DOI:
10.1039/D3NJ03668E
(Paper)
New J. Chem., 2024,
48, 394-405
Nonlinear optical-active ferrocene conjugated Y-shaped imidazole donor–π–acceptor [(D–π)2–IM–π–A] compounds for dye-sensitized solar cells using non-corrosive copper complexes as a redox mediator†
Received
5th August 2023
, Accepted 20th November 2023
First published on 20th November 2023
Abstract
Two new bis-ferrocene (Fc) conjugated Y-shaped imidazole (IM) based donor–π–acceptor [(D–π)2–IM–π–A] type “push–pull” compounds [(Fc–CH
CH)2–IM–C8H17–C6H4–R] {R = COOC2H5 (1); COOH (2)} have been synthesized for second-order nonlinear optics (1, ester) and dye-sensitized solar cell (2, acid) applications. The redox potentials of the compounds were examined using cyclic voltammetry, which shows one-electron charge transfer from the ferrocene to ferrocenium ion (Fe2+ ⇌ Fe3+), and these potentials were employed to calculate the related energy gap. The second-order nonlinear optical (NLO) properties of the ester compound 1 were explored using the Electric-Field-Induced Second Harmonic generation (EFISH) technique in CHCl3 solution with an incident wavelength of 1907 nm, and it shows an interesting μβEFISH value of −780 × 10−48 esu. Besides, the carboxylic acid compound 2, adequately functionalized for anchoring to TiO2, was tested as a photosensitizer in dye-sensitized solar cells (DSSCs) with non-corrosive Cu(I)/Cu(II) complexes as the redox mediator. Although the photovoltaic performance of 2 attached to TiO2 in a DSSC device is rather limited (JSC = 1.51 mA cm−2, VOC = 404 mV, FF = 42.7, η = 0.26%), it confirms the potential of ferrocenyl conjugated D–π–A systems as photosensitizers. In addition, frontier molecular orbital levels, ground and excited state dipole moments (μg and μe), and the origin of electronic absorption spectra were studied by using density functional theory (DFT) with the B3LYP method using 6-31+G** as the basis set.
1 Introduction
Energy demand is a worldwide problem. In order to develop an alternative source for non-renewable energy, dye-sensitized solar cells (DSSCs) were first reported by O'Regan and Grätzel using ruthenium(II) (polypyridyl) complexes as photosensitizers; they now reach photon-to-current conversion efficiencies of up to 14% with nanocrystalline TiO2.1 Sensitizers are essential as DSSC components because they control light harvesting and charge separation abilities, which are necessary for better efficiencies.2,3 Remarkably, by changing the chromophore and anchoring groups of sensitizers, it is possible to increase their molar extinction coefficient and solar light harvesting capacity, thus improving the photocurrent and efficiency of DSSCs.4,5 A number of metal-based sensitizers with notable power conversion efficiencies (PCE) have been reported, such as Ru-based dyes,6–9 Zn porphyrins,10 and perovskites.11 However, these devices have some drawbacks, particularly the Ru-based dyes, due to their high costs and the low abundance of metals and their toxicity in nature.12 Therefore, it is necessary to explore alternative, low cost, eco-friendly new dyes. Hence, the design of photosensitizers in DSSCs is even today a challenging task.
Thus, in the last two decades, researchers have been interested in the use of ferrocene-based complexes for DSSC applications, due to the versatile oxidation states (Fe2+ ⇌ Fe3+) of iron in the ferrocene moiety; in fact, ferrocene is responsible for the reversible one-electron redox reaction and can act as a low-cost, non-toxic donor in DSSCs. The redox potential of the dyes can be tuned by varying the substitution on cyclopentadienyl rings.13 Thanks to these aspects, various ferrocenyl derivatives have been studied in DSSCs as possible photosensitizers. Thus, ferrocenyl bearing Ni(II) and Cu(II) dithiocarbamate complexes (3.87 and 2.80%),14 ferrocenyl substituted triphenylamine based donor–acceptor dyes (3.65%),15 ferrocene appended porphyrin derivatives (0.0081%),16 ferrocene diketopyrrolopyrrole based acceptors (6.44%),17 ferrocenyl benzimidazoles (5.81%),18 Y-shaped biferrocenyl quinoxaline (0.81%),19 ferrocene-based cyanovinyl compounds (0.18 and 0.10%),20,21 ferrocene appended azine spacers (4.04%)22 and π-extended ferrocenyl dyes (0.68%)23 with different anchoring groups have been investigated using I−/I3− as a redox mediator and their efficiencies were compared with the standard N719 dye under similar experimental conditions, showing the potential of ferrocene derivatives for DSSCs. However, to date, no ferrocene-based Y-shaped imidazole compound has been studied as a sensitizer.
Nevertheless, heterocyclic scaffolds have been widely used in donor–acceptor (D–A) systems because they provide high chemical and thermal robustness.24 Among them, imidazole moieties act as a good auxiliary electron donor or acceptor because of their high electron deficiency originating from two asymmetric nitrogen atoms that lower the π* level of the conjugated system.25 Thus, imidazole-based organic dyes are used as molecular sensitizers in DSSCs.26 Ferrocenyl-based imidazole molecules appear to be of great interest in this field, because the conjugated framework offers improvement in the charge-transfer transition from the auxiliary electron donor to first electron donor.18 The Y-shaped imidazole (IM) molecules [(D–π)2–IM–π–A], with the two donors at the peripheral C4/C5 positions of the imidazole and the acceptor at the C2 position, show high charge transferability due to the presence of electron deficient nitrogen in the imidazole unit. Compared with linear molecules the Y-shaped molecules have better intramolecular charge transfer (ICT) behaviours due to the presence of more electron donor or acceptor units.25,27 Literature reports are available on organic Y-shaped imidazole compounds for DSSCs,28 light-emitting diodes (OLEDs),29 nonlinear optics (NLO),26 and two-photon absorbers.30 We have reported some ferrocene conjugated Y-shaped imidazole compounds for their second-order NLO properties,31 but their photovoltaic applications as dyes in DSSCs have never been investigated. To develop new imidazole-based organometallic dyes for DSSCs, herein we report a new Y-shaped ferrocene conjugated imidazole dye (D2–π–A) as a sensitizer with electron-deficient nitrogen units as an additional electron acceptor (2) and a parental complex (1) with interesting second-order nonlinear optical (NLO) properties (Chart 1).
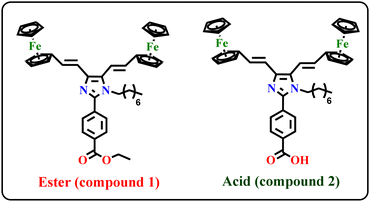 |
| Chart 1 The molecular structure of the Y-shaped ferrocene conjugated imidazole compounds 1 and 2. | |
Generally, the I−/I3− redox couple is used as a redox mediator in DSSCs,32 but this couple has some drawbacks, for example I2 is volatile and its long-term cell sealing is quite complicated. The I3− reduction at the counter electrode is obtained by the DSSC cathode only by the platinum coating, but it is unstable, and it faces photovoltage loss due to the mismatching with the dye redox potential. In addition, I−/I3− is a highly corrosive material and it will corrode most metals and the light harvesting efficiency of the dye is limited due to the dark colour of I3−.33 These are the serious disadvantages in using metal grid collectors for upgrading DSSCs to large areas. To overcome these problems, some of us introduced the Cu(II)/Cu(II) redox couple for DSSC applications.34 The mechanism of a Cu redox couple is similar to that of “blue copper proteins” due to a distorted tetragonal geometry, which is an intermediate geometry between Cu(I) and Cu(II) and therefore leads to fast electron exchange.35,36 In this respect, we have explored heteroleptic Cu(I)/Cu(II) complexes as redox mediators in DSSCs and the efficiencies start from less than 0.1% to reach a maximum of 3.0%.37,38 In the literature, reports are available with ferrocene conjugated donor–π–acceptors as sensitizers using I−/I3− and cobalt(II/III) electrolyte as redox mediators,14,19 but non-corrosive Cu(I)/Cu(II) redox couples have not been reported yet. So, to the best of our knowledge, there is no report on ferrocene-based sensitizers in dye-sensitized solar cells using copper complexes as redox mediators.
Besides, it is worth pointing out that a ferrocene complex was the first reported NLO-active organometallic species.39 It was shown that the ferrocene moiety plays an important role as an electron-donor in charge transfer processes, when it is attached to an acceptor moiety in push–pull NLO molecules.39,40 Clearly organometallic compounds are an attractive and growing class of second-order NLO-phores, because they provide several advantages over the inorganic and organic molecules, owing to the presence of NLO active electronic charge-transfer transitions of the metal to ligands at relatively low energy and of high intensity, tunable by virtue of the nature, oxidation state, and coordination sphere of the metal center.40 Several investigations have been carried out with ferrocene-based molecules in the field of NLO with different acceptor groups such as metal carbonyls, porphyrinoids and heteroarenes. An increase in the strength of the electron-withdrawing moiety and in the length of the π-bridge between the donor and the acceptor groups leads to an increase in the β values.41 Recently, we have investigated Y-shaped biferrocene conjugated quinoxaline and imidazole compounds, demonstrating their strong second-order NLO response by using the Electric Field Induced Second Harmonic (EFISH) technique in solution. These complexes found application as polymethylmethacrylate composite films with a good second harmonic generation (SHG) response.31
Considering the aforementioned information, we have synthesized two new ferrocene conjugated Y-shaped heterocyclic complexes (Chart 1), starting from the same scaffold: ester (1) and acid (2); they both present an alkyl chain, in order to prevent aggregation of the molecules and charge recombination which would decrease the photovoltaic properties. The linear optical properties have been investigated using UV-visible spectroscopic techniques and the nonlinear optical behavior of compound 1 was studied using the EFISH technique in solution. The DSSC properties of compound 2, with an appropriate anchoring group, was investigated with different electrolytes (solutions containing I−/I3− or homoleptic copper(I/II) complexes bearing two 2-n-butyl-1,10-phenanthrolines) and compared with the standard N719 dye. In addition, the absorption band and the electron transition effect of the imidazole auxiliary acceptor were investigated theoretically by using density functional theory (DFT) and time-dependent DFT (TD-DFT) at the B3LYP/6-31+G** level. As previously reported, we confirm that it was not possible to measure, with the EFISH technique, the second-order NLO response of complexes bearing a –COOH moiety, such as complex 2 of the present paper, due to dissociation of –COOH.42
2 Experimental sections
2.1 Materials and methods
Chemicals were procured from TCI and Sigma Aldrich Chemical & Co. The commercially available solvents were used after purification using a distillation process. According to the reported procedure, (1E, 5E)-1,6-bisferrocenyl-hexa-1,5-diene-3,4-dione was synthesized.43 The product was purified using the column chromatographic method with silica gel (AVRA, 60–120 mesh), hexane and ethyl acetate as solvents. The Debus-Radziszewski reaction was followed to prepare the ferrocene conjugated Y-shaped imidazole ester compound 1.
2.2 General characterization
The NMR spectra were recorded on a BRUKER (400 MHz) spectrometer. Chemical shifts were reported in δ (ppm). The ESI-mass spectra were recorded on LC/MS, 6230B4 Time of Flight (TOF), Agilent technologies. The elemental analysis was carried out using CHNS Elemental Analyzer-PerkinElmer-2400 CHNS/O Series. FT-IR spectra were obtained using a SHIMADZU IR Affinity-1 instrument equipped with a high-sensitivity DLATGS detector; spectra were recorded as KBr discs. Electronic absorption spectra were recorded in dichloromethane, in a 1 cm2 quartz cuvette at room temperature, using a JASCO V-670 UV-Visible spectrophotometer. Electrochemical measurements were recorded using a CHI620E electrochemical analyser; they were performed in 5 × 10−3 M solution in dichloromethane with 0.1 M tetrabutylammonium perchlorate (TBAP, Aldrich) as the supporting electrolyte at a scan rate of 100 mV s−1. Platinum wire was used as the counter electrode, glassy carbon as the working electrode and an Ag/AgCl electrode as the reference electrode.
2.3 Synthesis of Y-shaped imidazole ester (compound 1)
The ferrocene conjugated Y-shaped imidazole ester 1 was synthesized by condensation between (1E,5E)-1,6-bisferrocenyl-hexa-1,5-diene-3,4-dione (1 mmol), ethyl 4-formylbenzoate (1 mmol) and n-octyl amine (1 mmol), in the presence of ammonium acetate and acetic acid, under reflux for 3 hours. After completion of the reaction, the solvent was evaporated to give a crude product, that was further purified by column chromatography using hexane and ethyl acetate (8
:
2). Yield: 411 mg, 55%. C44H48Fe2O2N2: calcd C, 70.60; H, 6.46; N, 3.74; found C, 70.62, H, 6.50, N, 4.04. ESI-MS: exact mass: 748.2415, found mass: 748.2425. 1H NMR (400 MHz, CDCl3) δ (ppm): 8.16 (d, J = 8 Hz, 2H, C6H4), 7.71 (d, J = 8 Hz, 2H, C6H4), 7.27 (d, J = 16 Hz, 1H, CH), 6.87 (d, J = 16 Hz, 1H, CH), 6.70 (d, J = 16 Hz, 1H, CH), 6.54 (d, J = 16 Hz, 1H, CH), 4.51 (s, 2H, Hβ C5H4), 4.47 (s, 2H, Hβ C5H4), 4.44 (q, J = 14.4Hz, 2H, –O–CH2), 4.36 (s, 2H, Hα C5H4), 4.25 (s, 2H, Hα C5H4), 4.23 (s, 5H, C5H5), 4.15 (s, 5H, C5H5), 3.96 (t, J = 7.6 Hz, 2H, N–CH2), 1.45 (t, J = 7 Hz, 3H, CH3), 1.17 (m, 12H, C6H12), 0.87 (t, J = 6.8 Hz, 3H, CH3). 13C NMR (100 MHz, CDCl3) δ (ppm): 166.2 (1C, C
O), 146.9 (1C, N–C), 137.9 (1C, p-C), 135.4 (1C p-C), 132.3 (–N–C), 130.5 (2C o-C), 129.8, 129.0, 128.9, 126.3 (2C m-C), 117.6, 112.2, 84.3 (1C, Cipso), 83.2 (1C, Cipso), 69.5 (2C, Cα C5H4), 69.4 (5C, C5H5), 69.3 (5C, C5H5), 68.7 (2C, Cα C5H4), 66.8 (2C, Cβ C5H4), 66.7 (2C, Cβ C5H4), 61.2 (1C, –O–CH2), 44.9 (1C, N–CH2), 31.7 (1C, CH2), 30.6 (1C, CH2), 29.0 (1C, CH2), 28.9 (1C, CH2), 26.5 (1C, CH2), 22.6 (1C, CH2), 14.3 (1C, CH3), 14.1(1C, CH3). FT-IR: 3084(w) ν(C–H arom), 2918(s) ν(C–H aliph), 2839(s) ν(C–H aliph), 1713(s) ν(C
O), 1641(m) ν(C
C), 1494(m), 1470(s) ν(C–H methyl), 1405(s), 1255(m) ν(C–O),1105(s) ν(C
C Fc), 1035(s), 1006(s), 924(s) ν(C–C Fc), 824(s) ν(CH arom), 695(s), 492(s) cm−1. UV-visible data λmax (CH2Cl2) = 245 (π–π*), 363 (n–π*) and 461 (d–d) nm, and molar extinction coefficient εmax = 15
791, 12
736 and 2305 M−1 cm−1.
2.4 Synthesis of Y-shaped imidazole acid (compound 2)
The ferrocene conjugated Y-shaped imidazole acid 2 was synthesized by hydrolysis of compound 1 in the presence of 1N NaOH solution in methanol under reflux for 3 hours. After completion of the reaction, the solvent was removed and 20 mL of water was added to this residue and acidified at pH 2 using HCl. The solid was filtered, washed with water and dried to obtain compound 2. Yield: 532 mg, 74%. C42H44Fe2O2N2: calcd C, 70.01; H, 6.16; N, 3.89; found C, 69.97; H, 6.01; N, 3.90. ESI-MS: exact mass: 720.2102, found mass: 720.2114. 1H NMR (400 MHz, CDCl3) δ (ppm): 8.18 (d, J = 7.6 Hz, 2H, C6H4), 7.71 (d, J = 7.6 Hz, 2H, C6H4), 7.37 (d, J = 16 Hz, 1H, CH), 6.81 (d, J = 15.6 Hz, 1H, CH), 6.70 (d, J = 15.6 Hz, 1H, CH), 6.52 (d, J = 15.6 Hz, 1H, CH), 4.51 (s, 2H, Hβ C5H4), 4.47 (s, 2H, Hβ C5H4), 4.36 (s, 2H, Hα C5H4), 4.23 (s, 2H, Hα C5H4), 4.22 (s, 5H, C5H5), 4.09 (s, 5H, C5H5) 3.96 (t, J = 7 Hz, 2H, N–CH2), 1.24 (m, 12H), 0.86 (t, J = 6.8 Hz, 3H, CH3). 13C NMR (100 MHz, CDCl3) δ (ppm): 169.1 (1C, C
O), 146.9 (1C N–C), 136.7 (1C p-C), 133.5 (1C p-C), 133.1, 132.3 (–N–C), 130.2 (2C o-C), 129.1, 128.6, 127.7 (2C m-C), 116.4, 111.7, 84.1 (1C, Cipso), 82.9 (1C, Cipso), 69.6 (2C, Cα C5H4), 69.5 (5C, C5H5), 69.3 (5C, C5H5), 68.9 (2C, Cα C5H4), 66.9 (2C, Cβ C5H4), 66.7 (2C, Cβ C5H4), 44.9 (1C, N–CH2), 31.7 (1C, CH2), 30.5 (1C, CH2), 29.0 (1C, CH2), 28.9 (1C, CH2), 26.5 (1C, CH2), 22.6 (1C, CH2), 14.1 (1C, CH3). FT-IR: 3431(b) ν(OH), 3084(w) ν(C–H arom), 2918(s) ν(C–H aliph), 2839(s) ν(C–H aliph), 1703(s) ν(C
O), 1641(m) ν(C
C), 1494(m), 1470(s) ν(C–H methyl), 1405(s), 1316(w) ν(C–H aliph), 1255(m) ν(C–O), 1105(s) ν(C
C Fc), 1035(s), 1006(s), 924(s) ν(C–C Fc), 824(s) ν(CH arom), 695(s), 492(s) cm−1. UV-visible data λmax (CH2Cl2) = 231 (π–π*), 371 (n–π*) and 453 (d–d) nm, and molar extinction coefficient εmax = 9193, 12
500 and 3194 M−1 cm−1.
2.5 Theoretical calculations
The electronic structures and the molecular properties of ferrocene conjugated Y-shaped imidazole compounds 1 and 2 were investigated using Density Functional Theory (DFT) for understanding the bonding patterns, electronic charge and molecular orbital energy distributions. The geometries of the synthesized compounds in the gas phase were optimized using Becke's three-parameter and the Lee–Yang–Parr functional at the B3LYP level.44 The B3LYP level of computational calculations were carried out using the 6-31+G** basis set to determine the global minimum energy structure of all the dyes and their molecular properties were calculated. The computation calculations were performed using the Gaussian 16 Revision C.01 program45 and the frontier molecular orbital density plots were visualized using the GaussView 6.1.1 program.46
2.6 EFISH measurements
The Y-shaped imidazole ester compound 1 was studied using the EFISH technique47 in CHCl3 solution at 10−3 M concentration, with a non-resonant incident wavelength of 1907 nm, obtained by Raman-shifting the fundamental 1064 nm wavelength produced by a Q-switched, mode-locked Nd3+:YAG laser manufactured by Atalaser. The apparatus used for EFISH measurements is a prototype made by SOPRA (France). The values of μβEFISH reported are the mean values of 16 measurements performed on the same sample. The sign of μβ is determined by comparison with the reference solvent (CHCl3).
2.7 Fabrication and evaluation of solar cells
TiO2 electrodes were prepared by spreading (doctor blading) a colloidal TiO2 paste (20 nm sized; “Dyesol” DSL 18NR-T) onto a conducting glass slide (FTO, Hartford glass company, TEC 8, with a thickness of 2.3 mm and a sheet resistance in the range 6–9 Ω cm−2) that had been cleaned with water and EtOH treated with a plasma cleaner at 100 W for 10 min, dipped in a freshly prepared aqueous TiCl4 solution (4.5 × 10−2 M), at 70 °C for 30 min, and finally washed with ethanol. After first drying at 125 °C for 15 min, a reflecting scattering layer containing >100 nm sized TiO2 (“Solaronix” Ti-Nanoxide R/SP) was bladed over the first TiO2 coat and sintered until 500 °C for 30 min. Then, the glass coated TiO2 was dipped again into a freshly prepared aqueous TiCl4 solution (4.5 × 10−2 M), at 70 °C for 30 min, then washed with ethanol, and heated once more at 500 °C for 15 min. At the end of these operations, the final thickness of the TiO2 electrode was in the range of 8–12 μm, as determined by SEM analysis. After the second sintering, the FTO glass coated TiO2 was cooled at about 80 °C and immediately dipped into a CH2Cl2 solution (1.5 × 10−4 M) of the selected dye at r.t. for 18 h. The dyed titania glasses were washed with EtOH and dried at r.t. under a N2 flux. Finally, the excess TiO2 was removed with a sharp Teflon penknife, and the exact active area of the dyed TiO2 was calculated by means of microphotography. A 50 μm thick Surlyn spacer (TPS 065093-50 from Dyesol) was used to seal the photoanode and a platinized FTO counter electrode. Then, the cell was filled up with the desired electrolyte solution (see the details reported in Table 3). The photovoltaic performance of the cells was measured with a solar simulator (Abet 2000) equipped with a 300 W xenon light source; the light intensity was adjusted with a standard calibrated Si solar cell (“VLSI Standard” SRC-1000-RTD-KG5). The current–voltage characteristics were acquired by applying an external voltage to the cell and measuring the generated photocurrent with a “Keithley 2602A” (3A DC, 10A Pulse) digital source meter. For a given complex and configuration, at least four different devices were made and characterized on different days; the difference between the average and the highest or lowest efficiency values was usually lower than 5%.
3 Results and discussion
3.1 Synthesis of compounds 1 and 2
The ferrocene conjugated Y-shaped imidazole ester compound 1 was obtained via the Debus-Radziszewski condensation reaction. (1E,5E)-1,6-bisferrocenyl-hexa-1,5-diene-3,4-dione reacted with ethyl 4-formylbenzoate and n-octyl amine in the presence of ammonium acetate and acetic acid under reflux conditions. The obtained ferrocene conjugated Y-shaped imidazole ester 1 was further hydrolyzed with 1N NaOH solution in methanol to give compound 2, as shown in Scheme 1.
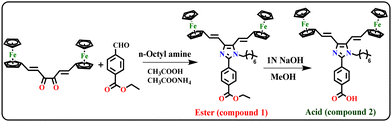 |
| Scheme 1 The synthesis of compounds 1 (ester) and 2 (acid). | |
3.2 Characterization of compounds 1 and 2
The purity of compounds 1 and 2 was examined using 1H and 13C NMR spectra, determined in CDCl3 at room temperature; the spectra are shown in Fig. S1–S4 (ESI†). In the 1H NMR spectra of compounds 1 and 2, the ferrocene protons of the two unsubstituted cyclopentadienyl rings (η5-C5H5) appear as a singlet in the region 4.1–4.2 ppm, whereas the substituted cyclopentadienyl ring (η4-C5H4) is at 4.3–4.5 ppm. The aliphatic CH protons are resonating at 0.8–1.9 ppm and the –N attached CH2 protons are at 3.9 ppm as a triplet. In the case of compound 1, the ester CH2 protons appear at 4.4 ppm as a quartet. The phenyl protons of compounds 1 and 2 appear as two doublets at 7.69–8.16 ppm. In the 13C NMR spectra of compounds 1 and 2, the ferrocene carbons resonate at 66.7–69.2 ppm and Cipso carbon of the substituted cyclopentadienyl ring are in the range of 82.9–84.3 ppm. The carbonyl carbon (–C
O) appears at 166.3 (1) and 169.1 (2) ppm and aliphatic carbons are resonating in the range at 14.0–44.8 ppm. In compound 1, the oxygen attached carbon appears at 61.2 ppm and all other phenyl carbons for compounds 1 and 2 appear in the range of 126–138 ppm. In the FT-IR spectra of compounds 1 and 2, the C
O stretching is around 1700 cm−1. The aromatic C–H stretching vibrations are observed at 3084 cm−1 and the aliphatic C–H stretching vibrations are in the range of 2840–2918 cm−1 for both compounds 1 and 2. The –OH band is at 3431 cm−1, which confirms the formation of acid in compound 2, and the spectra are shown in Fig. S5 (ESI†). The mass spectra of compounds 1 and 2 show a peak with the expected isotopic pattern at m/z = 748.2425 (for 1) and 720.2114 (for 2), respectively, and the obtained data coincide with the calculated data, as shown in Fig. S6 and S7 (ESI†).
3.3 Electrochemical properties
The redox behavior of compounds 1 and 2 was studied in dichloromethane solution with 0.1 M supporting electrolyte [N(C4H9-n)4]ClO4 (TBAP) at a scan rate of 100 mV s−1. Platinum wire was used as the counter electrode, glassy carbon as the working electrode and Ag/AgCl electrode acted as the reference electrode. The cyclic voltammograms of compounds 1 and 2 are shown in Fig. 1. The ratio of anodic to cathodic current is (ipa/ipc) close to unity and it is quasi-reversible for the electrochemical assessment. The oxidation potentials of compounds 1 and 2 are observed in the range of 0.66 to 0.73 V, which indicates the one-electron charge transfer from the ferrocene to ferrocenium ion (Fe2+ ⇌ Fe3+). The observed half-wave potential (E1/2 = 0.579 for 1 and 0.661 V for 2) and peak separation (ΔE = 0.159 for 1 and 0.145 V for 2) values of compounds 1 and 2 are different from the parent ferrocene (E1/2 = 0.44 V and ΔE = 0.71 V for FcH/FcH+ reversible system).48 In addition, compounds 1 and 2 exhibit higher halfwave potentials than the bisferrocenyl imidazole43 and Y-shaped imidazole aldehyde compounds reported in the literature,49 due to an increase of the electron withdrawing ability of the COOH group. The half-wave potential (E1/2) of compound 2 is higher and shifted towards a more positive potential than 1 due to the presence of the electron-withdrawing carboxylic acid group, which leads to a more effective charge transfer process 2.18 Besides, it is necessary to know the highest occupied molecular orbital (HOMO) and lowest unoccupied molecular orbital (LUMO) energy levels. These parameters are useful to understand the regeneration of dyes and the electron transfer process in DSSCs.50 The HOMO and LUMO energy level were derived by the formula EHOMO = −(Eox + 4.4) and ELUMO = Eopticalg + EHOMO,48 as shown in Table 1. The energy gaps of the compounds are 3.07 (1) and 2.89 (2) eV, which follows the same trend as that obtained with the theoretical (DFT) calculations.
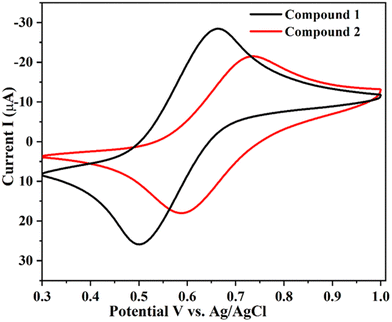 |
| Fig. 1 Cyclic voltammogram of 1 and 2 in the presence of 0.1 M TBAP supporting electrolyte at 0.1 mV s−1 in 10−3 M CH2Cl2 solution. | |
Table 1 Cyclic voltammetry data and experimental HOMO, LUMO and optical bandgaps
Compound |
E
pa
(mV) |
E
pc
(mV) |
i
pa/ipc |
E
1/2
(mV) |
ΔEa (mV) |
E
HOMO
(eV) |
E
LUMO
(eV) |
λ
onset
(nm) |
E
opticalg c (eV) |
Calculated as the HOMO and LUMO level obtained from cyclic voltammetry using EHOMO = −(Eox + 4.4). Eoptical onset values obtained from the oxidation peak in the cyclic voltammogram. ELUMO = Eopticalg + EHOMO.
Calculated as λonset values from the absorption spectra in CH2Cl2 solvent.
Calculated as the optical bandgap calculated from the absorption onset/edge using the equation (Eopticalg) = 1240/λonset.
|
1
|
659 |
500 |
1.3 |
579 |
159 |
−5.27 |
−2.23 |
403 |
3.07 |
2
|
734 |
589 |
1.2 |
661 |
145 |
−5.26 |
−2.37 |
429 |
2.89 |
3.4 Optical properties
The electronic absorption spectra of compounds 1 and 2 were obtained in dichloromethane (CH2Cl2) solution at 10−5 M (see Fig. 2) and different concentrations (see Fig. S8, ESI†). In these spectra, we observe three major transitions, within the range of 200–700 nm. The higher energy absorptions are 245 nm (ε = 15 791 M−1 cm−1) and 231 nm (ε = 9193 M−1 cm−1) for compounds 1 and 2, due to the π–π* transition and originate from a ligand-centered transition.43 The medium energy n–π* transition of compounds 1 and 2 at 363 (ε = 12
736 M−1 cm−1) and 371 nm (ε = 12
500 M−1 cm−1) respectively, can be described by metal-to-ligand charge transfer (MLCT) or ligand-to-metal charge transfer (LMCT) due to intramolecular charge transfer (ICT).51 The low-energy less intense electronic absorption band at 461 nm (ε = 2305 M−1 cm−1) for 1 and 453 nm (ε = 3194 M−1 cm−1) for 2 (d–d transition assigned to 1E1g ← 1A1g) is due to the degenerate transition of Fe(II) for metal–ligand charge transfer (dπ–π*).52 It should be pointed out that the introduction of a carboxylic acid group produces a noticeable lowering of the ε values of the absorption bands with respect to the corresponding ethyl ester. These spectra can be compared with those of the parent ferrocene unit: the wavelengths are red-shifted due to the presence of the extended π-conjugation of an imidazole moiety, which enables the improved charge transfer process of the compounds, leading to a bathochromic shift53 (see Fig. 2).
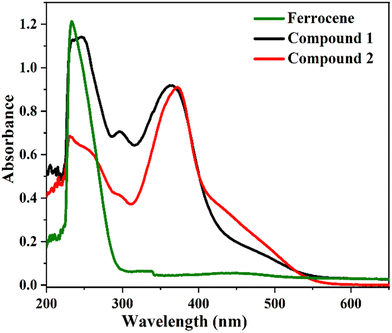 |
| Fig. 2 Absorption spectra of 1 and 2 in CH2Cl2 solution at (1 × 10−5 M). | |
3.5 Second-order nonlinear optical properties of compound 1
The second-order NLO properties of compound 1 were investigated using the Electric Field Induced Second Harmonic (EFISH) generation technique,47 working in CHCl3 at a 10−3 M concentration with a non-resonant incident wavelength of 1.907 μm, obtained by Raman-shifting under high hydrogen pressure by a Q-switched, mode-locked Nd3+:YAG laser. This method is a valuable alternative to Hyper-Rayleigh scattering (HRS), which suffers from the restriction of possible overestimation of the quadratic hyperpolarizability values due to multiphoton fluorescence. EFISH can offer direct information on the intrinsic molecular NLO properties through eqn (1) | γEFISH = (μβλ/5kT) + γ(−2ω, ω, ω, 0) | (1) |
where, μβλ/5kT is the dipolar orientational contribution of nonlinearity to the molecule, and γ(−2ω, ω, ω, 0), the third order polarizability at frequency ω of the incident light, is the purely electronic cubic contribution to γEFISH which can usually be ignored, when studying the second-order NLO properties of dipolar molecules. Compound 1 shows a good μβEFISH value of −780 × 10−48 esu. To estimate the quadratic hyperpolarizability (βEFISH) value of compound 1, it is necessary to know the dipole moment (μ). Here, we have calculated a theoretical dipole moment in the gas phase as well as in the solvent phase (CH2Cl2) with the B3LYP/6-31+G** level of theory. There is a slight difference in the gas and solvent phases, as reported in Table 2, indicating that there is no significant solute–solvent interactions. Compound 1 shows a negative value of μβEFISH, corresponding to a negative value of Δμeg (the difference between the excited and ground state dipole moments) upon excitation based on the two-level model.40d,42 A slightly higher negative value of μβEFISH (−1000 × 10−48 esu) was reported for a ferrocene conjugated Y-shaped imidazole compound, due to a higher ground state dipole moment (5.1 × 10−18 esu). Compound 1 shows a very large βEFISH value (−211 × 10−30 esu), which is slightly higher (1.2 times) than that previously reported for other ferrocene imidazole compounds.31b
Table 2 Photophysical and second order NLO properties of compounds 1 and 2
Compound |
λ
MEmax a [nm (eV)−1] εa (×105) [M−1 cm−1] |
λ
LEmax a [nm (eV−1)] εa (×105) [M−1 cm−1] |
HOMOb (eV) |
LUMOb (eV) |
λ
max
[nm (eV)] |
μ
gas/DCMb (×10−18 esu) |
μβ
EFISH
(×10−48 esu) |
β
EFISH
(x10−30 esu) |
Experimental data (ME = medium energy, LE = low energy).
Theoretical calculations using TD-DFT.
In anhydrous CHCl3, the estimated uncertainty in the EFISH measurement is 10%.
β
EFISH was calculated using the computed μDCM value.
|
1
|
363(3.41)/1.27 |
461(2.68)/0.23 |
−4.99 |
−1.90 |
372 (3.33) |
3.2/3.7 |
−780 |
−211 |
2
|
371(3.34)/1.25 |
453(2.73)/0.31 |
−4.98 |
−2.06 |
390 (3.17) |
3.6/4.8 |
— |
— |
3.6 DFT studies
Density functional theory (DFT) was used to analyze the molecular geometries and electronic properties of the ferrocene conjugated Y-shaped compounds 1 and 2. Unfortunately, the single crystal data of compounds 1 and 2 were not obtained due to the poor diffraction quality. The synthesized compounds were optimized in the gas phase and the geometrical parameters were determined using the DFT-B3LYP functional with 6-31+G** basis set. For transition metals, the B3LYP function is known to produce reasonably accurate geometries and the molecular geometries of compounds 1 and 2 are shown in Fig. S9 (ESI†). The optimized structure has accurate geometry in agreement with previously reported Y-shaped structures.31b,49
The highest occupied molecular orbital (HOMO), lowest unoccupied molecular orbital (LUMO) energies and absorption maxima (λmax), were calculated using the DFT-B3LYP functional with the 6-31+G** basis set, and the values are reported in Table 2. Based on the computed results, it is clear that the HOMO and LUMO orbital distribution was developed through the charge exchange from the bi-ferrocenyl donor and imidazole with the acceptor unit. In compounds 1 and 2, the HOMO is mainly localized on the ferrocene moiety and the π-spacer double bond along with a part of the imidazole unit and the calculated HOMO energy level for compounds 1 and 2 are −4.99 eV and −4.98 eV, respectively. The LUMO is localized in both the imidazole unit and the para-position of the aromatic ring in the presence of ester (1) and acid (2), as shown in Fig. 3. Here, we notice that the imidazole ring participates in the HOMO as well as in the LUMO levels and it contributes to the balanced charge transfer in compounds 1 and 2.
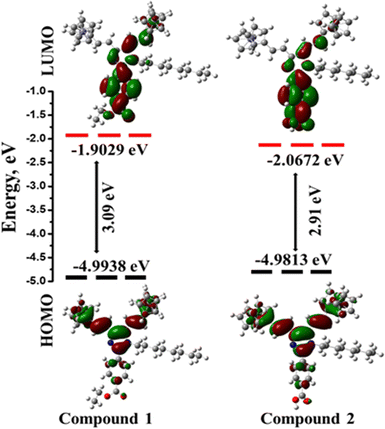 |
| Fig. 3 Schematic orbital energy levels of 1 and 2 at the B3LYP/6-31+G** level of theory in the gas phase. Energy gaps and orbital distribution of the HOMO and LUMO are also shown. | |
Furthermore, we have carried out Time-Dependent Density Functional Theory (TD−DFT) using the B3LYP function with the 6-31+G** basis set for compounds 1 and 2 to assign the definite transitions observed in the experiments (Table 2). The theoretically observed absorption spectra for compounds 1 and 2 are shown in Fig. S10 (ESI†): the lower-energy (LE) band is at 474 nm (1) and 483 nm (2) whereas the higher-energy (HE) band is at 408 nm (1) and 390 nm (2). The LE, with less intense electronic transition, is mainly described by HOMO → LUMO excitations (more than 73%). This excited state has large oscillator strengths, because it is dominated by a one-electron transition with a large transition dipole moment. The HE transitions, more intense, are described by HOMO → LUMO+1 (more than 36%) for compounds 1 and 2; these excitations can be assigned as n → π* due to the electronic transition within the molecules.49 The electronic structures, excited energies, oscillator strengths and orbital transitions of compounds 1 and 2 are listed in Tables S1 and S2 (ESI†).
3.7 Dye-sensitized solar cells with compound 2
Dye-sensitized solar cells were prepared using FTO glass coated TiO2 sensitized with bis-ferrocenyl Y-shaped imidazole compound 2 as the photoanode, platinized FTO as the counter electrode and an electrolyte solution containing I−/I3− or homoleptic copper(I/II) complexes bearing two 2-n-butyl-1,10-phenanthrolines (E1/E2, Fig. 4) as the redox couples, already reported and successfully employed by some of us.54
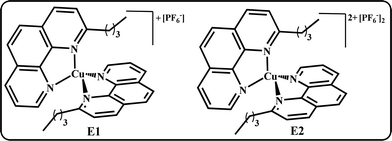 |
| Fig. 4 Chemical structures of the investigated copper-based redox mediator. | |
The newly synthesized bis-ferrocenyl Y-shaped imidazole compound 2 was employed as a dye in DSSCs. In fact, it has a COOH group that might provide good anchorage on the TiO2 surface. In dye-sensitized solar cells, the ability to generate electricity is determined by various components, such as TiO2, dyes, redox mediator and Pt surfaces. Among these, the redox mediator plays an important role in the power conversion efficiency of a DSSC as it involves regeneration of the dye molecules. From a general point of view we can say that the widely used I−/I3− redox mediator can cause some problems among which the most important are: the long-term stability of the devices, compromised by the corrosive power of the oxidant I2 towards the sealing material and the competition with the dye in the light harvesting, due to the absorption characteristics of I3−.32,55 To overcome these problems, some non-corrosive Cu(I)/Cu(II) redox couples have been proposed for DSSCs, leading to a fast electron exchange.35 The results of the investigated DSSCs are presented in Table 3 together with those obtained with the Ru(II) benchmark N719; in the same table, the details of the composition of the electrolyte solutions employed are also reported whereas the corresponding (J–V) curves are shown in Fig. 5. The best PCE obtained for compound 2 is 0.26%, reached with I−/I3− based electrolyte, while in the presence of the standard Cu(I)/Cu(II) electrolyte composition54 the PCE decreases up to 0.16% due mainly to a significant drop in JSC, from 1.51 to 0.95 mA cm−2 (see Table 3). We tried to limit this inconvenience by modifying the copper-based electrolyte composition. An increase of the LiTFSi concentration up to 0.2 M leads to an improvement in both JSC and PCE which reaches the values of 1.18 mA cm−2 and 0.19%, respectively, but which remain far from the values obtained with the I−/I3− based electrolyte. The values of JSC obtained with compound 2 do not exceed 1.51 mA cm−2 and we can explain this result considering the very low absorption in the visible region (Fig. 2) and the electron transfer between the acceptor and ferrocene units, as confirmed by the completely quenched fluorescence, which leads low photon conversion efficiency.56,57 Finally, the low values of PCE, obtained with compound 2, compared to those obtained, in this study with the bench mark N719, have also been caused by a low VOC: around 0.4 V, regardless of the electrolyte used, mainly attributable to the electrochemical characteristic of 2, as well as from FF values not higher than 43% probably due to bad interfaces inside the devices. The lower value of VOC for compound 2 is due to the higher level of ground state oxidation potential (0.73 V), which enhances the electron back transfer from the conduction band to the oxidized dye.15
Table 3 Main photovoltaic parametersa of DSSCs based on compound 2
Entry |
Dye |
Solvent |
Electrolyte |
J
SC (mA cm−2) |
V
OC (V) |
FF (%) |
η (%) |
Values measured in the absence of any mask.
0.65 M N-methyl-N-butylimidazolium iodide, 0.04 M iodine, 0.025 M LiI, 0.28 M t-BuPy in a 15/85 (v/v) mixture of valeronitrile/acetonitrile.
0.17 M Cu(I), 0.017 M Cu(II), 0.1 M LiTFSI in CH3CN.
0.17 M Cu(I), 0.017 M Cu(II), and 0.2 M LiTFSI in CH3CN.
0.6 M N-methyl-N-butylimidazolium iodide, 0.03 M iodine, 0.1 M guanidinium thiocyanate, and 0.5 M t-BuPy in a 15/85 (v/v) mixture of valeronitrile/acetonitrile.
|
1 |
2 |
CH2Cl2 |
I−/I3−b |
1.51 |
0.40 |
42.7 |
0.26 |
2 |
2 |
CH2Cl2 |
Cu(I)/Cu(II)c |
0.95 |
0.40 |
40.6 |
0.16 |
3 |
2 |
CH2Cl2 |
Cu(I)/Cu(II)d |
1.18 |
0.41 |
39.1 |
0.19 |
|
N719 |
EtOH |
I−/I3−e |
19.1 |
0.70 |
65.5 |
8.77 |
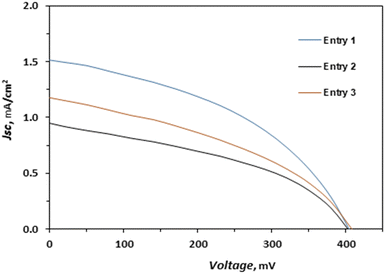 |
| Fig. 5
J–V characteristics of DSSCs in the presence of 2 as the dye and various electrolytes (see Table 3). | |
The HOMO levels of compound 2 is found to be −5.26 V vs. Ag/Ag+ in dichloromethane, which is higher than the redox mediators [−4.4 V (I−/I3−) and −4.9 (E1 and E2)] vs. Ag/Ag+ in CH2Cl2).54a This implies that the oxidized dyes produced from respective electron injection into the CB of TiO2 will favourably accept electrons from redox mediators. The schematic energy level diagram of TiO2 and dye with the redox mediators are presented in Fig. S11 (ESI†), based on the absorption spectrum and electrochemical data, which indicates that compound 2 has appropriate electronic energy levels to be deployed as promising sensitizers in DSSCs. In addition, the energy gap between compound 2 and the Cu-based redox mediator is 0.3 eV, which is sufficient for the dye regeneration process.17,58
Table S3 (ESI†) summarizes the DSSC performances of previously reported ferrocene based sensitizers, which indicate that the device performance of the sensitizers reported in the presented investigation is comparable with that of the previously reported derivatives. Significantly, compound 2 shows much higher photoconversion efficiencies than those previously reported for a ferrocene conjugated multi-donor system in the presence of a I−/I3− redox mediator (η = 0.015%),56 probably due to the presence of two ferrocene units. Moreover, the power conversion efficiencies are higher than those obtained with a ferrocene-based porphyrin derivative tested in the presence of a cobalt(II)/cobalt(III) electrolyte system (η = 0.008%),16 a ferrocene-modified zinc phthalocyanine (η = 0.003%),57 ferrocenyl-chalcones (η = 0.211%),59 ferrocenyl dyes with different alkyne units as π-spacers (η = 0.115%),20 a bifunctional ferrocene-based cyanovinyl (η = 0.10%),21 and ferrocene methoxy phenyl conjugated multi donor systems (η = 0.015%),56 all of them examined using a iodide/triiodide (I−/I3−) electrolyte system.
4 Conclusion
In this work, we have synthesized new biferrocenyl conjugated imidazole compounds with two different terminal functional groups: ester (compound 1) and anchoring carboxylic acid (compound 2). These compounds were characterized with the aid of different spectroscopic and analytical techniques. The second-order nonlinear optical (NLO) properties of compound 1 were determined using the EFISH technique. The related βEFISH value is −211 × 10−30 esu, slightly higher than that of previously reported ferrocenyl imidazole compounds. The photovoltaic performances of compound 2 with a carboxylic anchoring group was carried out in DSSCs using both a conventional iodide/triiodide (I−/I3−) electrolyte system and non-corrosive Cu based electrolyte. This dye may become a promising starting point to develop DSSCs employing low-cost, non-toxic components such as iron-based dyes and copper-based electrolytes. In addition, the experimentally observed HOMO and LUMO values are in good agreement with the theoretical calculation using the DFT-B3LYP method with 6-31+G** as the basis set. The ester compound 1 is a good second-order NLO-phore in solution and could be a precursor for the preparation of second harmonic generation active polymeric films. Higher DSSC efficiencies could be achieved by using effective ferrocenyl conjugated D–π–A systems, which absorb more in the visible region, and this is currently in progress in our laboratory.
Conflicts of interest
There are no conflicts to declare.
Acknowledgements
We gratefully acknowledge the financial support from the DST Indo-Italian Joint Project (no. INT/Italy/P-15/2016(SP). S. P. thanks the Indian Council of Medical Research (ICMR) for the Senior Research Fellowship (SRF) [File No. 45/30/2020-BIO/BMS]. The authors thank VIT for providing “VIT SEED GRAND (File No. SG20210122)” for carrying out this research work. The authors gratefully acknowledge the VIT-SIF for providing the instrumental facilities.
References
-
(a) B. O'regan and M. Grätzel, A low-cost, high-efficiency solar cell based on dye-sensitized colloidal TiO2 films, Nature, 1991, 353(6346), 737–740 CrossRef;
(b) M. Urbani, M. Grätzel, M. K. Nazeeruddin and T. Torres, Meso-substituted porphyrins for dye-sensitized solar cells, Chem. Rev., 2014, 114(24), 12330–12396 CrossRef CAS PubMed.
- S. Mathew, A. Yella, P. Gao, R. Humphry-Baker, B. F. Curchod, N. Ashari-Astani, I. Tavernelli, U. Rothlisberger, S. M. Nazeeruddin and M. Grätzel, Dye-sensitized solar cells with 13% efficiency achieved through the molecular engineering of porphyrin sensitizers, Nat. Chem., 2014, 6(3), 242–247 CrossRef CAS PubMed.
-
(a) M. Grätzel, Recent advances in sensitized mesoscopic solar cells, Acc. Chem. Res., 2009, 42(11), 1788–1798 CrossRef PubMed;
(b) A. Yella, C. L. Mai, S. M. Zakeeruddin, S. N. Chang, C. H. Hsieh, C. Y. Yeh and M. Grätzel, Molecular engineering of push–pull porphyrin dyes for highly efficient dye-sensitized solar cells: The role of benzene spacers, Angew. Chem., Int. Ed., 2014, 126(11), 3017–3021 CrossRef.
- F. Gao, Y. Wang, D. Shi, J. Zhang, M. Wang, X. Jing, R. Humphry-Baker, P. Wang, S. M. Zakeeruddin and M. Gratzel, Enhance the optical absorptivity of nanocrystalline TiO2 film with high molar extinction coefficient ruthenium sensitizers for high performance dye-sensitized solar cells, J. Am. Chem. Soc., 2008, 130(32), 10720–10728 CrossRef CAS PubMed.
- D. Kuang, S. Ito, B. Wenger, C. Klein, J. E. Moser, R. Humphry-Baker, S. M. Zakeeruddin and M. Gratzel, High molar extinction coefficient heteroleptic ruthenium complexes for thin film dye-sensitized solar cells, J. Am. Chem. Soc., 2006, 128(12), 4146–4154 CrossRef CAS PubMed.
- S. Aghazada, P. Gao, A. Yella, G. Marotta, T. Moehl, J. Teuscher, J. E. Moser, F. De Angelis, M. Grätzel and M. K. Nazeeruddin, Ligand engineering for the efficient dye-sensitized solar cells with ruthenium sensitizers and cobalt electrolytes, Inorg. Chem., 2016, 55(13), 6653–6659 CrossRef CAS PubMed.
- Y. Huang, W. C. Chen, X. X. Zhang, R. Ghadari, X. Q. Fang, T. Yu and F. T. Kong, Ruthenium complexes as sensitizers with phenyl-based bipyridine anchoring ligands for efficient dye-sensitized solar cells, J. Mater. Chem. C, 2018, 6(35), 9445–9452 RSC.
- C. Dragonetti, A. Colombo, M. Magni, P. Mussini, F. Nisic, D. Roberto, R. Ugo, A. Valore, A. Valsecchi, P. Salvatori, M. G. Lobello and F. De Angelis, Thiocyanate-free ruthenium(II) sensitizer with a pyrid-2-yltetrazolate ligand for dye-sensitized solar cells, Inorg. Chem., 2013, 52(19), 10723–10725 CrossRef CAS PubMed.
- L. Mauri, A. Colombo, C. Dragonetti, D. Roberto and F. Fagnani, Recent Investigations on Thiocyanate-Free Ruthenium(II) 2,2’-Bipyridyl Complexes for Dye-Sensitized Solar Cells, Molecules, 2021, 26, 7638 CrossRef CAS PubMed.
- H. Yin, S. Chen, S. H. Cheung, H. W. Li, Y. Xie, S. W. Tsang, X. Zhu and S. K. So, Porphyrin-based thick-film bulk-heterojunction solar cells for indoor light harvesting, J. Mater. Chem. C, 2018, 6(34), 9111–9118 RSC.
- A. Magomedov, E. Kasparavičius, K. Rakstys, S. Paek, N. Gasilova, K. Genevičius, G. Juška, T. Malinauskas, M. K. Nazeeruddin and V. Getautis, Pyridination of hole transporting material in perovskite
solar cells questions the long-term stability, J. Mater. Chem. C, 2018, 6(33), 8874–8878 RSC.
- T. Higashino and H. Imahori, Porphyrins as excellent dyes for dye-sensitized solar cells: recent developments and insights, Dalton Trans., 2015, 44(2), 448–463 RSC.
-
(a)
P. Stepnicka, Ferrocenes: Ligands, Materials and Biomolecules, John Wiley and Sons Ltd., 2008 CrossRef;
(b) J. Conradie, T. S. Cameron, M. A. S. Aquino, G. J. Lamprecht and J. C. Swarts, Synthetic, electrochemical and structural aspects of a series of ferrocene-containing dicarbonyl β-diketonato rhodium(I) complexes, Inorg. Chim. Acta, 2005, 358, 2530–2542 CrossRef CAS.
-
(a) A. Kumar, R. Chauhan, K. C. Molloy, G. Kociok-Köhn, L. Bahadur and N. Singh, Synthesis, structure and light-harvesting properties of some new transition-metal dithiocarbamates involving ferrocene, Chem. – Eur. J., 2010, 16(14), 4307–4314 CrossRef CAS PubMed;
(b) V. Singh, R. Chauhan, A. N. Gupta, V. Kumar, M. G. Drew, L. Bahadur and N. Singh, Photosensitizing activity of ferrocenyl bearing Ni(II) and Cu(II) dithiocarbamates in dye sensitized TiO2 solar cells, Dalton Trans., 2014, 43(12), 4752–4761 RSC.
- R. Misra, R. Maragani, K. R. Patel and G. D. Sharma, Synthesis, optical and electrochemical properties of new ferrocenyl substituted triphenylamine based donor–acceptor dyes for dye sensitized solar cells, RSC Adv., 2014, 4(66), 34904–34911 RSC.
- D. Sirbu, C. Turta, A. C. Benniston, F. Abou-Chahine, H. Lemmetyinen, N. V. Tkachenko, C. Wood and E. Gibson, Synthesis and properties of a meso-tris–ferrocene appended zinc(II) porphyrin and a critical evaluation of its dye sensitised solar cell (DSSC) performance, RSC Adv., 2014, 4(43), 22733–22742 RSC.
- Y. Patil, R. Misra, R. Singhal and G. D. Sharma, Ferrocene-diketopyrrolopyrrole based non-fullerene acceptors for bulk heterojunction polymer solar cells, J. Mater. Chem. A, 2017, 5(26), 13625–13633 RSC.
- R. Yadav, A. Singh, G. Kociok-Köhn, R. Chauhan, A. Kumar and S. Gosavi, Ferrocenyl benzimidazole with carboxylic and nitro anchors as potential sensitizers in dye-sensitized solar cells, New J. Chem., 2017, 41(15), 7312–7321 RSC.
- R. Chauhan, M. Shahid, M. Trivedi, D. P. Amalnerkar and A. Kumar, Dye-Sensitized Solar Cells with Biferrocenyl Antennae Having Quinoxaline Spacers, Eur. J. Inorg. Chem., 2015, 3700–3707 CrossRef CAS.
- M. Cariello, S. Ahn, K. W. Park, S. K. Chang, J. Hong and G. Cooke, An investigation of the role increasing π-conjugation has on the efficiency of dye-sensitized solar cells fabricated from ferrocene-based dyes, RSC Adv., 2016, 6(11), 9132–9138 RSC.
- A. Ghosh, S. Mishra, S. Giri, S. M. Mobin, A. Bera and S. Chatterjee, Electrolyte-free dye-sensitized solar cell with high open circuit voltage using a bifunctional ferrocene-based cyanovinyl molecule as dye and redox couple, Organometallics, 2018, 37(13), 1999–2002 CrossRef CAS.
- A. Singh, G. Kociok-Köhn, R. Chauhan, M. Muddassir, S. W. Gosavi and A. Kumar, Ferrocene Appended Asymmetric Sensitizers with Azine Spacers with phenolic/nitro anchors for Dye-Sensitized Solar Cells, J. Mol. Struct., 2022, 1249, 131630 CrossRef CAS.
- R. Chauhan, M. Trivedi, L. Bahadur and A. Kumar, Application of π-extended ferrocene with varied anchoring groups as photosensitizers in TiO2-based dye-sensitized solar cells (DSSCs), Chem. – Asian J., 2011, 6(6), 1525–1532 CrossRef CAS PubMed.
- J. Kulhánek and F. Bureš, Imidazole as a parent π-conjugated backbone in charge-transfer chromophores, Beilstein J. Org. Chem., 2012, 8(1), 25–49 CrossRef PubMed.
-
(a) Y. Wu and W. Zhu, Organic sensitizers from D–π–A
to D–A–π–A: effect of the internal electron-withdrawing units on molecular absorption, energy levels and photovoltaic performances, Chem. Soc. Rev., 2013, 42(5), 2039–2058 RSC;
(b) J. Kulhánek, F. Bureš, T. Mikysek, J. Ludvík and O. Pytela, Imidazole as a central π-linkage in Y-shaped push–pull chromophores, Dyes Pigm., 2011, 90(1), 48–55 CrossRef.
-
(a) D. Kumar, K. R. Justin Thomas, C. P. Lee and K. C. Ho, Organic dyes containing fluorene decorated with imidazole units for dye-sensitized solar cells, J. Org. Chem., 2014, 79(7), 3159–3172 CrossRef CAS PubMed;
(b) G. B. Bodedla, X. Zhu, Z. Zhou and W. Y. Wong, Small molecules containing amphoteric imidazole motifs as sensitizers for dye-sensitized solar cells: An overview, Top. Curr. Chem., 2022, 380(6), 49 CrossRef CAS PubMed.
-
(a) J. Ren, S. M. Wang, L. F. Wu, Z. X. Xu and B. H. Dong, Synthesis and properties of novel Y-shaped NLO molecules containing thiazole and imidazole chromophores, Dyes Pigm., 2008, 76(2), 310–314 CrossRef CAS;
(b) J. Santos, E. A. Mintz, O. Zehnder, C. Bosshard, X. R. Bu and P. Günter, New class of imidazoles incorporated with thiophenevinyl conjugation pathway for robust nonlinear optical chromophores, Tetrahedron Lett., 2001, 42(5), 805–808 CrossRef CAS.
- M. B. Desta, S. Chaurasia and J. T. Lin, Reversed Y-shape di-anchoring sensitizers for dye sensitized solar cells based on benzimidazole core, Dyes Pigm., 2017, 140, 441–451 CrossRef.
- J. E. Kwon, S. Park and S. Y. Park, Realizing molecular pixel system for full-color fluorescence reproduction: RGB-emitting molecular mixture free from energy transfer crosstalk, J. Am. Chem. Soc., 2013, 135(30), 11239–11246 CrossRef CAS PubMed.
- K. Feng, L. De Boni, L. Misoguti, C. R. Mendonça, M. Meador, F. L. Hsu and X. R. Bu, Y-shaped two-photon absorbing molecules with an imidazole–thiazole core, Chem. Commun., 2004, 1178–1180 RSC.
-
(a) K. Senthilkumar, K. Thirumoorthy, C. Dragonetti, D. Marinotto, S. Righetto, A. Colombo, M. Haukka and N. Palanisami, Ferrocene–quinoxaline Y-shaped chromophores as fascinating second-order NLO building blocks for long lasting highly active SHG polymeric films, Dalton Trans., 2016, 45(30), 11939–11943 RSC;
(b) S. Prabu, E. David, T. Viswanathan, K. Thirumoorthy, T. Panda, C. Dragonetti, A. Colombo, D. Marinotto, S. Righetto, D. Roberto and N. Palanisami, NLO-active Y-shaped ferrocene conjugated imidazole chromophores as precursors for SHG polymeric films, Dalton Trans., 2020, 49(6), 1854–1863 RSC.
-
(a) G. Boschloo and A. Hagfeldt, Characteristics of the iodide/triiodide redox mediator in dye-sensitized solar cells, Acc. Chem. Res., 2009, 42(11), 1819–1826 CrossRef CAS PubMed;
(b) S. N. A. M. Nizar, M. M. Rosli, S. A. M. Samsuri, I. A. Razak and S. Arshad, Involvement of halogen and polyaromatic substituents in chalcone derivatives as dye sensitizers in solar cell applications, New J. Chem., 2023, 47(12), 5804–5815 RSC.
- S. Yanagida, Y. Yu and K. Manseki, Iodine/iodide-free dye-sensitized solar cells, Acc. Chem. Res., 2009, 42(11), 1827–1838 CrossRef CAS PubMed.
- M. Magni, R. Giannuzzi, A. Colombo, M. P. Cipolla, C. Dragonetti, S. Caramori, S. Carli, R. Grisorio, G. P. Suranna, C. A. Bignozzi, D. Roberto and M. Manca, Tetracoordinated Bis-phenanthroline Copper-Complex Couple as Efficient Redox Mediators for Dye Solar Cells, Inorg. Chem., 2016, 55, 5245–5253 CrossRef CAS PubMed.
-
(a) M. Magni, P. Biagini, A. Colombo, C. Dragonetti, D. Roberto and A. Valore, Versatile copper complexes as a convenient springboard for both dyes and redox mediators in dye sensitized solar cells, Coord. Chem. Rev., 2016, 322, 69–93 CrossRef CAS;
(b) L. Mauri, A. Colombo, C. Dragonetti and F. Fagnani, A fascinating trip into iron and copper dyes for DSSCs, Inorganics, 2022, 10(9), 137–167 CrossRef CAS.
-
(a)
H. B. Gray and E. I. Solomon, in Copper Proteins, ed. T. G. Spiro, Wiley, New York, 1981, pp. 1–39 Search PubMed;
(b) A. G. Sykes, Active-site properties of the blue copper proteins, Adv. Inorg. Chem., 1991, 36, 377–408 CrossRef CAS;
(c) D. B. Rorabacher, Electron transfer by copper centers, Chem. Rev., 2004, 104(2), 651–698 CrossRef CAS PubMed;
(d) A. Colombo, C. Dragonetti, D. Roberto and F. Fagnani, Copper Complexes as Alternative Redox Mediators in Dye-Sensitized Solar Cells, Molecules, 2021, 26, 194–213 CrossRef CAS PubMed.
- N. Alonso-Vante, J. F. Nierengarten and J. P. Sauvage, Spectral sensitization of large-band-gap semiconductors (thin films and ceramics) by a carboxylated bis (1, 10-phenanthroline) copper(I) complex, J. Chem. Soc., Dalton Trans., 1994, 11, 1649–1654 RSC.
- A. Colombo, C. Dragonetti, D. Roberto, A. Valore, P. Biagini and F. Melchiorre, A simple copper(I) complex and its application in efficient dye sensitized solar cells, Inorg. Chim. Acta, 2013, 407, 204–209 CrossRef CAS.
- M. L. Green, S. R. Marder, M. E. Thompson, J. A. Bandy, D. Bloor, P. V. Kolinsky and R. J. Jones, Synthesis and structure of (cis)-[1-ferrocenyl-2-(4-nitrophenyl) ethylene], an organotransition metal compound with a large second-order optical nonlinearity, Nature, 1987, 330(6146), 360–362 CrossRef CAS.
-
(a) S. D. Bella, Second-order nonlinear optical properties of transition metal complexes, Chem. Soc. Rev., 2001, 30(6), 355–366 RSC;
(b) F. Tessore, D. Roberto, R. Ugo, P. Mussini, S. Quici, I. Ledoux-Rak and J. Zyss, Large, Concentration-dependent enhancement of the quadratic hyperpolarizability of [Zn(CH3CO2)2 (L)2] in CHCl3 on substitution of acetate by triflate, Angew. Chem., Int. Ed., 2003, 42(4), 456–459 CrossRef CAS PubMed;
(c) E. Rossi, A. Colombo, C. Dragonetti, S. Righetto, D. Roberto, R. Ugo, A. Valore, J. G. Williams, M. G. Lobello, F. De Angelis and S. Fantacci, Tuning the dipolar second-Order nonlinear optical properties of cyclometalated platinum(II) complexes with tridentate N^C^N binding ligands, Chem. Eur. J., 2013, 19(30), 9875–9883 CrossRef CAS PubMed;
(d)
S. Di Bella, C. Dragonetti, M. Pizzotti, D. Roberto, F. Tessore and R. Ugo, in Topics in organometallic chemistry 28. Molecular organometallic materials for optics, ed. H. L. Bozec and V. Guerchais, Springer-Verlag, Berlin/Heidelberg, 2010, pp. 1–55 Search PubMed;
(e) M. Fontani, E. Garoni, A. Colombo, C. Dragonetti, S. Fantacci, H. Doucet, J. F. Soulé, J. Boixel, V. Guerchais and D. Roberto, Novel cyclometallated 5-π-delocalized donor-1,3-di(2-pyridyl)benzene platinum(II) complexes with good second-order nonlinear optical properties, Dalton Trans., 2019, 48(1), 202–208 RSC;
(f) A. Colombo, C. Dragonetti, V. Guerchais, C. Hierlinger, E. Zysman-Colman and D. Roberto, A trip in the nonlinear optical properties of iridium complexes, Coord. Chem. Rev., 2020, 414, 213293–213313 CrossRef CAS.
- S. Kaur, M. Kaur, P. Kaur, K. Clays and K. Singh, Ferrocene chromophores continue to inspire fine-tuning and switching of the second-order nonlinear optical response, Coord. Chem. Rev., 2017, 343, 185–219 CrossRef CAS.
- F. Nisic, A. Colombo, C. Dragonetti, E. Garoni, D. Marinotto, S. Righetto, F. De Angelis, M. G. Lobello, P. Salvatori, P. Biagini and F. Melchiorre, Functionalized ruthenium dialkynyl complexes with high second-order nonlinear optical properties and good potential as dye sensitizers for solar cells, Organometallics, 2015, 34(1), 94–104 CrossRef CAS.
- G. Sathyaraj, D. Muthamilselvan, M. Kiruthika, T. Weyhermüller and B. U. Nair, Ferrocene conjugated imidazolephenols as multichannel ditopic chemosensor for biologically active cations and anions, J. Organomet. Chem., 2012, 716, 150–158 CrossRef CAS.
-
(a) C. Lee, W. Yang and R. G. Parr, Development of the Colle-Salvetti correlation-energy formula into a functional of the electron density, Phys. Rev. B: Condens. Matter Mater. Phys., 1988, 37, 785 CrossRef CAS PubMed;
(b)
R. G. Parr and Y. Weitao, Density-functional theory of atoms and molecules, Oxford University Press, Oxford, 1994 Search PubMed.
-
M. J. Frisch, G. W. Trucks, H. B. Schlegel, G. E. Scuseria, M. A. Robb, J. R. Cheeseman, G. Scalmani, V. Barone, B. Mennucci, G. A. Petersson, H. Nakatsuji, M. Caricato, X. Li, H. P. Hratchian, A. F. Izmaylov, J. Bloino, G. Zheng, J. L. Sonnenberg, M. Hada, M. Ehara, K. Toyota, R. Fukuda, J. Hasegawa, M. Ishida, T. Nakajima, Y. Honda, O. Kitao, H. Nakai, T. Vreven, J. A. Montgomery, J. E. Peralta, F. Ogliaro, M. Bearpark, J. J. Heyd, E. Brothers, K. N. Kudin, V. N. Staroverov, R. Kobayashi, J. Normand, K. Raghavachari, A. Rendell, J. C. Burant, S. S. Iyengar, J. Tomasi, M. Cossi, N. Rega, J. M. Millam, M. Klene, J. E. Knox, J. B. Cross, V. Bakken, C. Adamo, J. Jaramillo, R. Gomperts, R. E. Stratmann, O. Yazyev, A. J. Austin, R. Cammi, C. Pomelli, J. W. Ochterski, R. L. Martin, K. Morokuma, V. G. Zakrzewski, G. A. Voth, P. Salvador, J. J. Dannenberg, S. Dapprich, A. D. Daniels, O. Farkas, J. B. Foresman, J. V. Ortiz, J. Cioslowski and D. J. Fox, Gaussian16 Rev. C.01, Gaussian, Inc., Wallingford CT, 2009 Search PubMed.
-
R. D. Dennington, T. A. Keith and J. Millam, GaussView Version 6.1.16, Semichem Inc., Shawnee Mission. KS, 2016 Search PubMed.
-
(a) B. F. Levine and C. G. Bethea, Second and third order hyperpolarizabilities of organic molecules, J. Chem. Phys., 1975, 63(6), 2666–2682 CrossRef CAS;
(b) I. Ledoux and J. Zyss, Influence of the molecular environment in solution measurements of the Second-order optical susceptibility for urea and derivatives, Chem. Phys., 1982, 73, 203–213 CrossRef CAS.
-
(a) E. David, T. Viswanathan, S. Prabu and N. Palanisami, N-Arylated bisferrocene pyrazole for the dual-mode detection of hydrogen peroxide: an AIE-active fluorescent ‘‘turn ON/OFF’’ and electrochemical non-enzymatic sensor, New J. Chem., 2019, 43, 8539 RSC;
(b) S. Prabu and N. Palanisami, Aggregation induced emission (AIE)-active ferrocene conjugated linear π-extended multi donor–π–acceptor (D–D′–π–A) chromophores: synthesis, structural, theoretical, linear and nonlinear optical studies, Dyes Pigm., 2022, 201, 110193 CrossRef CAS.
- S. Prabu and N. Palanisami, Ferrocene appended Y-shaped imidazole aldehyde chromophores: Synthesis, the effect of alkyl chain in the second order nonlinear optical properties and theoretical studies, J. Mol. Struct., 2022, 1264, 133137 CrossRef CAS.
- C. M. Cardona, W. Li, A. E. Kaifer, D. Stockdale and G. C. Bazan, Electrochemical considerations for determining absolute frontier orbital energy levels of conjugated polymers for solar cell applications, Adv. Mater., 2011, 23, 2367–2371 CrossRef CAS PubMed.
- K. Senthilkumar, M. Pizzotti, K. Thirumoorthy, G. Di Carlo, S. Righetto, A. Orbelli Biroli, M. Haukka and N. Palanisami, New internal-charge-transfer second-order nonlinear optical chromophores based on the donor ferrocenyl pyrazole moiety, J. Phys. Chem. C, 2016, 120(36), 20277–202311 CrossRef CAS.
- Y. S. Sohn, D. N. Hendrickson and H. B. Gray, Electronic structure of metallocenes, J. Am. Chem. Soc., 1971, 93(15), 3603–3612 CrossRef CAS.
- R. Chauhan, R. Yadav, A. K. Singh, M. Trivedi, G. Kociok-Köhn, A. Kumar, S. Gosavi and S. Rane, Ferrocenyl chalcones with phenolic and pyridyl anchors as potential sensitizers in dyesensitized solar cells, RSC Adv., 2016, 6(100), 97664–97675 RSC.
-
(a) C. Dragonetti, M. Magni, A. Colombo, F. Fagnani, D. Roberto, F. Melchiorre, P. Biagini and S. Fantacci, Towards efficient sustainable full-copper dye-sensitized solar cells, Dalton Trans., 2019, 48, 9703–9711 RSC;
(b) C. Dragonetti, M. Magni, A. Colombo, F. Melchiorre, P. Biagini and D. Roberto, Coupling of a Copper Dye with a Copper Electrolyte: A Fascinating Springboard for Sustainable Dye-Sensitized Solar Cells, ACS Appl. Energy Mater., 2018, 1, 751–756 CrossRef CAS;
(c) A. Colombo, C. Dragonetti, F. Fagnani, D. Roberto, F. Melchiorre and P. Biagini, Improving the efficiency of copper-dye-sensitized solar cells by manipulating the electrolyte solution, Dalton Trans., 2019, 48, 9818–9823 RSC.
- H. Tian and L. Sun, Iodine-free redox couples for dye-sensitized solar cells, J. Mater. Chem., 2011, 21(29), 10592–10601 RSC.
- S. Prabu, T. Viswanathan, E. David, S. Jagadeeswari and N. Palanisami, Enhancement of photovoltaic performance in ferrocenyl π-extended multi donor–πacceptor (D–D′–π–A) dyes using chenodeoxycholic acid as a dye co-adsorbent for dye sensitized solar cells, RSC Adv., 2023, 13, 9761–9772 RSC.
- M. S. An, S. W. Kim and J. D. Hong, Synthesis and characterization of peripherally ferrocene-modified zinc phthalocyanine for dye-sensitized solar cell, Bull. Korean Chem. Soc., 2010, 31(11), 3272–3278 CrossRef CAS.
- W. Yang, N. Vlachopoulos, Y. Hao, A. Hagfeldt and G. Boschloo, Efficient dye regeneration at low driving force achieved in triphenylamine dye LEG4 and TEMPO redox mediator based dye-sensitized solar cells, Phys. Chem. Chem. Phys., 2015, 17(24), 15868–15875 RSC.
- A. H. Anizaim, D. A. Zainuri, M. F. Zaini, I. A. Razak, H. Bakhtiar and S. Arshad, Comparative analyses of new donor–π–acceptor ferrocenyl-chalcones containing fluoro and methoxy-fluoro acceptor units as synthesized dyes for organic solar cell material, PLoS One, 2020, 15(11), e0241113 CrossRef CAS PubMed.
Footnote |
† Electronic supplementary information (ESI) available: Characterization data (1H and 13C NMR spectra, ESI-mass data); FT-IR, computational studies (DFT/TD-DFT). See DOI: https://doi.org/10.1039/d3nj03668e |
|
This journal is © The Royal Society of Chemistry and the Centre National de la Recherche Scientifique 2024 |
Click here to see how this site uses Cookies. View our privacy policy here.