DOI:
10.1039/D3NJ04571D
(Paper)
New J. Chem., 2024,
48, 216-227
Simple devising of N-doped carbon dots (N-CDs) as a low-cost probe for selective environmental toxin detection and security applications†
Received
30th September 2023
, Accepted 10th November 2023
First published on 17th November 2023
Abstract
Nitrogen-doped carbon dots (N-CDs) possess outstanding photostability and interesting physicochemical and photochemical properties. Herein, we present cyan-emitting N-CDs that were fabricated through the treatment of salicylic acid with ethylene diamine using a simple microwave-assisted method. It was observed that the reaction produced N-CDs that strongly emitted cyan light with a quantum yield (QY) of 33%. The N-CDs were examined with the assistance of HR-TEM, XPS, XRD, FTIR, PL, and UV measurements. Due to the fluorescence enrichment effect caused by the N atoms in carbon dots, these N-CDs can be used as a fluorescent material for the detection of nitro-explosives and toxic metal cations. The addition of picric acid resulted in splendid quenching of the N-CDs, with a limit of detection (LOD) value of approximately 82.9 nM that was ascribed to the Förster resonance energy transfer between the N-CDs and the nitro-aromatics. Furthermore, the powerful coordination between oxygen-rich moieties and N-CDs for the detection of Fe3+ and Hg2+ cations induced fluorescence quenching through photo-induced electron transfer and the inner-filter effect pathway, leading to the effective recognition of metal ions. The sensor probe exhibited a wide-ranging linear response with an LOD of approximately 30 nM for Fe3+ and 160 nM for Hg2+ ions. Thus, the as-prepared N-CDs can function as a fluorescence sensor, a secure portable information device, and a paper-based probe for detection in biological systems.
1. Introduction
Through previous discoveries and research, nanostructured materials have been obtained with unique optical properties.1 Semiconductor-based luminescent quantum dots (QDs) are one of the finest nanomaterials for heavy metal ion sensor applications due to their excellent optical sensing, tunable size, color, and narrow emission spectral properties.2,3 The size-dependent optical properties in semiconductor QDs have empowered new opportunities for detection. However, due to the harmful nature of semiconductor-based QDs, major shortcomings exist that restrict their applications. There have been many attempts to improve biocompatible luminescent QDs. New materials provide similar types of beneficial properties with the goal of overcoming the drawbacks (e.g., toxicity) of QDs.
Carbon quantum dots (CQDs) are a recently developed carbon-based zero-dimensional (0-D) nanomaterial with excellent optical properties and a size less than 10 nm, and they can be used in bioimaging, biosensors, chemosensors, catalysis, and several other applications.4–7 CQDs can be synthesized from organic materials using several types of processing methods that will result in numerous functions for extensive applications in detection.8,9 While earlier findings have shown the wide capability of CQDs as a platform material, the use of their optical properties to understand fundamental mechanisms has been a lesser improvement.
Luminous CQDs from carbon nanotubes and graphite were first reported in 2004 and 2006, respectively.10,11 There is a graphitic core in CQDs bounded by a hydrophilic surface.12,13 CQDs exist as a supplement to the carbon nanotube (CNT) graphene class due to the graphitic core. The hydrophilic surface properties impart promising solubility from the carboxylate moieties.14 Two studies reported that dopamine can alter the fluorescent nature of CQDs, but one study reported enhanced fluorescence, and in contrast, quenched fluorescence was observed in the other study.15 Moreover, a study on the quenched fluorescence of CQDs stated that CQDs might be assisted by electron acceptors with N,N-diethylamine donors, or electron donors with nitro-aromatic acceptors.16 To understand such conflicting actions, standardization of the material's synthesis and characterization is necessary, along with additional fundamental investigation of CQD properties.
Photoluminescent CQDs are classified into three types: those with a (i) graphitic core, (ii) surface functional assemblies, and (iii) molecular fluorophore bound to the surface.17 Hydroxyl, amino, and carboxyl groups are the surface functional moieties, and their speciations such graphitic-N, amino-N, pyrrolic-N, and pyridinic-N play a prominent role in the photostability, solubility, and photophysical properties of CQDs.18,19 Therefore, using different organic precursors to tune the properties is a practical approach for functionalization of surface states.20 Ding et al. reported that a high degree of oxidation that governs the color of the photoluminescent property requires additional surface defects in CQDs.21 However, because of π–π interactions and the aggregation of CQDs, self-quenching in the solid-state form often occurs.22 These drawbacks restrict the use of CQDs, particularly in sensors and optoelectronic devices, where solid luminescent features are mainly required.23
Picric acid (PA) as well as 2,4,6-trinitrophenol are in a primary class of highly explosive nitro-aromatic compounds. PA is mainly used as a reagent in the dye and pesticide industries, and also for producing fireworks and pharmaceuticals.24 Due to its explosive nature, it is used as a bursting agent in land mines. Because of its distinctive solubility in water, it can enter the human body through aquatic systems. PA exposure at very low concentrations can strongly impact the human body and result in eye/skin damage, cancer, respiratory issues, and can also cause lethal effects to the environment from water and soil pollution.25,26 Hence, the admissible concentration of PA must be less than 2.18 μM, as required by the surface water environmental quality standard (GB3838-2002) and drinking water health standards of China (GB5749-2006).27,28
Toxic heavy metals that pollute the environment are a foremost concern because they are responsible for harmful impacts on a global scale. The primary cause of environmental degradation is the disposal of inorganic industrial contaminants in ecological water bodies.29,30 This is predominantly occurring due to the rapid development of industry, and illegitimate and improper dumping of waste, pesticides, and fertilizers.31 Heavy metal ions are considered hazardous to the well-being of humans and animals due to their stable nature in the environment.32 Iron is one of the most essential elements for humans and animals because it is involved in important roles such as the electron transfer process, enzyme catalysis, and oxygen metabolism in biological systems.33 However, the excessive accumulation or deficiency of Fe3+ from the normal allowable limits can cause severe health problems such as blood disorders, oxidation of cells, organ damage, and eventually death.34,35
Every year, 3000 tons of mercury enter the atmosphere.36 Even at low-level exposure to mercury, severe health issues can result that affect the lungs, kidneys, and the digestive, immune, and nervous systems, and can also cause DNA damage and impair mitosis.37 There are 4–5 g of different forms of iron in the human body, and considerable concentrations of mercury in the human body at 10–20 μg L−1. Hence, there is an urgent need to decrease the level of Hg2+ in biological and environmental systems. The allowable presence of Fe3+ and Hg2+ in drinking water is 0.3 μM38 and 10 nM (2 ppb),39 respectively, according to the United States Environmental Protection Agency (EPA). Therefore, it is a vital and crucial task for ecologists to recognize the presence of nitro-explosives and heavy metal cations such as Fe3+ and Hg2+ in a polluted environment.
Various traditional analytical techniques used for detection are inductively coupled plasma optical emission spectrometry (ICP-OES),40 infrared spectroscopy (IR),41 and atomic absorption spectroscopy (AAS).42 However, there are several disadvantages to these systems such as high instrument expenses, difficult sample preparation, and immobility. Therefore, other technologies are required to sense and eliminate nitro-explosives and heavy metal pollutants from the environment. To recognize nitro-explosives and heavy metal cations in the atmosphere, there have been substantial efforts to improve economical and selective fluorescent detection. There are numerous benefits to the fluorometric technique, such as low cost, increased sensitivity, and rapid response.43 The intention and improvement of monitoring devices such as sensors for the recognition of nitro-explosives and toxic metal cations are of substantial importance with concern to environmental safeguards and human well-being.
Many scientists have adopted green methods for the preparation of 0-D nanostructures. Several approaches have been implemented for the preparation of CQDs, such as microwave-assisted, hydrothermal-carbonization, calcination, laser-irradiation, ultrasonics, electrochemical oxidation, and pyrolysis. The microwave-assisted approach is considered to be a modest, rapid, and efficient generation method and results in a high product yield.44,45 Based on these approaches, we synthesized N-CDs with cyan-colored emission through the microwave method using salicylic acid and ethylene diamine as precursors. The as-synthesized N-CDs exhibited outstanding photostability and aqueous solution pH sensitivity.
The significant finding from this work is the use of a sequence of nitro-aromatic acceptors with diverse absorptive spectral behaviors. The fluorescence quenching for N-CDs in nitro-aromatics is primarily due to a Förster resonance energy transfer (FRET) mechanism, with a calculated limit of detection (LOD) value of 82.9 nM. We also found that N-CDs could be employed to detect Fe3+ and Hg2+ ions in an aqueous solution because of their fluorescence quenching. In the fluorescence sensing probe, LOD values as low as 30 nM for Fe3+ and 160 nM for Hg2+ were successfully detected, and a stronger linear relationship in the series was obtained. In this work, we also fabricated a fluorescence-based ink for information security application. Our results will enable the extensive use of N-CDs for imaging, sensing, and optoelectronic applications.
2. Experimental section
2.1. Materials
Salicylic acid, ethylene diamine, picric acid (PA), o-nitrophenol (ONP), o-nitroaniline (ONT), p-nitrophenol (PNP), p-nitroaniline (PNT), 2,4-dinitrophenol (DNP), 4-nitrotoluene (4-NT), m-dinitrobenzene (DNB), Pb(CH3COO)2, NiCl2·6H2O, CrCl3·6H2O, Hg(CH3COO)2, FeCl3, FeCl2, MnCl2·4H2O, CoCl2·6H2O, CuCl2·2H2O, NaCl, KCl, and AgCl were purchased from SRL Private Limited. The chemicals were used as received without further purification. Throughout these experiments, de-ionized water was used.
2.2. Characterization
The high-resolution transmission electron microscopy (HR-TEM) images were captured by a TECNAI G20 F20 (FEI, USA) electron microscope functioning at 200 kV. X-ray diffraction (XRD) patterns were calculated using a D/max-3A. The Fourier transform infrared (FTIR) spectra were documented using a Bruker Alpha spectrometer ranging from 4000 cm−1 to 400 cm−1. X-ray photoelectron spectroscopy (XPS) was performed with a ULVAC-PHI 5000 probe III-spectroscope. Dynamic light scattering (DLS) particle-size analysis and zeta potential measurement were performed using a Horiba Scientific SZ-100. A UV-3600 (Shimadzu, Japan) spectrophotometer was used for optical absorption measurements. Fluorescence spectroscopy was performed using an LS-45 fluorescent spectrometer (PerkinElmer) with a slit width of 10 nm for emission, and excitation at a PMT voltage of 650 V. Time-correlated single photon counting (TCSPC) measurements were obtained through a Fluorolog-3 spectrometer (Horiba Jobin Yvon, France). All optical properties were recorded using a standard quartz cuvette path length of 1 cm.
2.3. Synthesis of N-CDs using a microwave-assisted method
First, 0.5 g of salicylic acid was added to 5 mL of ethylene diamine in a beaker and then heated in a 750 W domestic microwave oven for 5 min. After completion of the reaction, the mixture was removed from the microwave and equilibrated to room temperature. The resultant semi-solid material was centrifuged at 3000 rpm for 15 min to remove the insoluble materials. After subsequent dilution, the translucent and yellowish supernatant liquid emitted cyan-colored fluorescence emission under ultraviolet radiation. The crude N-CDs from the product mixture were then dialyzed against deionized water using a dialysis membrane bag to remove larger particles. The resulting N-CDs were well-dispersed in water and then stored in a refrigerator for further studies. After two months of storage at room temperature, no noticeable loss of fluorescence was detected, which demonstrated the stability of the as-crafted material (Fig. 1).
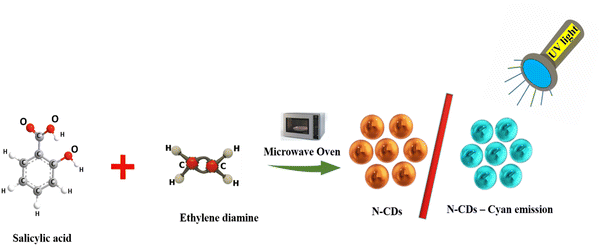 |
| Fig. 1 Schematic representation of N-CDs. | |
2.4. N-CDs can be used as a probe for the detection of PA, Fe3+, and Hg2+ ions
We investigated the fluorescence properties of the as-prepared N-CDs in the presence of various nitro-aromatics and metal cations. First, 1 mM aqueous stock solutions of various nitro-aromatics and metal cations were prepared. For the selectivity of PA, Fe3+, and Hg2+ detection, we tested various nitro-aromatic compounds and metal cations (ONP, DNB, PNP, DNP, ONT, PNT, NT, PA, Fe2+, Fe3+, Cu2+, Co2+, Mn2+, Na+, K+, Hg2+, Ag+, Pb2+, Ni2+, and Cr3+), which were individually added to N-CD solutions. At room temperature, gradually increasing concentrations of PA were added to the N-CD solutions. Under the same conditions, increasing concentrations of Fe3+ and Hg2+ were added to the N-CD solutions. Using a photoluminescence quenching experimental procedure, selective as well as sensitive fluorometric recognition of nitro-explosive compounds and metal cations was performed using the usual titration method with the excitation wavelength of 350 nm. Using a slope method, the LOD for selective nitro-explosive compounds and metal cations was also determined through the changes observed in the photoluminescence (PL) intensity equivalent to the PA, Fe3+, and Hg2+ concentration.
2.5. Quantum yield measurements
The quantum yield for the N-CDs was measured with the assistance of quinine sulfate (QS) as a reference. N-CDs were liquefied in ultrapure water (η = 1.33), and QS with a QY of 0.54 was dissolved in H2SO4 (0.1 M). Thus, we created five different concentrations with less than 0.1 absorbance at 340 nm. The fluorescence was observed at the excitation of 366 nm. The quantum yield for the N-CDs with the reference (QS) sample was calculated by the comparison of the integrated PL intensities (excited at 366 nm) and the absorption values (excited at 366 nm). The general formula for calculation of the quantum yield of N-CDs is: | ϕ = QR × (IAS/IAR) × (ODR/ODS) × (ηS2/ηR2) | (1) |
where ϕ denotes the quantum yield of N-CDs; QR denotes the quantum yield of quinine sulfide; IAS and IAR represent the integrated emission intensity of the sample and reference, respectively; ODR and ODS represent the optical density of the reference and sample, respectively; and η represents the refractive index.
3. Results and discussion
3.1. Morphological characterization of N-CDs
With the assistance of high resolution transmission electron microscopy (HR-TEM), the morphology of the N-CDs was initially characterized and is displayed in Fig. 2a. The N-CDs were well dispersed along a quasi-spherical array-like structure. We also calculated the lattice spacing, with lines spaced approximately 0.32 nm apart, as displayed in Fig. 3b, to illustrate the materials. Fig. 3c shows a histogram for the HR-TEM average size distribution of the N-CDs. The measured size of the nanoparticles (n > 10) was nearly 4.5 nm, and was calculated by ImageJ software. Subsequently, X-ray diffraction (XRD) studies were performed to determine the amorphous nature of the N-CDs. The XRD pattern for the N-CDs in Fig. 3d exhibits a broad hump at 2θ of approximately 24.7°, which was attributed to highly disordered carbon atoms with an amorphous nature. The (002) plane was consistent with an amorphous region described in earlier reports.46 Along with the HR-TEM data, the XRD reports prove that N-CDs are amorphous in nature.
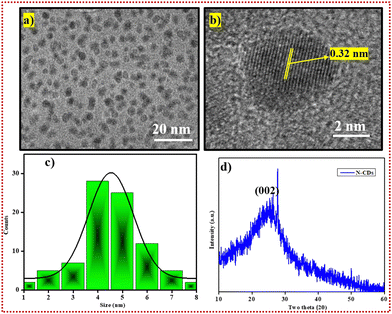 |
| Fig. 2 (a) HR-TEM image of N-CDs. (b) HR-TEM image with lattice fringes of N-CDs. (c) The average particle size distribution histogram for N-CDs. (d) The XRD spectrum for N-CDs. | |
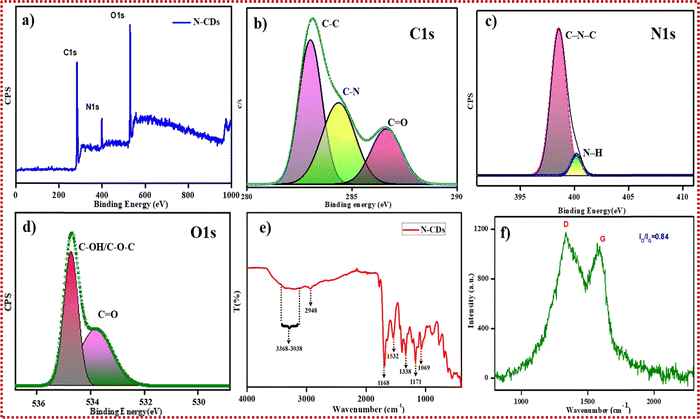 |
| Fig. 3 (a) XPS survey spectrum for N-CDs, (b) C 1s spectrum for N-CDs, (c) N 1s spectrum for N-CDs, (d) O 1s spectrum for N-CDs, (e) FTIR spectrum for N-CDs, and (f) Raman spectrum for N-CDs. | |
3.2. Surface studies of N-CDs
Using the XPS studies, the chemical composition on the exterior surface of the as-prepared N-CDs was examined. The exterior of the N-CDs is chiefly composed of carbon (C), nitrogen (N), and oxygen (O) atoms. The existing three peaks at 284 eV for C 1s, 400 eV for N 1s, and 535 eV for O 1s (Fig. 3a) show the full-scan survey spectrum for the N-CDs.47Fig. 3b displays the C 1s spectrum, which confirms the presence of deconvoluted peaks at 283 eV, 284.3 eV, and 286.6 eV that correspond to the functional groups of C–C/C
C, C–N, and C
O, respectively. Two peaks were deconvoluted to C–N–C and N–H groups in the N 1s spectrum, as depicted in Fig. 3c, which occurred at approximately 398.6 eV and 400.2 eV, respectively. Fig. 3d shows the O 1s deconvoluted spectrum, which exhibits two peaks at 533.8 eV and 535.1 eV corresponding to the C
O and C–OH/C–O–C functional groups, respectively.
The preceding results confirm that N-CDs were derived from ethylene diamine after microwave carbonization and possessed functional amino, carboxyl, and hydroxyl groups, which is in satisfactory agreement with the clear FTIR spectral bands. Also, the surface (exterior) charge for the N-CDs was characterized by DLS measurements, and was measured to be approximately −3.0 mV (zeta potential), as shown in Fig. S1e (ESI†).
Then, FTIR measurements were performed to examine the bonding composition and surface functional assemblies of the N-CDs, as shown in Fig. 3e. The broad peak from 3368 cm−1 to 3038 cm−1 was ascribed to the stretching vibrations of O–H and N–H. Bands observed at approximately 2948 cm−1 and 1338 cm−1 were attributed to the stretching as well as bending vibrations of C–H. The band at 1532 cm−1 was assigned to the C
C stretching vibrations on the phenyl ring. In addition, a sharp absorption band near 1688 cm−1 was assigned to the C
O stretching vibration of aryl ketone. The peak at 1171 cm−1 was attributed to the occurrence of C–O stretching vibrations. A band located at 1069 cm−1 proved the existence of the C–N stretching vibration of the amine linkage, and the band at 686 cm−1 was attributed to the N–H deformation vibration.
The presence of oxygen-containing functional assemblies (as carbonyl, carboxyl, and hydroxyl groups) endows the N-CDs with excellent solubility. To obtain evidence for the hybridized state of the designed N-CDs, Raman spectroscopic measurements were performed. There were two distinctive peaks, with the peak at 1335 cm−1 being due to a cluttered D-band, and the peak at 1585 cm−1 indicating a crystalline G-band of the carbon nanomaterials, as shown in Fig. 3f. The calculated ratio of the D-band and G-band intensity revealed an amorphous carbon phase for the formed N-CDs, and was found to be 0.84.48 Hence, the Raman spectra proved the existence of sp3 hybridized carbons with sp2 graphitic carbons as defects and confirmed the carbonization of precursors by the microwave-assisted approach.
3.3. Optical properties of N-CDs
To investigate the photosensitivity of the N-CDs, their optical capture ability was analyzed via PL spectroscopy. Fig. 3a shows an absorption peak at 242 nm due to the existence of a π–π* shift, and the broad absorption position at 350 nm was ascribed to an n–π* electron shift for various functional sets of atoms present on the N-CDs.
Furthermore, we investigated the fluorescent behaviors by continuously subjecting N-CDs in aqueous solution to varying excitation wavelengths. Fig. 4a illustrates the intrinsic PL nature of the N-CDs, and shows the emission of cyan when exposed to UV light excitation. Excitation at 350 nm resulted in an increase in emission, with the greatest intensity at 447 nm. Fig. 4b shows the emission spectrum for N-CDs excited at wavelengths of 250, 300, 320, 340, 350, 360, 380, 400, and 450 nm. The N-CDs excited at 350 nm displayed the greatest PL intensity, and there was a null redshift in the emission spectrum. The PL quantum yield (QY) for the N-CDs was approximately 33% when it was envisioned using quinine sulphate as the standard. The N-CDs were constructed with a graphitic core and rich functional moieties on the surface. Thus, the high quantum yield was mainly due to the delocalized electrons in the core structure and on the passivated surface in rich functional groups, which endows N-CDs with the characteristics of a high quantum yield material.
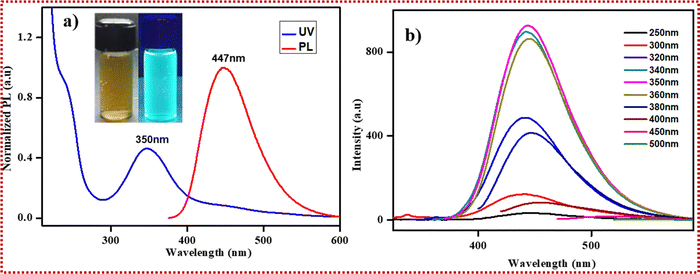 |
| Fig. 4 (a) Normalized UV and PL spectra of N-CDs. (b) Emission spectra of N-CDs. | |
3.4. Stability of N-CDs
The stability of N-CDs is of prime importance for extensive applications. To validate the optical steadiness of N-CDs, we deeply focused on the properties of manifold variables. In Fig. S1a (ESI†), the photostability of N-CDs was proved through continuous irradiation with UV light at 365 nm. There were no noticeable changes in the PL intensity with increasing irradiation time, even up to 5 h. The color of the N-CD solution remained unaffected even after 60 days of storage (Fig. S1b, ESI†), and it continued to exhibit the glow of luminescence. These results show that the stability of the N-CDs is satisfactory. Fig. S1c (ESI†) displays the fluorescence intensity, which remained consistent throughout several concentrations of NaCl, signifying that N-CDs can be used in saline environments for real-world applications. Moreover, pH studies (Fig. S1d, ESI†) of N-CDs were performed, and it was observed that there is great impact of the solution pH on the PL intensity of the N-CDs. When the range of the pH was from 1 to 7, the PL intensity was enhanced by increasing the pH values, and the highest PL intensity was observed at neutral pH. After pH 7, there was a gradual reduction in the PL intensity.
The pH-receptive properties of the N-CDs denote their great potential for pH-sensing applications. The as-prepared N-CDs remained unchanged in a pH range of 5–8. Fig. S1g (ESI†) shows the stability of N-CDs at different temperatures. There are nearly no changes at different temperatures, and this shows that our as-prepared N-CDs are thermally stable. These results highlight the ideal photostability and thermal stability of the fluorescent probe and its long-term colloidal stability.
4. Selectivity and sensitivity of N-CDs against nitro-explosives and metal ions
4.1. Effect of PA and metal ions on the PL intensity of N-CDs
Under neutral pH conditions, we established various nitro-aromatics such as PA, ONP, PNP, DNP, ONT, PNT, 4-NT, DNB, as well as Fe3+, Fe2+, Cu2+, Mn2+, Na+, K+, Co2+, Hg2+, Ag+, Pb2+, Ni2+, Cr3+, Al3+, Zn2+, and Cd2+ ions to study the effect of N-CDs on the PL intensity at 447 nm, and thus, respective concentrations of 1 mM were prepared. The presence of N- and C-rich functional groups on the exterior of N-CDs and the adsorbed nitro-aromatics and metal cations might markedly alter the PL strength of the N-CDs. Only PA, Fe3+, and Hg2+ were able to modify the substantial decrease in the PL intensity. These outcomes revealed that the as-made N-CDs are evidently adequate for the sensing of PA, Fe3+, and Hg2+ in aqueous solution. Fig. 5a shows the selective quenching efficiency of increasing PA, Fe3+, and Hg2+ ion concentrations and their effect on the PL intensity of N-CDs among the multiple analytes tested. When the concentrations of the PA, Fe3+, and Hg2+ ions were increased, the PL intensity of the N-CDs decreased. Additionally, the PL intensity of the N-CDs of additional metal ions exhibited slight quenching properties. The PL intensities of PA, Fe3+, and Hg2+ were significantly quenched, which indicated the selective recognition of nitro-explosives and metal cations. Fig. 5b and c displays the sensitivity of N-CDs for PA and Fe3+ and Hg2+ ions in the presence of additional nitro-aromatics and metal ions, excluding for Fe3+, Hg2+, and PA, which are similar. On account, there was increased sensitivity towards other metal ions and nitro-aromatics.
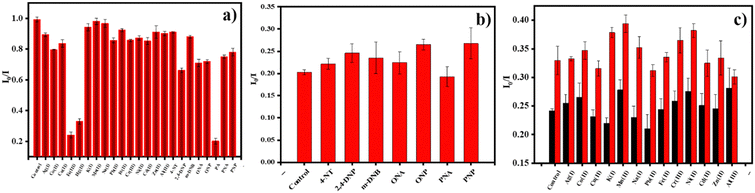 |
| Fig. 5 (a) Selectivity for various nitro-aromatics and metal cations. (b) and (c) Sensitivity of N-CDs in the presence of PA, Fe3+, and Hg2+ ions. | |
4.2. Fluorescence quenching of PA
We also examined the absorption bands of the N-CDs after adding diverse concentrations of PA. With increasing PA concentrations, the intensity in the absorption peak at 358 nm (conforming to PA in Fig. 7a—the magenta line) increased, but no new absorption spots were detected. We analyzed the absorption spectra of N-CDs after the accumulation of diverse nitro-aromatics, and no absorption changes were observed in the spectra of a combination of N-CDs and nitro-aromatics. This analysis showed that the formation of non-emissive substances was not observed amongst N-CDs and nitro-aromatics. In physical organic chemistry, nitro-aromatics are used for model studies because of the significant changes in their physicochemical properties due to the substituents present in the phenyl ring.
To study quenching characteristics, we combined N-CDs with different nitro-aromatics. Fig. 6a shows the illustrative results for quenching studies with PA. As we predicted, quenching can be increased with increasing concentrations of nitro-aromatic compounds. Other nitro-aromatic quenching was comparable to the corresponding addition of other nitro-compounds such as ONP, PNP, DNP, ONT, PNT, DNB, and 4-NT, as shown in Fig. 5a. The quenching for PA and other nitro-aromatics was concentration-dependent, as demonstrated in the Stern–Volmer plots (Fig. 6b). With these Stern–Volmer plots, we observed satisfactory linearity for various nitro-aromatics. The Stern–Volmer quenching constants (Ksv) for various nitro-aromatic compounds were calculated from eqn (2):49,50
where
I0 and
I denote the fluorescence intensities in the presence or absence of quenching agent [Q]. A linear relation of
R2 = 0.97 was determined from the linear series of the fluorescence response, whereby 1–26 μM (
Fig. 6c and b) assured a non-linear plot for the detection of PA. The
Ksv can be calculated through the linear response of the Stern–Volmer graph. Among these nitro-aromatics, PA resulted in extra-efficient emission quenching, and their respective
Ksv values of 3.3 × 10
4 M
−1 were very similar to those of other nitro-aromatics displaying lesser quenching ability. Their
Ksv values were inversely relative to the number of nitro-substituents on the aromatic ring. Based on the 3-Sigma method (
i.e., the slope method), the limit of detection = 3
σ/
k, where
σ denotes the standard deviation of blank measurement (N-CDs), and
k denotes the slope acquired from the linear fit (regression coefficient). The calculated LOD value for PA (82.9 nM) was similar to values obtained from other reported analyses, as given in Table S1 (ESI
†).
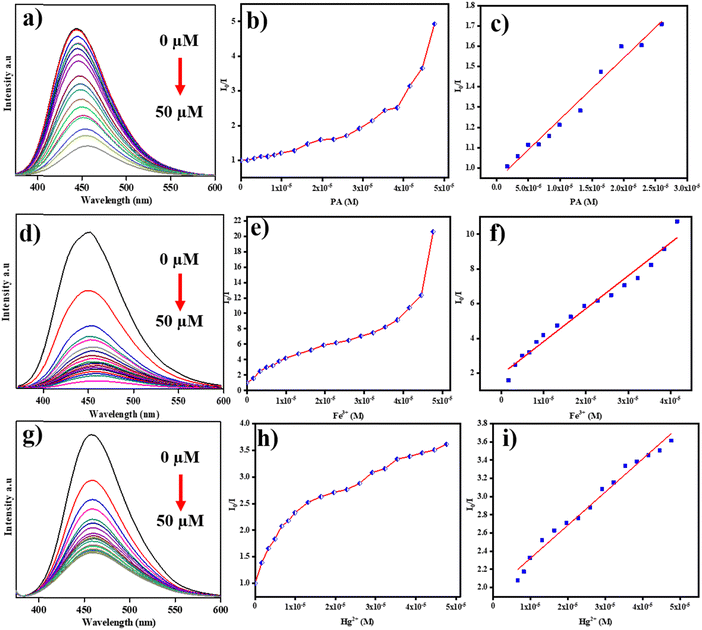 |
| Fig. 6 (a), (d) and (g) The fluorescence quenching spectrum of N-CDs at increasing concentrations of PA, Fe3+, and Hg2+ ions at the excitation wavelength of 350 nm. (b), (e) and (h) The mono-exponential variation of the quenching efficiency of (I0/I) with PA, Fe3+, and Hg2+ ions, respectively. (c), (f) and (i) The linear variation of (I0/I) with PA, Fe3+, and Hg2+ ions, respectively. | |
4.3 Fluorescence quenching of Fe3+ and Hg2+ ions
The fluorescence quenching spectra for N-CDs is shown in Fig. 6d and g for increasing concentrations of Fe3+ and Hg2+ ions at the excitation wavelength of 350 nm. The obtained outcomes indicated that the fluorescence peaks of the N-CDs in the Fe3+ and Hg2+ system were significantly quenched. These results indicate that as the concentration of Fe3+ and Hg2+ ions increased, the I0/I value demonstrated non-linear affiliation (Fig. 6e and h). The direct relations of R2 = 0.97 and R2 = 0.97 were determined from the linear range for fluorescence response at concentrations of 1–40 μM and 6.5–50 μM for Fe3+ and Hg2+ ions with N-CDs, respectively (Fig. 6f and i). The calculated Ksv values for the Fe3+ and Hg2+ ions were noted as 1.8 × 105 M−1 and 3.6 × 104 M−1, respectively. Based on the 3-σ method, the limit of detection = 3σ/k. The LOD values for Fe3+ and Hg2+ ions were calculated as 30 nM and 160 nM, respectively. The efficiency of the as-prepared N-CDs was determined from the limit of detection (LOD) values for the Fe3+ and Hg2+ ions, which were compared with values from other reported analyses, as given in Tables S2 and S3 (ESI†).
4.4. Quenching mechanism of the N-CDs-PA system
The quenching mechanism used by the fluorescent N-CDs in the PA system is normally ascribed to electron transfer or an energy transfer mechanism. Nitro-substituted benzene rings are greatly electron-deficient in nature, and thus, the fluorescent quenching allegedly occurs mainly via an electron transfer mechanism. The efficiency of fluorescence quenching is absolutely associated with the electron-capturing ability of the nitro-substituents existing in the aromatic ring. The energy gap among the lowest unoccupied molecular orbitals (LUMOs) of the acceptor and donor is the main driving force for the e− transfer mechanism. If the nitro-aromatic compounds with a lower LUMO exhibit the largest quenching, then the predominant quenching mechanism is electron transfer. A greater similarity does not exist between the quenching efficiency of nitro-aromatic compounds and the dipole moments or energy gaps. These outcomes demonstrate that the quenching of N-CDs by nitro-aromatic compounds cannot be described by electron transfer itself.
The optical studies of N-CDs and PA were examined to increase our understanding of the fluorescence recognition mechanism between PA and the as-prepared N-CDs. Fig. 7a shows that there is satisfactory overlap between the UV-visible absorption spectrum of PA in an evident spectral range, which indicates that there were two probable mechanisms that caused fluorescence quenching of the N-CDs: the inner filter effect (IFE) and FRET mechanisms. There was overlay between the donor fluorophore of the energy emission spectrum and the acceptor (quenching agent) of the energy absorption spectrum. The widespread overlap between donor's absorption spectrum and the emission spectrum illustrates the efficiency of energy transfer. Thus, this indicates that the FRET process is composed of experimental quenching along with satisfactory spectral overlays. Fig. 7a displays the donor–acceptor spectral merge for the instance of PA, as there is substantial overlap for the emission spectrum of the N-CD donor and the absorption spectrum of the PA acceptor.
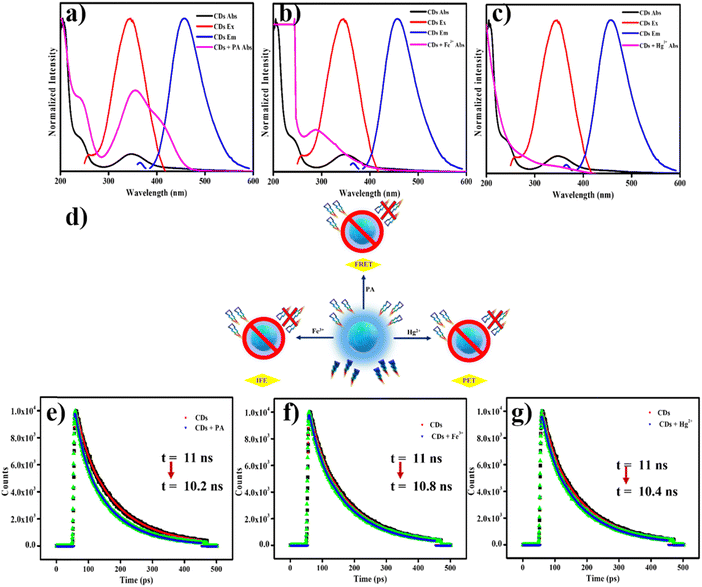 |
| Fig. 7 (a)–(c) The spectral overlap of absorption and emission for N-CDs/PA, N-CDs/Fe3+, and N-CDs/Hg2+. (d) Graphical representation of the possible detection mechanism. (e)–(g) The average lifetime of N-CDs with PA, Fe3+, and Hg2+. | |
The FRET mechanism can be further confirmed by TCSPC, and tests were conducted to verify the fluorescent lifetime of N-CDs, with and without PA. Fig. 7e displays the illustrative results from lifetime measurements of PA along with N-CDs. Here, a decrease in the fluorescent lifetime was observed. The fluorescent lifetime decreases were ascribed to the FRET pathway between the electron-rich amino groups present on the surface of the N-CDs and the electron-deficient phenyl ring of PA, which is supported by previous studies. Thus, the mechanism of fluorescence quenching should be attributed to the FRET pathway instead of IFE. The average fluorescence lifetime was reduced by the addition of PA (10.2 ns) when compared with N-CDs alone (11 ns), which indicates that the FRET51 process is occurring between N-CDs (electron-donor) and PA (electron-acceptor), and is in agreement with previous studies. In summary, all the evidence demonstrates that fluorescence quenching of N-CDs is preferably ascribed to an energy transfer mechanism. Table S1 (ESI†) displays a comparison of the efficiency values obtained in the current work and values from earlier reports.
4.5. Quenching mechanism of the N-CDs-Fe3+ and Hg2+ systems
The quenching mechanism of fluorescence in response to metal ions was triggered by the three mechanisms of IFE, photo-induced electron transfer (PET), and the energy transfer pathway. The changes in the absorption by N-CDs in the presence of Fe3+ and Hg2+ metal cations were individually studied. The absorption spectra of Fe3+ with N-CDs and the excitation spectrum of N-CDs are elegantly overlapped, with re-absorption in the excitation region of N-CDs (Fig. 7b). There was nearly no change in the average lifetime, from 11 to 10.8 ns (Fig. 7f) in the presence of Fe3+, which indicated that the fluorescence quenching was a static process whereby a steady ground-state non-fluorescent complex was formed. Hence, the fluorescence quenching of N-CDs is favored over the precise addition of N-CDs and suitably follows the IFE pathway. In contrast, a steady increase in the absorption band was noted through the addition of Hg2+ ions to the N-CDs, as displayed in (Fig. 7c), because no type of interaction can occur with Hg2+ ions when N-CDs are in the ground state. Moreover, we determined if there was any other overlap between the excitation spectrum of N-CDs and the absorption spectrum of Hg2+ ions with N-CDs (Fig. 7c), which ruled out the possibility of the IFE pathway.
We then scrutinized the probability of the FRET mechanism as the reason for the fluorescence quenching, and thus, we tested for other emissions from the Hg2+ ion solution under UV-light irradiation. Unfortunately, there was no emission, and therefore, these results neglect the assumption of the FRET mechanism. Hence, the quenching attained was due to the PET pathway, whereby the negatively charge-bound N-CDs associated with positively charge-bound metal cations, and also, the unaffected average lifetime of N-CDs with Hg2+ was 11–10.4 ns. Fig. 7g denotes the turn-off fluorescence achieved via a static quenching method and provides a stable ground state of non-fluorescence. Consequently, the transference of electrons to Hg2+ cations after the occurrence of photoexcitation via N-CDs reveals the PET pathway. The quenching processes of FRET, IFE, and PET are illustrated in Fig. 7d.
4.6. Detection of Fe3+ and Hg2+ ions and PA in real water sample analysis
The capability of N-CDs to detect ions in commercial water samples (mineral water obtained from a marketplace) was tested. Analytical methods were used to analyze the recoverability and gauge the practical application of N-CDs for sensing PA and the metal cations Fe3+ and Hg2+. Among the toxic metal cations, the two different carcinogenic metal cations were prepared at diverse concentrations of approximately 10, 20, and 30 μM. The recovery percentage was calculated for Fe3+, Hg2+, and PA, as shown in Table S4 (ESI†). Thus, the obtained outcomes explore the possibility of the use of probes in sensor studies.
4.7. Fluorescence-based anti-counterfeiting, ink studies, and a paper-based probe for the visual detection of toxins
The excellent features of N-CDs in terms of stability, fluorescence properties, decreased toxicity, and great stability indicate that they can be used for multiple applications. The as-prepared N-CDs were employed for anti-counterfeiting as a security application to hide highly confidential information so that it cannot be communicated through modern technologies. Therefore, we dissolved N-CDs to achieve a concentration greater than 0.005 g mL−1 and transferred the liquid to an ink pen. Then, a digital style was used to draw a pattern of three sets of the numbers 888 on Whatman filter paper, as shown in Fig. 8(a). Different numbers were also sketched on the paper using fluorescent ink prepared from N-CDs, and the confidential numbers were not visible to the naked eye. After irradiation with 365 nm UV light, the sketched numbers became clearly visible, as shown in Fig. 8(c). The same procedure was performed with fluorescent ink on Whatman filter paper to draw a rose and write “Tamizh Thirukkural”. Both are clearly visible under 365 nm light, as illustrated in Fig. 8(b) and (d). Our preliminary tests indicate that our N-CDs are an ideal choice for ensuring information security with low-cost materials.
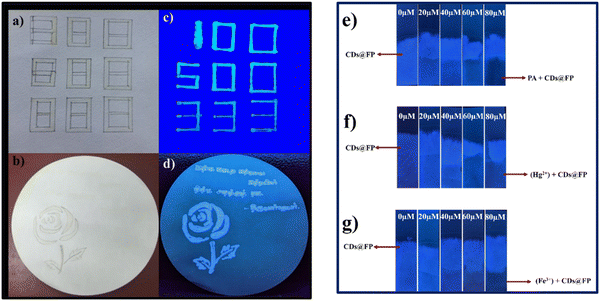 |
| Fig. 8 (a) and (c) Anti-counterfeiting application of CDs without and with UV-light irradiation. (b) and (c) Fluorescent ink without and with UV-light irradiation. (e)–(g) Fluorescent paper-based probe for visual detection of PA, Hg2+, and Fe3+. | |
For the detection of hazardous water toxins using the naked eye, we fabricated fluorescent paper strip probes containing CDs. To do this, we cut cellulose-based Whatman filter paper into a desired dimension. The piece of filter paper was then soaked for 2 seconds in a freshly prepared CD solution. The soaked paper containing CDs was dried for 2 h at 60 °C. Different concentrations of different toxins were then individually added to the surface of the fabricated CDs@filter paper. Increasing concentrations of PA, Hg2+, and Fe3+ were individually added at 0 μM, 20 μM, 40 μM, 60 μM, and 80 μM, and then dried at room temperature. The dried strips were placed under UV light irradiation at 365 nm.
The obtained results clearly show that the fluorescent intensity from the filter paper gradually decreased, and emission was finally quenched at the concentration of 80 μM. These obtained results revealed that our prepared CDs not only detected the toxins using an instrument-based low-cost technique, but detection also occurred through visual means. A comparison of N-CDs as a probe for detection of nitro-explosives and metal ions appears in Table S5 (ESI†), and a comparison of high quantum dots with unique properties is shown in Table S6 (ESI†).
4.8 Current challenges and outlook for the security and toxicity fields
For future applications of CDs in data security, anticounterfeiting, and toxicity studies, it is necessary to upgrade the quantum yield, lifetime measurements, and the stability of CD-based phosphorescent materials. Moreover, a joint diverse technology for the fortification of confidential papers and the design of anti-counterfeiting labels would be a desirable forthcoming development for CD security encryption studies. Furthermore, a methodical investigation should be performed using animal models to determine the exact molecular mechanism and involved metabolic pathways that result in CD toxicity prior to any forthcoming clinical uses.
5. Conclusion
Fluorescent N-CDs were successfully produced by a simplistic and green microwave-assisted technique using salicylic acid and ethylene diamine as precursors. Significantly, N-CDs possess outstanding water solubility, exceptional excitation, and wavelength-independent, pH-sensitive PL properties. The ability of N-CDs to quench the fluorescence of nitro-aromatics is preferably ascribed to a FRET mechanism with an LOD of 82.9 nM. The evidence supporting the FRET mechanism is the reduction in fluorescent lifetime linked with the Stern–Volmer quenching constants (Ksv). Furthermore, it is striking that N-CDs can behave as active fluorescent probes for selective and sensitive recognition of Fe3+ and Hg2+ in aqueous solution with a detection limit of 30 nM and 160 nM via IFE and PET mechanisms, respectively. The N-CDs played a major role in the detection of nitro-explosives and harmful noxious metal ions in an analysis of a real water sample. Excellent results were also obtained in the detection of pollutants using a low-cost fabricated paper-based probe system. In addition to environmental monitoring, the excellent properties of the prepared N-CDs were utilized so that they could be employed as fluorescence-based anti-counterfeiting and ink agents for low-cost security and ink applications. These N-CDs will enable the usage of evolving nanomaterials as potential candidates for a new class of pH sensors and exclusively for detection by portable devices, as well as for bioimaging applications.
Author contributions
Kumaresan Annamalai – design and performance of experiments, analysis of experimental data, writing – original draft, Arun Annamalai – conceptualization, methodology, reviewing and editing, Ramya Ravichandran – conceptualization, methodology, reviewing and editing, Anandhavalli Jeevarathinam – conceptualization, methodology, reviewing and editing, Padmanaban Annamalai – conceptualization, methodology, reviewing and editing, Hector Valdes – conceptualization, methodology, reviewing and editing, Sundaravadivel elumalai – visualization, investigation, project administration, and supervision.
Conflicts of interest
There are no conflicts of interest to declare.
Acknowledgements
The authors acknowledge the SRM Institute of Science and Technology for providing the lab and instrumentation facilities at the NRC-SRMIST and the HRTEM facility at SCIF set up with support from MNRE (Project No. 31/03/2015-15/PVSE-R&D), Government of India. Author AP acknowledges FONDECYT/ANID (2022) Post-doctoral Project No. 3220176, funded by the Government of Chile.
References
-
A. L. Rogach, et al., Organization of Matter on Different Size Scales: Monodisperse Nanocrystals and Their Superstructures.
- J. Kaur,
et al., Glutathione Modified Fluorescent CdS QDs Synthesized Using Environmentally Benign Pathway for Detection of Mercury Ions in Aqueous Phase, J. Fluoresc., 2020, 30, 773–785 CrossRef CAS.
- D. Song,
et al., Optically induced insulator-to-semiconductor transition in fluorescent carbon quantum dots measured by terahertz time-domain spectroscopy, Carbon N Y, 2021, 174, 741–749 CrossRef CAS.
- H. Zhang,
et al., A fluorescent quenching performance enhancing principle for carbon nanodot-sensitized aqueous solar cells, Nano Energy, 2015, 13, 124–130 CrossRef CAS.
- A. Tyagi, K. M. Tripathi, N. Singh, S. Choudhary and R. K. Gupta, Green synthesis of carbon quantum dots from lemon peel waste: applications in sensing and photocatalysis, RSC Adv., 2016, 6, 72423–72432 RSC.
- H. Li, Z. Kang, Y. Liu and S. T. Lee, Carbon nanodots: synthesis, properties and applications, J. Mater. Chem., 2012, 22, 24230–24253 RSC.
- Q. Xu,
et al., Synergistic effect of electrode defect regulation and Bi catalyst deposition on the performance of iron–chromium redox flow battery, Chin. Chem. Lett., 2023, 34 DOI:10.1016/j.cclet.2023.108188.
- Y. Wang and A. Hu, Carbon quantum dots: synthesis, properties and applications, J. Mater. Chem. C, 2014, 2, 6921–6939 RSC.
- S. N. Baker and G. A. Baker, Luminescent carbon nanodots: emergent nanolights, Angew. Chem., Int. Ed., 2010, 49, 6726–6744, DOI:10.1002/anie.200906623 Preprint at.
- X. Xu,
et al., Electrophoretic analysis and purification of fluorescent single-walled carbon nanotube fragments, J. Am. Chem. Soc., 2004, 126, 12736–12737 CrossRef CAS PubMed.
- Y. P. Sun,
et al., Quantum-sized carbon dots for bright and colorful photoluminescence, J. Am. Chem. Soc., 2006, 128, 7756–7757 CrossRef CAS PubMed.
- A. B. Bourlinos,
et al., Photoluminescent carbogenic dots, Chem. Mater., 2008, 20, 4539–4541 CrossRef CAS.
- L. Han,
et al., Nanosized carbon particles from natural gas soot, Chem. Mater., 2009, 21, 2803–2809 CrossRef.
- X. Wang,
et al., Bandgap-like strong fluorescence in functionalized carbon nanoparticles, Angew. Chem., Int. Ed., 2010, 49, 5310–5314 CrossRef CAS.
- Y. Mao, Y. Bao, D. Han, F. Li and L. Niu, Efficient one-pot synthesis of molecularly imprinted silica nanospheres embedded carbon dots for fluorescent dopamine optosensing, Biosens. Bioelectron., 2012, 38, 55–60 CrossRef CAS.
- X. Wang,
et al., Photoinduced electron transfers with carbon dots, Chem. Commun., 2009, 3774–3776, 10.1039/b906252a.
- M. K. Barman and A. Patra, Current status and prospects on chemical structure driven photoluminescence behaviour of carbon dots, J. Photochem. Photobiol., C, 2018, 37, 1–22, DOI:10.1016/j.jphotochemrev.2018.08.001.
- L. Wang and H. S. Zhou, Green synthesis of luminescent nitrogen-doped carbon dots from milk and its imaging application, Anal. Chem., 2014, 86, 8902–8905 CrossRef CAS PubMed.
- P. Zhu,
et al., Exploring multi-element co-doped carbon dots as dual-mode probes for fluorescence/CT imaging, Chem. Eng. J., 2023, 470 DOI:10.1016/j.cej.2023.144042.
- F. Arcudi, L. Ďorďević and M. Prato, Design, Synthesis, and Functionalization Strategies of Tailored Carbon Nanodots, Acc. Chem. Res., 2019, 52, 2070–2079 CrossRef CAS.
- H. Ding, S. B. Yu, J. S. Wei and H. M. Xiong, Full-color light-emitting carbon dots with a surface-state-controlled luminescence mechanism, ACS Nano, 2016, 10, 484–491 CrossRef CAS.
- D. Wang, W. U. Khan and Y. Wang, Solid-state carbon dots with efficient cyan emission towards white light-emitting diodes, Chem. – Asian J., 2019, 14, 286–292 CrossRef CAS PubMed.
- Y. Chen,
et al., A Self-Quenching-Resistant Carbon-Dot Powder with Tunable Solid-State Fluorescence and Construction of Dual-Fluorescence Morphologies for White Light-Emission, Adv. Mater., 2016, 28, 312–318 CrossRef CAS PubMed.
- Y. Peng, A. J. Zhang, M. Dong and Y. W. Wang, A colorimetric and fluorescent chemosensor
for the detection of an explosive-2,4,6-trinitrophenol (TNP), Chem. Commun., 2011, 47, 4505–4507 RSC.
- J. Li, L. Zhang, P. Li, Y. Zhang and C. Dong, One step hydrothermal synthesis of carbon nanodots to realize the fluorescence detection of picric acid in real samples, Sens. Actuators, B, 2018, 258, 580–588 CrossRef CAS.
- A. Pal, M. P. Sk and A. Chattopadhyay, Conducting Carbon Dot-Polypyrrole Nanocomposite for Sensitive Detection of Picric acid, ACS Appl. Mater. Interfaces, 2016, 8, 5758–5762 CrossRef CAS.
- Y. Wang, X. Chang, N. Jing and Y. Zhang, Hydrothermal synthesis of carbon quantum dots as fluorescent probes for the sensitive and rapid detection of picric acid, Anal. Methods, 2018, 10, 2775–2784 RSC.
- M. Tian, Y. Wang and Y. Zhang, Synthesis of Fluorescent Nitrogen-Doped Carbon Quantum Dots for Selective Detection of Picric Acid in Water Samples, J. Nanosci. Nanotechnol., 2018, 18, 8111–8117 CrossRef CAS PubMed.
- T. Anusuya, V. Kumar and V. Kumar, Hydrophilic graphene quantum dots as turn-off fluorescent nanoprobes for toxic heavy metal ions detection in aqueous media, Chemosphere, 2021, 282, 131019, DOI:10.1016/j.chemosphere.2021.131019.
- H. Yuan,
et al., Fluorescent wood with non-cytotoxicity for effective adsorption and sensitive detection of heavy metals, J. Hazard. Mater., 2021, 416, 126166, DOI:10.1016/j.jhazmat.2021.126166.
- S. Liu, Y. Zhang, S. Gao, T. Fei and T. Zhang, A universal sugar-blowing approach to synthesize fluorescent nitrogen-doped carbon nanodots for detection of Hg(II), Appl. Surf. Sci., 2021, 544, 148725, DOI:10.1016/j.apsusc.2020.148725.
- S. Zhao,
et al., Green production of fluorescent carbon quantum dots based on pine wood and its application in the detection of Fe3+, J. Cleaner Prod., 2020, 263, 121561, DOI:10.1016/j.jclepro.2020.121561.
- K. Qu, J. Wang, J. Ren and X. Qu, Carbon dots prepared by hydrothermal treatment of dopamine as an effective fluorescent sensing platform for the label-free detection of iron(III) ions and dopamine, Chem. – Eur. J., 2013, 19, 7243–7249 CrossRef CAS PubMed.
- Y. Su,
et al., Facile preparation of fluorescent polydihydroxyphenylalanine nanoparticles for label-free detection of copper ions, Sens. Actuators, B, 2016, 225, 334–339 CrossRef CAS.
- J. Shangguan,
et al., Highly Fe3+-Selective Fluorescent Nanoprobe Based on Ultrabright N/P Codoped Carbon Dots and Its Application in Biological Samples, Anal. Chem., 2017, 89, 7477–7484 CrossRef CAS PubMed.
- H. Tan, Y. Zhang and Y. Chen, Detection of mercury ions (Hg2+) in urine using a terbium chelate fluorescent probe, Sens. Actuators, B, 2011, 156, 120–125 CrossRef CAS.
- P. Zhu,
et al., Bi-doped carbon quantum dots functionalized liposomes with fluorescence visualization imaging for tumor diagnosis and treatment, Chin. Chem. Lett., 2023, 108689, DOI:10.1016/j.cclet.2023.108689.
- X. Gong,
et al., Facile synthesis of nitrogen-doped carbon dots for Fe3+ sensing and cellular imaging, Anal. Chim. Acta, 2015, 861, 74–84 CrossRef CAS.
- T. Xia,
et al., A Terbium Metal–Organic Framework for Highly Selective and Sensitive Luminescence Sensing of Hg2+ Ions in Aqueous Solution, Chem. – Eur. J., 2016, 22, 18429–18434 CrossRef CAS PubMed.
- R. N. C. S. Carvalho,
et al., Multi-element determination of copper, iron, nickel, manganese, lead and zinc in environmental water samples by ICP OES after solid phase extraction with a C18 cartridge loaded with 1-(2-pyridylazo)-2-naphthol, Anal. Methods, 2015, 7, 8714–8719 RSC.
- X. Chen, K. Liu, J. Cai, D. Zhu and H. Chen, Identification of heavy metal-contaminated Tegillarca granosa using infrared spectroscopy, Anal. Methods, 2015, 7, 2172–2181 RSC.
- A. O. Ogunfowokan,
et al., Determination of Heavy Metals in Urine of Patients and Tissue of Corpses by Atomic Absorption Spectroscopy, Chem. Africa, 2019, 2, 699–712 CrossRef CAS.
- H. Zhang,
et al., Solid-phase synthesis of highly fluorescent nitrogen-doped carbon dots for sensitive and selective probing ferric ions in living cells, Anal. Chem., 2014, 86, 9846–9852 CrossRef CAS PubMed.
- V. Ramanan,
et al., Outright green synthesis of fluorescent carbon dots from eutrophic algal blooms for in vitro imaging. ACS Sustain, Chem. Eng., 2016, 4, 4724–4731 CAS.
- Q. Xu,
et al., Machine learning guided microwave-assisted quantum dot synthesis and an indication
of residual H2O2 in human teeth, Nanoscale, 2022, 14, 13771–13778 RSC.
- A. Annamalai,
et al., Simple synthesis of water-soluble ruthenium carry CDs as admirable probe for improved environment and biological applications, Mater. Today Chem., 2023, 30, 101536, DOI:10.1016/j.mtchem.2023.101536.
- Y. Sun, C. Shen, J. Wang and Y. Lu, Facile synthesis of biocompatible N,S-doped carbon dots for cell imaging and ion detecting, RSC Adv., 2015, 5, 16368–16375 RSC.
- R. Purbia and S. Paria, A simple turn on fluorescent sensor for the selective detection of thiamine using coconut water derived luminescent carbon dots, Biosens. Bioelectron., 2016, 79, 467–475 CrossRef CAS PubMed.
- A. Annamalai, K. Annamalai, R. Ravichandran, S. Bharathkumar and S. Elumalai, Multi-functional carbon dots from simple precursors: an excellent heavy metal ions sensor with photocatalytic activity in aqueous environment, Colloids Surf., A, 2022, 652, 129800, DOI:10.1016/j.colsurfa.2022.129800.
- K. Annamalai, A. Annamalai, R. Ravichandran and S. Elumalai, Recyclable waste Dry-cell batteries derived carbon dots (CDs) for detection of Two-fold metal ions and degradation of BTB dye, Waste Manage., 2023, 163, 61–72 CrossRef CAS.
- Z. Liang, M. Kang, G. F. Payne, X. Wang and R. Sun, Probing Energy and Electron Transfer Mechanisms in Fluorescence Quenching of Biomass Carbon Quantum Dots, ACS Appl. Mater. Interfaces, 2016, 8, 17478–17488 CrossRef CAS PubMed.
|
This journal is © The Royal Society of Chemistry and the Centre National de la Recherche Scientifique 2024 |