DOI:
10.1039/D3NJ03511E
(Paper)
New J. Chem., 2024,
48, 367-376
Bacterial outer membrane vesicle-modified metal–organic frameworks for sonodynamic therapy–immunotherapy of breast cancer†
Received
31st July 2023
, Accepted 15th November 2023
First published on 23rd November 2023
Abstract
Triple negative breast cancer seriously threatens the life health of women. Here, we develop a metal–organic framework based a nanoplatform for sonodynamic therapy–immunotherapy of breast cancer, loaded with the sonosensitizer chlorin e6 and modified bacterial outer membrane vesicles (OMVs). Sonodynamic therapy not only produced reactive oxygen species to kill tumor cells directly, but also promoted anti-tumor immunity. More importantly, OMV modification improved tumor targeting and immune activation of the nanoplatform, and further increased dendritic cell maturation and T cell infiltration in the tumor microenvironment. This novel therapeutic strategy led to a notable inhibitory effect on the development of breast cancer in mice.
1. Introduction
Breast cancer is one of the most common female malignancies.1–3 The treatment of breast cancer is receiving increasing attention. However, the invasive growth and recurrence of triple negative breast cancer make it difficult to be surgically eradicated. Moreover, triple negative breast cancer is not sensitive to many chemotherapy drugs.4,5 Although traditional treatments such as surgery and chemotherapy could temporarily inhibit tumor growth, recurrence and drug resistance are still critical barriers to breast cancer treatment.6–8 Therefore, there is a need to develop new therapeutic strategies to enhance breast cancer treatment.
Sonodynamic therapy (SDT) is a treatment method that produces cytotoxic reactive oxygen species (ROS) by irradiating sonosensitizers at a tumor site with low intensity ultrasound.9 Currently, SDT is receiving increasing attention because of its non-invasiveness and high tissue penetration. However, biological barriers of the tumor microenvironment hinder the accumulation of sonosensitizers in the tumor tissue.10–12 Most kinds of sonosensitizers such as porphyrins (hematoporphyrin) and pyrroles (chlorin e6 (Ce6)) have a short in vivo half-life, making it difficult for delivery into the tumor tissue by passive transport.13,14 Therefore, how to improve the non-specific distribution and tumor targeting ability of sonosensitizers remains a key problem to enhance the therapeutic efficiency of SDT.
Nanotechnology offers a promising platform for targeted delivery of sonosensitizers.15 Among them, zeolitic imidazolate framework-8 (ZIF-8), metal–organic framework (MOF) nanoparticles consisting of metal ions combined with organic molecular ligands, has been applied for drug delivery to various kinds of tumors.16,17 ZIF-8 can release loaded drugs by cleavage in the acidic tumor microenvironment.18 Recent studies have shown that parthenogenic anaerobic bacteria are able to selectively colonize tumor tissues due to their targeting of the tumor hypoxic microenvironment.19,20 Bacterial outer membrane vesicles (OMVs) have the same function of hypoxic targeting due to their surface specific receptors. Moreover, the application of bacterial OMVs can avoid the possible bacteraemia caused by direct injection of bacteria.21,22 Importantly, the pathogen-associated molecular pattern of bacterial OMVs can synergistically enhance the immunogenic cell death (ICD) effect of SDT for immunotherapy against tumors.23 Therefore, we designed to modify bacterial OMVs with sonosensitizer-loaded nanoparticles to enhance the therapeutic efficiency of breast cancer depending on the hypoxic targeting and immune activation of the bacterial membrane.
In this study, we constructed a MOF nanoparticle-based platform for the tumor-targeted drug delivery of sonosensitizers. ZIF-8 nanoparticles loaded with the sonosensitizer Ce6 (CMOF) were first prepared. Then, the bacterial OMVs of E. coli MG 1655 were extracted and modified on the surface of the CMOF to obtain an OCMOF. We evaluated the tumor cell endocytosis and cytotoxicity of the OCMOF using cellular assays. The mice subcutaneous transplantation breast cancer model was used to evaluate the in vivo tumor targeting and therapeutic efficacy of the OCMOF. The immune activation effect of SDT in the tumor microenvironment was also validated (Fig. 1). We hope that the developed OMV-modified drug delivery nanoplatform can be used for a series of subsequent SDT therapeutic applications.
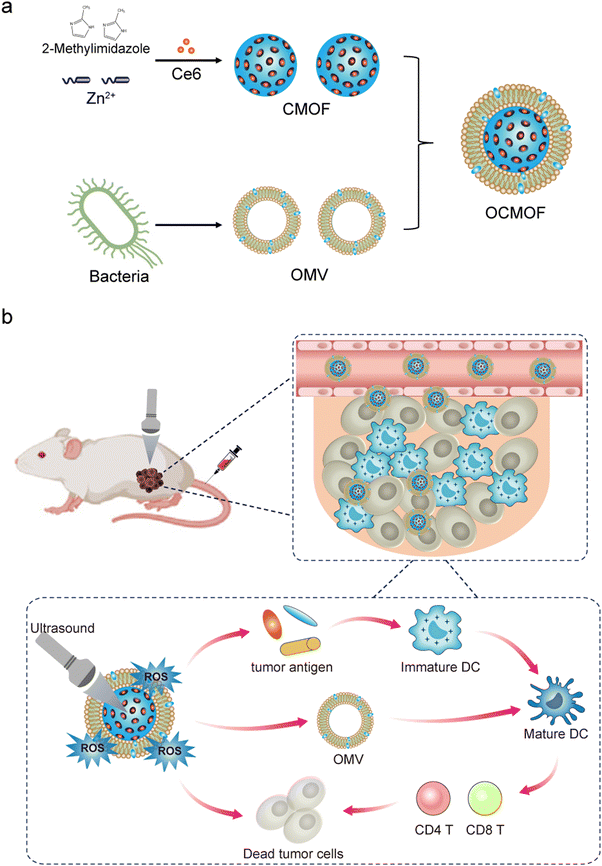 |
| Fig. 1 Schematic illustration of the preparation of OMV-modified Ce6-loaded MOF (OCMOF) nanoparticles (a) and their applications in sonodynamic therapy and immunotherapy of breast cancer (b). | |
2. Methods
2.1. Bacterial culture and OMV acquisition
The monoclonal colonies of E. coli MG 1655 on LB solid culture plates were inoculated into culture tubes. After overnight incubation at 37 °C, the bacterial fluid was collected when the OD600 value was 1. The bacteria were removed by centrifugation with 5000 rpm and the remaining medium was filtered through the 0.45 μm filter. The medium was filtered using a 100 KDa ultrafiltration tube to concentrate the OMV. The OMV was resuspended using PBS and the protein concentration of the OMV was measured using the BCA assay.
2.2. Preparation of nanoparticles
162 mg of 2-methylimidazole, 73.32 mg of zinc nitrate and 2 mg of Ce6 were dissolved in methanol. The nanoparticles were stirred for 30 min at room temperature to form Ce6-loaded MOF (CMOF) nanoparticles. The CMOF nanoparticles were resuspended in ultrapure water and mixed with the OMV protein of E. coli MG 1655. A squeezer with a 100 nm filter membrane was used to squeeze the mixed solution (10 round trips) to obtain bacterial OMV-modified Ce6-loaded MOF (OCMOF) nanoparticles.
2.3. Characterization of nanoparticles
The zeta potential and particle size of OCMOF and CMOF nanoparticles were analysed by DLS. The morphology of OCMOF and CMOF nanoparticles was observed by TEM. The successful preparation of OCMOF and CMOF nanoparticles was evaluated by UV spectroscopy with fluorescence spectroscopy detection. The encapsulation efficiency of the OCMOF was tested through dialysis. Particle size changes of OCMOF and CMOF nanoparticles were detected for consecutive seven days to assess the aqueous phase stability of the nanoparticles. The OCMOF and CMOF nanoparticles (50 μg mL−1) were added to the 96-well plate with the ROS probe DPBF (5 mM). The wells were irradiated with low-intensity focused ultrasound (1 MHz, 1.5 W cm−2, 35% duty cycle, 10 min). The ROS levels of the solution were detected by ultraviolet spectrograph. All experiments involving Ce6 were conducted in a dark environment to avoid the effects of phototoxicity and photobleaching.
2.4. Cell uptake
Mouse triple-negative breast cancer 4T1 cells were added into 24-well plates (50
000 cells per well). The OCMOF and CMOF nanoparticles (Ce6 concentrations = 50 μg mL−1) were added to the cell wells, respectively. Fluorescence micrographs were taken at different incubation time points to observe the cellular uptake of nanoparticles. And the cellular uptake efficiency of nanoparticles was quantitatively analyzed by flow cytometry.
2.5.
In vitro anti-tumor cell assay
293T cells were added into 96-well plates (10
000 cells per well). The OCMOF and CMOF nanoparticles with corresponding Ce6 concentrations of 25, 50, 100 and 200 μg mL−1 were added to the cell wells. The 293T cells viability was detected by CCK-8. 4T1 cells were added into 96-well plates (10
000 cells per well). The OCMOF and CMOF nanoparticles (50 μg mL−1) were added to the cell wells. After 6 h of incubation, the cell well plates were irradiated with low-intensity focused ultrasound (1 MHz, 1.5 W cm−2, 35% duty cycle, 10 min), and the ROS detection probe DCFH-DA (10 μM) was added to the wells for 30 min incubation. The ROS levels in the cells were observed by fluorescence microscopy after washing with PBS three times. Flow cytometry was used to analyze the quantitative results of intracellular ROS generation. After the same treatment measures as above, cells were stained with a mixture of Calcein and PI solution for 30 min and the ratio of live and dead cells was observed by fluorescence microscopy. Meanwhile, the CCK-8 assay was used to quantify the activity of breast cancer cells after SDT treatment.
2.6.
In vitro DC maturation assay
The ICD marker (calreticulin, CRT) was used to detect the ICD effect. 4T1 cells were added into 24-well plates (50
000 cells per well). The tumor cells were treated as above and washed with PBS. The CRT antibody was added and incubated overnight. The FITC-labeled secondary antibody was added and incubated for 1 h. The CRT expression of 4T1 cells was observed by fluorescence microscopy. Mouse DC 2.4 cells in 24-well plates (50
000 cells per well) were collected. The tumor cell medium was collected after above treatment and added to the DC wells. After 48 hours of incubation, treated DCs were collected to treat with flow cytometry antibodies against CD80 and CD86 for 30 min, and then DC maturation was quantified by flow cytometry.
2.7. Animal experiments
Female BALB/c mice (4–6 weeks) used in this study were purchased from Zhejiang Vital River Laboratory Animal Technology Company. 2 × 106 breast cancer 4T1 cells were injected under skin on the right back of the mice to establish tumor-bearing mice models. Seven days after tumor cell injection, the tumor-bearing mice were used for in vivo experiments. Isoflurane gas was used to maintain anesthesia of mice during the experiment. After completing the in vivo treatment experiments, all mice were euthanized. All animal experiments in this study were approved by the animal ethics committee of the Zhejiang University School of Medicine.
2.8. Tumor targeting assay
The tumor-bearing mice were randomly divided into two groups: OCMOF and CMOF (n = 3). The corresponding nanoparticles were injected into the tumor-bearing mice via the tail vein (5 mg kg−1). The distribution of nanoparticles in the tumor-bearing mice was continuously observed by the in vivo imaging system after nanoparticle injection.
2.9.
In vivo anti-tumor assay
The tumor-bearing mice were randomly divided into 6 groups (n = 5): (1) control, (2) US, (3) CMOF, (4) OCMOF, (5) US + CMOF, and (6) US + OCMOF. In the control and US group, PBS was injected into the mice via the tail vein. In the other group, the CMOF or OCMOF (5 mg kg−1) was injected into the mice. Mice tumors were irradiated by focused ultrasound at the 24th hour after nanoparticle injection. The ultrasound parameters were as follows: 1 MHz, 1.5 W cm−2, 35% Duty cycle, and 10 min of irradiation time. The tumor volume and mice body weight changes were measured every two days after treatment.
The survival time was recorded when the mice died naturally or when the mice tumor volume was greater than 2000 mm3. Mice tumors and lungs were collected for weighing and recorded the lung metastases on the fifteenth day after treatment.
2.10. Exploration of immune mechanisms
To investigate the anti-tumor immune response of SDT, post-treatment mice tumors were collected and prepared into single cell suspensions. The single cell suspensions were labeled with the corresponding flow antibodies: CD45, CD3, CD4, CD8, CD11c, CD80, and CD86 and then analyzed by flow cytometry. IFN-γ, TNF-α and IL-2 ELISA kits were used to analyze the cytokine content in mouse tumor tissues. The rechallenged tumor models are used to evaluate the long-term anti-tumor immune response. The mice were divided into three groups (n = 5): (1) control group, (2) US + CMOF group, and (3) US + OCMOF group. The control group was new and untreated mice that received 4T1 cells (1 × 106) injected into the skin. In the other two groups, 4T1 cells were replanted 15 days after the corresponding treatment. The mice tumor volume was recorded to evaluate the antitumor efficiency.
2.11. Safety assessment
The heart, liver, spleen, lung and kidney of treated mice were collected and H&E sections were stained to assess the biotoxicity of SDT treatment. Blood of treated mice was collected, and the effect on the liver and kidney functions was assessed using blood biochemical assays. The blood routine was detected and the serum CRP level was analyzed using the ELISA assay.
2.12. Statistical analysis
Data are presented as mean ± S.D. The statistical difference between the different groups was calculated by one-way analysis of variance (ANOVA). SPSS 26.0 was used for statistical analysis. P values < 0.05 were considered as significant (***P < 0.001; **P < 0.01; *P < 0.05).
3. Results and discussion
3.1. Preparation and characterization of the OCMOF
In this study, the tumor-targeting nanoplatform was prepared by modifying the OMVs of E. coli MG1655 on the photosensitizer Ce6-loaded MOF nanoparticles. The synthetic route of the nanoplatform is shown in Fig. 1a. The DLS results showed that the hydrodynamic diameters of the CMOF and OCMOF were 111.33 ± 4.73nm and 133.67 ± 7.64 nm (Fig. 2a). The zeta potential of the CMOF is −13.63 ± 2.52 mV. After modifying with the OMV, the zeta potential of the OCMOF had been changed to −9.61 ± 1.85 mV (Fig. 2b). Transmission electron microscopy (TEM) images further showed the polygonal structures of the CMOF and OCMOF (Fig. 2c). The ultraviolet spectrum results showed that the CMOF and OCMOF have characteristic peaks at 410 nm and 660 nm similar to that of free Ce6 (Fig. 2d). Under an excitation of 410 nm, the CMOF and OCMOF both showed emission peaks at 650–670 nm (Fig. 2e). The results of both the ultraviolet spectrum and the fluorescence spectrum indicated that Ce6 was successfully loaded into the nanoparticles. Furthermore, the encapsulation efficiency of the OCMOF was 76.74 ± 6.72% (Fig. S1, ESI†). SDS-PAGE was used to test the protein molecules modified on the MOF nanoparticles. We found that the OCMOF group showed similar protein bands to the OMV group and there is no protein band in the CMOF group, which confirmed that bacterial OMVs had been successfully modified onto the nanoparticles (Fig. S2, ESI†). We also found that the size of the CMOF and OCMOF in PBS does not change significantly for 7 days (Fig. 2f). The ROS generated by the CMOF and OCMOF upon ultrasound irradiation was detected by DPBF. If the solution contains ROS, the absorbance of DPBF will decrease. The UV absorption spectrum results showed that the absorbance of the US + CMOF group and US + OCMOF group decreased significantly compared with those of the control group, the US group, the CMOF group and the OCMOF group (Fig. S3, ESI†).
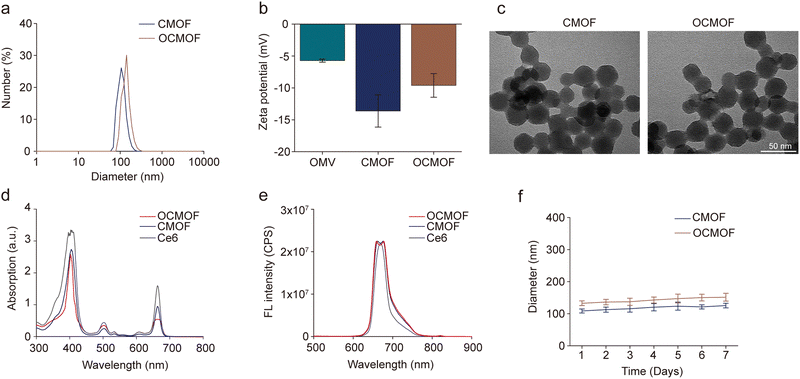 |
| Fig. 2 (a) Diameter distributions of the CMOF and OCMOF. (b) Zeta potentials of the OMV, CMOF and OCMOF. (c) TEM images of the CMOF and OCMOF. Bar = 50 nm. (d) Ultraviolet spectra of the OCMOF, CMOF and Ce6. (e) Fluorescence spectra of the OCMOF, CMOF and Ce6. (f) Seven-day particle size changes of the CMOF and OCMOF. | |
3.2. Cellular uptake, ROS generation and in vitro cytotoxicity of the OCMOF
In this study, bacterial outer membrane proteins were camouflaged on the surface of nanoparticles, hoping to enable nanoparticles to inherit the corresponding functions of the host bacteria. The function of the bacterial outer membrane can enhance the tumor targeting aggregation of nanoparticles. The CMOF and OCMOF were added to co-incubate with mouse triple-negative breast cancer 4T1 cells to assay their tumor cell uptake efficiency. Fluorescence microscopy results showed that the OCMOF could enter more into the cytoplasm of breast cancer cells compared to the CMOF during the 4 h of incubation (Fig. 3a). Flow cytometry was used to quantify the cellular uptake efficiency of the nanoparticles. The results showed that the cellular uptake of the OCMOF group was significantly higher than that of the CMOF group at each incubation time point (Fig. 3b). Importantly, the biggest difference was at the 4 hour time point, the OCMOF group (78.37 ± 4.78%) was 1.96 times as large as that of the CMOF group (39.87 ± 3.79%) (Fig. 3c). This indicated that modification of the bacterial OMV enhanced the tumor cell uptake of MOF nanoparticles.
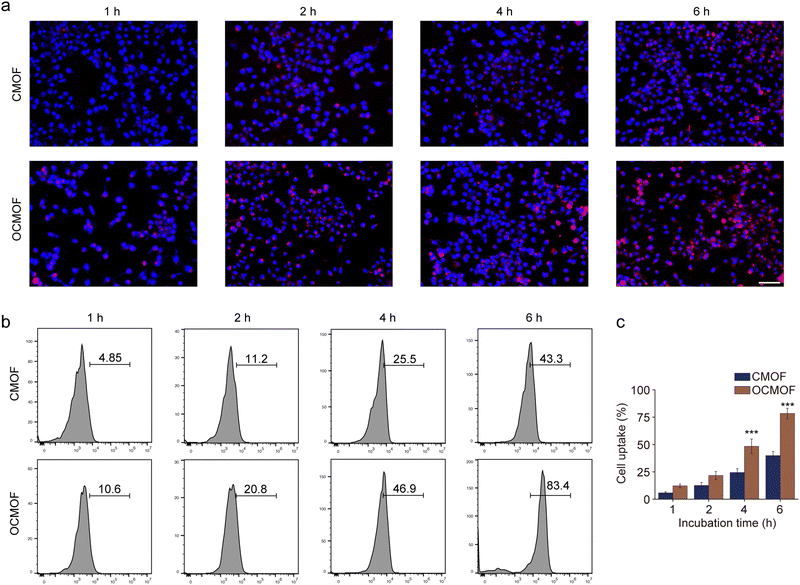 |
| Fig. 3 (a) Fluorescence microscopy images of 4T1 cells incubated with the CMOF or OCMOF at different time points. Bar = 200 μm. (b) Flow cytometry results of 4T1 cells incubated with the CMOF or OCMOF at different time points. (c) Quantitative results of the flow cytometry of 4T1 cells. | |
We first analyzed the cytotoxicity of each concentrations of the OCMOF or CMOF to the 293T cells. The results showed that the OCMOF or CMOF with a Ce6 concentration of 25–200 μg mL−1 both exhibited weak cytotoxicity on normal cells (Fig. S4, ESI†). The ROS probe 2′,7′-dichlorodihydrofluorescein diacetate (DCFH-DA) was used to detect intracellular ROS levels in SDT. As shown in Fig. 4a, only little green fluorescence was present in 4T1 cells after incubation with the OCMOF or CMOF. In contrast, after using focused ultrasound irradiation (1.5 W cm−2), strong green fluorescence was present inside the 4T1 cells in the US + OCMOF group and was much stronger than that in the US + CMOF group. The quantitative results of flow cytometry also showed that the green fluorescence intensity of the US + OCMOF group was significantly higher than that of other treatment groups (Fig. 4b). Moreover, the CCK-8 and live-dead cell staining kits were used to evaluate the cytotoxicity of OCMOF-mediated SDT. The tumor cell viability was significantly inhibited in the OCMOF + US group compared to the control, US, CMOF and OCMOF groups, with a cell survival rate of 39.91 ± 5.25%, which was significantly lower than that of the CMOF + US group (66.94 ± 3.10%). The similar results were found in the live–dead cell staining (Fig. 4c and d). The above results suggested that modification of the bacterial OMV enhanced the ROS generation level and cytotoxicity of SDT on tumor cells by enhancing the tumor cell uptake of the sonosensitizer-loaded MOF.
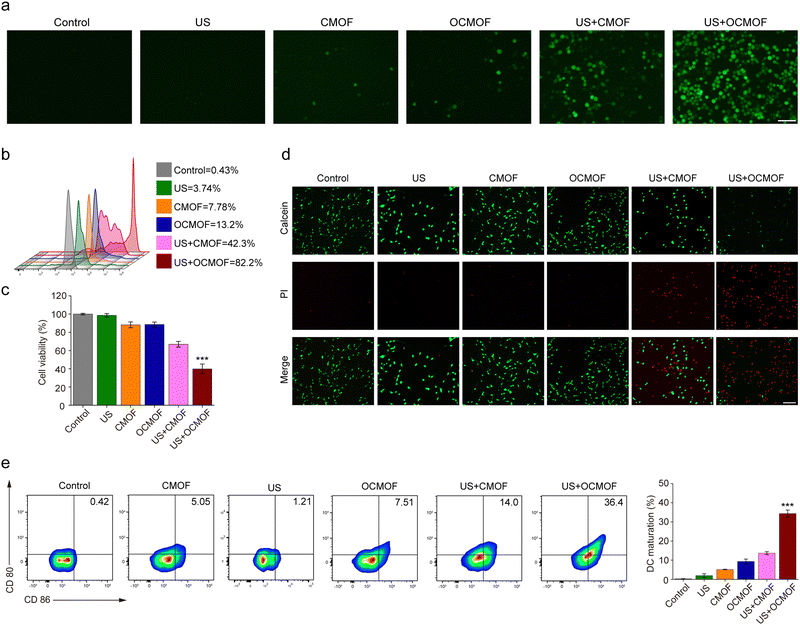 |
| Fig. 4 (a) Fluorescence microscopy images of ROS generation in 4T1 cells after different treatments. Bar = 200 μm. (b) Flow cytometry results of ROS generation in 4T1 cells after different treatments. (c) Cell viability of 4T1 cells after different treatments. (d) Live–dead cell staining of 4T1 cells after different treatments. Bar = 200 μm. (e) Flow cytometry of DC maturation (CD80+ CD86+) after different treatments. | |
Previous findings suggested that SDT-generated ROS could kill tumor cells while also inducing ICD effects, contributing to DC maturation and antitumor immunity activation.
The ICD marker (calreticulin, CRT) was used to detect the ICD effect of SDT in 4T1 breast tumor cells. According to the fluorescence microscopy results, we found that the strong green fluorescence of CRT appeared on the tumor cells treated with the US + MOF and US + OCMOF groups (Fig. S5, ESI†). To verify whether OCMOF-mediated SDT could activate antitumor immunity, the OCMOF and CMOF were incubated with 4T1 cells for 6 h followed by focused ultrasound irradiation for 10 min. Then, the tumor cell medium was added and co-incubated with DCs for 48 h. The flow cytometry results showed that the expression of CD80 and CD80 in DCs in the US + OCMOF group was significantly higher than that of the other treatment groups (Fig. 4e). This suggested that OCMOF-mediated SDT significantly induced 4T1 cells to release tumor-associated antigens to promote DC maturation. Most importantly, the presence of bacterial OMVs gave the OCMOF a stronger immune activation capacity than the CMOF, which may be attributed to the presence of antigens from the bacterial outer membrane.
3.3.
In vivo distribution of the OCMOF
To evaluate the tumor targeting of nanoparticles, the OCMOF or CMOF was intravenously injected into 4T1 tumor-bearing mice and a small animal imaging system was used to monitor the in vivo distribution of nanoparticles at different time points. The in vivo fluorescence imaging results showed that the fluorescence signal in the mice tumor injected with the OCMOF gradually enhanced and reached the peak at 24 h after injection, and was significantly higher than that of the mice injected with the CMOF (Fig. 5a). At 24 h after injection, the fluorescence signal intensity of tumor in the OCMOF group was 1.56 times that in the CMOF group (Fig. 5b). This indicated that the modification of the bacterial outer membrane can effectively increase the nanoparticle enrichment in the tumor.
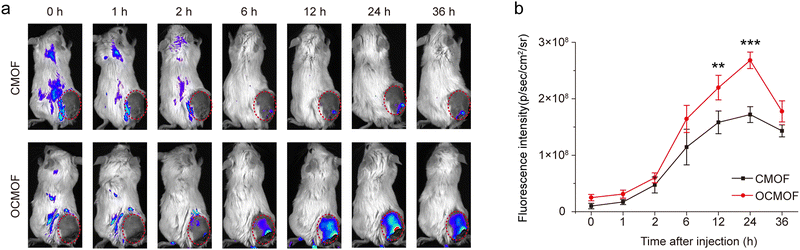 |
| Fig. 5 (a) In vivo fluorescence imaging of 4T1 tumor-bearing mice intravenously injected with the CMOF and OCMOF at different time points (n = 3). (b) Quantitative in vivo distributions of the CMOF and OCMOF in the tumor site at different time points. | |
3.4. OCMOF-mediated SDT for mice breast tumor
Based on the results of previous experiments, mice subcutaneous transplantation breast cancer models were used to evaluate the in vivo antitumor effect of OCMOF-mediated SDT. The OCMOF or CMOF was injected into 4T1 tumor-bearing mice via the tail vein. Focused ultrasound was used to irradiate mice tumor at 24 h after nanoparticle injection to trigger SDT. Tumor volume changes were continuously monitored during treatment (Fig. 6a). As shown in Fig. 6b, compared to the control, US, CMOF, OCMOF and US + CMOF groups, the mice tumor growth in the US + OCMOF group was significantly lower. The average tumor volume of the control group was 1847.50 ± 174.02 mm3, while the average tumor volume of the US + OCMOF group was 157.09 ± 110.31 mm3. The mice tumors were collected for weighing on day 15 after SDT treatment. The tumor weights of the control group, US group, CMOF group, OCMOF group, US + CMOF group and US + OCMOF group were 2.70 ± 0.29 g, 2.55 ± 0.35 g, 2.16 ± 0.18 g, 2.01 ± 0.29 g, 1.65 ± 0.26 g and 0.69 ± 0.17 g, respectively. The tumor weight in the US + OCMOF group was only 0.256 times that of the control group, which indicated that the tumor inhibition rate of US + OCMOF treatment was 74.44% (Fig. 6c). Importantly, the treatment of US + OCMOF remarkably prolonged the survival time of tumor-bearing mice in comparison with other groups (Fig. 6d). US + OCMOF and US + CMOF treatment would not negatively affect the weight of the mice (Fig. S6, ESI†). On the 15th day after treatment, we detected the lung metastases of tumor-bearing mice in different treatment groups. The number of lung metastasis and the lung weight in the US + OCMOF group were significantly lower than other groups, indicating that US + OCMOF treatment can inhibit the lung metastases of triple-negative breast tumor (Fig. S7, ESI†). The results indicated that the OCMOF-mediated SDT treatment was more efficient than the other treatments, which possibly due to more enrichment of sonosensitizers in the tumor site as well as stronger immune responses.
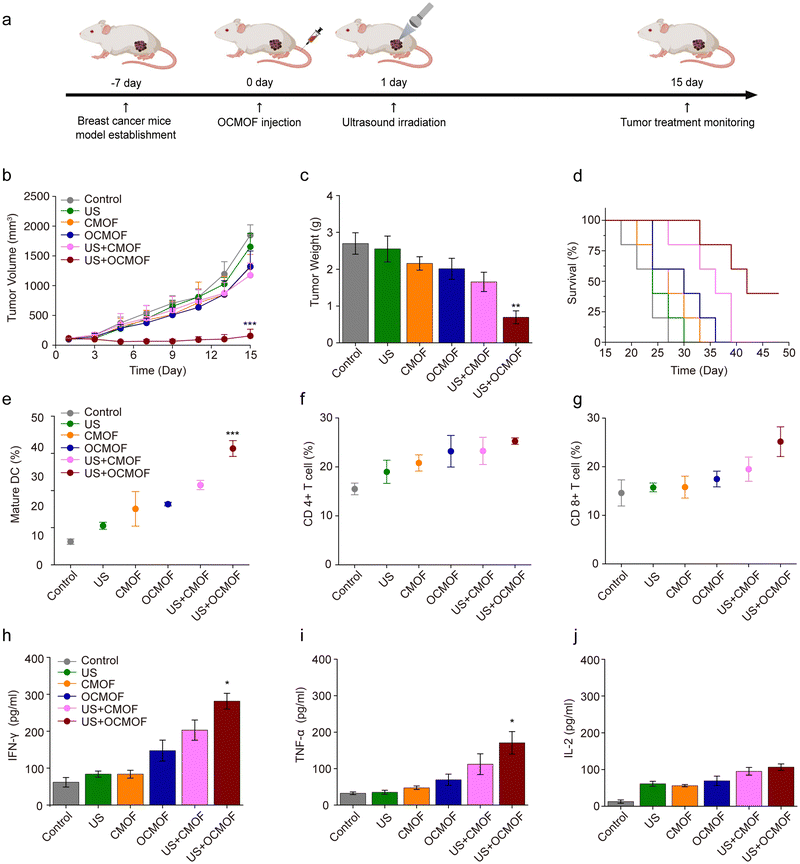 |
| Fig. 6 (a) Schematic diagram of experimental procedures for breast tumor-bearing mice. (b) Tumor volume of mice that received different treatments (n = 5). (c) Tumor weight of mice that received different treatments. (d) Survival time of tumor-bearing mice in each treatment group. (e) Quantification of the mature DC of mice tumor in each group. (f) Quantification of CD4+ T cells of mice tumor in each group. (g) Quantification of CD8+ T cells of mice tumor in each group. (h)–(j) Levels of IFN-γ, TNF-α and IL-2 of mice tumor in each group. | |
Next, we further evaluated OCMOF-mediated SDT-induced antitumor immune responses. Mice tumors were collected on day 7 after SDT treatment for the detection of intratumoral immune cells and cytokine levels. Flow cytometry results showed that the proportion of mature DCs in the tumors of mice treated with US + OCMOF was significantly higher than that of other treatment groups (Fig. 6e). Also, the DC maturation increased the infiltration of CD4+ T cells and CD8+ T cells in the tumor, which facilitated the anti-tumor immune response (Fig. 6f and g). In addition, ELISA results revealed that the levels of IFN-γ and TNF-α were significantly increased in the mice tumors of the US + OCMOF group, while IL-2 levels were slightly increased (Fig. 6h–j). Furthermore, the rechallenged tumor model treatment results showed that the rechallenged tumor growth was slow at the beginning of tumor cell replanting in the US + OCMOF group, but after two weeks, the mice tumor still grew to a similar size as the control group and US + CMOF group (Fig. S8, ESI†). The results suggested that US + OCMOF therapy could induce the strong but not long-term antitumor immune response, which may be because the exogenous protein stimulation of OMVs cannot exist in the mouse body for a long time. The in vivo tumor treatment results suggested that, due to the immunogenicity of bacterial OMVs, OCMOF-mediated SDT activated DCs in tumor, which led to CD4+ T cell and CD8+ T cell infiltration and secretion of pro-inflammatory cytokines. The strong immune response is also one of the important mechanisms of OCMOF-mediated SDT against tumors.
3.5. Security assessment of the OCMOF
The in vivo safety of the OCMOF was evaluated after performing tumor treatment in mice. As shown in (Fig. S9a, ESI†), after OCMOF or CMOF-mediated SDT, no significant pathological changes were observed in the H&E-stained sections of the heart, liver, spleen, lung and kidney of the mice in each treatment group. Meanwhile, the blood biochemical results showed that liver and kidney functional parameters, including ALT, AST, BUN and Cre, were at normal ranges after SDT (Fig. S9b–e, ESI†). The blood routine and serum CRP levels showed that all indexes of the US + OCMOF group were not significantly different from those of other groups (Fig. S10, ESI†). This demonstrated that the OMV did not cause obvious inflammatory response in mice at 15 days after treatment. The above results indicated that OCMOF-mediated SDT had good biosafety and did not cause significant toxic side effects to mice.
4. Conclusions
The results showed that the developed OCMOF nanoplatform had efficient tumor targeting ability to delivery sonosensitizers due to bacterial OMV modification. More importantly, the nanoplatform can lead to a series of immune responses. First, the bacterial OMVs on the surface of the nanoplatform can activate immune cells in tumor tissues and facilitate anti-tumor immunity. Then, in addition to directly killing tumor cells, the enhanced SDT can also improve the release of tumor-associated antigens from apoptotic tumor cells via ICD, induce DC maturation, and further increase CD4+ and CD8+ T cell infiltration within the tumor. This strategy of SDT combined with immunotherapy is expected to enhance the treatment of triple negative breast cancer.
In summary, we prepared bacterial OMV-modified sonosensitizer-loaded MOF nanoparticles as a multifunctional therapeutic platform to precisely target tumor and modulate the tumor immune microenvironment for enhanced combined SDT-immunotherapy of breast cancer. Compared with the other reported drug-loaded MOF nanoparticles, the novel nanoplatform prepared in this study has advantages in tumor targeting and immunomodulation.24 The bacterial membrane coated on the surface of the nanoplatform enables tumor-targeted drug delivery. Moreover, the immunogenicity of the bacterial OMVs not only stimulated the tumor immune microenvironment, but also synergistically enhanced the SDT-induced ICD effect, and further promoted post-treatment anti-tumor immunity. The therapeutic strategy first killed primary tumor cells directly by SDT, and then achieved longer-term tumor suppression through activated anti-tumor immunity. Based on the good biosafety, our OMV-modified nanoplatform may show greater potential for clinical translation than bacterial-mediated tumor therapy. It could mediate the synergistic treatment of SDT and immunotherapy, providing efficient therapeutic efficiency in triple negative breast cancer and even other types of tumors.
Ethical statement
All experiments in our study were performed in compliance with relevant laws or guidelines of China. In addition, all experiments followed institutional guidelines of the Zhejiang University School of Medicine. All animal experiments were approved by the animal ethics committee of the Zhejiang University School of Medicine.
Conflicts of interest
None of the authors has any potential conflict of interest related to the work described in the manuscript.
References
- N. Harbeck and M. Gnant, Breast cancer, Lancet, 2017, 389(10074), 1134–1150 CrossRef.
- A. G. Waks and E. P. Winer, Breast Cancer Treatment A Review, JAMA, J. Am. Med. Assoc., 2019, 321(3), 288–300 CrossRef CAS PubMed.
- L. Fan, K. Strasser-Weippl, J. J. Li, J. St Louis, D. M. Finkelstein, K. D. Yu, W. Q. Chen, Z. M. Shao and P. E. Goss, Breast cancer in China, Lancet Oncol., 2014, 15(7), E279–E289 CrossRef.
- S. K. Yeo and J. L. Guan, Breast Cancer: Multiple Subtypes within a Tumor, Trends Cancer, 2017, 3(11), 753–760 CrossRef CAS.
- Y. R. Liang, H. W. Zhang, X. J. Song and Q. F. Yang, Metastatic heterogeneity of breast cancer: Molecular mechanism and potential therapeutic targets, Semin. Cancer Biol., 2020, 60, 14–27 CrossRef CAS PubMed.
- D. Hammerl, M. Smid, A. M. Timmermans, S. Sleijfer, J. W. M. Martens and R. Debets, Breast cancer genomics and immuno-oncological markers to guide immune therapies, Semin. Cancer Biol., 2018, 52, 178–188 CrossRef CAS.
- N. Tray, J. Taff and S. Adams, Therapeutic landscape of metaplastic breast cancer, Cancer Treat. Rev., 2019, 79, 101888 CrossRef CAS.
- N. Jokar, I. Velikyan, H. Ahmadzadehfar, S. J. Rekabpour, E. Jafari, H. H. Ting, H. J. Biersack and M. Assadi, Theranostic Approach in Breast Cancer A Treasured Tailor for Future Oncology, Clin. Nucl. Med., 2021, 46(8), E410–E420 CrossRef PubMed.
- Y. Zhang, X. Q. Zhang, H. C. Yang, L. Yu, Y. Xu, A. Sharma, P. Yin, X. Y. Li, J. S. Kim and Y. Sun, Advanced biotechnology-assisted precise sonodynamic therapy, Chem. Soc. Rev., 2021, 50(20), 11227–11248 RSC.
- S. Son, J. H. Kim, X. W. Wang, C. L. Zhang, S. A. Yoon, J. Shin, A. Sharma, M. H. Lee, L. Cheng, J. S. Wu and J. S. Kim, Multifunctional sonosensitizers in sonodynamic cancer therapy, Chem. Soc. Rev., 2020, 49(11), 3244–3261 RSC.
- X. Q. Zhou, P. Y. Wang, V. Ramu, L. Y. Zhang, S. H. Jiang, X. Z. Li, S. Abyar, P. Papadopoulou, Y. Shao, L. Bretin, M. A. Siegler, F. Buda, A. Kros, J. L. Fan, X. J. Peng, W. Sun and S. Bonnet, In vivo metallophilic self-assembly of a light-activated anticancer drug, Nat. Chem., 2023, 15(7), 980–987 CrossRef CAS.
- G. L. He, M. M. He, R. Wang, X. Z. Li, H. Z. Hu, D. S. Wang, Z. Q. Wang, Y. Lu, N. Xu, J. J. Du, J. L. Fan, X. J. Peng, W. Sun and A. Near-Infrared, Light-Activated Photocage Based on a Ruthenium Complex for Cancer Phototherapy, Angew. Chem., Int. Ed., 2023, 62(24), 202218768 CrossRef PubMed.
- Q. L. Guo, X. L. Dai, M. Y. Yin, H. W. Cheng, H. S. Qian, H. Wang, D. M. Zhu and X. W. Wang, Nanosensitizers for sonodynamic therapy for glioblastoma multiforme: current progress and future perspectives, Mil. Med. Res., 2022, 9(1), 26 CAS.
- T. T. Wu, Y. Liu, Y. Cao and Z. H. Liu, Engineering Macrophage Exosome Disguised Biodegradable Nanoplatform for Enhanced Sonodynamic Therapy of Glioblastoma, Adv. Mater., 2022, 34(15), e2110364 CrossRef.
- Z. H. Su, D. M. Xi, Y. C. Chen, R. Wang, X. L. Zeng, T. Xiong, X. Xia, X. Rong, T. Liu, W. K. Liu, J. J. Du, J. L. Fan, X. J. Peng and W. Sun, Carrier-Free ATP-Activated Nanoparticles for Combined Photodynamic Therapy and Chemotherapy under Near-Infrared Light, Small, 2023, 19(11), 2205825 CrossRef CAS PubMed.
- B. B. Ding, H. Chen, J. Tan, Q. Meng, P. Zheng, P. A. Ma and J. Lin, ZIF-8 Nanoparticles Evoke Pyroptosis for High-Efficiency Cancer Immunotherapy, Angew. Chem., Int. Ed., 2023, 62(10), e202215307 CrossRef CAS PubMed.
- C. S. Li, J. Ye, X. Yang, S. Liu, Z. Y. Zhang, J. Wang, K. F. Zhang, J. T. Xu, Y. J. Fu and P. P. Yang, Fe/Mn Bimetal-Doped ZIF-8-Coated Luminescent Nanoparticles with Up/Downconversion Dual-Mode Emission for Tumor Self-Enhanced NIR-II Imaging and Catalytic Therapy, ACS Nano, 2022, 16(11), 18143–18156 CrossRef CAS.
- M. R. Xu, Y. Hu, W. P. Ding, F. F. Li, J. Lin, M. Wu, J. J. Wu, L. P. Wen, B. S. Qiu, P. F. Wei and P. Li, Rationally designed rapamycin-encapsulated ZIF-8 nanosystem for overcoming chemotherapy resistance, Biomaterials, 2020, 258, 120308 CrossRef CAS PubMed.
- M. T. Q. Duong, Y. S. Qin, S. H. You and J. J. Min, Bacteria-cancer interactions: bacteria-based cancer therapy, Exp. Mol. Med., 2019, 51(12), 1–15 CrossRef CAS PubMed.
- W. G. Wang, H. H. Xu, Q. S. Ye, F. Tao, I. Wheeldon, A. H. Yuan, Y. Q. Hu and J. H. Wu, Systemic immune responses to irradiated tumours via the transport of antigens to the tumour periphery by injected flagellate bacteria, Nat. Biomed. Eng., 2022, 6(1), 44–53 CrossRef CAS PubMed.
- Q. Q. Feng, X. T. Ma, K. M. Cheng, G. N. Liu, Y. Li, Y. L. Yue, J. Liang, L. Z. Zhang, T. J. Zhang, X. W. Wang, X. Y. Gao, G. J. Nie and X. Zhao, Engineered Bacterial Outer Membrane Vesicles as Controllable Two-Way Adaptors to Activate Macrophage Phagocytosis for Improved Tumor Immunotherapy, Adv. Mater., 2022, 34(40), e2206200 CrossRef PubMed.
- J. M. Pan, X. L. Li, B. F. Shao, F. N. Xu, X. H. Huang, X. Guo and S. B. Zhou, Self-Blockade of PD-L1 with Bacteria-Derived Outer-Membrane Vesicle for Enhanced Cancer Immunotherapy, Adv. Mater., 2022, 34(7), e2106307 CrossRef PubMed.
- S. Qing, C. L. Lyu, L. Zhu, C. Pan, S. Wang, F. Li, J. H. Wang, H. Yue, X. Y. Gao, R. R. Jia, W. Wei and G. H. Ma, Biomineralized Bacterial Outer Membrane Vesicles Potentiate Safe and Efficient Tumor Microenvironment Reprogramming for Anticancer Therapy, Adv. Mater., 2020, 32(47), e2002085 CrossRef.
- J. T. Liu, J. Huang, L. Zhang and J. P. Lei, Multifunctional metal-organic framework heterostructures for enhanced cancer therapy, Chem. Soc. Rev., 2021, 50(2), 1188–1218 RSC.
|
This journal is © The Royal Society of Chemistry and the Centre National de la Recherche Scientifique 2024 |
Click here to see how this site uses Cookies. View our privacy policy here.