DOI:
10.1039/D3NJ03993E
(Paper)
New J. Chem., 2024,
48, 193-202
π-Extended indoloquinoxaline functionalized triarylamines with ethynyl and tetracyanobutadiene bridges for p-channel and ambipolar OFETs†
Received
25th August 2023
, Accepted 16th November 2023
First published on 21st November 2023
Abstract
New D–A indoloquinoxaline functionalized triarylamine semiconductors with various π-bridging motifs were designed and synthesized for p-channel and ambipolar organic transistors. This is the first attempt to use indoloquinoxaline as an effective inbuilt donor system for air-stable organic field-effect transistors (OFETs). The structure–property relationship of triarylamine–indoloquinoxalines (TAA–IQ) is systematically studied in terms of their electrochemical, photophysical, structural, and electrical characteristics. Bottom-gate top contact OFET devices fabricated using TAA–IQ with an ethynyl spacer exhibited p-channel characteristics with a field-effect mobility of 1.50 cm2 V−1 s−1 and an on/off current ratio of 105. The electron-deficient TCBD group, as a central acceptor in the TAA–IQ architecture, exhibited low-lying LUMO energy levels at around −3.96 eV, allowing easy electron injection and stable electron transfer under ambient conditions. The TCBD bridged TAA–IQ compound exhibited ambipolar characteristics with the highest hole mobility μh of 0.35 cm2 V−1 s−1, an on/off current ratio of up to 106 and an electron mobility μe of 2.1 cm2 V−1 s−1. Remarkably, the devices based on IQ–TAA with TCBD showed a much better performance than those based on the related ambipolar compounds.
Introduction
Organic electronics have evolved into an exciting technological area replacing conventional inorganic electronics in the past two decades. The huge demand for flexible, low-cost, solution-processable organic electronic materials continues because these semiconductors can be precisely synthesized at a cheaper cost. The properties of the organic semiconductors can be easily tailored through structural modifications to meet several requirements.1,2 Among all the electronic applications of organic semiconductors, organic field-effect transistors (OFETs) are some of the essential fundamental building blocks of integrated circuits, e-cards, radio frequency identification (RFID) tags, etc.3–7 Many organic semiconductors with good stability and charge carrier mobility have been reported.8–13 However, new organic semiconductors with good characteristics are highly desired to utilize their real-world applications in flexible electronic systems.
Recent studies show that the appropriate construction of a donor–acceptor (D–A) framework is feasible for creating new semiconductors with interesting electronic properties.14–16 To date, numerous D–A-based small molecules and macromolecules have been reported for hole, electron, and ambipolar transport.14,17 In this regard, a wide range of electron donors or acceptors have been extensively investigated, and some of the π-conjugated D–A frameworks, including indigo, isoindigo, diketopyrrolopyrroles and quinolines, have been reported as favorable backbone structures for designing high performance ambipolar transistors.18–21 The better ambipolar properties of these D–A systems are highly associated with the following structural and electronic factors: (i) better interaction of the highest occupied molecular orbitals (HOMO) of the donor unit with the lowest un-occupied molecular orbital (LUMO) of the acceptor unit and (ii) planarity and self-assembly of the molecular skeleton and functional groups, respectively.22 D–A molecules generally consist of electron-rich donor and electron-deficient acceptor units arranged in an order that decides a semiconductor's electron/charge transport.23,24 While p-channel behavior has been observed in most D–A types, the strong ambipolar or n-type behavior depends on the strength of the acceptor.25 The exact role of the acceptor unit in n-channel transport is unclear, which is notable for studying.
6H-Indolo[2,3-b]quinoxaline (IQ) is an inbuilt donor–acceptor system, fusing electron-rich indole with electron-deficient quinoxaline.26,27 These unique properties endow IQ with intramolecular charge transfer (ICT) characteristics, valid for the system's charge transportation, stability, and durability. In addition, the planarity of the system and the ability to form hydrogen bonding and van der Waals interactions result in good molecular packing.22 However, in the context of a strong donor–acceptor, IQ can be defined as electron neutral22 as it has electron-donating and accepting nitrogen atoms. Still, based on the connecting group, it is presumed to be a weak donor28 or weak acceptor.29 Recently, indoloquinoxaline molecules have been utilized as active materials in organic solar cells and organic light-emitting diodes due to their excellent emission and electron transport properties and high thermal stability and durability.30–32 In the case OFETs, indole-fused or quinoxaline-fused heteroaromatic systems have been studied, but indole-fused quinoxaline systems have not been explored. The high-performance hole transport transistors are made of the indolo[3,2-b] indole-based π-conjugated molecule-fused heteroaromatic core showing a hole mobility of up to μh = 0.9 cm2 V−1 s−1.33 Baumgarten et al.34 reported quinoxaline fused naphthalenediimides, and the OFETs prepared using these molecules exhibited a maximum n-type mobility of 0.03 cm2 V−1 s−1 with an on/off ratio of 2 × 105.
In this article, we have designed and synthesized a new class of indoloquinoxaline–triarylamines with three different architectures TAA-IQ (8a–b), TAA-π-IQ (9a–b), and TAA-TCBD-IQ (10a–b) for high-performance OFETs. We first attempted to use indoloquinoxaline as an effective inbuilt donor–acceptor system for OFETs. We believed that the fusion of electron-deficient quinoxaline and electron-rich indole fragments might significantly enhance photophysical, electrochemical, and self-assembly properties. The alkyl chain connected to IQ induces non-covalent interactions, which are expected to form better self-assembly in molecular packing. TAA was chosen as a donor due to its peculiar propeller shape, electron-donating ability, high ionization potential, solubility, and superior hole-transporting properties.35–37 Besides, synthetic versatility is another advantage for making TAA with various arrangements.35 We have also introduced various linkers between the TAA and IQ units, such as ethynyl38,39 and tetracyano butadiene (TCBD) spacers. TCBD was chosen as a potent bridging acceptor unit to increase n-channel character through intramolecular charge transfer (ICT) and high thermal stability. It was employed in various optoelectronic and thermoelectric devices by utilizing its self-assembly and better electronic properties.40–43 Yuan44 and coworkers used the TCBD moiety to synthesize TCBD adducted polyyne molecules for organic solar cells. The push–pull systems exhibited low LUMO levels leading to the formation of low energy gaps. The easy synthetic methods also led to the formation of a variety of molecules for a wide range of applications.45,46 These properties encouraged us to use TCBD for transistor applications. TCBD and TAA are strong electron-accepting and donating units; IQ is considered as a weak acceptor or π-terminal unit. Among our newly designed molecules, directly connected TAA–IQ (8a–b) and ethynyl bridged TAA–π–IQ (9a–b) exhibited p-channel characteristics with the highest field effect mobility of 1.50 cm2 V−1 s−1, which were transformed into ambipolar characteristics when TAA and IQ were connected through electron accepting TCBD (10a–b).
Results and discussion
Design and synthesis
The structures of the synthesized organic semiconductors 8a–b, 9a–b, and 10a–b are shown in Fig. 1. The structural engineering of these three different TAA–IQ, TAA–π–IQ, and TAA–TCBD–IQ systems contains TAA as a donor and the IQ unit as a fused donor-–acceptor unit, which support the ICT behavior. In addition, all the architectures contain triphenylamine or tBu-phenyl end-capped triphenylamine, which helps to investigate the effect of donor molecules’ strength on photophysical, electrochemical, and device performances. Also, these TAA and IQ end-groups are connected directly and through an ethynyl spacer and the TCBD acceptor unit to analyze the effect of the acceptor strength on OFET properties.
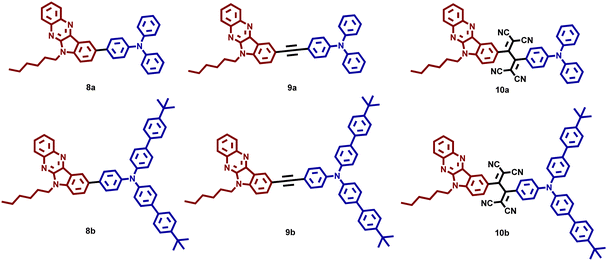 |
| Fig. 1 Molecular structures of compounds 8a–b, 9a–b and 10a–b. | |
The experimental details of compounds 1–10 are given in Schemes 1 and 2. Initially, 1-hexyl-5-bromoisatin (1) was prepared by treating 5-bromoisatin with 1-bromohexane with a 91% yield. Compound 1 has a hexyl unit as an alkyl chain at indole nitrogen to increase the solubility and facilitate the molecular self-assembly of the molecules. The condensation reaction of compound 1 was carried out with orthophenylene diamine to obtain 9-bromo-6-hexyl-6H-indolo[2,3-b]quinoxaline (2), which was then subsequently coupled with bis(pinacolato)diboron and 2-methylbut-3-yn-2-ol followed by deprotection to obtain 3 and 4, respectively. The functionalized TAAs 5–7 were synthesized as per the literature.35 Compounds 3 and 4 were appropriately transformed into various TAA derivatives 8a–b and 9a–bvia Suzuki and Sonogashira coupling reactions in the presence of Pd(PPh3)4 and Pd(PPh3)2Cl2 as the catalysts, respectively.
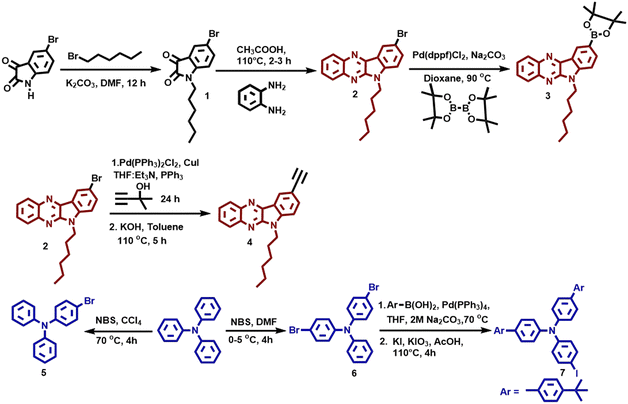 |
| Scheme 1 Synthetic route of compounds 1–7. | |
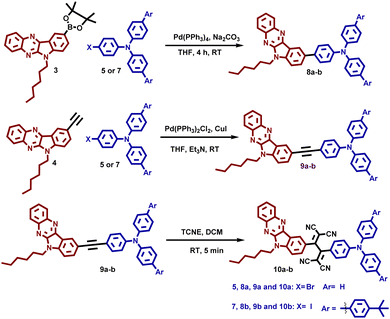 |
| Scheme 2 Synthetic route of compounds 8a–b, 9a–b and 10a–b. | |
Compounds 9a–b were finally reacted with tetracyanoethylene through [2+2] cycloaddition–retroelectrocyclization47,48 to afford compounds 10a–b with a high yield of ∼95%. The compounds are yellow (8a–b and 9a–b) and dark red (10a–b) in color, and the compounds are soluble in common organic solvents such as dichloromethane, chloroform, tetrahydrofuran, and dimethylformamide. The molecular structures of the synthesized compounds are characterized by NMR and high-resolution mass spectral techniques.
Photophysical properties
The absorption (10−5 M) and emission (<10−8 M) spectra of compounds 8a–b, 9a–b, and 10a–b were recorded in dichloromethane solution and are displayed in Fig. 2, and the relevant data are compiled in Table 1. The UV-vis spectra of 8a–b, 9a–b, and 10a–b are dominated by three major bands of IQ units around 260, 290, and 360 nm. The low energy bands are assigned to the n–π* transition, and higher energy bands are due to the π–π* transition of the IQ and TAA units.29 An extra minor band was observed around 430 nm due to the IQ's weak intramolecular charge transfer transition.30 This higher wavelength transition indicates the inbuilt D–A character attributed to ICT from the electron-rich indole to the electron-poor quinoxaline moiety. The CT probability from the indole to quinoxaline unit was slightly enhanced by introducing the electronegative sp-character (ethynyl spacer) between indoloquinoxaline and TAA. These ICT transitions were bathochromically shifted for derivatives 8b and 9b substituted with electron-donating t-butylphenyl connected triphenylamine compared to 8a and 9a, respectively. However, a minor peak above 400 nm having higher molar absorption for 10a–b with the tetracyano acceptor is due to the induced D–A character of indoloquinoxaline or ICT from the indoloquinoxaline to tetracyano unit.
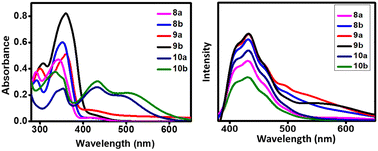 |
| Fig. 2 Absorption and emission spectra of compounds 8a–b, 9a–b and 10a–b in dichloromethane. | |
Table 1 Photophysical properties of compounds 8a–b, 9a–b and 10a–b
|
λ
abs (nm) |
λ
em (nm) |
Stokes shift (nm) |
ε (103 × dm3 mol−1 cm−1) |
Quantum yield (ϕF) |
Stokes shift was calculated by subtracting λem from n–π* λabs Quantum yield determined by using quinine sulphate as a standard. |
8a
|
426, 343, 291 |
432 |
89 |
47 |
0.35 |
8b
|
426, 361, 300 |
432 |
71 |
51 |
0.32 |
9a
|
428, 352, 292 |
432 |
80 |
60 |
0.38 |
9b
|
428, 360, 307 |
433 |
73 |
82 |
0.37 |
10a
|
495, 433, 354 |
431 |
77 |
26 |
0.08 |
10b
|
512, 434, 334 |
431 |
97 |
37 |
0.10 |
Interestingly, compounds 10a–b displayed a distinctive lower energy band around ∼500 nm, attributed to the ICT from the triphenylamine to the central tetracyano group. Furthermore, it can be found that the presence of the tert-butylphenyl substituent on TAA side arms in 10b has also increased the electron-donating character of triphenylamine. This allows compound 10b to show a significant red shift in the CT band from 10a, showing the significance of the electron-rich groups in narrowing the semiconductor band gap (Eg).27
All the compounds were excited at their absorption maxima (n–π*) to get the emission spectra. Compounds 8a–b and 9a–b show broad emission in the 370–650 nm range. On the other hand, compounds 10a–b exhibited emission spectra with a reduced emission intensity or quenching of emission. The compounds’ quantum yield (ΦF) was analyzed in dichloromethane to investigate the emission behavior. Compounds 10a–b showed low quantum yields of ∼0.1 compared to other compounds (Table 1).
Electrochemical properties
The redox behavior of compounds 8a–b, 9a–b and 10a–b was studied by the cyclic voltammetry technique. The cyclic voltammograms are given in Fig. S17 (ESI†); the data are compiled in Table 2. All of the compounds exhibited one electron quasi-reversible peak around 1 V corresponding to the removal of an electron from triphenylamine, and other oxidation waves arise from the indoloquinoxaline unit. The compounds’ oxidation potential (Eox) decreased with increasing electron-donating nature. Compounds 8b, 9b, and 10b with π-extended tert-butylphenyl substituents at TAA side arms possess the lowest oxidation potential compared to the corresponding compounds 8a, 9a, and 10a containing simple triphenylamine. In addition, 9a and 9b with an ethynyl spacer displayed a slightly increased oxidation potential compared to compounds 8a and 8b, respectively. Among the compounds, 8b possesses the lowest oxidation potential of 0.82 V, while the corresponding compound 9b possesses a higher oxidation potential due to the electron-accepting sp-hybridized ethynyl spacer.30,49 As expected, introducing electron-accepting TCBD into 10a–b enhances acceptor strength; thus, a positive shift is observed in the oxidation potential. For example, the first oxidation potential shifts towards a more positive value from 0.9 (8a) to 1.31 V (10a).
Table 2 Electrochemical properties of compounds 8a–b, 9a–b and 10a–b
|
E
ox (V) |
E
red (V) |
HOMO (eV) |
LUMO (eV) |
Band gap (eV) |
E
g (eV) |
E
ox - oxidation potential, Ered - reduction potential, and Eg - band gap. |
8a
|
0.90 |
−1.71 |
−5.246 |
−2.747 |
2.50 |
2.58 |
8b
|
0.82 |
−1.71 |
−5.166 |
−2.746 |
2.42 |
2.61 |
9a
|
0.95 |
−1.67 |
−5.301 |
−2.788 |
2.51 |
2.46 |
9b
|
0.86 |
−1.66 |
−5.214 |
−2.792 |
2.42 |
2.49 |
10a
|
1.31 |
−0.49 |
−5.659 |
−3.969 |
1.69 |
1.86 |
10b
|
1.16 |
−0.49 |
−5.506 |
−3.967 |
1.54 |
1.75 |
The more shifted oxidation potential due to the transfer of electron density from triphenylamine (donor) to the cyano group (acceptor) hinders the oxidation of the donor. Thus the anodic potential reflects the efficiency of the ground-state ICT directly.50 Furthermore, the cathodic sweep of compounds displays one quasi-reversible reduction wave around −1.60 V attributed to the addition of an electron to the quinoxaline moiety. In addition, two extra reversible reduction potentials (Ered) were observed for compounds 10a–b containing TCBD, ascribed to the formation of stable anion and dianion species at
C(CN)2 acceptor moieties.50,51 The HOMO and LUMO of the compounds were calculated from the Eox of ferrocene.52 Furthermore, the calculated HOMO–LUMO band gap from electrochemical (Δ(Eox,1–Ered,1)) analysis shows a linear correlation (R = 0.982) with the optical gaps estimated from the absorption edge of the UV spectra, indicating that the same molecular orbital is involved in both electrochemical and optical band-gaps.53 Among the molecules, 8b and 9b were observed with the highest-lying HOMO levels around −5.2 eV, which reduce the energy barrier for hole injection. Compounds 10a–b with tetracyano groups displayed low-lying HOMO and LUMO levels, illustrating that these compounds can act as ambipolar semiconductors. Furthermore, the reversibility of the reduction and oxidation processes and the observed frontier molecular orbitals show that they could transport both holes and electrons, being suitable bipolar semiconductors for OFETs.
Computational studies
It is well established that the electronic structure of semiconductors is a significant factor determining transistor performance. To gain more insights into the molecular structures of 8a–b, 9a–b, and 10a–b, they were optimized by DFT using the Gaussian 03 program at the B3LYP/6-31(d) level. The optimized structures were utilized for HOMO–LUMO energy distributions, listed in Fig. 3, and the energy levels are summarized in Table 2. Optimized configurations dictate the planarity of IQ terminal units and the propeller structure of the TAA. The band-gap and FMO energy levels derived from DFT analysis are quantitatively consistent with the experimental results. An organic semiconductor should have delocalized electron density distribution, an appropriate level of HOMO–LUMO, and peculiar geometry to facilitate efficient hole–electron transportation. The low-lying LUMO levels of 10a–b enable n-channel or ambipolar charge transport, and high-lying HOMO levels of 8a–b and 9a–b allow p-channel charge transport. The HOMOs of 8a–b and 9a–b were mainly spanned over the TAA and indole segments of the IQ unit, whereas LUMOs were spanned over IQ units. From this, it is evident that the electronic excitation from the HOMO to LUMO will result in a charge movement from TAA to the indoloquinoxaline unit.30 Besides, for compounds 10a–b, the HOMO is located on the TAA and the central unit, and the LUMO is spanned over the central TCBD unit and spread over the indole unit. The observed HOMO/LUMO values suggest that these compounds can be used as ambipolar semiconductors.
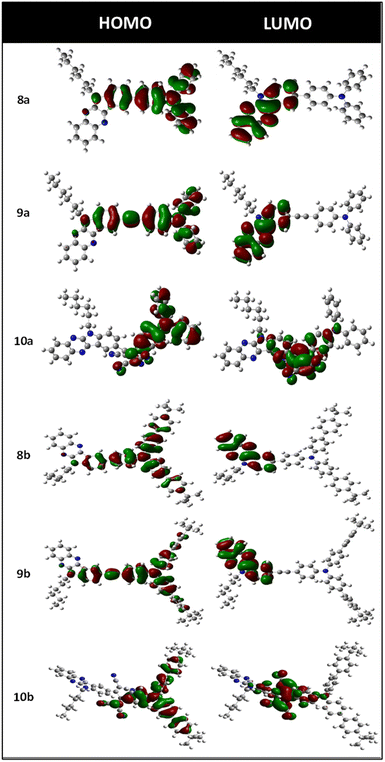 |
| Fig. 3 FMO diagrams of compounds 8a–b, 9a–b and 10a-b. | |
Time-dependent-SCF calculations were performed on these molecules to recognize the origin of exclusive spectroscopic properties of compounds 8a–b, 9a–b, and 10a–b. The optical bands of these compounds arise from the combinations of multiple HOMO−n → LUMO+m transitions, which are entirely in agreement with experimental results. The oscillator strength, transition energies, and the most relevant singlet excited state assignments are given in Table S1 (ESI†). The lowest energy transition of the compounds above 400–500 nm corresponds to the charge transfer from the HOMO to LUMO. The band around 350 nm is attributed to the multiple overlaps of π–π* transitions involving HOMO−1 and LUMO+1 orbitals.54
SEM and XRD analysis
The surface morphologies of fabricated thin films were analyzed by scanning electron microscopy. To control the self-assembly of the films, the spinning time, speed, solvent choice, and post-deposition methods were optimized because these are important challenges for organic devices.55–57Fig. 4 shows the variation in the microstructure with film thickness for thin films of compounds 8a–b, 9a–b, and 10a–b. First, a suspension of a homogeneous solution of semiconductors was spin-coated over the Si substrate and annealed for 40 minutes at 70 °C to remove the solvent to get a better self-assembled film. Thin films of compounds 8a–b were observed with flower and rod-like morphologies. On the other hand, films of 9a–b with tert-butylphenyl substituents exhibited a uniform rod-like structure. Although the same processing conditions were used, variations in the TAA-endcap and central bridging units were responsible for the hole, electron, and ambipolar activity. Thus, the surface morphology and self-assembly of the films were strongly dependent on the central bridge spacer system and TAA end-cap units. Especially, compounds 9a–b exhibited rod-like grains with an average length of 2 μm. Besides, compounds 10a and 10b with the TCBD substituent exhibited flower-like and rod-like crystals, respectively.
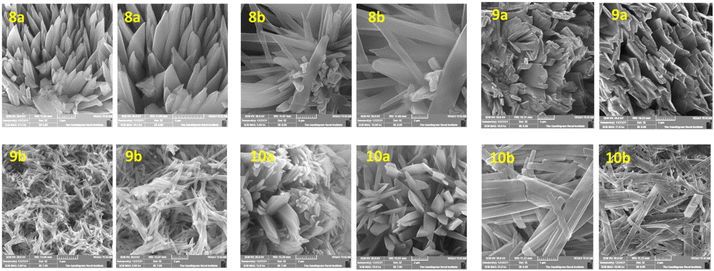 |
| Fig. 4 SEM images of thin films of compounds 8a–b, 9a–b and 10a–b. | |
X-ray diffraction measurements were performed to investigate semiconductor films’ orientations and interchain packing (Fig. S14, ESI†). The highest crystalline peaks observed for all the compounds indicate the long-range molecular ordering of the thin film.
Transistor characteristics
To evaluate the charge carrier properties of compounds 8a–b, 9a–b, and 10a–b, the bottom-gate top-contact OFETs have been fabricated and examined on Si/SiO2 surfaces. The output and transfer curves were measured, the charge transport performance of five devices is summarized in Table 3, and the characteristic curves are presented in Fig. 5 and 6. The average OFET mobilities obtained were calculated in the saturated region58 using the equation
(where ID is the drain current, VG is the gate voltage, C is the specific capacitance of SiO2, and L and W are the channel length and width, respectively) considering the kink observed in the transfer curve.59–61 The device performance was evaluated under ambient conditions. All the synthesized IQ-incorporated TAAs 8a–b and 9a–b exhibited typical p-channel semiconducting properties with high hole-transporting mobilities and TCBD-incorporated TAA–IQs 10a–b exhibited ambipolar behavior with enhanced n-channel characteristics due to their low lying LUMO (−3.97 eV) energy levels.62 Though all the molecules have donor–acceptor IQ units, other than 10a–b, no other molecules showed ambipolar behavior. This is because of the hindered electron transport from the weak acceptor moiety and the localized LUMO on the electron-deficient regions.63 In addition, the electron-donating nature of the TAA and indole fragments also dominates the hole-transport characteristics rather than electron transport.
Table 3 OFET characteristics of compounds 8a–b, 9a–b and 10a–b
|
μ
h cm2 V−1 s−1 |
μ
e cm2 V−1 s−1 |
|
μ
max
|
μ
Avg
|
On/off ratio |
V
Th (V) |
μ
max
|
μ
Avg
|
On/off ratio |
V
Th (V) |
Error bars are calculated from fifteen devices. |
8a
|
0.63 |
0.5 ± 0.12 |
105 |
−6 |
— |
|
— |
— |
9a
|
1.50 |
1.1 ± 0.4 |
105 |
−10 |
— |
|
— |
— |
10a
|
0.33 |
0.2 ± 0.12 |
106 |
−20 |
1.6 |
0.9 ± 0.7 |
105 |
19 |
8b
|
0.64 |
0.5 ± 0.1 |
105 |
−4 |
— |
|
— |
— |
9b
|
1.25 |
1.1 ± 0.1 |
106 |
−12 |
— |
|
— |
— |
10b
|
0.35 |
0.3 ± 0.05 |
105 |
−22 |
2.1 |
1.2 ± 0.8 |
106 |
22 |
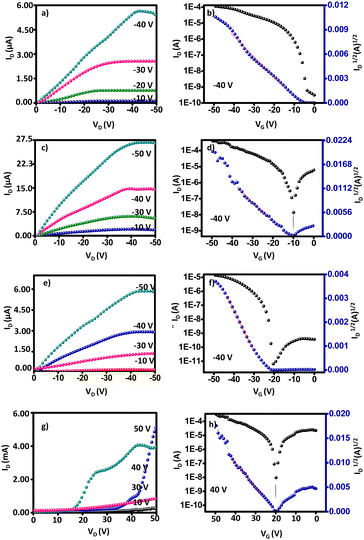 |
| Fig. 5 OFET characteristics of compounds 8a (a) and (b), 9a (c) and (d), 10a p-type (e) and (f), n-type (g) and (h). | |
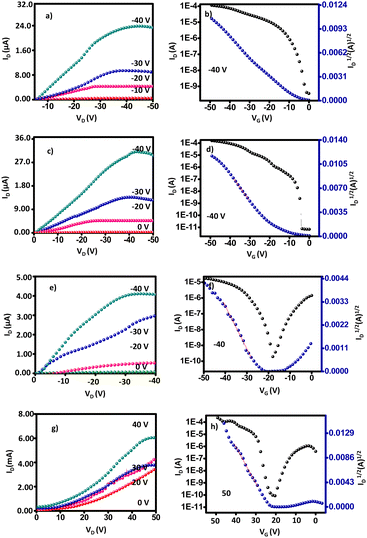 |
| Fig. 6 OFET characteristics of compounds 8b (a) and (b), 9b (c) and (d), 10b p-type (e) and (f), n-type (g) and (h). | |
Compounds 8a–b and 9a–b showed a hole mobility of up to 1.50 cm2 V−1 s−1 with an on/off current ratio of 106 and a minimum threshold voltage of −4 V. The better OFET properties of the related compounds are due to the planar IQ part of the molecule in the system enhancing the intermolecular π–π interactions to increase the molecular ordering in the thin film. It was found that the ICT interactions between IQ and TAA in a three-dimensional π-stacking network gave rise to well-ordered film morphology and reduced band gap.33 Besides, the hexyl chain attached to the IQ part induces better non-covalent interactions in the active layer. Notably, aliphatic side chains in the IQ unit do not hamper charge carrier transport, while providing largely increased solubility and mobility.62 Even though 8a–b and 9a–b have comparable HOMO energy levels (−3.16 eV), devices based on 9a–b with an ethynyl spacer manifested a better hole-transporting performance than those based on 8a–b, which may be related to their high planarity and rigidity and the extended π-conjugation of the ethynyl spacer, resulting in improved mobility.64 SEM and XRD morphological studies supported the results with the maximum surface coverage and long-range molecular ordering over the substrate. Also, compound 9a with an ethynyl spacer showed the highest performance and mobility of 1.50 cm2 V−1 s−1 with a threshold voltage of −10 V. Although the tert-butylphenyl groups may extend the conjugation, the steric interactions of the alkyl and tert-butyl groups tend to reduce the performance. Compound 8a has an almost similar backbone to 9a, differing only in the spacer, but displays significant variation in structural, morphological, and electrical characteristics. SEM, XRD, and FMO orbital analysis seek to explain the origin of the self-assembly properties in these compounds and characterize the electronic distribution in the molecular orbital in the context of the slight structural alteration they present.
By replacing the bridge moieties between IQ and TAA with the electron-deficient TCBD, compounds 10a–b showed ambipolar transport with enhanced n-channel semiconductivity and reduced p-channel character. TCBD forms charge transfer (CT) complexes with other organic systems, which exhibit technologically interesting magnetic and electrical conductivities.53 The low-lying LUMO (−4.2 eV) levels allowed easy electron injection and stable electron transfer under ambient conditions. Compound 10a–b showed ambipolar semiconductivity with the highest electron mobility of 2.1 cm2 V−1 s−1 and hole mobility of 0.35 cm2 V−1 s−1 with an on/off current ratio of 105. The increased n-channel mobility or reduced p-channel behavior is due to the presence of electronegative cyano groups, which reduce the LUMO levels and energy barrier for electron injection and increase the energy barrier for hole injection from metal electrodes. Also, these molecules exhibited good charge carrier mobility in air, which may be assisted by the CN groups. For instance Tsukamoto62 has reported that tetracyanobutadiene incorporated-quinoidal derivatives exhibit only n-channel performance with the highest mobility of 5.5 × 10−2 cm2 V−1 s−1 with air stability. Besides, the observed lowest hopping distances (3.817 Å) from computational studies and low Eg support the high performance.
Conclusion
Many efforts have been dedicated to develop new types of D–A molecules showing ambipolar transistor characteristics. Fused donor–acceptor IQ units have been chosen as weak acceptor units and functionalized TAA as a donor to construct ambipolar transistors, though p-channel characteristics have dominated more. Low-lying LUMO levels are necessary to manifest superior ambipolar characteristics. We incorporated the TCBD moiety between the electron-rich TAA and donor–acceptor IQ units to attain low-lying LUMO levels. A series of TAA–IQ, TAA–π–IQ, and TAA–TCBD–IQ molecules were designed and synthesized via Suzuki, Sonogashira, and adduct formation reactions in moderate to good yields. The absorption–emission spectra and cyclic voltammetric curves reveal the presence of strong intramolecular charge transfer from electron-donating TAA to indoloquinoxaline or the TCBD moiety. The low-lying LUMO level of ∼−3.9 eV for the compounds enabled ambipolar transport and reduced the energy levels for charge injection. The BGTC devices of compounds with TCBD bridges demonstrated ambipolar semiconductivity with an n-channel mobility of 2.1 cm2 V−1 s−1 and an on/off ratio of 106 and p-channel behavior with a mobility of 0.35 cm2 V−1 s−1 and an on/off current ratio of 105. All other compounds without TCBD substitution exhibited the highest hole mobility of 1.50 cm2 V−1 s−1 with an on/off current ratio of 105. SEM, XRD, and DFT studies reveal field-effect mobility through uniform surface morphology and molecular orbital charge distribution.
Conflicts of interest
There are no conflicts to declare.
Acknowledgements
The authors acknowledge the Central University of Tamil Nadu for the research facilities.
References
- L. Luo, W. Huang, C. Yang, J. Zhang and Q. Zhang, Recent advances on π-conjugated polymers as active elements in high performance organic field-effect transistors, Front. Phys., 2021, 16, 1–32 Search PubMed
.
- M. Muccini, A bright future for organic field-effect transistors, Nat. Mater., 2006, 5, 605–613 CrossRef CAS PubMed
.
- B. Raj, P. Kaur, P. Kumar and S. S. Gill, Comparative Analysis of OFETs Materials and Devices for Sensor Applications, Silicon, 2021, 1–9 Search PubMed
.
-
N. Gay and W.-J. Fischer, in Polytronic 2007-6th International Conference on Polymers and Adhesives in Microelectronics and Photonics, IEEE, 2007, 143–148.
- M. E. Harb, S. Ebrahim, M. Soliman and M. Shabana, Fabrication of organic transistors using nanomaterials for sensing applications, J. Electron. Mater., 2018, 47, 353–358 CrossRef CAS
.
-
S. Shen, L. Sun, R. Wang and L. Gu, 2016 3rd International Conference on Information Science and Control Engineering (ICISCE), 2016, 1413–1416.
- H. Matsui, Y. Takeda and S. Tokito, Flexible and printed organic transistors: From materials to integrated circuits, Org. Electron., 2019, 75, 105432 CrossRef CAS
.
- B. Balambiga, P. Devibala, P. M. Imran, N. S. P. Bhuvanesh and S. Nagarajan, High Mobility and ON/OFF Ratio of Solution-Processable p-Channel OFETs from Arylacetylene End-Capped Alkoxyphenanthrenes, ChemPhysChem, 2022, 23, e202200350 CrossRef CAS PubMed
.
- P. Devibala, P. M. Imran, N. S. P. Bhuvanesh and S. Nagarajan, Influence of Tetraphenylbenzene on the OFET Behavior of Triarylamines, Adv. Electron. Mater., 2022, 8, 2200484 CrossRef CAS
.
- K. Takimiya, T. Yamamoto, H. Ebata and T. Izawa, Design strategy for air-stable organic semiconductors applicable to high-performance field-effect transistors, Sci. Technol. Adv. Mater., 2007, 8, 273 CrossRef CAS
.
- Y.-S. Guan, J. Qiao, Y. Liang, H. K. Bisoyi, C. Wang, W. Xu, D. Zhu and Q. Li, A high mobility air-stable n-type organic small molecule semiconductor with high UV–visible-to-NIR photoresponse, Light: Sci. Appl., 2022, 11, 1–7 CrossRef
.
- C. Wang, Y. Qin, Y. Sun, Y.-S. Guan, W. Xu and D. Zhu, Thiophene-diketopyrrolopyrrole-based quinoidal small molecules as solution-processable and air-stable organic semiconductors: tuning of the length and branching position of the alkyl side chain toward a high-performance n-channel organic field-effect tran, ACS Appl. Mater. Interfaces, 2015, 7, 15978–15987 CrossRef CAS
.
- F. Wu, Y. Liu, J. Zhang, S. Duan, D. Ji and H. Yang, Recent advances in high-mobility and high-stretchability organic field-effect transistors: from materials, devices to applications, Small Methods, 2021, 5, 2100676 CrossRef CAS
.
- Z. Cai, Y. Guo, S. Yang, Q. Peng, H. Luo, Z. Liu, G. Zhang, Y. Liu and D. Zhang, New donor–acceptor–donor molecules with Pechmann dye as the core moiety for solution-processed good-performance organic field-effect transistors, Chem. Mater., 2013, 25, 471–478 CrossRef CAS
.
- J. Chen, J. Yang, Y. Guo and Y. Liu, Acceptor Modulation Strategies for Improving the Electron Transport in High-Performance Organic Field-Effect Transistors, Adv. Mater., 2022, 34, 2104325 CrossRef CAS
.
- Y. Lei, P. Deng, J. Li, M. Lin, F. Zhu, T.-W. Ng, C.-S. Lee and B. S. Ong, Solution-processed donor-acceptor polymer nanowire network semiconductors for high-performance field-effect transistors, Sci. Rep., 2016, 6, 1–9 CrossRef
.
- J. T. E. Quinn, J. Zhu, X. Li, J. Wang and Y. Li, Recent progress in the development of n-type organic semiconductors for organic field effect transistors, J. Mater. Chem. C, 2017, 5, 8654–8681 RSC
.
- B. Balambiga, R. Dheepika, P. Devibala, P. M. Imran and S. Nagarajan, Picene and PTCDI based solution processable ambipolar OFETs, Sci. Rep., 2020, 10, 1–13 CrossRef PubMed
.
- A. Anjali, P. M. Imran, N. S. P. Bhuvanesh and S. Nagarajan, Influence of π-Endcaps on the Performance of Functionalized Quinolines for p-Channel OFETs, Macromol. Rapid Commun., 2022, 43, 2100472 CrossRef CAS PubMed
.
- N. M. Randell and T. L. Kelly, Recent advances in isoindigo-inspired organic semiconductors, Chem. Rec., 2019, 19, 973–988 CrossRef CAS PubMed
.
- J. Zhang, W. Xu, P. Sheng, G. Zhao and D. Zhu, Organic donor–acceptor complexes as novel organic semiconductors, Acc. Chem. Res., 2017, 50, 1654–1662 CrossRef CAS PubMed
.
- A.-J. Payne, J. S. J. McCahill and G. C. Welch, Indoloquinoxaline as a terminal building block for the construction of π-conjugated small molecules relevant to organic electronics, Dyes Pigm., 2015, 123, 139–146 CrossRef CAS
.
- R. Mondal, N. Miyaki, H. A. Becerril, J. E. Norton, J. Parmer, A. C. Mayer, M. L. Tang, J.-L. Bredas, M. D. McGehee and Z. Bao, Synthesis of acenaphthyl and phenanthrene based fused-aromatic thienopyrazine co-polymers for photovoltaic and thin film transistor applications, Chem. Mater., 2009, 21, 3618–3628 CrossRef CAS
.
- J. A. Letizia, M. R. Salata, C. M. Tribout, A. Facchetti, M. A. Ratner and T. J. Marks, n-Channel polymers by design: optimizing the interplay of solubilizing substituents, crystal packing, and field-effect transistor characteristics in polymeric bithiophene-imide semiconductors, J. Am. Chem. Soc., 2008, 130, 9679–9694 CrossRef CAS
.
- M. L. Tang, A. D. Reichardt, N. Miyaki, R. M. Stoltenberg and Z. Bao, Ambipolar, High Performance, Acene-Based Organic Thin Film Transistors, J. Am. Chem. Soc., 2008, 130, 6064–6065 CrossRef CAS
.
- H. Xiao, Y. Zhang, W. Zhang, S. Li and R. Xu, Two novel dyes containing spirobifluorene and triphenylamine: Synthesis, one- and two-photon excited fluorescence and applications as probes for silver ions, water and cell imaging, Sens. Actuators, B, 2016, 233, 469–475 CrossRef CAS
.
- X. Qian, H.-H. Gao, Y.-Z. Zhu, L. Lu and J.-Y. Zheng, 6H-Indolo [2,3-b] quinoxaline-based organic dyes containing different electron-rich conjugated linkers for highly efficient dye-sensitized solar cells, J. Power Sources, 2015, 280, 573–580 CrossRef CAS
.
- D. Dong, D. Fang, H. Li, C. Zhu, X. Zhao, J. Li, L. Jin, L. Xie, L. Chen and J. Zhao, C− H Direct Arylated 6H-Indolo [2,3-b] quinoxaline Derivative as a Thickness-Dependent Hole-Injection Layer, Chem. – Asian J., 2017, 12, 920–926 CrossRef CAS
.
- P. S. Singh, P. M. Badani and R. M. Kamble, Impact of the donor substituent on the optoelectrochemical properties of 6 H-indolo [2, 3-b] quinoxaline amine derivatives, New J. Chem., 2019, 43, 19379–19396 RSC
.
- A. Venkateswararao, P. Tyagi, K. R. J. Thomas, P.-W. Chen and K.-C. Ho, Organic dyes containing indolo [2, 3-b] quinoxaline as a donor: synthesis, optical and photovoltaic properties, Tetrahedron, 2014, 70, 6318–6327 CrossRef CAS
.
- S. S. Mahadik, D. R. Garud, R. V. Pinjari and R. M. Kamble, Synthesis, optical, electrochemical and theoretical studies of 2, 3-Di (pyridin-2-yl) quinoxaline amine derivatives as blue-orange emitters for organic electronics, J. Mol. Struct., 2022, 1248, 131541 CrossRef CAS
.
- C. Fan, P. Sun, T. Su and C. Cheng, Host and dopant materials for idealized deep-red organic electrophosphorescence devices, Adv. Mater., 2011, 23, 2981–2985 CrossRef CAS
.
- I. Cho, S. K. Park, B. Kang, J. W. Chung, J. H. Kim, W. S. Yoon, K. Cho and S. Y. Park, Dicyanovinyl-substituted indolo
[3, 2-b] indole derivatives: low-band-gap π-conjugated molecules for a single-component ambipolar organic field-effect transistor, J. Mater. Chem. C, 2016, 4, 9460–9468 RSC
.
- B.-L. Hu, K. Zhang, C. An, W. Pisula and M. Baumgarten, Thiadiazoloquinoxaline-Fused Naphthalenediimides for n-Type Organic Field-Effect Transistors (OFETs), Org. Lett., 2017, 19, 6300–6303 CrossRef CAS PubMed
.
- P. Devibala, B. Balambiga, P. M. Imran, N. S. P. Bhuvanesh and S. Nagarajan, Butterfly-like triarylamines with high hole mobility and on/off ratio in bottom-gated OFETs, Chem. – Eur. J., 2021, 27, 15375–15381 CrossRef CAS PubMed
.
- P. Devibala, R. Dheepika, P. Vadivelu and S. Nagarajan, Synthesis of Aroylbenzoate-Based Push-Pull Molecules for OFET Applications, ChemistrySelect, 2019, 4, 2339–2346 CrossRef CAS
.
- J. Wang, K. Liu, L. Ma and X. Zhan, Triarylamine: versatile platform for organic, dye-sensitized, and perovskite solar cells, Chem. Rev., 2016, 116, 14675–14725 CrossRef CAS
.
- B. Balambiga, P. Devibala, D. Harshini, P. K. M. Imran and S. Nagarajan, Acetylene bridged alkoxyphenanthrene and triarylamine-based triads for low threshold voltage with high mobility OFETs, Mater. Chem. Front., 2023, 7, 2225–2234 RSC
.
- P. Devibala, B. Balambiga, P. M. Imran, N. S. P. Bhuvanesh and S. Nagarajan, Synthesis of Anthracene and Pyrene End-capped Triarylamines for p-Channel High-performance OFETs, Eur. J. Org. Chem., 2022, e202200825 CrossRef CAS
.
- K.-H. Tu, Y. Wang, Y. Kiyota, T. Iwahashi, Y. Ouchi, T. Mori and T. Michinobu, A cyano-rich small molecule dopant for organic thermoelectrics, Org. Electron., 2020, 87, 105978 CrossRef CAS
.
- N. Cho, J. Kim, K. Song, J. K. Lee and J. Ko, Synthesis and characterization of push–pull organic semiconductors with various acceptors for solution-processed small molecule organic solar cells, Tetrahedron, 2012, 68, 4029–4036 CrossRef CAS
.
- Y. Patil and R. Misra, Diketopyrrolopyrrole-Based and Tetracyano-Bridged Small Molecules for Bulk Heterojunction Organic Solar Cells, Chem. – Asian J., 2018, 13, 220–229 CrossRef CAS
.
- A. A. Raheem, C. Kumar, R. Shanmugam, P. Murugan and C. Praveen, Molecular engineering of twisted dipolar chromophores for efficiency boosted BHJ solar cells, J. Mater. Chem. C, 2021, 9, 4562–4575 RSC
.
- Y. Yuan, T. Michinobu, J. Oguma, T. Kato and K. Miyake, Attempted Inversion of Semiconducting Features of Platinum Polyyne Polymers: A New Approach for All-Polymer Solar Cells, Macromol. Chem. Phys., 2013, 214, 1465–1472 CrossRef CAS
.
- C. Philippe, M. Coste, Y. Bretonnière, L. Lemiègre, S. Ulrich and Y. Trolez, Quadruple Functionalization of a Tetraphenylethylene Aromatic Scaffold with Ynamides or Tetracyanobutadienes: Synthesis and Optical Properties, Eur. J. Org. Chem., 2022, e202200049 CrossRef CAS
.
-
F. D’Souza, R. Misra, R. Sharma, P. Gautam, J. Shinde, M. Thomas, Y. Jang and A. Alsaleh, Electrochemical Society Meeting Abstracts 237, The Electrochemical Society, Inc., 2020, 919.
- J. Choi, E. S. Kim, J. H. Ko, S. M. Lee, H. J. Kim, Y.-J. Ko and S. U. Son, Hollow and microporous triphenylamine networks post-modified with TCNE for enhanced organocathode performance, Chem. Commun., 2017, 53, 8778–8781 RSC
.
- T. Michinobu, C. Boudon, J. Gisselbrecht, P. Seiler, B. Frank, N. N. P. Moonen, M. Gross and F. Diederich, Donor-Substituted 1, 1, 4, 4-Tetracyanobutadienes (TCBDs): New Chromophores with Efficient Intramolecular Charge-Transfer Interactions by Atom-Economic Synthesis, Chem. – Eur. J., 2006, 12, 1889–1905 CrossRef CAS
.
- C. Teng, X. Yang, C. Yang, H. Tian, S. Li, X. Wang, A. Hagfeldt and L. Sun, Influence of Triple Bonds as π-Spacer Units in Metal-Free Organic Dyes for Dye-Sensitized Solar Cells, J. Phys. Chem. C, 2010, 114, 11305–11313 CrossRef CAS
.
- X. Tang, W. Liu, J. Wu, C.-S. Lee, J. You and P. Wang, Synthesis, crystal structures, and photophysical properties of triphenylamine-based multicyano derivatives, J. Org. Chem., 2010, 75, 7273–7278 CrossRef CAS PubMed
.
- T. Ivan, L. Vacareanu and M. Grigoras, Synthesis and Photophysical Properties of Two Intramolecular Charge-transfer Chromophores obtained by [2+ 2] Cycloaddition of TCNE and TCNQ to an Electron-rich Alkyne, Rev. Chim., 2013, 64, 372–377 CAS
.
- Q. Wang, L. Duan, Q. Tao, W. Peng, J. Chen, H. Tan, R. Yang and W. Zhu, Photovoltaic Small Molecules of TPA (F x BT-T-Cz) 3: Tuning Open-Circuit Voltage over 1.0 V for Their Organic Solar Cells by Increasing Fluorine Substitution, ACS Appl. Mater. Interfaces, 2016, 8, 30320–30327 CrossRef CAS PubMed
.
- M. Kivala, C. Boudon, J. Gisselbrecht, B. Enko, P. Seiler, I. B. Müller, N. Langer, P. D. Jarowski, G. Gescheidt and F. Diederich, Organic Super-Acceptors with Efficient Intramolecular Charge-Transfer Interactions by [2+2] Cycloadditions of TCNE, TCNQ, and F4-TCNQ to Donor-Substituted Cyanoalkynes, Chem. – Eur. J., 2009, 15, 4111–4123 CrossRef CAS
.
- K. R. J. Thomas and P. Tyagi, Synthesis, spectra, and theoretical investigations of the triarylamines based on 6 H-indolo[2,3-b]quinoxaline, J. Org. Chem., 2010, 75, 8100–8111 CrossRef CAS PubMed
.
- C. Bao, M. Kaur and W. S. Kim, Toward a highly selective artificial saliva sensor using printed hybrid field effect transistors, Sens. Actuators, B, 2019, 285, 186–192 CrossRef CAS
.
- Z. Tao, T. Mohammed-Brahim, W. Lei, M. Harnois and E. Jacques, Impact of the post-thermal annealing on OFETs using printed contacts, printed organic gate insulator and evaporated C60 active layer, Solid State Electron., 2018, 150, 51–59 CrossRef CAS
.
- J. W. Jeong, G. Jo, S. Choi, Y. A. Kim, H. Yoon, S.-W. Ryu, J. Jung and M. Chang, Solvent additive-assisted anisotropic assembly and enhanced charge transport of π-conjugated polymer thin films, ACS Appl. Mater. Interfaces, 2018, 10, 18131–18140 CrossRef CAS
.
-
D. Choi, P.-H. Chu, M. McBride and E. Reichmanis, Best practices for reporting organic field effect transistor device performance, 2015, 27, 4167–4168 Search PubMed.
- H. Klauk, Will We See Gigahertz Organic Transistors?, Adv. Electron. Mater., 2018, 4, 1700474 CrossRef
.
- A. F. Paterson, S. Singh, K. J. Fallon, T. Hodsden, Y. Han, B. C. Schroeder, H. Bronstein, M. Heeney, I. McCulloch and T. D. Anthopoulos, Recent Progress in High-Mobility Organic Transistors: A Reality Check, Adv. Mater., 2018, 30, 1801079 CrossRef
.
- H. H. Choi, K. Cho, C. D. Frisbie, H. Sirringhaus and V. Podzorov, Critical assessment of charge mobility extraction in FETs, Nat. Mater., 2018, 17, 2–7 CrossRef CAS PubMed
.
- K. Tsukamoto, K. Takagi, S. Nagano, M. Hara, Y. Ie, K. Osakada and D. Takeuchi, π-Extension of electron-accepting dithiarubicene with a cyano-substituted electron-withdrawing group and application in air-stable n-channel organic field effect transistors, J. Mater. Chem. C, 2019, 7, 12610–12618 RSC
.
- H. S. Ryu, M. J. Kim, M. S. Kang, J. H. Cho and H. Y. Woo, Dicyanodistyrylbenzene-Based Copolymers for Ambipolar Organic Field-Effect Transistors with Well-Balanced Hole and Electron Mobilities, Macromolecules, 2018, 51, 8258–8267 CrossRef CAS
.
- H. Chen, Q. Cui, G. Yu, Y. Guo, J. Huang, M. Zhu, X. Guo and Y. Liu, Synthesis and characterization of novel semiconductors based on thieno [3, 2-b][1] benzothiophene cores and their applications in the organic thin-film transistors, J. Phys. Chem. C, 2011, 115, 23984–23991 CrossRef CAS
.
|
This journal is © The Royal Society of Chemistry and the Centre National de la Recherche Scientifique 2024 |