DOI:
10.1039/D3NJ04494G
(Paper)
New J. Chem., 2024,
48, 96-104
Micelle assisted synthesis of bismuth oxide nanoparticles for improved chemocatalytic degradation of toxic Congo red into non-toxic products†
Received
26th September 2023
, Accepted 16th November 2023
First published on 17th November 2023
Abstract
Water released by industries that generate coloured pollutants must be treated to safeguard the environment and ensure that the treated water is non-toxic. In this study, we demonstrate the utility of glycine-derived designer micelles, N-lauroyl-N-(pyridin-2-ylmethyl)glycine (PyN12G), as a capping agent to prepare black bismuth oxide nanoparticles (Bi2O3 NPs). The obtained Bi2O3 NPs were confirmed by X-ray photoelectron spectroscopy and energy-dispersive X-ray spectroscopy. Transmission electron microscopy demonstrated that the Bi2O3 NPs are crystalline, with a quasi-spherical morphology and an average size of 42.3 nm, which are further corroborated by powder X-ray diffraction studies to be monoclinic. Fourier transform infrared spectra revealed that the surface of Bi2O3 NPs was stabilized by PyN12G and that the –COOH group of the micelles was involved in surface functionalization. The application of Bi2O3 NPs in environmental remediation was demonstrated by degrading a toxic dye, Congo red (CR). Bi2O3 NPs catalyze sodium borohydride-mediated CR degradation with an efficiency of 88.8% in 15 minutes and a rate constant of 1.92 × 10−3 s−1. Besides CR, Bi2O3 NPs catalyzed the degradation of methylene blue, bromophenol blue, Coomassie blue, crystal violet, and rhodamine B with efficiencies of 95%, 92%, 95%, 90%, and 86%, respectively. Gas chromatography-mass spectrometry analysis reveals that CR is degraded into smaller molecules. The disclosed dye degradation process eliminates the hazardous feature of the dye, which was proven by the seed germination assay. Furthermore, toxicology experiments revealed that Bi2O3 NPs do not affect bacterial growth or seed germination, proving that the NPs are non-toxic and safe to use as a chemocatalyst to eliminate toxic dyes without affecting the environment.
Introduction
Dyes are compounds with a wide range of applications, primarily in the colouring of textiles, precious stones, leather, paper, polymers, and food. About 8 × 105 tonnes of synthetic dyes are produced annually.1 The textile industry accounts for over 75% of the global dye business, with over 10
000 dyes used to colour and print different textiles.2 When colours or pigments are used excessively, some dyes do not adhere adequately to textiles and are discharged into the environment, contaminating surface water and groundwater. Congo red (CR), a benzidine-based anionic diazo dye, is one of the most used dyes in the textile industry.3 This dye can break down into benzidine, which needs to be removed from industrial effluents, since it harms flora and fauna and can cause human cancer.4 Removing synthetic dyes from wastewater via physical and chemical processes necessitates expensive modern technology, which is sometimes ineffective and energy-intensive.5
Removing hazardous organic pollutants from wastewater using nanoparticles (NPs) is a unique strategy and a viable alternative to the existing procedures.6 Among the nanomaterials, metals and metal oxides such as Ag, Au, Cu, ZnO, CuO, TiO2, Fe2O3, Fe2O4, and Mn2O3 have been widely investigated for degrading a variety of organic dyes.7–11 Nanocomposites prepared using greener routes were explored as heterogeneous catalysts for effective degradation of organic dyes.12–16 These metal oxides display good degradation efficiency, but concern over the toxicity of NPs hampered their development. The metal oxidation state significantly influences the toxicity of NPs. For example, compared to Fe2+ in Fe2O4, Fe3+ in Fe2O3 is more toxic and causes more DNA oxidation.17 The concentration and properties of NPs, like size, shape, capping agents and surface functionalization, also modulate their toxicity. Shalani et al. showed that ZnO NPs are more genotoxic, while ZnO microparticles are cytotoxic.18 Kose et al. have demonstrated that positively surface functionalized TiO2 NPs are slightly more toxic than the negatively charged ones against A459 cell lines.19 Hence, it is imperative to assess the toxic effects of NPs and their environmental applications. To remove unwanted colours (dye hues) from wastewater, we have developed viable, bismuth oxide NPs (Bi2O3 NPs) for chemocatalytic dye degradation and reported their biocompatibility by examining their nanotoxicity. Bi2O3 is known for its semiconducting properties and its band gap ranges from 2.47 to 3.55 eV.20,21
Bismuth was chosen for this investigation because bismuth-based nanostructures have applications in solid oxide fuel cells, gas sensors, photocatalysts, energy, and medicine.22–24 Many papers describe the photocatalytic activity of metal and metal oxide NPs,25,26 but light sources like sunlight are not constant throughout the day and night. Hence, photoreactors are used for photocatalytic reactions, which require a skilled technician.27 An alternative method is the use of a chemocatalyst, the surface of which contributes to its activity.28 Nanodimensional materials possess a higher surface-to-volume ratio and are hence more suitable for chemocatalysis. The chemical preparation steps play a key role in obtaining high-quality NPs, and therefore, a micelle-assisted synthesis method was selected because micelles allow the reactants to solubilise separately and prevent the NPs from agglomeration. Recently, we demonstrated the application of N-acyl-N-(pyridin-2-ylmethyl)glycine micelles in the organic green synthesis of chromenoquinoline.29 In this paper, the potential of N-lauroyl-N-(pyridin-2-ylmethyl)glycine (PyN12G) micelles in synthesizing oxygen-deficient black Bi2O3 NPs is demonstrated for the first time and the application of these NPs in the effective degradation of CR is also demonstrated.
Results and discussion
Synthesis and characterization of bismuth oxide nanoparticles
Bi2O3 NPs were synthesized by the chemical reduction of bismuth ions in PyN12G micelles in an aerobic atmosphere at room temperature (Fig. 1(A)). For synthesis, the PyN12G micelles were solubilised in DDW and the pH was adjusted to 12 by adding aqueous NaOH. The transparent alkaline PyN12G solution turns milky white, while adding Bi(NO3)3·5H2O, suggesting the formation of bismuth oxide hydrates. When NaBH4 is added, the suspension turns black, but after 12 hours of air exposure, the solution begins to aggregate into a larger black residue that settles at the bottom of the flask. Thus, diethylamine was added to the suspension before reduction with NaBH4. An attempt to prepare Bi2O3 NPs only with diethylamine is unsuccessful, indicating that PyN12G micelles play a key role in forming Bi2O3 NPs, and diethylamine co-stabilizes the NPs to prevent agglomeration. The deep black Bi2O3 NPs in the solution phase stay without agglomeration in open air for more than 6 months, suggesting that the formed Bi2O3 NPs are stable. The optical band gap energy of Bi2O3 NPs was determined by using UV-vis diffuse reflectance spectroscopy (see ESI,† Fig. S1). The band gap calculated using the Tauc equation yields a value of 3.72 eV, which is higher than the reported band gap of photocatalytic Bi2O3 NPs (2.8–2.93 eV). The obtained higher value can be attributed to the smaller size of the NPs and also suggests that the material may not be suitable for visible light photocatalysis but it may be useful as a catalyst in the UV region.20,21
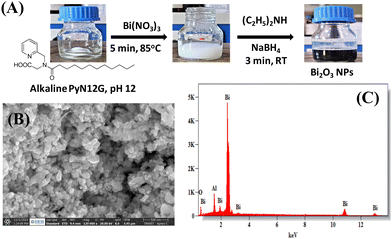 |
| Fig. 1 (A) Synthesis of Bi2O3 NPs. The structure of the capping agent, PyN12G, is shown. (B) SEM image of Bi2O3 NPs. (C) EDX spectra show that the preparation contains Bi and O. For SEM, the samples were coated on aluminium foil, and hence it appears in EDX. | |
Scanning electron microscopy images of Bi2O3 NPs showed spherical particles with the size ranging from 40–55 nm (Fig. 1(B)). Energy-dispersive X-ray spectroscopy (EDS) used to assess the elemental composition identifies bismuth and oxygen in the preparation, implying that the NPs are made of bismuth oxide (Fig. 1(C)). Generally, Bi2O3 NPs are reported as yellow semiconductors, but any lattice defects, particularly oxide vacancies, may produce black oxides. Earlier studies showed that the high-pressure torsion method produced Bi2O3 NPs exhibiting a black colour due to the large fraction of oxygen vacancies.30 The calcination of the black Bi2O3 NPs was done in an oxygen-enriched atmosphere to obtain yellow Bi2O3, suggesting that the surface oxygen vacancies were repaired (see ESI,† Fig. S2). However, further studies are required to confirm the lattice defects. Considering the interest in black oxides as high-energy materials,31,32 we proceed with black Bi2O3 to evaluate its catalytic potential.
The transmission electron microscopy image of the NPs presented in Fig. 1(B) revealed quasi-spherical morphology with a size range from 20–55 nm. The high-resolution TEM micrograph shown in Fig. 2(A) indicated a lattice fringe with a d-spacing of about 0.33 nm, which can be attributed to the (1 2 0) lattice plane of crystalline Bi2O3 NPs. Selected area electron diffraction patterns showed a concentric ring pattern with intermittent bright spots, suggesting that the Bi2O3 NPs are crystalline (inset of Fig. 2(B)). The sharp peaks in powder XRD further reveal that Bi2O3 NPs are crystalline. The XRD pattern matched with the Joint Committee on Powder Diffraction Standards, JCPDS 01-076-1730, which corresponds to the monoclinic phase of Bi2O3 with a space group of P21/c. The crystallite size determined from XRD data using the Scherrer equation was 40.22 nm, which matches closely with TEM analysis. The zeta potential of the Bi2O3 NPs is −19.8 mV, which showed that the electrical boundaries are sufficiently separated to prevent agglomeration (see ESI,† Fig. S3A). The particle size, as determined using the Zetasizer, is 111.8 nm, bigger than the size determined using the TEM (see ESI,† Fig. S3B). This can be understood by considering the fundamental distinction between the techniques, where the Zetasizer measures the hydrodynamic size, while the TEM directly observes the surface morphology. The larger zeta potential values suggest that the NPs are surface functionalized or stabilized by the capping agent, PyN12G micelles.
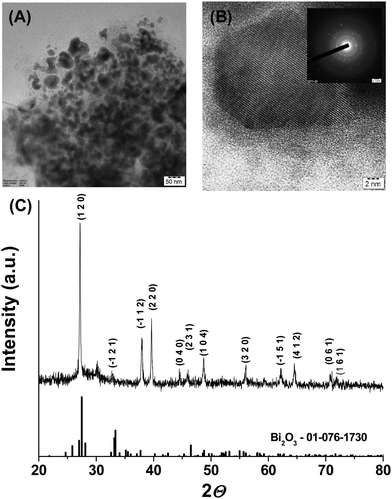 |
| Fig. 2 (A) Transmission electron micrograph showing the quasi-spherical Bi2O3 NPs. (B) High-resolution TEM image depicting the lattice fringe pattern. The inset corresponds to the SAED pattern of Bi2O3 NPs, which confirms that the NPs are crystalline. (C) X-ray diffraction of Bi2O3 NPs. | |
PyN12G micelle stabilized Bi2O3 NPs
The surface composition of the Bi2O3 NPs was analyzed using X-ray photoelectron spectroscopy.33 The XPS survey showed the binding energy peaks corresponding to bismuth, oxygen, carbon and nitrogen (Fig. 3(A)). The binding energy peaks of the 4f orbital of bismuth at 158.9 and 164.2 eV were attributed to the 4f7/2 and 4f5/2 of Bi3+ in Bi2O3 (see ESI,† Fig. S4A) The O 1s spectra are composed of three components, 531.6, 533.1, and 536.14 eV, of which the lower binding energy component is ascribed to lattice oxygen (O
C–) and the higher binding energy component can be attributed to the adsorbed oxygen (see ESI,† Fig. S4B). The binding energy of 533.1 eV was attributed to the oxygen (–C–OH) of PyN12G, the capping agent involved in stabilizing the Bi2O3 NPs. Three components of the C1s binding energy were identified and ascribed to carbon in PyN12G. The peak at 285.1 eV represents the C 1s chemical states of C–C/C–H, whereas the peaks at 286.2 and 288.1 eV are assigned to the –C–OH/C–N and –C=O chemical states, respectively (see ESI,† Fig. S4C). The amide (N 1s) binding energy peak at 399.6 eV indicates that the NPs are capped with PyN12G (see ESI,† Fig. S4D). The FTIR spectra of Bi2O3 NPs were studied to get insight into surface functionalization by the capping agent, PyN12G (Fig. 3(B)). The FTIR spectra of PyN12G showed broad IR stretching vibrations at 3450 cm−1, which account for the combination of carboxylic acid, –OH group and amide –NH stretching. The stretching vibrations corresponding to carboxylic –C=O and amide –C=O were observed at 1721 cm−1 and 1653 cm−1, respectively. The amide –NH bending and carboxylic acid –OH bending were detected at 1600 cm−1 and 1434 cm−1, respectively. The 2918 cm−1 and 2850 cm−1 peaks correspond to –CH stretching vibrations. It is noted from Fig. 3(B) that the peak at 3450 cm−1 is visible for Bi2O3 NPs, indicating that either the –OH or the –NH group is involved in NP stabilization. Surprisingly, the peaks at 1721 cm−1 and 1434 cm−1 are absent, showing that the –COOH group stabilizes the NPs. Other than these groups, the IR vibrations of other groups remain in a similar position, indicating that the surface of Bi2O3 NPs is capped with PyN12G and the –COOH group of the micelles is involved in surface functionalization, which is consistent with XPS analysis.
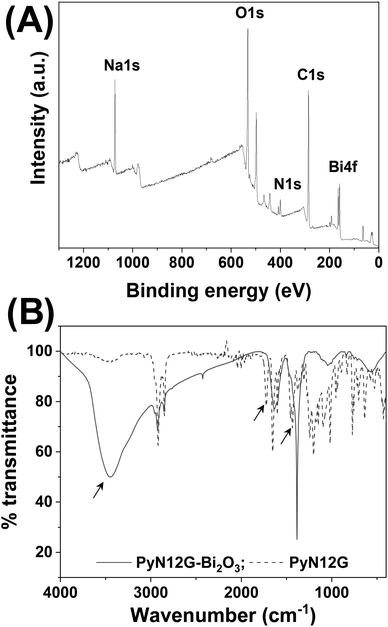 |
| Fig. 3 (A) XPS survey showing the surface elemental composition of Bi2O3 NPs. (B) FTIR spectra depict the functional group involved in stabilizing the NPs. | |
Antioxidant activity of Bi2O3 NPs
The reducing power of Bi2O3 NPs was assessed by antioxidant assay. A popular, quick, simple, and inexpensive method for determining the antioxidant characteristics of compounds is the 2,2-diphenyl-1-picrylhydrazyl (DPPH) method.34 It uses free radicals to determine whether a material can give hydrogen or act as a free-radical scavenger. The stable dark-purple DPPH free radical turns yellow when Bi2O3 NPs are added, suggesting that it has been reduced and transformed into DPPH-H. The reducing power of Bi2O3 NPs was followed by absorption spectroscopy at 517 nm. As seen in Fig. 4, Bi2O3 NPs exhibit a concentration-dependent antioxidant activity of up to 80% at a concentration of 0.5 mM. This finding suggests that Bi2O3 NPs may help in reducing and transforming any harmful reactive species in the environment.
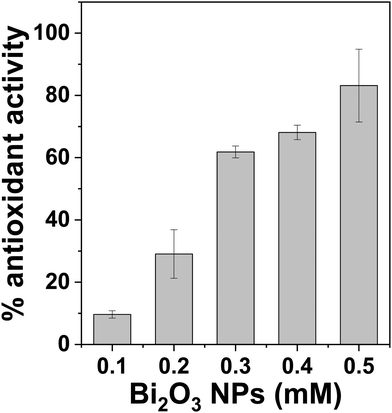 |
| Fig. 4 DPPH radical scavenging activities of Bi2O3 NPs. Experiments are done in triplicates and present mean ± SD. | |
Bi2O3 NPs catalyze dye degradation
The capacity to degrade dyes was evaluated to explore the potential of Bi2O3 NPs in environmental remediation. Dye degradation can be accomplished through either oxidation or reduction.35,36 Herein, we used a sodium borohydride mediated reduction of Congo red. The absorption peak of CR in UV-Vis spectra is at 497 nm. The CR absorbance spectra were barely affected by the addition of NaBH4, even after 15 minutes. However, the addition of NaBH4 in the presence of catalytic amounts of Bi2O3 NPs caused a noticeable shift in the colour of the solution. Also, it caused time-dependent changes in the absorbance spectra (Fig. 5(A)). The dye degradation percentage was calculated to be 88.8% in 15 minutes. The scope of the catalyst was further tested against methylene blue, bromophenol blue, Coomassie blue, crystal violet, and rhodamine B (see ESI,† Fig. S5). The decolourization of methylene blue, bromophenol blue, Coomassie blue, crystal violet, and rhodamine B was reported to be efficiently catalyzed by Bi2O3 NPs, with efficiencies of 95%, 92%, 95%, 90%, and 86%, respectively. This finding demonstrates that Bi2O3 NPs effectively catalyze the decolourization of dyes in the presence of NaBH4. The catalyst was collected by centrifugation at the end of the process to assess its reusability. The catalyst was dried at 60 °C and washed with distilled water before being used in the subsequent reduction cycles. The catalyst was shown to decolorize CR with an efficiency of 80–85% after being used five times in succession for the NaBH4-mediated reduction, suggesting that Bi2O3 is reusable.
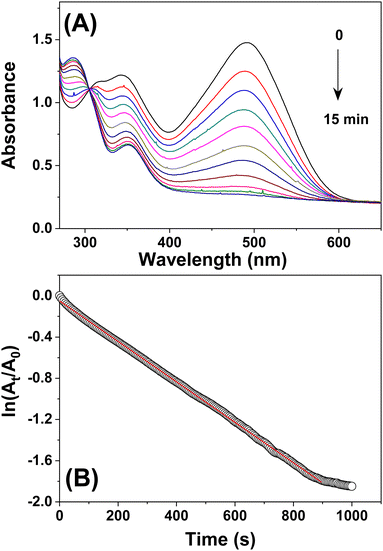 |
| Fig. 5 Dye degradation. (A) UV-visible spectra of Congo red in the presence of Bi2O3 NPs + NaBH4. The arrow indicates the spectra measured at different time points. (B) Kinetic trace fitted with a pseudo-first order equation. The slope obtained from the linear portion of the data was used to calculate the rate constant. The red line depicts the linear portion. | |
Monitoring the changes in CR absorbance allowed us to determine the rate of dye decolourization. As seen in Fig. 5(B), adding the catalyst and the reducing agent affected the absorption maximum significantly. The reaction was completed within 900 s, as evidenced by the decreased absorption peak intensity at 497 nm and the loss of red colour. The reduction kinetics can be explained by a pseudo-first-order rate law concerning CR alone because the concentration of NaBH4 is significantly higher than that of CR. Therefore, the equation ln (At/A0) = −kt, where k is the apparent rate constant and t is the reaction time, can characterize the kinetics. The absorbances of CR at times t and 0 are represented as At and A0, respectively. As a result of the direct proportionality between CR concentration and absorbance intensity in the medium, the ratio of absorbance (At/A0) is considered equal to the ratio of CR concentration (Ct/C0). A linear relationship existed between ln (At/A0) and reaction time. Thus, the rate constant was calculated directly from the linear section of the kinetic trace's slope as 1.92 × 10−3 s−1.
The likely mechanism of catalytic dye degradation is as follows: (i) NaBH4 aqueous solution releases the hydride ion, which then adsorbs on the catalytic surface, (ii) the dyes are adsorbed on the Bi2O3 NPs to activate the catalyst surface, and (iii) the closer proximity of the hydride ion and dyes on the catalytic surface causes the dyes to be reduced and released. The obtained k values were comparable to those reported for substrate-supported metallic nanoparticles.37–39
GC–MS analysis of the degraded product
To get an insight into the degradation products, the GC–MS spectra of Congo red after degradation were recorded.40 It is noted from Fig. S6 (ESI†) that the retention time of degraded Congo red is 7.450 min, and MS analysis of the peak at 7.450 min shows multiple peaks. The m/z values were compared to the NIST library of molecules. Based on the comparison with the NIST library, we proposed a plausible mechanistic pathway for the catalytic Bi2O3 NP mediated degradation of the Congo red dye (see ESI,† Fig. S6). The Bi2O3 NPs catalyze the cleavage of the azo bond of Congo red, forming benzidine, naphthalene sulfonic acid, naphthylamine and methyl benzene products. Initially, the Congo red dye undergoes homolytic azo bond cleavage into benzidine (m/z = 184) and two molecules of the naphthalene intermediate (m/z = 271). This naphthalene intermediate upon further deamination (m/z = 244) followed by desulfonation (m/z = 142) or deamination (m/z = 207) formed the sulfonated naphthalene product, which, upon further degradation, gave methyl benzene (m/z = 91) as a smaller fragmented product. However, additional studies are required to fully understand all the mass fragmentation and other potential molecular pathways to support the experimental data.
Assessment of degraded dye water toxicity
Nanotoxicity is the major concern in developing nanomaterial applications.41 Hence, the nanotoxicity of Bi2O3 was first evaluated using a seed germination assay because polluted soil can affect plant growth.42 To examine the phytotoxicity, the chickpea seeds were soaked in Bi2O3 NPs (25, 50, 100, and 200 μM) for 24 h and transferred to a cotton cloth for sprouting. The seeds soaked in tap and saline water were used as positive and negative controls, respectively. After three days, the shoot and root lengths were measured and the ratio of the shoot to root length was plotted with respect to the concentration of Bi2O3 NPs (Fig. 6(A)). As seen in Fig. 6(A), the seed germination was unaffected even at the highest measured concentration, which is equivalent to a concentration eight times greater than the catalytic concentration. Notably, seeds exposed to saline water displayed slowed germination. Next, the influence of Bi2O3 NPs on microbiota was assessed by the zone of inhibition (ZOI) test,43 an accepted quick method to assess the bactericidal properties of any product or surface (see ESI,† Fig. S7). Bi2O3 NPs were evaluated at three concentrations (25 μM, 100 μM, and 200 μM) against uropathogenic Escherichia coli (UPEC). It is clear from Fig. S7 (ESI†) that the standard antibiotic ciprofloxacin is the only one showing the ZOI, suggesting that NPs are non-toxic to the bacteria. These findings reveal that Bi2O3 NPs may not harm soil or environmental microbiota and can be employed as a non-toxic nanomaterial for environmental remediation. The study aimed to show that the dye toxicity may be eliminated by using Bi2O3 NPs. It is known that CR can break down into toxic benzidine.4 The phytotoxicity of CR and chemo-catalytically degraded CR was therefore evaluated. As seen in Fig. 6(B), CR prevented seed germination, but seedlings exposed to CR water that had undergone dye degradation grew like control seeds. This result implies that CR becomes non-toxic as a result of catalytic degradation.
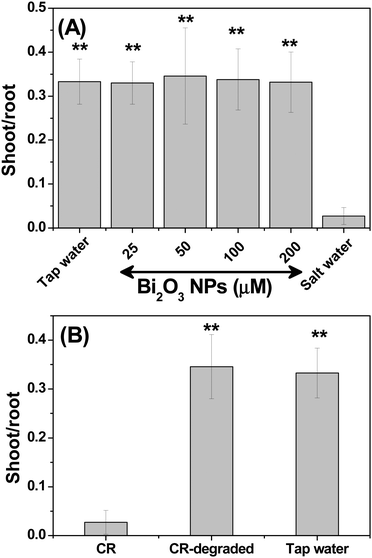 |
| Fig. 6 Seed germination assay. (A) The ratio of shoot to root length of Bi2O3 NP treated chickpea seeds. Seeds exposed to tap and saline water without NPs are positive and negative controls, respectively. (B) The ratio of shoot to root length of chickpea exposed to Congo red and catalytically degraded Congo red water. | |
Experimental
Materials
Bismuth nitrate pentahydrate, diethylamine and sodium cyanoborohydride were purchased from Avra, India. 2,2-Diphenyl-1-picrylhydrazyl (DPPH), Congo red (CR), and dodecanoylchloride were bought from Sigma, India. Glycine ester, 2-pyridinecarboxaldehyde, and lithium hydroxide were supplied by Tokyo Chemical Industries, India. Escherichia coli (MTCC729) and Staphylococcus aureus (MTCC3160) were obtained from the Institute of Microbial Technology, India. The N-dodecanoyl-N-(pyridine-2-ylmethyl) glycine (PyN12G) molecule was synthesized using a reported procedure.20 Briefly, glycine ester and 2-pyridinecarboxaldehyde reacted to form an imine, which was then in situ reduced with sodium cyanoborohydride. Following column chromatography purification, the amine was treated with dodecanoylchloride in the presence of triethylamine to produce N-acyl amide. PyN12G is obtained by deblocking the ester group with lithium hydroxide. All the other chemicals and solvents used were of analytical grade.
Synthesis of Bi2O3 NPs
PyN12G (8 mM) was dissolved in distilled water at 80 °C and brought to pH 12 using NaOH. Separately, a 10 mM Bi(NO3)3 stock solution was made in distilled water. 5 mL of PyN12G and 5 mL of Bi(NO3)3 were mixed at 1000 rpm for 15 min at 25 °C to produce a milky colloidal solution. About 10 μL of diethylamine was added to this reaction mixture and then reduced using 15 mM NaBH4. The colour changes from milky white to black, suggesting the formation of Bi2O3 NPs.
Characterization of Bi2O3 NPs
High-resolution transmission electron microscopy (HRTEM) was performed using a JEOL Japan, JEM-2100 Plus, operating at an acceleration voltage of 200 kV. The samples for HRTEM were prepared by drop casting 10 μL of Bi2O3 NPs on a carbon-coated copper grid, air-dried and subjected to vacuum before measurement. Bi2O3 NPs collected by centrifugation at 14
000 rpm for 30 min were dried at 60 °C and subjected to powder X-ray diffraction using a BRUKER USA D8 Advance. X-ray photoelectron spectroscopy (XPS) measurements were performed on the Thermo Fisher Scientific, UK, Kα surface analysis model using Al-X-ray in a wide range of 0–1350 eV. The Fourier transform infrared spectrum of the NPs was recorded on a PerkinElmer spectrum-one instrument in the diffuse reflectance mode at a resolution of 1 cm−1 in KBr pellets. The zeta potential and zeta size of aqueous solution (1
:
10 diluted) of Bi2O3 NPs were measured using a Zetasizer 1000 HS (Malvern Instruments, UK).
Antioxidant assay
About 1 mL of DPPH solution prepared in methanol was added to different concentrations of Bi2O3 NPs, and the final volume was adjusted to 5 mL using methanol. The solution was incubated in the dark for 10 min, and the absorbance at 517 nm was measured using a Thermo Scientific Evolution 201 UV-Vis spectrophotometer. DPPH solution without NPs serves as a control. The antioxidant activity was calculated using eqn (1): | % antioxidant activity = [(Ac – As)/Ac] × 100 | (1) |
where Ac and As are the absorbances of the control and sample, respectively.
Catalytic activity
The colorimetric properties of Congo red were used to determine the catalytic activity of Bi2O3 NPs. Briefly, 3 mL of 0.1 mM CR aqueous solution in the presence of 50 μM Bi2O3 NPs was treated with 100 μL of NaBH4 (1 mg). The colorimetric changes were monitored visually and also using a UV-Visible spectrometer. The time-dependent changes in CR absorbance at 497 nm were followed for the kinetic analysis. The degradation percentage was estimated using eqn (2) | Degradation % = [(A0 – At)/A0] × 100 | (2) |
where A0 and At are the absorbances at the initial time and time t, respectively.
Gas chromatography–mass spectrometry (GC–MS)
A GCMS-QP2010 Plus Shimadzu System with a 60-meter capillary column was used for the measurement. The measurement was done in the temperature programming mode at the initial temperature for 1 minute, and it was linearly increased at 10 °C intervals up to 250 °C for 2 minutes. The temperature of the GC-MS interface was 250 °C. The flow rate of 1 mL per minute was maintained. Helium was used as a carrier gas with a 1.0 mL min−1 flow rate. The degraded products were identified by comparing the fragmentation pattern's mass spectra (m/z) with the NIST library list of fragmented species.
Phytotoxicity
Chickpea seeds purchased from the local stores in Thanjavur, India were used as a model to estimate the phytotoxicity of the nanoparticles, the dye water and the degraded dye water. Typically, 10 chickpea seeds of approximately equal weight were soaked in 50 mL of water containing Bi2O3 NPs or dye or degraded dye solution for 24 h. The soaked seeds were transferred to a cotton cloth for sprouting for three days. Seeds soaked in tap water served as control. After three days, root and shoot development was measured using a thread and the thread length was measured with a ruler. The experiments were repeated in five different batches to arrive at statistical significance. The statistically significant root and shoot length difference for each group compared to the control was determined using a paired t test at α = 0.05.
Conclusions
Black Bi2O3 NPs stabilized by designer micelles, PyN12G, were synthesized at room temperature. XRD, XPS, EDAX, SEM, TEM and FTIR were used to characterize the structure, elemental composition, morphology and surface of the Bi2O3 NPs. The chemocatalytic activity of the Bi2O3 NPs was analyzed in detail with Congo red (CR), and it was found that the degradation efficiency was 88.8% in 5 min at room temperature. The catalyst was reused for five cycles in the hydride transfer reaction without losing the degradation efficiency. The potential of the NPs to degrade other dyes is also demonstrated. The toxicology study proves that the Bi2O3 NPs and the degraded dye water are non-toxic, which implies that the chemocatalytic technology that has been successfully portrayed in this work can be a viable eco-friendly methodology to mitigate dye pollution in wastewater.
Author contributions
AP, SM – synthesis and catalysis; DKS – phytotoxicity; PN, MM – characterization; AS – GC analysis; FA – bacteriotoxicity; and MG – data analysis. VA designed the project. The manuscript was written through the contributions of all authors.
Conflicts of interest
There are no conflicts to declare.
Acknowledgements
The teaching assistantship provided to AP and DKS by SASTRA Deemed University was gratefully acknowledged. The authors thank the Central Research Facility (R&M/0021/SCBT-007/2012–13), SASTRA Deemed University, for providing infrastructure and SCIF, SRMIST and Nanotechnology Research Center (NRC), SRMIST for providing the instrumentation facility. This research was also funded by the Researchers Supporting Project number (RSP2023R364), King Saud University, Riyadh, Saudi Arabia.
Notes and references
- D. Bhatia, N. R. Sharma, J. Singh and R. S. Kanwar, Biological methods for textile dye removal from wastewater: a review, Crit. Rev. Environ. Sci. Technol., 2017, 47, 1836–1876, DOI:10.1080/10643389.2017.1393263.
- S. Madhav, A. Ahamad, P. Singh and P. K. Mishra, A review of textile industry: wet processing, environmental impacts, and effluent treatment methods, Environ. Qual. Manag., 2018, 27, 31–41, DOI:10.1002/tqem.21538.
- S. Mandal, J. Calderon, S. B. Marpu, M. A. Omary and S. Q. Shi, Mesoporous activated carbon as a green adsorbent for the removal of heavy metals and Congo red: Characterization, adsorption kinetics, and isotherm studies, J. Contam. Hydrol., 2021, 243, 103869, DOI:10.1016/j.jconhyd.2021.103869.
- M. Hernández-Zamora and F. Martínez-Jerónimo, Congo red dye diversely affects organisms of different trophic levels: a comparative study with microalgae, cladocerans, and zebrafish embryos, Environ. Sci. Pollut. Res. Int., 2019, 26(12), 11743–11755, DOI:10.1007/s11356-019-04589-1.
- R. Al-Tohamy, S. S. Ali, F. Li, K. M. Okasha, Y. A. Mahmoud, T. Elsamahy, H. Jiao, Y. Fu and J. Sun, A critical review on the treatment of dye-containing wastewater: Ecotoxicological and health concerns of textile dyes and possible remediation approaches for environmental safety, Ecotoxicol. Environ. Saf., 2022, 231, 113160, DOI:10.1016/j.ecoenv.2021.113160.
- W. B. Mbarek, L. Escoda, J. Saurina, E. Pineda, F. M. Alminderej, M. Khitouni and J. J. Suñol, Nanomaterials as a Sustainable Choice for Treating Wastewater: A Review, Materials, 2022, 15(23), 8576, DOI:10.3390/ma15238576.
- Y. Thakare, S. Kore, I. Sharma and M. Shah, A comprehensive review on sustainable greener nanoparticles for efficient dye degradation, Environ. Sci. Pollut. Res. Int., 2022, 29(37), 55415–55436, DOI:10.1007/s11356-022-20127-y.
- S. Megarajan, F. Ameen, D. Singaravelu, M. A. Islam and A. Veerappan, Synthesis of N-myristoyltaurine stabilized gold and silver nanoparticles: Assessment of their catalytic activity, antimicrobial effectiveness and toxicity in zebrafish, Environ Res., 2022, 212(Pt A), 113159, DOI:10.1016/j.envres.2022.113159.
- K. B. Ayaz Ahmed, S. Subramanian, A. Sivasubramanian, G. Veerappan and A. Veerappan, Preparation of gold nanoparticles using Salicornia brachiata plant extract and evaluation of catalytic and antibacterial activity, Spectrochim. Acta, Part A, 2014, 130, 54–58, DOI:10.1016/j.saa.2014.03.070.
- S. Taghavi Fardood, A. Ramazani and P. A. Asiabi,
et al., A Novel Green Synthesis of Copper Oxide Nanoparticles Using a Henna Extract Powder, J. Struct. Chem., 2018, 59, 1737–1743, DOI:10.1134/S0022476618070302.
- S. Taghavi Fardood, A. Ramazani and S. Moradi,
et al., Green synthesis of zinc oxide nanoparticles using arabic gum and photocatalytic degradation of direct blue 129 dye under visible light, J. Mater. Sci.: Mater. Electron., 2017, 28, 13596–13601, DOI:10.1007/s10854-017-7199-5.
- F. Moradnia, S. T. Fardood, A. Ramazani and V. K. Gupta, Green synthesis of recyclable MgFeCrO4 spinel nanoparticles for rapid photodegradation of direct black 122 dye, J. Photochem. Photobiol., A, 2020, 392, 112433, DOI:10.1016/j.jphotochem.2020.112433.
- F. Moradnia, S. Taghavi Fardood, A. Ramazani, B. ki Min, S. W. Joo and R. S. Varma, Magnetic Mg0.5Zn0.5FeMnO4 nanoparticles: Green sol–gel synthesis, characterization, and photocatalytic applications, J. Cleaner Prod., 2021, 288, 125632, DOI:10.1016/j.jclepro.2020.125632.
- F. Moradnia, S. Taghavi Fardood, A. Ramazani, S. Osali and I. Abdolmaleki, Green sol–gel synthesis of CoMnCrO4 spinel nanoparticles and their photocatalytic application, Micro Nano Lett., 2020, 15, 674–677, DOI:10.1049/mnl.2020.0189.
- S. Taghavi Fardood, A. Ramazani and Z. Golfar,
et al., Green Synthesis Using Tragacanth Gum and Characterization of Ni–Cu–Zn Ferrite Nanoparticles as a Magnetically Separable Catalyst for the Synthesis of Hexabenzylhexaazaisowurtzitane
Under Ultrasonic Irradiation, J. Struct. Chem., 2018, 59, 1730–1736, DOI:10.1134/S0022476618070296.
- B. Eskandari Azar, A. Ramazani, S. Taghavi Fardood and A. Morsali, Green synthesis and characterization of ZnAl2O4@ZnO nanocomposite and its environmental applications in rapid dye degradation, Optik, 2020, 208, 164129, DOI:10.1016/j.ijleo.2019.164129.
- A. L. Cortajarena, D. Ortega, S. M. Ocampo, A. Gonzalez-García, P. Couleaud, R. Miranda, C. Belda-Iniesta and A. Ayuso-Sacido, Engineering iron oxide nanoparticles for clinical settings, Nanobiomedicine, 2014, 1, 2, DOI:10.5772/58841.
- D. Shalini, S. Senthilkumar and P. Rajaguru, Effect of size and shape on toxicity of zinc oxide (ZnO) nanomaterials in human peripheral blood lymphocytes, Toxicol. Mech. Methods, 2018, 28(2), 87–94, DOI:10.1080/15376516.2017.1366609.
- O. Kose, M. Tomatis, L. Leclerc, N. B. Belblidia, J. F. Hochepied, F. Turci, J. Pourchez and V. Forest, Impact of the Physicochemical Features of TiO2 Nanoparticles on Their In Vitro Toxicity, Chem. Res. Toxicol., 2020, 33(9), 2324–2337, DOI:10.1021/acs.chemrestox.0c00106.
- Y. Qiu, M. Y. Hongbo Fan, Y. Zuo, Y. Shao, Y. Xu, X. Yang and S. Yang, Nanowires of α- and β-Bi2O3: phase-selective synthesis and application in photocatalysis, CrystEngComm, 2011, 13, 1843–1850, 10.1039/C0CE00508H.
- L. Leontie, Optical properties of bismuth oxide thin films prepared by reactive magnetron sputtering, J. Optoelec. Adv. Mater., 2006, 8, 1221–1224 CAS.
- L. Zhang, P. Ghimire, J. Phuriragpitikhon, B. Jiang, A. A. S. Gonçalves and M. Jaroniec, Facile formation of metallic bismuth/bismuth oxide heterojunction on porous carbon with enhanced photocatalytic activity, J. Colloid Interface Sci., 2018, 513, 82–91, DOI:10.1016/j.jcis.2017.11.011.
- M. Bartoli, P. Jagdale and A. Tagliaferro, A Short Review on Biomedical Applications of Nanostructured Bismuth Oxide and Related Nanomaterials, Materials, 2020, 13(22), 5234, DOI:10.3390/ma13225234.
- M. Shi, M. Zhao, Q. Zheng, L. Jiao, Z. Su, M. Li, X. Zhao, X. Song and S. Yang, Uniform Bi–Bi2O3 nanoparticles/reduced graphene oxide composites for high-performance aqueous alkaline batteries, Dalton Trans., 2022, 51(32), 12114–12124, 10.1039/d2dt01217k.
- S. Yadav, K. Shakya, A. Gupta, D. Singh, A. R. Chandran, A. Varayil Aanappalli, K. Goyal, N. Rani and K. Saini, A review on degradation of organic dyes by using metal oxide semiconductors, Environ. Sci. Pollut. Res. Int., 2023, 30(28), 71912–71932, DOI:10.1007/s11356-022-20818-6.
- M. Mehta, M. Sharma, K. Pathania, P. K. Jena and I. Bhushan, Degradation of synthetic dyes using nanoparticles: a mini-review, Environ. Sci. Pollut. Res. Int., 2021, 28(36), 49434–49446, DOI:10.1007/s11356-021-15470-5.
- D. Bertagna Silva, G. Buttiglieri and S. Babić, State-of-the-art and current challenges for TiO2/UV-LED photocatalytic degradation of emerging organic micropollutants, Environ. Sci. Pollut. Res. Int., 2021, 28(1), 103–120, DOI:10.1007/s11356-020-11125-z.
- S. Megarajan and V. Anbazhagan, N-myristoyltaurine capped copper nanoparticles for selective colorimetric detection of Hg2+ in wastewater and as effective chemocatalyst for organic dye degradation, Microchem. J., 2019, 148, 1–9, DOI:10.1016/j.microc.2019.04.049.
- A. Pious, R. K. Kamlekar, S. Muthusamy, A. Jothi, V. K. K. Praneeth, S. Ramesh and A. Veerappan, Effectiveness of the hydrophobic core of pyridine tethered N-acyl glycine micelles in improving chromenoquinoline synthesis in water, Colloids Surf., A, 2023, 664, 131129, DOI:10.1016/j.colsurfa.2023.131129.
- I. Fujita, P. Edalati, Q. Wang, M. Watanabe, M. Arita, S. Munetoh, T. Ishihara and K. Edalati, Novel black bismuth oxide (Bi2O3) with enhanced photocurrent generation, produced by high-pressure torsion straining, Scr. Mater., 2020, 187, 366–370, DOI:10.1016/j.scriptamat.2020.06.052.
- S. Liu, J. Sun, G. Ren and X. Meng, Vacancy-engineered bismuth-based semiconductor with enhanced photocatalytic activity: A review, Mater. Sci. Semicond. Proc., 2022, 137, 106230, DOI:10.1016/j.mssp.2021.106230.
- X. Wang, Y. Xiang, B. Zhou, Y. Zhang, J. Wu, R. Hu, L. Liu, J. Song and J. Qu, Enhanced photocatalytic performance of Ag/TiO2 nanohybrid sensitized by black phosphorus nanosheets in visible and near-infrared light, J. Colloid Interface Sci., 2019, 534, 1–11, DOI:10.1016/j.jcis.2018.09.013.
- R. Yuvakkumar and S. I. Hong, Structural, compositional and textural properties of monoclinic α-Bi2O3 nanocrystals, Spectrochim. Acta, Part A, 2015, 144, 281–286, DOI:10.1016/j.saa.2015.02.093.
- H. A. Wiggers, M. T. Fin, N. M. Khalil and R. M. Mainardes, Polyethylene Glycol-Stabilized Zein Nanoparticles Containing Gallic Acid, Food Technol. Biotechnol., 2022, 60(2), 145–154, DOI:10.17113/ftb.60.02.22.6981.
- A. I. del Río, J. Molina, J. Bonastre and F. Cases, Influence of electrochemical reduction and oxidation processes on the decolourization and degradation of CI Reactive Orange 4 solutions, Chemosphere, 2009, 75(10), 1329–1337, DOI:10.1016/j.chemosphere.2009.02.063.
- D. Nagarajan and S. Venkatanarasimhan, Copper(II) oxide nanoparticles coated cellulose sponge-an effective heterogeneous catalyst for the reduction of toxic organic dyes, Environ. Sci. Pollut. Res. Int., 2019, 26(22), 22958–22970, DOI:10.1007/s11356-019-05419-0.
- P. Saikia, A. T. Miah and P. P. Das, Highly efficient catalytic reductive degradation of various organic dyes by Au/CeO2–TiO2 nano-hybrid, J. Chem. Sci., 2017, 129, 81–93, DOI:10.1007/s12039-016-1203-0.
- C. Umamaheswari, A. Lakshmanan and N. S. Nagarajan, Green synthesis, characterization and catalytic degradation studies of gold nanoparticles against congo red and methyl orange, J. Photochem. Photobiol., B, 2018, 178, 33–39, DOI:10.1016/j.jphotobiol.2017.10.017.
- Q. Zhang, A. Qileng, J. Li, Y. Cao, W. Liu and Y. Liu, Grafting a Porous Metal–Organic Framework [NH2-MIL-101(Fe)] with AgCl Nanoparticles for the Efficient Removal of Congo Red, ACS Omega, 2023, 8(5), 4639–4648, DOI:10.1021/acsomega.2c06300.
- K. Liu, Y. Yang, F. Sun, Y. Liu, M. Tang and J. Chen, Rapid degradation of Congo red wastewater by Rhodopseudomonas palustris intimately coupled carbon nanotube – Silver modified titanium dioxide photocatalytic composite with sodium alginate, Chemosphere, 2022, 299, 134417, DOI:10.1016/j.chemosphere.2022.134417.
- S. Sharma, B. Shree, Aditika, A. Sharma, M. Irfan and P. Kumar, Nanoparticle-based toxicity in perishable vegetable crops: Molecular insights, impact on human health and mitigation strategies for sustainable cultivation, Environ Res., 2022, 212(Pt A), 113168, DOI:10.1016/j.envres.2022.113168.
- R. Szőllősi, Á. Molnár, S. Kondak and Z. Kolbert, Dual Effect of Nanomaterials on Germination and Seedling Growth: Stimulation vs. Phytotoxicity, Plants, 2020, 9(12), 1745, DOI:10.3390/plants9121745.
- G. J. Miller, A. M. G. Cunningham, Y. Iwase, N. L. Lautensack and W. M. Sattley, A Laboratory Activity Demonstrating the Antibacterial Effects of Extracts from Two Plant Species, Moringa oleifera and Allium sativum (Garlic), J. Microbiol. Biol. Educ., 2017, 18(3), 18.3.56, DOI:10.1128/jmbe.v18i3.1306.
Footnotes |
† Electronic supplementary information (ESI) available: NMR, REMA plates, zone of inhibition, and bacterial colony count plates. See DOI: https://doi.org/10.1039/d3nj04494g |
‡ AP and SM contributed equally. |
|
This journal is © The Royal Society of Chemistry and the Centre National de la Recherche Scientifique 2024 |