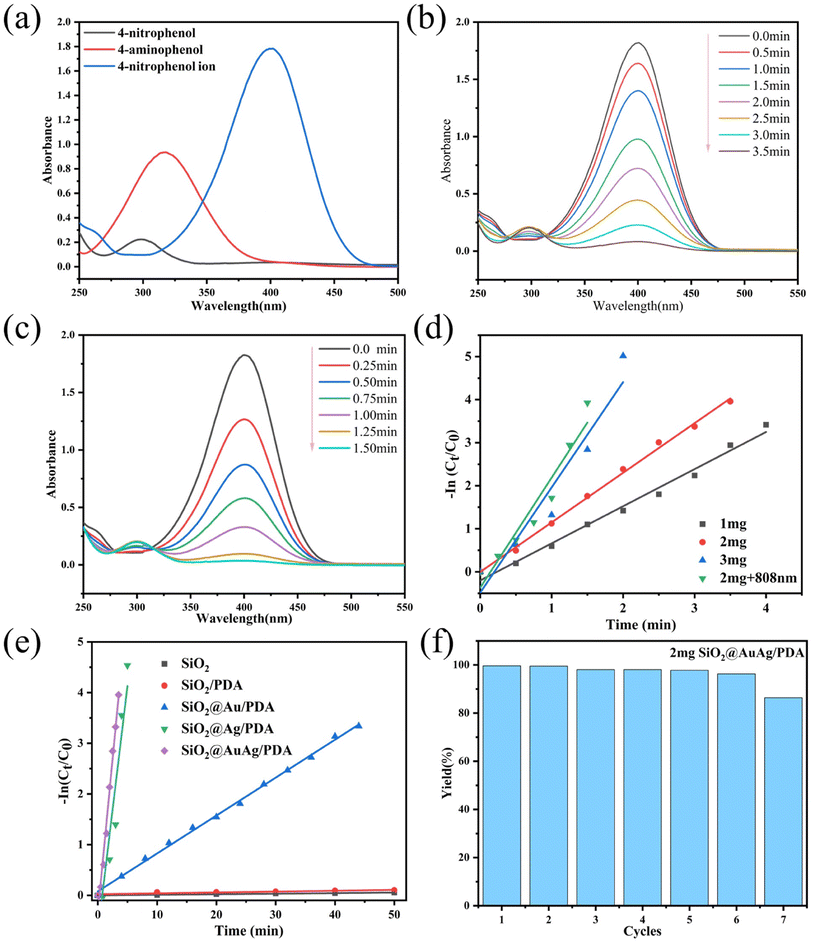 |
| Fig. 7 UV-Vis absorption spectra of 4-nitrophenol, 4-aminophenol and 4-nitrophenol ions (a); UV-Vis spectra of the reaction of 4-nitrophenol catalyzed by 2 mg SiO2@AuAg/PDA nanospheres (b) and UV-Vis spectra of the reaction of 4-nitrophenol catalyzed under NIR laser (808 nm, 2.5 W cm−2) irradiation (c). Linear relationships between ln(Ct/C0) and reaction time for the SiO2@AuAg/PDA nano-catalyst at different weights (d). Linear relationship between ln(Ct/C0) and time for SiO2, SiO2/PDA, SiO2@Au/PDA, SiO2@Ag/PDA and SiO2 @AuAg/PDA (average weight 2 mg) nanocatalysts (e). Catalyst recovery study (f). | |
Interestingly, the catalytic reaction can be made faster by applying NIR (808 nm) irradiation. As shown in Fig. 7c, the reaction time was drastically shortened (57%), which indicates that the NIR treatment significantly improves the catalytic kinetics. Fig. 7d shows the linear relationship between ln(Ct/C0) and reaction time t for different catalyst qualities under different reaction conditions, where Ct represents the concentration of 4-nitrophenol at moment t and C0 represents the initial concentration of 4-nitrophenol. The reaction rate constants for the reduction of 4-nitrophenol to 4-aminophenol with different masses (1 mg, 2 mg and 3 mg) of SiO2@AuAg/PDA nanocatalyst as well as for the NIR (808 nm, 2.5 W cm−2) treatment group (with 2 mg catalyst) were 0.862 min−1, 1.152 min−1, 2.448 min−1 and 2.559 min−1, respectively. In addition, the reaction rate constants with the weight ratios of the nanosphere catalyst were calculated to be 0.862 min−1 mg−1, 0.576 min−1 mg−1 and 0.816 min−1 mg−1. After NIR (2 mg + 808 nm) treatment, the value of the reaction rate constant k reaches 1.279 min−1 mg−1, which indicates that the photothermal effect greatly enhances the catalytic process.
Similarly, as shown in Fig. 7e, the reaction rate constants for different samples (SiO2, SiO2/PDA, SiO2@Au/PDA, SiO2@Ag/PDA and SiO2@AuAg/PDA) at the same weight (2 mg) were also tested and the values were 1.15 × 10−3 min−1, 1.71 × 10−3 min−1, 0.074 min−1, 0.972 min−1 and 1.217 min−1, respectively. The ratios of reaction rate constant to weight are 0.475 × 10−3 min−1 mg−1, 0.575 × 10−3 min−1 mg−1, 3.7 × 10−2 min−1 mg−1, 0.486 min−1 mg−1 and 0.608 min−1 mg−1. It can be found that the presence of SiO2@AuAg/PDA nanospheres in the catalytic reaction is the key factor and the AuAg bimetallic nanocrystals show the best synergistic effect. Fig. 7f shows the cyclic catalytic activity of the SiO2@AuAg/PDA nanospheres. After 7 cycles of catalytic reaction, the catalyst still maintains a high catalytic activity (more than 86%). This is similar to previously reported results.47 The PDA shell layer attached to the surface of the AuAg nanoparticles slows down the oxidation process and improves the stability of the metal nanoparticles, which is conducive to the separation and possible recycling of catalysts. The small reduction at the 7th cycle must result from leakage of the AuAg nanoparticles during separation.48 The results show that the catalyst surface remains active during successive cycles.49 A comparison of this catalyst with other reported catalysts is provided in Table 1.
Table 1 SiO2@AuAg/PDA-catalyzed reduction of pollutants compared to reported catalysts
Substrate |
Catalyst |
Time (min) |
Ref. |
4-NP |
Ag/MgO |
6.7 |
50 |
KCC-1/Au |
12 |
51 |
Au-GO |
30 |
52 |
Ag/TiO2–Cu |
25 |
53 |
Au/graphene hydrogel |
12 |
54 |
SiO2@AuAg/PDA + NIR |
1.75 |
This work |
To further detect the catalytic performance, the infrared profiles of 4-nitrophenol and its reduction product 4-aminophenol were characterized (Fig. S3†). For 4-nitrophenol, two absorption peaks appear at 1342 cm−1 and 1498 cm−1, corresponding to N
O and N–O stretching vibrations, respectively. For 4-aminophenol, two absorption peaks appeared at 864 cm−1 and 1636 cm−1, attributed to N–H stretching vibrations.
3.4 “Photothermal–Ag+” coupled antimicrobial properties of SiO2@AuAg/PDA nanospheres
The antimicrobial properties of different samples (SiO2, SiO2/PDA, SiO2@Au/PDA, SiO2@Ag/PDA and SiO2@AuAg/PDA) were further measured. Fig. 8 shows the optical images of each group of samples incubated with E. coli and S. aureus. The results show that the SiO2@AuAg/PDA group (60 μg mL−1) displayed a clear inactivation of S. aureus without NIR light treatment, which may be related to the release of Ag+ under high-concentration conditions. After treatment with near-infrared light (808 nm, 2.5 W cm−2), the antimicrobial effect of the SiO2/PDA group was weaker, which must be due to the low photothermal effect induced by PDA. In addition, both the SiO2@Au/PDA and SiO2@Ag/PDA groups showed unique antibacterial effects; the former originates from the good photothermal effect of the metal ions, and the latter might be due to the promotion of Ag+ release by NIR treatment.
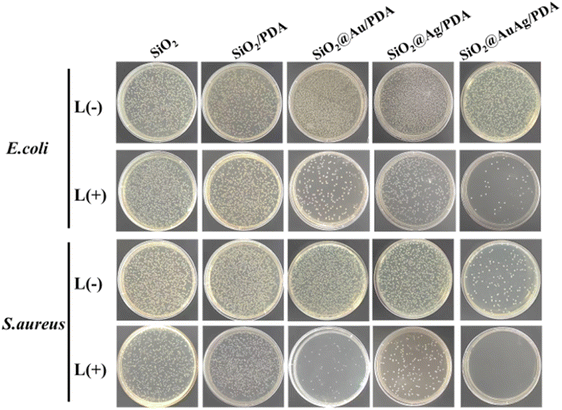 |
| Fig. 8 Images of bacteria colonies formed by E. coli and S. aureus treated with SiO2, SiO2/PDA, SiO2@Au/PDA, SiO2@Ag/PDA and SiO2@AuAg/PDA (60 μg mL−1) with or without NIR irradiation for 6 min (808 nm, 2.5 W cm−2). | |
Most importantly, the antibacterial effect of the SiO2@AuAg/PDA group was the best, and an ultra-low dose of Ag+ ions could effectively disrupt the bacterial membranes, which led to an increase in the permeability and a decrease in the heat-resistance of the cell membrane. On the other hand, thermotherapy can trigger the release of more Ag+, which further improves the bactericidal efficiency of chemothermal therapy.55–57 As the concentration of SiO2@AuAg/PDA nanospheres increased, the antibacterial effect became more and more significant. As shown in Fig. 9, when the concentration was 60 μg mL−1, almost all of the S. aureus was inactivated, while when the concentration was 90 μg mL−1, all of the bacteria were inactivated. The different degrees of bactericidal effect on the two kinds of bacteria might be related to the difference in the structure of the bacterial cell wall.
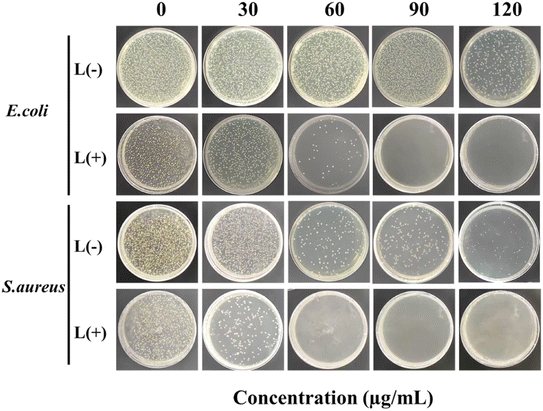 |
| Fig. 9 Images of bacteria colonies formed by E. coli and S. aureus treated with SiO2@AuAg/PDA (0, 30, 60, 90 and 120 μg mL−1) with or without NIR irradiation for 6 min (808 nm, 2.5 W cm−2). | |
Fig. 10 shows a schematic illustration of the photothermal antimicrobial process of the SiO2@AuAg/PDA nanospheres. Under weak alkaline conditions, the dopamine molecules undergo self-polymerization, which leads to the easy deposition of polydopamine (PDA) on the surface of the template SiO2, improving the stability and biocompatibility of the composite without any significant effect on bacteria. Under NIR treatment, the SiO2@Au/PDA nanospheres convert the light energy into heat energy due to their good photothermal properties, thereby destroying the protein structure of the bacteria and ultimately killing them (Fig. 10a). However, to better kill bacteria via photothermal therapy, a higher temperature is usually required, thus limiting its practical application. Ag+ is known to have broad-spectrum antibacterial activity, but this is weak in the absence of NIR treatment. Once NIR irradiation is introduced, Ag+ release is also promoted during the photothermal process, making it more helpful to kill bacteria (Fig. 10b). As a result, an “Ag+ release–photothermal” synergistic antibacterial effect was carried out and the coupled behavior showed exciting bactericidal effects. Finally, the SiO2@AuAg/PDA nanospheres have good photothermal properties and they are more destructive to bacteria. According to the related literature,58 the synergistic effect of two elements, bimetallic gold and silver, outperforms monometallic nanoclusters. Moreover, the AuAg bimetallic nanoclusters possess higher antibacterial efficiency compared to the monometallic nanoclusters. In conclusion, the “Ag+-release–photothermal” coupled sterilization method has great potential in nanomedicine.
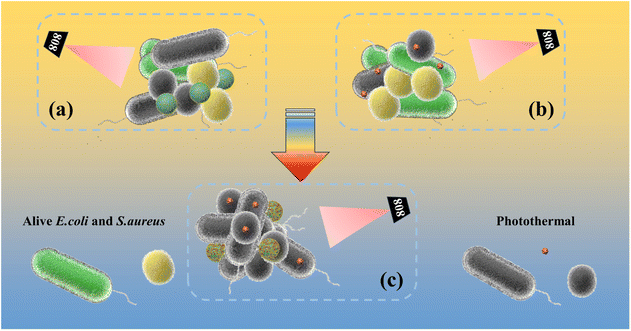 |
| Fig. 10 Illustration of the interaction between particles and bacteria in the antibacterial process. Schematic diagram of antimicrobial activity under (a) Au nanocrystal photothermal, (b) Ag+-release–photothermal, and (c) bimetallic Ag+-release–photothermal coupled conditions. | |
4. Conclusion
In this work, we report a simple method for the preparation of SiO2@AuAg/PDA core/shell hybrid nanospheres via in situ redox polymerization. The AuAg bimetallic nanoparticles were well-encapsulated by the PDA layer, which could effectively prevent their leaching during the catalytic reaction. Due to the synergistic effect of the AuAg bimetallic nanoparticles, the SiO2@AuAg/PDA nanospheres exhibited good catalytic activity, with the catalytic kinetics being improved by 2.2 times under near-infrared irradiation. In addition to the inherent bactericidal effect of released Ag+, the SiO2@AuAg/PDA nanospheres showed good photothermal antimicrobial activity against Gram-negative bacteria (E. coli) and Gram-positive bacteria (S. aureus). Importantly, all the bacteria could be inactivated at a concentration of 90 μg mL−1. As a result, due to the non-traditional “Ag+-release–photothermal” synergistic antibacterial function and NIR-improved catalytic activity, this material is expected to be widely used in industrial, agricultural, environmental and medical applications.
Author contributions
Dazheng Ci: conceptualization, investigation, methodology, visualization, writing – original draft. Ning Wang: investigation, methodology, supervision, funding acquisition. Yunqi Xu: visualization, supervision. Shanshan Wu: formula derivation. Jing Wang: visualization. Haoran Li: visualization. Shouhu Xuan: supervision, visualization. Qunling Fang: resources, supervision, project administration, funding acquisition.
Conflicts of interest
The authors declare no conflict of interest.
Acknowledgements
National Natural Science Foundation of China (Grant number 81703449) and the Fundamental Research Funds for the Central Universities (PA2020GDKC0005).
References
- H. Lv, M. Lin, C. Yu, H. Wang, M. Li, L. Zhang, Z. Liu and Z. Chen, Pd decorated MoS2 nanoflowers as photothermal catalyst for enhanced NIR-induced 4-nitrophenol reduction, J. Environ. Chem. Eng., 2023, 11(5), 110375 CrossRef CAS.
- M. Mamera, J. Van and M. Aghoghovwia, Treatment of faecal sludge and sewage effluent by pinewood biochar to reduce wastewater bacteria and inorganic contaminants leaching, Water Res., 2022, 221, 118775 CrossRef CAS PubMed.
- N. Yılmaz, T. Baran and M. Çalışkan, Production of Pd nanoparticles embedded on micro-sized chitosan/graphitic carbon nitride hybrid spheres for treatment of environmental pollutants in aqueous medium, Ceram. Int., 2021, 47(19), 27736–27747 CrossRef.
- S. Zhang, J. Wang, Y. Zhang, J. Ma, L. Huang, S. Yu, L. Chen, G. Song, M. Qiu and X. Wang, Applications of water-stable metal-organic frameworks in the removal of water pollutants: a review, Environ. Pollut., 2021, 291, 118076 CrossRef CAS PubMed.
- M. Frieri, K. Kumar and A. Boutin, Antibiotic resistance, J. Infect. Public Health, 2017, 10(4), 369–378 CrossRef PubMed.
- S. Li, B. Ondon, S. Ho, J. Jiang and F. Li, Antibiotic resistant bacteria and genes in wastewater treatment plants: from occurrence to treatment strategies, Sci. Total Environ., 2022, 838(4), 156544 CrossRef CAS PubMed.
- S. Shao, Y. Hu, J. Cheng and Y. Chen, Research progress on distribution, migration, transformation of antibiotics and antibiotic resistance genes (ARGs) in aquatic environment, Crit. Rev. Biotechnol., 2018, 38(8), 1195–1208 CrossRef CAS PubMed.
- M. Chang, Z. Hou, M. Wang, C. Yang, R. Wang, F. Li, D. Liu, T. Peng, C. Li and J. Lin, Single-atom Pd nanozyme for ferroptosis-boosted mild-temperature photothermal therapy, Angew. Chem., Int. Ed., 2021, 60(23), 12971–12979 CrossRef CAS PubMed.
- J. Pickering, V. Bhethanabotla and J. Kuhn, Plasmonic photocatalytic reactor design: use of multilayered films for improved organic degradation rates in a recirculating flow reactor, Chem. Eng. J., 2017, 314, 11–18 CrossRef CAS.
- J. Qiu, T. Yang, Y. Li, W. Qian and X. Liu, Au@Ag@Pt core–shell nanorods regulating Ag release behavior endow titanium antibacterial activity and biocompatibility, Rare Met., 2022, 41(2), 630–638 CrossRef CAS.
- J. Yan, H. Sun, J. Li, W. Qi and H. Wang, A theranostic plaster combining photothermal therapy and photodynamic therapy based on chlorin e6/gold nanorods (Ce6/Au nrs) composite, Colloids Surf., A, 2018, 537, 460–466 CrossRef CAS.
- L. He, L. Lv, S. Pillai, H. Wang, J. Xue, Y. Ma, Y. Liu, Y. Chen, L. Wu, Z. Zhang and L. Yang, Efficient degradation of diclofenac sodium by periodate activation using Fe/Cu bimetallic modified sewage sludge biochar/UV system, Sci. Total Environ., 2021, 783, 146974 CrossRef CAS PubMed.
- R. Liu, J. Guo, G. Ma, P. Jiang, D. Zhang, D. Li, L. Chen, Y. Guo and G. Ge, Alloyed crystalline Au-Ag hollow nanostructures with high chemical stability and catalytic performance, ACS Appl. Mater. Interfaces, 2016, 8(26), 16833–16844 CrossRef CAS PubMed.
- M. Marimuthu, H. Li and Q. Chen, Facile ultrasonic synthesis of silver-based bimetal nanoparticles for efficient catalytic reduction of 4-nitrophenol, J. Mol. Liq., 2021, 333, 115963 CrossRef CAS.
- Y. Sun, Z. Yang, P. Tian, Y. Sheng, J. Xu and Y. Han, Oxidative degradation of nitrobenzene by a fenton-like reaction with Fe-Cu bimetallic catalysts, Appl. Catal., B, 2019, 244, 1–10 CrossRef CAS.
- G. Liao, Y. Gong, L. Zhong, J. Fang, L. Zhang, Z. Xu, H. Gao and B. Fang, Unlocking the door to highly efficient Ag-based nanoparticles catalysts for NaBH4-assisted nitrophenol reduction, Nano Res., 2019, 12(10), 2407–2436 CrossRef CAS.
- A. Deshmukh and B. Kim, Flower-like biogenic gold nanostructures for improved catalytic reduction of 4-nitrophenol, J. Environ. Chem. Eng., 2021, 9(6), 106707 CrossRef CAS.
- S. Fu, C. Zhu, J. Song, M. Engelhard, X. Li, D. Du and Y. Lin, Highly ordered mesoporous bimetallic phosphides as efficient oxygen evolution electrocatalysts, ACS Energy Lett., 2016, 1(4), 792–796 CrossRef CAS.
- X. Zhang, R. Shen, X. Guo, X. Yan, Y. Chen, J. Hu and W. Lang, Bimetallic Ag-Cu nanoparticles anchored on polypropylene (PP) nonwoven fabrics: superb catalytic efficiency and stability in 4-nitrophenol reduction, Chem. Eng. J., 2021, 408, 128018 CrossRef CAS.
- H. Yu, W. Tang, K. Li, S. Zhao, H. Yin and S. Zhou, Enhanced catalytic performance for hydrogenation of substituted nitroaromatics over Ir-based bimetallic nanocatalysts, ACS Appl. Mater. Interfaces, 2019, 11(7), 6958–6969 CrossRef CAS PubMed.
- X. Liao, F. Yang, R. Wang, X. He, H. Li, R. Kao, W. Xia and H. Sun, Identification of catabolite control protein A from staphylococcus aureus as a target of silver ions, Chem. Sci., 2017, 8(12), 8061–8066 RSC.
- X. Bai, Y. Yang, W. Zheng, Y. Huang, F. Xu and Z. Bao, Synergistic photothermal antibacterial therapy enabled by multifunctional nanomaterials: progress and perspectives, Mater. Chem. Front., 2023, 7(3), 355–380 RSC.
- S. Sasidharan, R. Poojari, D. Bahadur and R. Srivastava, Embelin-mediated green synthesis of quasi-spherical and star-shaped plasmonic nanostructures for antibacterial activity, photothermal therapy, and computed tomographic imaging, ACS Sustain. Chem. Eng., 2018, 6(8), 10562–10577 CrossRef CAS.
- Y. Shi, J. Yin, Q. Peng, X. Lv, Q. Li, D. Yang, X. Song, W. Wang and X. Dong, An acidity-responsive polyoxometalate with inflammatory retention for NIR-II photothermal-enhanced chemodynamic antibacterial therapy, Biomater. Sci., 2020, 8(21), 6093–6099 RSC.
- Y. Xu, K. Wang, S. Zhao, Q. Xiong, G. Liu, Y. Li, Q. Fang, X. Gong and S. Xuan, Rough surface NiFe2O4@Au/Polydopamine with a magnetic field enhanced photothermal antibacterial effect, Chem. Eng. J., 2022, 437, 135282 CrossRef CAS.
- J. Jia, G. Liu, W. Xu, X. Tian, S. Li, F. Han, Y. Feng, X. Dong and H. Chen, Fine-tuning the homometallic interface of Au-on-Au nanorods and their photothermal therapy in the NIR-II window, Angew. Chem., Int. Ed., 2020, 59(34), 14443–14448 CrossRef CAS PubMed.
- E. Park, R. Selvaraj and Y. Kim, High-efficiency photothermal sterilization on PDMS film with Au@CuS yolk-shell nanoparticles, J. Ind. Eng. Chem., 2022, 113, 522–529 CrossRef CAS.
- G. Liu, Q. Xiong, Y. Xu, Q. Fang, K. Leung, M. Sang, S. Xuan and L. Hao, Sandwich-structured MXene@Au/polydopamine nanosheets with excellent photothermal-enhancing catalytic activity, Colloids Surf., A, 2022, 633, 127860 CrossRef CAS.
- Z. Lv, S. He, Y. Wang and X. Zhu, Noble metal nanomaterials for NIR-triggered photothermal therapy in cancer, Adv. Healthcare Mater., 2021, 10(6), e2001806 CrossRef PubMed.
- X. Chen, S. Ku, J. Weibel, E. Ximenes, X. Liu, M. Ladisch and S. Garimella, Enhanced antimicrobial efficacy of bimetallic porous CuO microspheres decorated with Ag nanoparticles, ACS Appl. Mater. Interfaces, 2017, 9(45), 39165–39173 CrossRef CAS PubMed.
- D. Kim, K. Park, J. Park and I. Choi, Photoactive MOF-derived bimetallic silver and cobalt nanocomposite with enhanced antibacterial activity, ACS Appl. Mater. Interfaces, 2023, 15(19), 22903–22914 CrossRef CAS PubMed.
- A. Jin, Y. Wang, K. Lin and L. Jiang, Nanoparticles modified by polydopamine: working as drug carriers, Bioact. Mater., 2020, 5(3), 522–541 Search PubMed.
- L. Liang, T. Hou, Q. Ouyang, L. Xie, S. Zhong, P. Li, S. Li and C. Li, Antimicrobial sodium alginate dressing immobilized with polydopamine-silver composite nanospheres, Composites, Part B, 2020, 188, 107877 CrossRef CAS.
- I. I. Niyonshuti, V. Krishnamurthi, D. Okyere, L. Song, M. Benamara, X. Tong, Y. Wang and J. Chen, Polydopamine surface coating synergizes the antimicrobial activity of silver nanoparticles, ACS Appl. Mater. Interfaces, 2020, 12(36), 40067–40077 CrossRef CAS PubMed.
- Y. Zhu, Y. Sun, J. Wang and B. Yu, Antimicrobial and antifouling surfaces through polydopamine bio-inspired coating, Rare Met., 2022, 41(2), 499–518 CrossRef CAS.
- N. Olteanu, C. Lazăr, A. Petcu, A. Meghea, E. Rogozea and M. Mihaly, One-pot synthesis of fluorescent Au@SiO2 and SiO2@Au nanoparticles, Arabian J. Chem., 2016, 9(6), 854–864 CrossRef CAS.
- J. Zhang, G. Lu and C. Cai, Regio- and stereoselective hydrosilylation of alkynes catalyzed by SiO2 supported Pd–Cu bimetallic nanoparticles, Green Chem., 2017, 19(11), 2535–2540 RSC.
- S. Mallakpour, E. Nikkhoo and C. Hussain, Application of MOF materials as drug delivery systems for cancer therapy and dermal treatment, Coord. Chem. Rev., 2022, 451, 214262 CrossRef CAS.
- C. Vaitsis, G. Sourkouni and C. Argirusis, Metal organic frameworks (MOFs) and ultrasound: a review, Ultrason. Sonochem., 2019, 52, 106–119 CrossRef CAS PubMed.
- P. Bazuła, P. Arnal, C. Galeano, B. Zibrowius, W. Schmidt and F. Schüth, Highly microporous monodisperse silica spheres synthesized by the Stöber process, Microporous Mesoporous Mater., 2014, 200, 317–325 CrossRef.
- H. Lin, S. Gao, C. Dai, Y. Chen and J. Shi, A two-dimensional biodegradable niobium carbide (MXene) for photothermal tumor eradication in NIR-I and NIR-II biowindows, J. Am. Chem. Soc., 2017, 139(45), 16235–16247 CrossRef CAS PubMed.
- V. Jawale, G. Gugale, M. Chaskar, S. Pandit, R. Pawar, S. Suryawanshi, V. Pandit, G. Umarji and S. Arbuj, Two- and three-dimensional zinc oxide nanostructures and its photocatalytic dye degradation performance study, J. Mater. Res., 2021, 36(7), 1573–1583 CrossRef CAS.
- V. Jawale, A. Al-fahdawi, S. Salve, S. Pandit, G. Dawange, G. Gugale, M. Chaskar, D. Hammiche, S. Arbuj and V. Pandit, 6, 13-pentacenequinone/zinc oxide nanocomposites for organic dye degradation, Mater. Today: Proc., 2022, 52, 17–20 CAS.
- Q. Fang, J. Zhang, L. Bai, J. Duan, H. Xu, K. Cham and S. Xuan, In situ redox-oxidation polymerization for magnetic core-shell nanostructure with polydopamine-encapsulated-Au hybrid shell, J. Hazard. Mater., 2019, 367, 15–25 CrossRef CAS PubMed.
- H. Zhang, D. Ke, L. Cheng, X. Feng, X. Hou, J. Wang, Y. Li and S. Han, CoPt-Co hybrid supported on amino modified SiO2 nanospheres as a high performance catalyst for hydrogen generation from ammonia borane, Prog. Nat. Sci.: Mater. Int., 2019, 29(1), 1–9 CrossRef CAS.
- P. Kate, V. Pandit, V. Jawale and M. Bachute, L-Proline catalyzed one-pot three-component synthesis and evaluation for biological activities of tetrahydrobenzo[b]pyran: evaluation by green chemistry metrics, J. Chem. Sci., 2022, 134, 4 CrossRef CAS.
- Z. Moradi and A. Ghorbani, Fe3O4@SiO2@KIT-6@2-ATP@CuI as a catalyst for hydration of benzonitriles and reduction of nitroarenes, Sci. Rep., 2023, 13(1), 7645 CrossRef CAS PubMed.
- B. Zhao, Z. Dong, Q. Wang, Y. Xu, N. Zhang, W. Liu, F. Lou and Y. Wang, Highly efficient mesoporous core-shell structured Ag@SiO2 nanosphere as an environmentally friendly catalyst for hydrogenation of nitrobenzene, Nanomaterials, 2020, 10(5), 883 CrossRef CAS PubMed.
- P. Chhattise, S. Saleh, V. Pandit, S. Arbuj and V. Chabukswar, ZnO nanostructures: a heterogeneous catalyst for the synthesis of benzoxanthene and pyranopyrazole scaffolds via a multi-component reaction strategy, Mater. Adv., 2020, 1(7), 2339–2345 RSC.
- M. Nasrollahzadeh, R. Akbari, Z. Issaabadi and S. Sajadi, Biosynthesis and characterization of Ag/MgO nanocomposite and its catalytic performance in the rapid treatment of environmental contaminants, Ceram. Int., 2020, 46(2), 2093–2101 CrossRef CAS.
- H. Yang, S. Li, X. Zhang, X. Wang and J. Ma, Imidazolium ionic liquid-modified fibrous silica microspheres loaded with gold nanoparticles and their enhanced catalytic activity and reusability for the reduction of 4-nitrophenol, J. Mater. Chem. A, 2014, 2(30), 12060 RSC.
- Y. Choi, H. Bae, E. Seo, S. Jang, K. Park and B. Kim, Hybrid gold nanoparticle-reduced graphene oxide nanosheets as active catalysts for highly efficient reduction of nitroarenes, J. Mater. Chem., 2011, 21(39), 15431 RSC.
- A. Hernández and V. González, Silver nanoparticles loaded on Cu-doped TiO2 for the effective reduction of nitro-aromatic contaminants, Chem. Eng. J., 2015, 261, 53–59 CrossRef.
- J. Li, C. Liu and Y. Liu, Au/graphene hydrogel: synthesis, characterization and its use for catalytic reduction of 4-nitrophenol, J. Mater. Chem., 2012, 22(17), 8426 RSC.
- M. Liu, D. He, T. Yang, W. Liu, L. Mao, Y. Zhu, J. Wu, G. Luo and J. Deng, An efficient antimicrobial depot for infectious site-targeted chemo-photothermal therapy, J. Nanobiotechnol., 2018, 16(1), 23 CrossRef PubMed.
- H. Wang, D. Wang, H. Huangfu, S. Chen, Q. Qin, S. Ren, Y. Zhang, L. Fu and Y. Zhou, Highly efficient photothermal branched Au-Ag nanoparticles containing procyanidins for synergistic antibacterial and anti-inflammatory immunotherapy, Biomater. Sci., 2023, 11(4), 1335–1349 RSC.
- K. Zhou, Z. Zhang, J. Xue, J. Shang, D. Ding, W. Zhang, Z. Liu, F. Yan and N. Cheng, Hybrid Ag nanoparticles/polyoxometalate-polydopamine nano-flowers loaded chitosan/gelatin hydrogel scaffolds with synergistic photothermal/chemodynamic/Ag(+) anti-bacterial action for accelerated wound healing, Int. J. Biol. Macromol., 2022, 221, 135–148 CrossRef CAS PubMed.
- Q. Zhai, H. Xing, X. Zhang, J. Li and E. Wang, Enhanced electrochemiluminescence behavior of gold-silver bimetallic nanoclusters and its sensing application for mercury(II), Anal. Chem., 2017, 89(14), 7788–7794 CrossRef CAS PubMed.
|
This journal is © The Royal Society of Chemistry 2024 |