DOI:
10.1039/D3NJ04345B
(Paper)
New J. Chem., 2024,
48, 384-393
Conductive single-walled carbon nanotubes synthesized using a Fe–Mo/MgO catalyst for LiNi0.5Co0.2Mn0.3O2 lithium-ion batteries
Received
15th September 2023
, Accepted 23rd November 2023
First published on 24th November 2023
Abstract
Herein, single-walled carbon nanotubes (SWNTs) with few defects and high aspect ratios were fabricated using a MgO-loaded Fe–Mo alloy as a catalyst for catalytic cracking of methane at 1000 °C. The metal impurities in SWNTs were removed via acid washing, and a SWNT (slurry) conductive agent was prepared by adding a dispersant (polyvinylpyrrolidone) to SWNTs and subjected to ultrasonic dispersion. Additionally, the addition of 2 wt% SWNTs (slurry) to the LiNi0.5Co0.2Mn0.3O2 cathode was sufficient to form an electronically conductive network, which improved the electronic conductivity of the cathode and optimized the battery performance at high rate currents. The results show that batteries fabricated using SWNTs (slurry) as a conductive additive in the LiNi0.5Co0.2Mn0.3O2 cathode exhibit excellent conductivity, with a specific capacity of 174.05 mA h g−1 at a first discharge at 0.1 C and a high specific discharge capacity of 112.5 mA h g−1 at 5 C. Additionally, the capacity retention of 100 cycles at 0.5 C was 98.75%, and their charge transfer impedance was minimized.
1. Introduction
Since the discovery of carbon nanotubes (CNTs) by Iijima in 1991,1 they have been one of the most actively studied materials, especially as semiconductor materials, in many fields2–5 because of their excellent electrical conductivity. CNTs and other carbon nanostructures like graphene and carbon dots have been used in other areas.6–11 CNTs are categorized into multiwalled CNTs (MWNTs), few-walled CNTs, double-walled CNTs (DWNTs), and single-walled CNTs (SWNTs).12 Currently, research on CNTs is focused on fabricating CNTs with fewer defects and higher aspect ratios. In addition, carbon nanotubes can be used in optics, electronics, magnetism, and pharmacy, and a growing number of potential applications for carbon nanotubes have been developed.13 Among them, carbon nanotubes are also widely used in electrodes.14–17
The rapid development of power, lithium-ion battery (LIB), and automobile industries is hindered by some problems such as the low energy density of LIBs, high internal resistance of LIBs, high internal consumption of LIBs, and so forth.18–22 One of the most effective solutions to these problems is to use an electrically conductive additive while fabricating batteries.23 CNTs are one of the ideal carbon-based conductive additives for LIB materials, compared to traditional conductive agents such as conductive carbon black (SuperP) and Ketjen black. This is because only a small amount of CNTs needs to be added to form a three-dimensional conductive network in the electrode with a low percolation threshold, ensuring the energy density of the battery while improving its electrochemical performance.24 SWNTs, as an advanced version of MWNTs, have a lower number of tube walls, a higher aspect ratio, and fewer defects than MWNTs. The performance of LIBs considerably improves when the cathode forms a thin composite layer of a homogeneously dispersed nanostructured active material.25,26 When SWNTs are used as conductive agents in cathodes, their high aspect ratios allow them to form a continuous conductive network, connecting isolated cathode particles and reducing the internal consumption of the cathode. SWNTs can be entangled on the surface of cathode particles; hence, they are not easily broken after multiple charging and discharging cycles, prolonging the cycle life of batteries. CNTs with fewer defects are favorable for electron transport and higher electrical conductivity.27
SWNTs can be fabricated via three methods: electric arc, laser evaporation, and chemical vapor deposition (CVD).28 Among these fabrication methods, the CVD method has the advantages of high efficiency, high controllability and low cost, so it has been widely used in carbon nanotube preparation. The CVD method can be used to catalyze the decomposition of hydrocarbons on SiO2, MgO, or Al2O329,30–loaded Fe, Co, and Mo31,32 metal nanoparticles. Recently, the synthesis of SWNTs by CVD has been reported in the literature, with carbon sources including carbon monoxide (CO),33–35 benzene (C6H6),36 ethylene (C2H4),37 methane (CH4),38,39 and so on. SWNTs can be purified and dispersed via acid washing,40 sonication, and dispersant addition.41
Herein, SWNTs with few defects and high aspect ratios were prepared using a MgO-loaded Fe–Mo bimetal alloy as a catalyst and simultaneously passing methane and hydrogen at 1000 °C. The simultaneous flow of hydrogen and methane reduced elemental Fe to Fe particles and promoted the bonding of elemental C from the catalytic cracking of methane. In contrast, if hydrogen is first introduced, it completely reduced elemental Fe, thereby forming large aggregates of metal particles. The addition of elemental Mo inhibited the agglomeration of elemental Fe, which helped in controlling the growth of CNTs. Additionally, metal impurities in SWNTs were removed via acid washing. SWNTs were ultrasonically dispersed by adding a dispersant (polyvinylpyrrolidone (PVP)) for preparing SWNTs (slurry), which was used as a conductive agent in the cathode. The LiNi0.5Co0.2Mn0.3O2 cathode with 2 wt% SWNTs (slurry) exhibited a specific first discharge capacity of 168.52 mA h g−1 at 0.1 C, a discharge retention rate of 99.15% for 5 charge–discharge cycles at 5 C, and a specific discharge capacity of 112.5 mA h g−1. Finally, a capacity retention of 98.75% after 100 charge–discharge cycles at 0.5 C was obtained. Electrochemical impedance spectroscopy (EIS) showed that the charge transfer impedance of the LiNi0.5Co0.2Mn0.3O2 cathode with 2 wt% SWNTs (slurry) was lower than those with MWNTs and SuperP (SP).
2. Experimental section
2.1 Preparation of Fe–Mo/MgO catalysts
Magnesium oxide (MgO), iron nitrate nonahydrate (Fe(NO3)3·9H2O), and ammonium molybdate tetrahydrate ((NH4)6Mo7O24·4H2O) were weighed in a mass ratio of 10
:
1.5
:
1. 4 mL of deionized water was added to Fe(NO3)3·9H2O and (NH4)6Mo7O24·4H2O and stirred until dissolved. Afterward, the mixed solution was added to the MgO powder drop-by-drop with stirring until an orange-yellow Fe–Mo/MgO slurry was obtained. The Fe–Mo/MgO mixed slurry was frozen at −20 °C for 12 h and then freeze-dried at 5 MPa for 48 h. After drying, the sample was milled and sintered in a tube furnace at 800 °C for 2 h in air. Finally, the Fe–Mo/MgO catalyst was obtained.
2.2 Preparation of SWNTs
0.2 g of the Fe–Mo/MgO catalyst was added in a tube furnace, followed by the passage of Ar at a flow rate of 500 sccm. The tube furnace was heated to 1000 °C at a heating rate of 5 °C min−1 while 400 sccm hydrogen flow and 70 sccm methane flow were passed for 30 min. Finally, the tube furnace was cooled to room temperature in Ar to obtain SWNTs.
2.3 Preparation of MWNTs
To compare with SWNTs, the same catalyst was added in a tube furnace to prepare MWNTs. First, 500 sccm argon flow was passed in the tube furnace. Then, the tube furnace was heated to 680 °C at a heating rate of 5 °C min−1. 500 sccm hydrogen flow was first passed in the tube furnace for 20 min. Then, the hydrogen flow was reduced to 50 sccm, and 300 sccm propane flow was passed in the tube furnace for 120 min. Finally, MWNTs were obtained after cooling down.
2.4 Preparation of SWNT conductive pastes
Concentrated nitric (30–36%) and hydrochloric (60–65%) acids were used to configure aqua regia in a volume ratio of 1
:
3. Aqua regia was poured onto the SWNTs, which were placed in a water bath and heated up to 80 °C with stirring for 8 h. The SWNTs were filtered using a sand-core funnel until a neutral pH was obtained. Then, they were dried in an oven at 120 °C for 4 h. A SWNT conductive paste was synthesized by mixing N-methyl pyrrolidone (NMP), SWNTs, and PVP in a 94
:
5
:
1 ratio with stirring and ultrasonication for 12 h.
2.5 LIB preparation
The LiNi0.5Co0.2Mn0.3O2 cathode material, binder (polyvinylidene fluoride (PVDF)), and NMP were mixed at a 92
:
6
:
2 mass ratio to obtain a solid content of 50% mixture, which was placed in a homogenizer and continuously stirred for 30 s at 800 rpm. Then, the speed of the homogenizer was automatically increased to 2000 rpm for 20 min to obtain a uniformly dispersed electrode slurry. The slurry was evenly coated onto an aluminum collector using a coating machine. Then, the obtained electrode sheet was dried at 120 °C for 24 h. The CR2032 button cells were assembled using Li metal as the anode in a glove box filled with Ar gas with moisture and oxygen contents <0.01 ppm. Additionally, the cells in which the SWNT slurry was used as the conductive agent in the LiNi0.5Co0.2Mn0.3O2 cathode were labeled NCM-SWNTs, and the cells prepared by substituting MWNTs and SP with SWNTs (slurry) as the conductive agent, while ensuring that all other conditions remained unchanged, were named NCM-MWNTs and NCM-SP, respectively.
2.6 Characterization
The morphology of the CNTs was characterized via scanning electron microscopy (SEM; EVO/MA10, ZEISS, Germany). Defects in SWNTs were characterized via Raman spectroscopy (Horiba HR800, Kyoto, Japan) with a laser emission wavelength of 514 nm. The distribution of SWNTs in the active material was studied via SEM. The first charge–discharge, multiplier, and cycling performance of the batteries were studied using the NEWARE battery test apparatus in a voltage range of 2.7–4.2 V. AC impedance (EIS) was measured using an electrochemical workstation (PGSTAT101, Metrohm Autolab) in the frequency range from 105 to 0.1 Hz and an amplitude of 5 mV. All characterization studies were performed at room temperature.
3. Results and discussion
3.1 Preliminary testing and analysis of materials
We tested the Fe–Mo/MgO catalyst and different conductors by energy dispersive spectroscopy, surface pore structure and X-ray diffraction analysis, respectively. Fig. 1(a) shows the SEM image of the Fe–Mo/MgO catalyst, and Fig. 1(b)–(f) show the energy-dispersive X-ray spectroscopy (EDS) images of the Fe–Mo/MgO catalyst. The distribution of Mg from MgO in the Fe–Mo/MgO catalyst in Fig. 1(d) is consistent with that in Fig. 1(a). As shown in Fig. 1(c) and (f), Fe and Mo are uniformly distributed on the surface of MgO. Notably, Fe does not exhibit a more drastic agglomeration phenomenon on the Fe–Mo/MgO catalyst, which shows that adding a small amount of elemental Mo in the catalyst helps to disperse the reactive metal and facilitates the control over the growth process of CNTs.
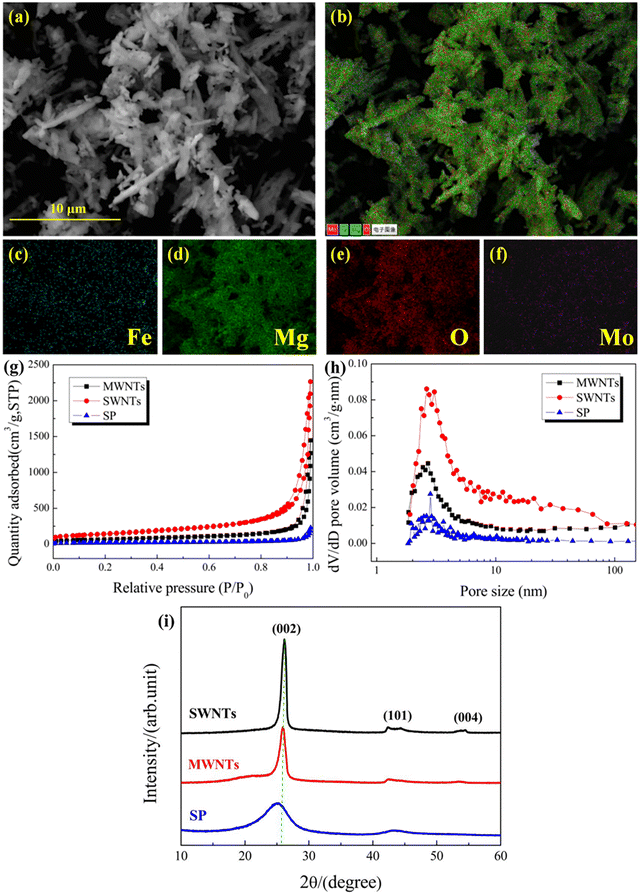 |
| Fig. 1 (a) SEM image of the Fe–Mo/MgO catalyst. (b)–(f) EDS images of the Fe–Mo/MgO catalyst. (g) N2 adsorption–desorption isotherms and (h) pore size distributions of MWNTs and SWNTs. (i) XRD patterns of SWNTs, MWNTs and SP. | |
Fig. 1(g) and (h) show the N2 adsorption–desorption isotherms and pore size distributions of SWNTs and MWNTs. As shown in Fig. 1(g), all curves are consistent, that is, a flat increase at relative pressures of 0.1–0.8 and a sharp increase at relative pressures of 0.8–1.0. All samples show type II N2 adsorption–desorption isotherms, according to the IUPAC classification. As shown in Fig. 1(h), the pore size distributions of SWNTs and MWNTs show two strong peaks at 2.7 and 3.1 nm, respectively. To understand, the information from Fig. 1(g) is statistically compared with that in Table 1. Notably, the specific surface area of SWNTs is 519.55 m2 g−1, much higher than those of MWNTs (243.35 m2 g−1) and SP (62.75 m2 g−1). And, the microporous volume of SWNTs was 0.0402 cm3 g−1, much higher than those of MWNTs (0.0327 cm3 g−1) and SP (0.0083 cm3 g−1). The higher the specific surface area of the carbon-based materials, the more contact area it provides for the active component in the electrode, increasing the utilization of cathode particles.
Table 1 Surface characterization of SWNTs, MWNTs and SP
Sample |
S
BET (m2 g−1) |
V
total (cm3 g−1) |
V
micro (cm3 g−1) |
Dp (nm) |
SWNTs |
519.55 |
3.3587 |
0.0402 |
25.86 |
MWNTs |
243.35 |
1.993 |
0.0327 |
32.75 |
SP |
62.75 |
0.2946 |
0.0083 |
17.63 |
Fig. 1(i) shows the XRD patterns of SWNTs, MWNTs, and SP. The XRD patterns of SWNTs and MWNTs show the diffraction peaks of the (002) carbon crystal plane at 2θ = 26.3°, but the intensity of the diffraction peaks in the XRD pattern of SWNTs is much higher than that in the XRD patterns of MWNTs and SP. And, SWNTs with sharp and high peaks are observed. This shows that the degree of graphitization of SWNTs is higher than those of MWNTs and SP and the carbon atoms in SWNTs have a more orderly arrangement than those in MWNTs and SP. Additionally, while the peak at 2θ = 24.8° in the XRD pattern of SP becomes broader, its intensity decreases, and it is shifted to the left compared with the XRD patterns of SWNTs and MWNTs. This shows that the carbon atom disorder in SP increases, and SP gradually exhibits amorphous features. Notably, the XRD pattern of SWNTs shows the peaks of (101) and (004) planes at 2θ = 44.5° and 54.5°, respectively. Conversely, compared with the XRD pattern of SWNTs, the intensity of the (101) and (004) peaks is lower in the XRD pattern of MWNTs, and the (101) and (004) peaks are almost absent in the XRD pattern of SP. The intensity of the (101) peak is related to the crystal orientation and that of the (004) peak is related to the lamellar structure of the crystals, indicating that the crystal orientation and lamellar structure of SWNTs are more intact than those of MWNTs and SP.
3.2 Scanning electron microscopy analysis
Fig. 2(a) and (b) show the SEM images of SWNTs and MWNTs, respectively. Fig. 2(c) and (d) show that SWNTs have a higher aspect ratio than MWNTs, and SWNTs have a lower tube diameter than MWNTs. Fig. 2(e) shows the SEM image of the cathode pole piece when SP is used as a conductive agent, and the arrows in the figure point to the distribution of some SP in the cathode particles. Fig. 2(e) shows that the SP and cathode particles have a “point-to-point” contact; the contact area is small and agglomeration is obvious. Therefore, SP cannot sufficiently form a continuous conductive network in the cathode. Additionally, some cathode particles are still isolated in the cathode. By contrast, SWNTs and cathode particles form a continuous conductive network, as shown in Fig. 2(f), where the particles of the cathode are connected to SWNTs, and SWNTs are entangled on the surface of the particles. This decreases the probability of the cathode breaking under high-rate and long-cycle charging and discharging cycles, improves the conductivity of the cathode, and protects cathode particles at the same time.
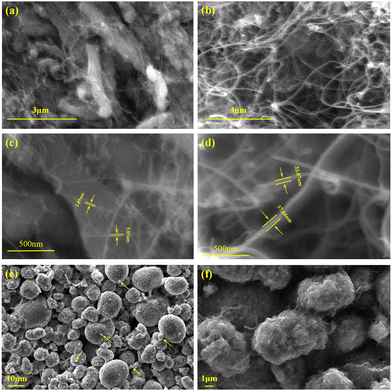 |
| Fig. 2 (a and c) SEM image of SWNTs. (b and d) SEM image of MWNTs. (e) SEM image of the SP cathode pole piece. (f) SEM image of the SWNT slurry cathode pole piece. | |
3.3 Raman spectroscopy analysis
To further study the structure and properties of SWNTs and MWNTs, Raman spectroscopy was performed, as shown in Fig. 3(a). The ring-breathing vibrational modes (RBMs) can be observed at 100–400 cm−1 in the Raman spectrum of SWNTs. The RBM corresponds to the collective movement of carbon atoms in SWNTs in the direction around the CNTs. The appearance of the RBM peak in the Raman spectrum indicates the presence of SWNTs in a sample because the RBM peak does not appear in the Raman spectrum when the number of walls in the CNTs is >3.42 For CNTs with large diameters, the intensity of the RBM peak is weak and harder to observe.43 Additionally, the SWNT diameter is 0.92–1.55 nm, as determined using the formula
.44 By contrast, the Raman spectrum of MWNTs exhibits no RBM peaks. The strong peak near 1580 cm−1 in the Raman spectrum of SWNTs (Fig. 4(a)) corresponds to the G peak, which indicates the degree of graphitization of CNTs. The strong peak near 1350 cm−1 in the Raman spectrum of SWNTs corresponds to the D peak, which indicates the disorder in the fine graphite structure, that is, the degree of disarray in the CNT structure.45Fig. 3(a) shows that the intensity of the D peak is lower than that of the G peak in the Raman spectrum of SWNTs, while the opposite is true in the Raman spectrum of MWNTs. This shows that SWNTs are more ordered, have a less disorganized graphite structure, and are more graphitized than MWNTs. As shown in Fig. 3(b), the ratios of the D peak intensity to the G peak intensity (ID/IG ratios) for SWNTs and MWNTs are 0.365 and 1.884, respectively. The ID/IG ratio of SWNTs is lower than that of MWNTs, which indicates that SWNTs have fewer defects and a higher degree of crystallization than MWNTs.46 Additionally, as shown in Fig. 3(a), the presence of the G* peaks at 2500–2750 cm−1 in the Raman spectrum of SWNTs indicates the presence of a high degree of graphitization in SWNTs. By contrast, the G* peak is almost absent in the Raman spectrum of MWNTs. In brief, the presence of RBM peaks in the Raman curves of SWNTs identifies them as SWNTs, and their ID/IG values reflect a high degree of graphitization and the presence of fewer defects than MWNTs.
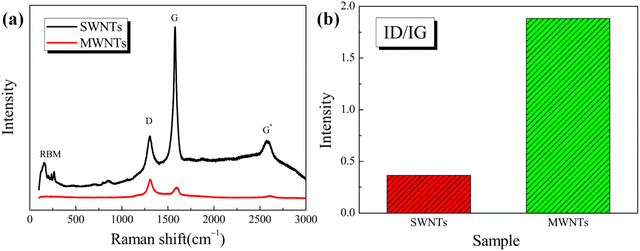 |
| Fig. 3 (a) Raman spectra of SWNTs and MWNTs. (b) Ratio curves of D and G peaks in the Raman spectra. | |
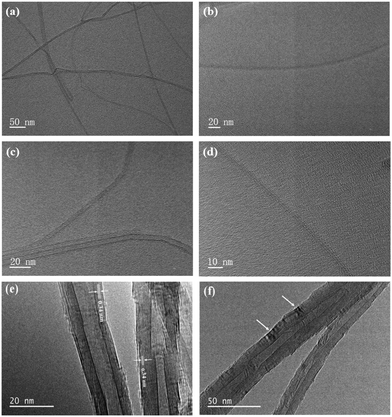 |
| Fig. 4 (a)–(d) TEM images of SWNTs. (e)–(f) TEM images of MWNTs. | |
3.4 Transmission electron microscopy analysis
To further prove that CNTs synthesized using the Fe–Mo/MgO catalyst and a simultaneous methane (carbon source) and hydrogen (reducing gas) flow at a certain temperature were SWNTs, transmission electron microscopy (TEM) was performed, as shown in Fig. 4. As shown in Fig. 4(a), CNTs have only one wall; hence, they are SWNTs and have a high aspect ratio. The high-resolution TEM images of SWNTs in Fig. 4(b)–(d) show that there are no obvious defects and fold streaks in the wall of the SWNTs, and the diameter of the SWNT is very fine. This is consistent with the Raman spectra in Fig. 4. Similarly, the Fe–Mo/MgO catalyst was used to synthesize MWNTs; the only difference is that the carbon source was changed to propane. The synthesized CNTs are confirmed to be MWNTs, as shown in Fig. 4(e) and (f). Fig. 4(e) shows that the CNTs have a higher number of walls, which is the characteristic of MWNTs. There are obvious folds and bends in the walls of the MWNTs, indicating the presence of more defects and a disordered arrangement of the carbon atoms, as shown in Fig. 4(f) (indicated by arrowheads), consistent with the Raman spectra in Fig. 3. Notably, the planar spacing of graphene layers in MWNTs is 0.34 nm.47 Additionally, the cracking temperature of methane is >600 °C. While it can be cracked at lower temperatures, the reaction rate is slow, which is not conducive to controlling the synthesis of CNTs. The cracking temperature of propane is >450 °C, and products such as amorphous carbon, tars, and so forth are formed. Therefore, when propane is used to synthesize MWNTs, more defects are observed in the synthesized MWNTs.48 In brief, high-quality, low-defect SWNTs were synthesized by changing the carbon source using the synthesis method with the same catalyst as a prerequisite.
3.5 Electrochemical analysis
Fig. 5(a) shows the first charge–discharge curves of the three samples, namely, NCM-SWNTs, NCM-MWNTs, and NCM-SP, at 0.1 C (1 C = 155 mA h g−1) and in the voltage range of 2.75–4.2 V. NCM-SWNTs, NCM-MWNTs, and NCM-SP exhibit first discharge capacities of 174.05, 159.61, and 152.26 mA h g−1, respectively. NCM-SWNTs exhibit the highest first specific discharge capacity, probably because SWNTs have fewer defects and an ordered arrangement of carbon atoms, resulting in higher electrical conductivity than MWNTs and SP. Notably, Hyuntae et al. noted that SWNTs embodied better electrochemical performance when NCM811 was used as the cathode, again superior to SP.49Fig. 5(b) shows the discharge capacity of the battery at different current rates (0.2 C, 0.5 C, 1 C, 2 C, 3 C, 4 C, 5 C, and 0.2 C). The cell capacities of NCM-SWNTs, NCM-MWNTs, and NCM-SP dramatically decrease with an increase in the current rate, and the specific discharge capacities of NCM-MWNTs and NCM-SP are 80.78 and 23.18 mA h g−1 at 5 C, respectively. By contrast, NCM-SWNTs still exhibit a specific discharge capacity of 112.50 mA h g−1 at 5 C, which is 31.72 and 89.32 mA h g−1 higher than those of NCM-MWNTs and NCM-SP, respectively. Additionally, NCM-SWNTs still exhibit a high and stable capacity after five cycles at a high current rate (5 C), followed by cycling at a low current rate (0.2 C). Notably, the discharge capacity of NCM-SWNTs exhibits a uniform stepwise decrease with an increase in the current rate unlike NCM-SP, which shows a cliff-like decrease from 2 to 5 C. This indicates that the electrical conductivity of SWNTs is superior to that of other samples, probably because SWNTs have a larger contact area than SP. This larger contact area improves the electron transport channel and accelerates the electron transport rate in NCM-SWNTs. Additionally, the cathode particles are prone to fragmentation under high rate currents. However, because SWNTs are entangled on the surface of the cathode particles, they protect them while transporting electrons, consistent with that shown in Fig. 5(d). By contrast, SP does not have this capability; hence, some particles in NCM-SP break under high rate currents, leading to an extremely rapid decrease in capacity.
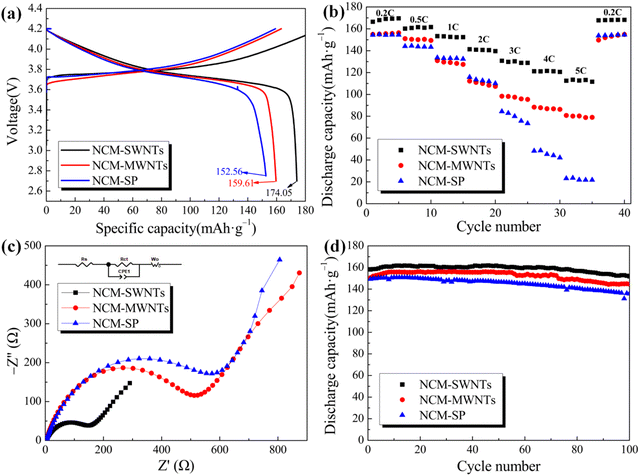 |
| Fig. 5 Electrochemical performance of the LiNi0.5Co0.2Mn0.3O2 cathode batteries prepared using SWNTs (slurry), MWNTs (slurry), and SP as conductive agents. (a) Initial galvanostatic charge–discharge profiles at 0.1 C, (b) rate performance, (c) EIS data, and (d) cycling performance at 0.5 C of NCM-SWNTs, NCM-MWNTs, and NCM-SP. | |
To further understand and compare the effect of SWNTs, MWNTs, and SP as conductive agents on the electrical conductivity of the LiNi0.5Co0.2Mn0.3O2 cathode, EIS analysis was performed on cells fabricated using NCM-SWNTs, NCM-MWNTs, and NCM-SP. The inset of Fig. 5(c) shows the model equivalent circuit used for EIS. As shown in Fig. 5(c), the Nyquist curves for all samples comprise a semicircle in the high-frequency or mid-frequency region and a straight line in the low-frequency region. The intercept of the curve with the horizontal axis (Z′) represents the ohmic resistance (R1), which mainly represents the electrolyte, diaphragm, and active substance of the battery. The arc in the mid-frequency region corresponds to the charge transfer impedance (R2) on the surface of the active substance. The diagonal line in the low-frequency region corresponds to the Warburg impedance, which is caused by the diffusion of Li ions in the active substance.50 Because the three sample cells use the same cathode electrolyte, they mainly differ in their solid–electrolyte interphase (SEI) membrane impedance, charge transfer impedance, and Li ion diffusion coefficient. As shown in Fig. 5(c), the sum of the SEI membrane impedance and charge transfer impedance of the NCM-SWNT-based sample cell is the lowest, indicating that the Li ion migration rate is the highest at this time, and the cell has low internal consumption and good conductivity. Additionally, the sum of the SEI membrane impedance and charge transfer impedance of the NCM-MWNT-based cell is higher than that of the NCM-SP-based cell, probably because of the unique structural properties of CNTs. Compared with the point-to-point contact between SP and cathode particles, the line-to-point contact of CNTs greatly improves the contact area with the cathode and increases the electron transport channel, thus enhancing the conductivity of the cathode. Notably, SWNTs, in addition to the basic properties of CNTs as a new type of conductive agent, exhibit a higher degree of graphitization, a higher length-to-diameter ratio, and fewer defects than MWNTs. Therefore, SWNTs, when used as a conductive agent in the LiNi0.5Co0.2Mn0.3O2 cathode, exhibit lower internal resistance and battery internal consumption than MWNTs.
A comparison of the cycling performance of the NCM-SWNTs, NCM-MWNTs, and NCM-SP samples for 100 charge–discharge cycles at 0.5 C is shown in Fig. 5(d). The sample of NCM-SWNTs (black curve in Fig. 5(d)) exhibits the most prominent, with an initial discharge capacity of 158.04 mA h g−1 at 0.5 C and a capacity retention of 96.02% after 100 charge–discharge cycles. Additionally, NCM-MWNTs and NCM-SP exhibit capacity retentions of 95.96% and 90.9% after 100 charge–discharge cycles, respectively. The capacity of the NCM-SWNT-based battery hardly changes after 100 cycles. By contrast, the initial capacities of NCM-MWNTs and NCM-SP-based batteries are 150.56 and 149.21 mA h g−1 at 0.5 C, respectively, lower than that of NCM-SWNTs. SWNTs are entangled on the surface of the cathode particles because they have higher aspect ratios, thus preventing the cathode particle fragmentation after multiple charging and discharging cycles while connecting the isolated cathode particles. This reduces the internal consumption of the battery and improves its energy density, consistent with the SEM and TEM results. Therefore, the excellent electrochemical performance of NCM-SWNTs can be attributed to their unique structure, higher degree of graphitization, and fewer defects, which facilitates electron transport and diffusion, improves cathode particle durability, and enhances the conductivity of the cathode. Similarly, Wen et al. suggested that when using conventional conductive agents (Super-P and KS-15) as well as graphene and carbon nanotubes as conductive agents for spherical LiFePO4, the battery with carbon nanotubes as conductive agents had the best overall performance (Table 2).50,51
Table 2 EIS parameters of NCM-SWNTs, NCM-MWNTs, and NCM-SP
Sample |
R
1 (mΩ) |
R
2 (mΩ) |
NCM-SWNTs |
2.292 |
132.1 |
NCM-MWNTs |
3.215 |
479.9 |
NCM-SP |
3.303 |
545.9 |
To investigate the effects of SWNTs (slurry), MWNTs (slurry), and SP as conductive agents on the electrical conductivity of the LiNi0.5Co0.2Mn0.3O2 cathode, the initial discharge curves of the different cells were analyzed at different current rates (0.2 C, 0.5 C, 1 C, 2 C, 3 C, 4 C, and 5 C), as shown in Fig. 6(a)–(c). The discharge voltage plateau and discharge capacity gradually decrease as the discharge rate increases as shown in Fig. 6(a)–(c). This is because the higher current rate polarizes the cell and prevents the migration of Li ions, thereby reducing the conductivity of the cathode. Comparing the changes in the discharge capacity of the three batteries, the NCM-SWNT-based battery exhibits the highest initial discharge capacity at all current rates, with a stable discharge plateau and no distortion in the discharge curve. By contrast, the NCM-SP-based battery not only exhibits the lowest capacity at all current rates but also exhibits a distorted curve at high current rates and a severe tilting of the charge–discharge platform. Additionally, NCM-MWNTs exhibit a lower capacity than NCM-SWNTs even though their discharge curves and plateaus are stable, suggesting the presence of higher polarization than NCM-SWNTs, which hinders electron transport.
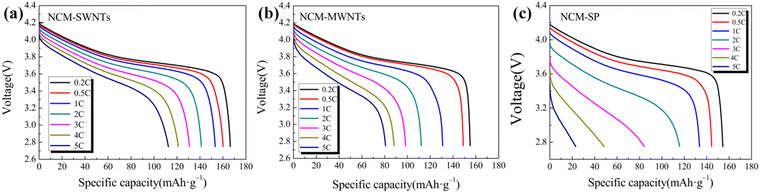 |
| Fig. 6 Initial discharge curves of different sample cells at different rates. | |
To further analyze the effects of SWNTs (slurry), MWNTs (slurry), and SP as conductive agents on the cycling performance of the LiNi0.5Co0.2Mn0.3O2 cathode, the charging and discharging curves of the three samples after the 1st, 50th, and 100th cycles were measured, as shown in Fig. 7(a)–(c). The cyclic voltammetric test curves of different cells at a scan rate of 0.1 mV s−1 are shown in Fig. 7(d)–(f), respectively. The discharge capacity decreases as the number of cycles increases, as shown in Fig. 7(a)–(c) and Table 3. Among the three samples, the difference in discharge capacity between the NCM-SP at the 1st and 100th times is large, indicating more rapid capacity decay. By contrast, the three curves of NCM-SWNTs and NCM-MWNTs are more concentrated and have less capacity decay. As shown in Fig. 7(d)–(f), the oxidation–reduction peaks of the three samples have been labeled in the graphs, corresponding to the redox reactions of Ni2+/Ni4+. And the oxidation–reduction peak of NCM-SWNTs is located at 3.826 V/3.675 V (ΔV = 0.151 V), the oxidation–reduction peak of NCM-MWNTs is located at 3.972/3.576 V (ΔV = 0.396 V), and the oxidation–reduction peak of NCM-SP is located at 3.958 V/3.572 V (ΔV = 0.467 V). The comparison yields the smallest potential difference (ΔV) for NCM-SWNTs. In contrast, the peak shifts of the CV curves of NCM-SP are larger, its potential difference is ΔV = 0.467 V, and the shift of the oxidation peak position indicates that the plateau potential changes, resistance of Li ions to embedding and detachment increases, polarization impedance increases, and the peak position of the reduction peak is considerably attenuated compared with that of the other two samples. It is worth noting that the one-cycle CV curves (black curves) deviate from the two-cycle curves (red curves) for all three sets of samples, due to the failure to fully activate the active materials in the first test. In addition, it can be seen that the two-turn CV curves of NCM-SWNTs have a smaller difference from those of NCM-MWNTs and NCM-SP, indicating that SWNTs are able to better activate the active materials and enhance the first-time efficiency of the cell, which is related to its high aspect ratio and specific surface area.52
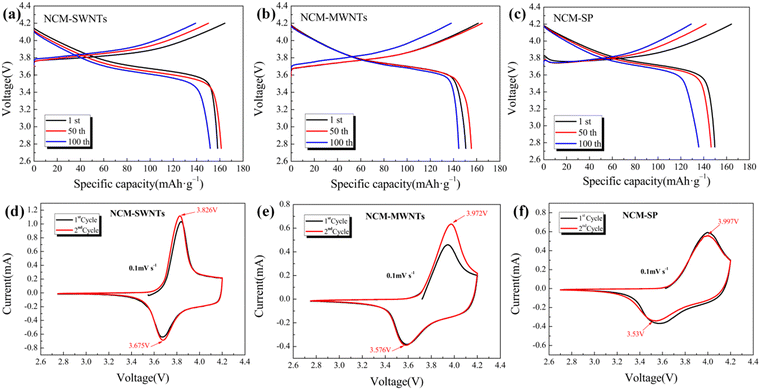 |
| Fig. 7 (a–c) Charge–discharge curves of three samples after different numbers of cycles at 0.5 C. (d–f) Cyclic voltammetric test curves of cells at a sweep rate of 0.1 mV s−1. | |
Table 3 Specific discharge capacity of the three samples at 0.5 C after 1, 50, and 100 charge–discharge cycles. Redox peak potential difference in the second loop of the cycle
Sample/discharge specific capacity |
Lap 1 (mA h g−1) |
Lap 50 (mA h g−1) |
Lap 100 (mA h g−1) |
ΔV (V) |
NCM-SWNTs |
158.04 |
161.19 |
151.75 |
0.151 |
NCM-MWNTs |
150.50 |
155.43 |
144.49 |
0.396 |
NCM-SP |
149.71 |
146.49 |
135.64 |
0.467 |
4. Conclusions
MgO-loaded Fe–Mo bimetallic catalysts were prepared via an impregnation method, in which Mo plays a dispersing role and can effectively inhibit the agglomeration of Fe. This allows the active metal to be uniformly distributed on the surface of the carrier (MgO). SWNTs (SWNTs) with few defects and high aspect ratios were prepared by simultaneously passing hydrogen and methane over the catalyst at 1000 °C. Additionally, SWNTs (slurry) prepared via a simple acid-washing purification and ultrasonic dispersion method were used in LiNi0.5Co0.2Mn0.3O2 cathodes to construct a continuous and stable conductive network. SWNTs increase the contact area with the cathode, protect and connect isolated cathode particles, and reduce the internal resistance of the battery. Notably, a simple, efficient, and environmentally friendly method for the preparation of an SWNT (slurry) conductive agent was reported, and the effects of SWNTs (slurry), MWNTs (slurry), and SP as conductive agents on the conductivity of the LiNi0.5Co0.2Mn0.3O2 cathode were examined and compared, which is of some reference value. This work provides new ideas for developing new high-performance SWNT-based conductive agents for LIBs.
Conflicts of interest
There are no conflicts to declare.
Acknowledgements
This work was supported by the National Natural Science Foundation of China (51874151), the Scientific Research Foundation for Universities from the Education Bureau of Jiangxi Province (GJJ170510), the Natural Science Foundation of Jiangxi Province (20151BBE50106) and the Jiangxi University of Science and Technology (NSFJ2014-G13 and Jxxjbs12005).
References
- S. Lijima, Helical microtubles of graphitic carbon, Nature, 1999, 37(11), 1873–1874 Search PubMed.
- K. Chen, W. Gao and S. Emaminejad,
et al., Printed carbon nanotube electronics and sensor systems, Adv. Mater., 2016, 28(22), 4397–4414 CrossRef CAS.
- A. E. Islam, J. A. Rogers and M. A. Alam., Recent Progress in Obtaining Semiconducting Single-Walled Carbon Nanotubes for Transitor Applic ations, Adv. Mater., 2015, 27(48), 7908–7937 CrossRef CAS.
- S. Nanot, E. H. Haroz and J. H. Kim,
et al., Optoelectronic properties of single-wall carbon nanotubes, Adv. Mater., 2021, 24(36), 4977–4994 CrossRef.
- L. Wen, F. Li and H. M. Cheng, Carbon nanotubes and graphene for flexible electrochemical energy storage: from materials to devices, Adv. Mater., 2016, 28(22), 4306–4337 CrossRef CAS.
- T. Tian, Y. Cheng and Z. Sun,
et al., Carbon nanotubes supported oxygen reduction reaction catalysts: role of inner tubes, Adv. Compos. Hybrid Mater., 2023, 6, 7 CrossRef CAS.
- G. Yuan, T. Wan and A. BaQais,
et al., Boron and fluorine Co-Doped laser-induced graphene towards high-performance micro-supercapacitors, Carbon, 2023, 8, 118101 CrossRef.
- J. Ruan, Z. Chang and H. Rong,
et al., High-conductivity nickel shells encapsulated wood-deriverd porous carbon for improved electromagnetic interference shielding, Carbon, 2023, 9, 118208 CrossRef.
- Y. Zhao, F. Liu and Z. Zhao,
et al., Direct ink printing reduced graphene oxide/KCu7S4 electrodes for high-performance supercapacitors. Advanced Composites and Hybrid, Materials, 2022, 6, 1516–1526 Search PubMed.
- M. Kumari, G. R. Chaudhary and S. Chaudhary,
et al., Rapid Analysis of Trace Sulphite Ion Using Fluorescent Carbon Dots Produced from Single Use Plastic Cups, Eng. Sci., 2022, 17, 101–112 CAS.
- A. Vijeata, G. R. Chaudhary and A. Umar,
et al., Distinctive Solvatochromic Response of Fluorescent Carbon Dots Derived from Different Components of Aegle Marmelos Plant, Eng. Sci., 2021, 15, 197–209 Search PubMed.
- B. J. Landi, M. J. Ganter and C. D. Cress,
et al., Carbon nanotubes for lithium ion batteries, Energy Environ. Sci., 2009, 2(6), 638–654 RSC.
- M. Kociak, A. Y. Kasumov and S. Guéron,
et al., Superconductivity in Ropes of Single-Walled Carbon Nanotubes, Phys. Rev. Lett., 2001, 86(11), 2416–2419 CrossRef CAS.
- L. Meng, C. Hou and J. Hou,
et al., Preparation and Performance of In Situ Carbon-Coated Silicon Monoxide@C@carbon Microspheres Composite Anode Material for Lithium-Ion Batteries, Eng. Sci., 2022, 20, 134–143 CAS.
- C. Hou, B. Wang and V. Murugadoss,
et al., Recent Advances in Co3O4 as Anode Materials for High-Performance Lithium-Ion Batteries, Eng. Sci., 2020, 11, 19–30 CAS.
- X. Jiang, Y. Chen and X. Meng,
et al., The impact of electrode with carbon materials on safety performance of lithium-ion batteries: A review, Carbon, 2022, 5, 448–470 CrossRef.
- Z. Yan, J. Li and Q. Chen,
et al., Synthesis of CoSe2/Mxene composites using as high-performance anode materials for lithium-ion batteries, Adv. Compos. Hybrid Mater., 2022, 8, 2977–2987 CrossRef.
- W. Qiu, Q. Hao and S. H. K. Annamareddy,
et al., Electric Vehicle Revolution and Implication: Ion Battery and Energy, Eng. Sci., 2022, 20, 100–109 CAS.
- A. Karthik, D. S. Chiniwar and M. Das,
et al., Electric Propulsion for Fixed Wing Aircrafts-A Review on Classifications, Designs, and Challenges, Eng. Sci., 2021, 16, 129–145 CAS.
- M. Dennis, Bushnell. Prospective Futures of Civilian Air Transportation, Eng. Sci., 2021, 16, 5–8 Search PubMed.
- V. Naik, M. Kumar and V. Kaup, A Review on Natural Fiber Composite Material in Automotive Applications, Eng. Sci., 2022, 18, 1–10 Search PubMed.
- J. Min, J. Hu and C. Sun,
et al., Fabrication processes of metal-fiber reinforced polymer hybrid components: a review, Adv. Compos. Hybrid Mater., 2022, 5, 651–678 CrossRef CAS.
- B. Nykvist and M. Nilsson, Rapidly falling costs of battery packs for electric vehicles, Nat. Clim. Change, 2015, 5(4), 329–332 CrossRef.
- P. Sehrawat, C. Julien and S. S. Islam, Carbon nanotubes in Li-ion batteries: A review, Mater. Sci. Eng., C, 2016, 213, 12–40 CrossRef CAS.
- A. Yamada, S. C. Chung and K. Hinokuma, Optimized LiFePO4 for lithium battery cathodes, J. Electrochem. Soc., 2001, 148(3), A224–A229 CrossRef CAS.
- A. Yamada, M. Hosoya and S.-C. Chung,
et al., Olivine-type cathodes: Achievements and problems, J. Power Sources, 2003, 119–121, 232–238 CrossRef CAS.
- J. Yoshida, S. Roth and N. Sato,
et al., Effect of single-walled carbon nanotubes as conuctive additives on the performance of LiCoO2-based electrodes, J. Electrochem. Soc., 2011, 158(2), A174–A179 CrossRef.
- H. J. Dai, Nanotube growth and charaterization, Top. Appl. Phys., 2001, 80, 29–53 CrossRef CAS.
- J.-F. Colomer, C. Stephan and S. Lefrant,
et al., Large-scale synthesis of single-wall carbon nanotubes by catalytic chemical vapor deposition (CCVD) method, Chem. Phys. Lett., 2000, 317(1–2), 83–89 CrossRef CAS.
- J. Kong, A. M. Cassell and H. Dai., Chemical vapor deposition of methane for single-walled carbon nanotubes, Chem. Phys. Lett., 1998, 292(4–6), 567–574 CrossRef CAS.
- A. A. Almarasy, T. Hayasaki and Y. Abiko,
et al., Characterization of single-wall carbon nanotubes produced by CCVD method, Chem. Phys. Lett., 2001, 345(1–2), 11–17 Search PubMed.
- H. Ago, S. Ohshima and K. Uchida,
et al., Carbon nanotube synthesis using colloidal solution of metal nanoparticles, Phys. B, 2002, 323(1–4), 306–307 CrossRef CAS.
- H. Yan, Q. Li and J. Zhang,
et al., Possible tactics to improve the growth of single-walled carbon nanotubes by chemical vapor deposition, Carbon, 2022, 40, 2693–2698 CrossRef.
- H. Dai, A. G. Rinzler and P. Nikolaev,
et al., Single-wall nanotubes poduced by metal-catalyzed disproprotionation of carbon monoxide, Chem. Phys. Lett., 1996, 260(3–4), 471–475 CrossRef CAS.
- X. Zhao, Q. Liu and Z. Zhang,
et al., Growth of single-walled carbon nanotubes on substrates using carbon monoxide as carbon source, Chem. Res. Chin. Univ., 2021, 37(5), 1125–1129 CrossRef CAS.
- M. He, A. I. Chernov and P. V. Fedotov,
et al., Predominant(6,5)single-walled carbon nanotube growth on a copper-promoted iron catalyst, J. Am. Chem. Soc., 2010, 132(40), 13994–13996 CrossRef CAS.
- Y. Yuan, H. Enis Karahan and C. Yıldırım,
et al., “Smart poisoning” of Co/SiO2 catalysts by sulfidation for chirality-selective synthesis of (9,8) single-walled carbon nanotubes, Nanoscale, 2016, 8(40), 17705–17713 RSC.
- L. Che-Jen, K. Kang and H. Swager,
et al., Pentiptycene Polymer/Single-Walled Carbon Nanotube Complexes: Applications in Benzene, Toluene, and O-Xylene Detection, ACS Nano, 2020, 14(6), 7297–7307 CrossRef.
- E. Flahaut, A. Govindaraj and A. Peigney, Synthesis of single-walled carbon nanotubes using binary (Fe, Co, Ni) alloy nanoparticles prepared in situ by the reduction of oxide solid solutions, Chem. Phys. Lett., 1999, 300(1–2), 236–242 CrossRef CAS.
- L. Xuanke, B. Rik and R. Brian,
et al., The synthesis of single-walled carbon nanotubes over an Al2O3Fe2O3 binary aerogel catalyst, J. Phys.: Conf. Ser., 2006, 26(1), 308 Search PubMed.
- Y. Li, Z. Han and W. Zhou,
et al., Direct growth of carbon nanotube junctions by a two-step chemical vapor deposition, Chem. Phys. Lett., 2006, 432(1–3), 177–183 Search PubMed.
- J. Li and Y. Zhang., A simple purification for single-walled carbon nanotubes, Phys. E, 2005, 28(3), 309–312 CrossRef CAS.
- H. Ago, S. Ohshima and K. Uchida,
et al., Carbon nanotube synthesis using colloidal soluting of metal nanoparticles, Phys. B, 2002, 323(1–4), 306–307 CrossRef CAS.
- M. S. Dresselhaus, G. Dresselhaus and A. Jorio, Raman spectroscopy of carbon nanotubes in 1997 and 2007, J. Phys. Chem. C, 2007, 111, 17887–17893 CrossRef CAS.
- P. T. Araujo, S. K. Doorn and S. Kilina,
et al., Third and fourth optical transitions in semiconducting carbon nanotubes, Phys. Rev. Lett., 2007, 98, 067401 CrossRef.
- J. M. Holden, P. Zhou and X. X. Bi,
et al., Raman scattering from nanoscale carbons generated in a colbalt-catalyzed carbon plasma, Chem. Phys. Lett., 1994, 220, 186–191 CrossRef CAS.
- M. S. Dresslhaus, G. Dresselhaus and R. Saito,
et al., Raman spectroscopy of carbon nanotubes, Phys. Rep., 2005, 409, 47–99 CrossRef.
- V. V. Boloyov, E. V. Knyazev and P. M. Korusenko,
et al., Functionalization of individual multi-wall carbon nanotubes during irradiation and annealing, J. Phys. C: Solid State Phys., 2020, 62(11), 2173–2183 Search PubMed.
- H. Kim, J. H. Lim and T. Lee,
et al., Ozone-Treated Carbon Nanotube as a Conductive Agent for Dry-Processed Lithium-Ion Battery Cathode, ACS Energy Lett., 2023, 8, 3460–3466 CrossRef CAS.
- L. Wen, L. Wang, G. Zhiwei and X. Liu,
et al., Effect of composite conductive agent on internal resistance and performance of lithium iron phosphate batteries, Ionics, 2022, 28, 3145–3153 CrossRef CAS.
- L. Wen, J. Sun and L. An,
et al., Effect of Conductive Material Morphology on Spherical Lithium Iron Phosphate, Nanomaterials, 2018, 8(11), 904 CrossRef.
- J. Zhu, M. S. D. Darma and M. Knapp,
et al., Investigation of lithium-ion battery degradation mechanisms by combining differential voltage analysis and alternationg current impedance, J. Power Sources, 2020, 227575 CrossRef CAS.
|
This journal is © The Royal Society of Chemistry and the Centre National de la Recherche Scientifique 2024 |