DOI:
10.1039/D3FO05221D
(Paper)
Food Funct., 2024,
15, 3199-3213
β-Nicotinamide mononucleotide supplementation prolongs the lifespan of prematurely aged mice and protects colon function in ageing mice†
Received
27th November 2023
, Accepted 16th February 2024
First published on 22nd February 2024
Abstract
Ageing is defined as the degeneration of physiological functions in numerous tissues and organs of an organism, which occurs with age. As we age, the gut undergoes a series of changes and weaknesses that may contribute to overall ageing. Emerging evidence suggests that β-nicotinamide mononucleotide (NMN) plays a role in regulating intestinal function, but there is still a lack of literature on its role in maintaining the colon health of ageing mice. In our research, Zmpste24−/− mice proved that NMN prolonged their life span and delayed senescence. This study was designed to investigate the effects of long-term intervention on regulating colon function in ageing mice. Our results indicated that NMN improved the pathology of intestinal epithelial cells and intestinal permeability by upregulating the expression of intestinal tight junction proteins and the number of goblet cells, increasing the release of anti-inflammatory factors, and increasing beneficial intestinal bacteria. NMN increased the expression of the proteins SIRT1, NMNAT2, and NMNAT3 and decreased the expression of the protein P53. It also regulated the activity of ISCs by increasing Wnt/β-catenin and Lgr5. Our findings also revealed that NMN caused a significant increase in the relative abundance of Akkermansia muciniphila and Bifidobacterium pseudolongum and notable differences in metabolic pathways related to choline metabolism in cancer. In summary, NMN supplementation can delay frailty in old age, aid healthy ageing, and delay gut ageing.
Introduction
Senescence is defined as the process of degenerative changes in physiological functions that occur in various tissues and organs of the body with age, increasing the susceptibility to infection and the risk of chronic diseases.1 There have been increasing reports on bowel dysfunction, Alzheimer's disease, and cardiovascular disease related to ageing.2,3
The colo-intestinal barrier includes the mechanical barrier, chemical barrier, immune barrier, and microbial barrier. The intestinal epithelium and its intercellular junctions form a remarkably discriminating barrier, shielding the immune system from undigested materials. In addition, they facilitate water uptake and the passage of essential nutrients into the bloodstream.4,5 To prevent excessive transfer of gut contents and metabolites into the circulation, a complete intestinal barrier is essential. Permeability plays an important role in the interaction between an organism, the external environment, and the gut microbiota. In the process of ageing, the intestinal tract may show an increase in the permeability of the epithelial barrier and impaired tight junction proteins.6 It is therefore important to pay attention to gut health.
The increasing prevalence of age-related illnesses has become a pressing global health concern that demands urgent attention and solutions. Historically, natural products have been employed in the management of diverse human ailments. Sirtuins (SIRTs), the mammalian target of rapamycin, p53 and insulin/insulin-like growth factor-1 signalling pathways have been extensively researched.7 As age increases, the levels of SIRT in the body will gradually decrease.8 Knockout experiments on the p53 gene in Caenorhabditis elegans extended the lifespan. SIRT1 deacetylates the transcriptional regulator p53 in an NAD+-dependent manner, leading to the inhibition of P53 activity and affecting pathways involved in the regulation of intestinal tissue homeostasis.9 Ageing alters the balance between inflammatory and anti-inflammatory cytokines, which in turn directly impacts the permeability of the intestines.10
The colon wall's integrity is maintained by several cell types, namely epithelial cells and goblet cells; these cells are regenerated by intestinal stem cells (ISCs) present in the crypts. The rapid renewal of the intestinal epithelium is maintained by ISCs. It has been reported that the number of ISCs in mice and their functional activity in vitro decrease with increasing age.11 In mammals, cells at the base of the crypt expressing G protein-coupled receptor 5 (Lgr5) make up the majority of ISCs under normal circumstances. The activity of ISCs is regulated by the Wnt/β-catenin signaling pathway; the Wnt pathway has been shown to play a significant role in repairing the mechanical barrier of epithelial cell proliferation.12
Shifts in the composition of bacterial communities have been associated with alterations in the characteristics of the mucus barrier.13 As age increases, the symbiotic association between the gut microbiota and the host declines, leading to an escalation in the prevalence of gut dysbiosis. This, in turn, has significant repercussions for the health and lifespan of the host.14
Dysbiosis of gut microbes has been well documented in experimental models of ageing and older adults, with undesirable changes in the composition of the microbiome.15 By maintaining the integrity of the intestinal epithelium, supporting digestion, training the intestinal immune system and inhibiting the growth of pathogenic bacteria, the gut microbiota influences the ongoing homeostasis of metazoans.16
In recent years, NAD+ has become the focus of anti-ageing research. NAD+ is synthesized through multiple pathways, including de novo synthesis and salvage pathways. Most tissues synthesize NAD+ through the NMN and Nicotinamide Riboside (NR) pathways, with some variability in vivo for the tissue types that can use the Preiss–Handler pathway. This process is well-established and reliable. The final step in both pathways involves the synthesis of NAD+ from NMN and ATP, which is catalyzed by one of the nicotinamide mononucleotide adenylate transferase (NMNAT) enzymes.17 NMN is a precursor to the biosynthesis of NAD+ and has received a great deal of attention in recent years for its anti-ageing properties.18 Studies have indicated a connection between the degeneration of the colon and changes in NAD+ levels in ageing mice,19 and some other studies have shown that NMN supplementation can help protect anti-inflammatory, antioxidant, and barrier functions in the jejunum, however, further research on the role of NMN in colonic function, ISCs, microbiota, and metabolites of senescent mice is still lacking.20
Therefore, an integrated analysis was carried out to assess the changes in the survival rates of Zmpste24−/− mice following the oral intake of NMN. We then conducted a comprehensive study examining the effects of NMN supplementation on colon stemness, both in vivo and in vitro, using a colon organoid approach. The aim of our study was to investigate the effects of NMN on the age-related characteristics of the colon. Our findings provide additional evidence for the limited research on age-related dysbiosis and intestinal physiology. We have provided valuable insight into how NMN affects colon barrier function and stemness, the microbiome composition, and metabolites in ageing animal models.
Experimental
Ethics statement
All animal experiments were conducted in accordance with the Regulations and Administration of the Committee at Zhejiang Chinese Medical University. The experiments were approved following a thorough evaluation process (IACUC-20221212-05). All animal experiments were conducted in accordance with the guidelines set out in the Guide for the Care and Use of Laboratory Animals.
Mice.
Zmpste24
−/− mice aged 5–7 weeks were procured from Gempharmatech Co., Ltd (Nanjing, China), while male C57BL/6 mice aged 10 months were sourced from Zhejiang Chinese Medical University (Hangzhou, China). The mice were kept in a specific pathogen-free (SPF) facility with a 12-hour light/dark cycle at 25 ± 2 °C, and free access was given to food and water.
Mouse procedures.
For the lifespan study, after 1 week of acclimatization, Zmpste24−/− mice were randomly assigned to three groups: a control group (PBS) and two groups receiving NMN (Gene Harbor Lifespan, CA, Ningbo, China) supplementation, according to a previous report,21 and divided into the N100 group and N300 group at different doses (100 mg kg−1 and 300 mg kg−1) by oral gavage on alternate days. The study was conducted until natural death, with body weight and frailty index measured every 2 weeks, and fecal samples collected regularly.22
The animal model of natural ageing C57BL/6 mice is simple to establish, reproducing the major physiological, pathological, biochemical and behavioural changes in the ageing process. Based on lifespan results, an appropriate NMN concentration (300 mg kg−1) was determined for investigating intestinal homeostasis in 10-month-old C57BL/6 mice. The C57BL/6 mice were randomly assigned to a PBS group or NMN group and received the assigned treatment by oral gavage every other day. After around 6 months, the mice were humanely killed using cervical dislocation, and several tissues, such as blood and colon, were gathered for further analysis.
Detection of intestinal permeability
The levels of fluorescein isothiocyanate (FITC)-dextran in the serum of Zmpste24−/− mice were determined. Mice were given new cages, deprived of bedding, and fasted without water (overnight) on the first day. Then, each mouse was given the same dose of FITC-dextran (Sigma-Aldrich 3-5kDA, USA) (200 μL per mouse of 400 mg kg−1) at the final concentration divided by its body weight on the second day. Four hours later, blood was collected from the medial canthus venous plexus. Note that a blank well was set up, with the serum of mice without FITC but with an equal amount of PBS, and then the serum was centrifuged at 12
000g for 5 min at 4 °C. Serum samples were prepared as 20 μL serum + 130 μL PBS and then transferred to 96-well ELISA tubes as 50 μL serum samples (set up with 3 duplicate wells). The standard curve was generated simultaneously. The sample concentration was calculated according to the fluorescence reading and the standard curve using a fluorescent enzyme marker at an excitation of 485 nm, and emission of 528 nm. The original concentration in serum was reduced by the dilution times and the relative multiplicity was calculated by the mean value of the control group.
We detected the levels of lipopolysaccharides (LPS) in the serum of C57BL/6 mice, using a Lipopolysaccharides kit (MultiScience, China).
Histology
Colon samples were immersed in 4% paraformaldehyde for 48 hours before being embedded in paraffin and sectioned at 3 μm. All sections were dewaxed and hydrated. They were then stained with haematoxylin and eosin (H&E), and periodic acid-Schiff (PAS).
Sections were sealed with 3% bovine serum albumin and incubated with primary antibodies, specifically anti-Lgr5 (Bioss, bs-20747R, 1
:
100, China). The slices were then incubated with secondary antibodies (FD0136, 1
:
500).
Subsequently, they underwent microscopic evaluation for analysis. The scans were conducted using the Pannoramic Scanner (Pannoramic DESK, 3DHISTECH) and later viewed through Caseviewer C.V 2.3.
Colon crypt isolation and culture
The mouse colon was sliced lengthwise and thoroughly washed with PBS before being vertically sectioned into small pieces. After multiple washing steps with PBS, 5 mL of 20 mM ethylenediaminetetraacetic acid (EDTA) was added to the samples. The test tube containing the intestinal pieces and EDTA was placed on ice for 30 minutes. The EDTA supernatant was then removed. In the next step, 0.1% BSA was added to the pieces and the pieces were allowed to settle by pipetting up and down, with crypts accumulating in the upper liquid. Tissue fragments were allowed to settle under normal gravity for 1 min and the supernatant was removed and examined using inverted microscopy. A 70 μm nylon cell strainer was used to filter the supernatant. Crypts were collected by centrifugation at 1200g for 5 minutes at 4 °C. After discarding the supernatant, 1 mL of DMEMF12 (Cienry, CR-12500, China) was added to the mixture and observed under an inverted microscope and the number of cells counted. When the cell density reached a certain threshold, the crypts were collected again by centrifugation. The resulting crypts were suspended in Matrigel (Corning, 356231, USA) and applied to a 37 °C pre-warmed well plate. After gel formation, 500 μL of complete medium (Stemcell Technologies, 06005, Canada) containing Y-27632 (10 μM, Selleck, 1049, USA) and CHIR (5 μM, Selleck, 1253, USA) was added for colonoid growth and replaced every other day. On day 3, colonoids were treated with NMN (100, 150, 200 mM, Gene Harbor Lifespan, CA, Ningbo, China) or PBS. The buds of colon organoids were analyzed using the Zen image program (Carl Zeiss).
RNA isolation and quantitative real-time PCR
RNA was extracted according to the manufacturer's instructions by homogenising and lysing tissues with AG RNAex Pro Reagent (AG, Cat. AG21101, China). ABScript III RT Master Mix for qPCR with gDNA Remover (ABclonal, Cat. RK20429, Wuhan, China) was used for reverse transcription. qRT-PCR was performed by a LightCycler® 480 Instrument II(Roche) using 2× Universal SYBR Green Fast qPCR MIX (ABclonal, Cat. RK21203, China) DNA in a ROCHE LightCycler®480II System (Rotor Gene 6000 Software, Australia) in triplicate, under the following conditions: 95 °C, 3 min, 40 cycles of 95 °C, 10 s and 60 °C, 30 s. Relative gene expression was quantified using the 2−ΔΔCt method. The primers used in the study are listed in ESI Table S1.†
Western blotting
RIPA extraction buffer was used to lyse tissues or cells. A BCA protein assay kit (FDbio Science Biotech Co., Ltd, FD2001, China) was used to quantify protein concentrations. Protein samples were separated using SDS-PAGE. The proteins were transferred to PVDF membranes. QuickBlock ™ blocking buffer for western blot (Beyotime, P0220, China) was used and incubated for 0.5 h. Incubation with primary antibodies specific to the target protein was performed overnight at 4 °C. In the experiment, Claudin1 (ABclonal, A11530, China), Lgr5 (Bioss, bs-20747R, China), β-catenin (Cell Signaling Technology, 8814S, China), p53 (Cell Signaling Technology, 2524T, America), SIRT1 (ab110304, Abcam, Britain), SIRT4 (Proteintech, 66543-1-lg, China), NMNAT1 (Proteintech, 11399-1-AP, China), NMNAT2 (Abcam, ab56980, Britain), NMNAT3 (Proteintech, 13236-1-AP, China) and β-actin (ABclonal, AC026, China) antibodies were used. The membranes were incubated with horseradish peroxidase-conjugated anti-rabbit immunoglobulin G antibody for 2 hours at room temperature. An ECL kit (FDbio Science, FD800, China) was used to visualise protein bands by chemiluminescence.
Fecal microbial analyses
Fresh mouse feces were collected and genomic DNA was extracted using the FastDNA Spin Kit for Feces (MP Biomedical, USA). Amplification of the 16S rRNA gene V3 to V4 hypervariable regions and paired-end sequencing of purified amplicons were conducted on an Illumina MiSeq platform using standard protocols.
Untargeted fecal metabolomics
Metabolites from the fecal samples of 16-month-old C57BL/6 mice that were gavaged with either PBS or NMN were extracted following standard protocols provided by Majorbio Bio-Pharm Technology Co. The fecal metabolites were extracted using a 20% methanol solution along with vortexing and sonication. All extracted metabolites were analysed according to the standard protocols of Majorbio Bio-Pharm Technology Co. Ltd using ultra-high-pressure liquid chromatography (UPLC) coupled to a triple quadrupole time-of-flight (TOF) system (ABSCIEX-Triple TOF 5600; AB SCIEX, Framingham, MA, USA). Criteria for differential metabolite screening using the Majorbio platform are as follows: fold change of 1 (the difference in metabolites between the control and experimental groups was more than 1-fold.); VIP ≥ 1 for the OPLS-DA model. The VIP value represents the influence of the difference between the corresponding metabolites on classifying and discriminating the samples of each group in the model; p-value < 0.05. Differential metabolites from both groups were condensed and linked to their respective biochemical pathways using metabolic enrichment and database search-based pathway analysis (KEGG).
Statistical analysis
GraphPad Prism version 9.0 software and Image J (https://imagej.nih.gov/ij/) were used for statistical analysis and illustrations. The Kruskal–Wallis test was used for data with non-normal distribution. Data were presented as mean ± SEM. An unpaired t-test (normally distributed data) between two groups was used to calculate p-values. All statistical tests were performed on a two-tailed basis and differences were considered significant at p < 0.05. For lifespan assays, the log
(rank) (Kaplan–Meier) test was performed. The Majorbio Cloud online platform (https://cloud.majorbio.com) was used to analyse the metabolite and microbiota Prof.
Results
NMN improved the life span performance in prematurely ageing mice
The Zmpste24−/− mice were often used as a model for assessing lifespan. To evaluate the effect of NMN supplementation on the lifespan of aged mice, 5–7 weeks-old Zmpste24−/− mice were administered by gavage with NMN (100
300 mg kg−1), and with the PBS as the control (Fig. 1A). N300 extended the overall lifespan (Fig. 1B) of Zmpste24−/− mice and the median life expectancy (Fig. 1C). The results showed that compared with the control group, the N300 group showed increased weight gain in the sixth and eighth weeks (p < 0.05) (Fig. 1D). But there was no significant difference in the N100 group (ESI Fig. S1A–C†).
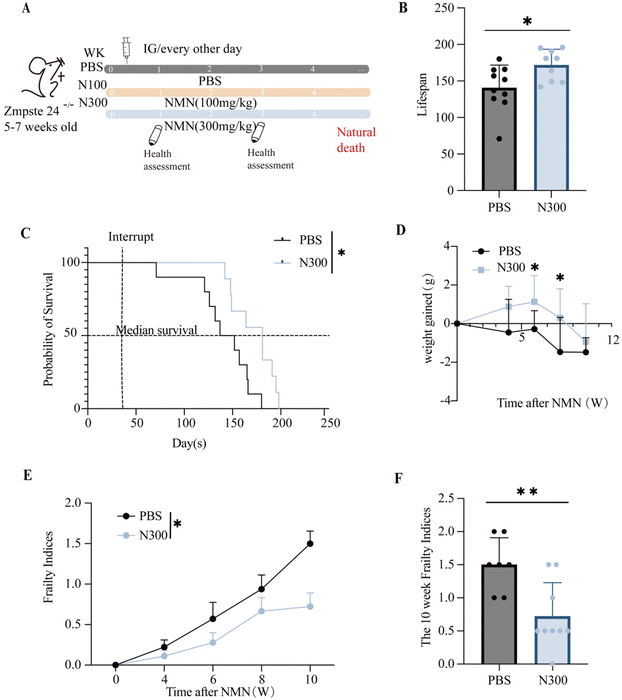 |
| Fig. 1 The life span and frailty indices in Zmpste24−/− mice after NMN treatment. (A) The Zmpste24−/− mice were dosed by gavage with PBS or NMN (100/300 mg kg−1) every other day until natural death, starting at 5–7 weeks of age. (B) The average life span (days) of Zmpste24−/− mice (n = 9 OR 10). (C) Survival curves for Zmpste24−/− mice (n = 9 OR 10) with a median lifespan (days) inset. (D) The weight gained by Zmpste24−/− mice (n ≥ 7). (E) Frailty indices were performed once every two weeks until natural death (n ≥ 7). (F) Frailty indices of Zmpste24−/− mice at the 10th week after intervention (n ≥ 7). *p < 0.05, **p < 0.01and ***p < 0.001 represent significant differences between the PBS group and the N300 group. | |
Sinclair's Frailty Indices table was used to assess the extent of frailty in mice. Frailty Indices indicated that N300 made the overall health of Zmpste24−/− mice better than that of the PBS group (Fig. 1E), and the decline was more significant at 10 weeks post-intervention (p < 0.05) (Fig. 1F), while there was no significant difference in the N100 group (Fig. S1D†).
NMN restored the integrity of the epithelial barrier in the colons of ageing mice
Based on the above results, we selected C57BL/6 mice to investigate the mechanism of NMN in the colon after long-term intervention, and we chose to continue to observe the concentration of NMN (300 mg kg−1) (Fig. 2A). The results showed a tendency to increase the weight gain in mice in the NMN group as compared with the PBS group; especially at 24, 26 and 27 weeks, the NMN group presented a prominently increased weight gain (p < 0.05) (Fig. 2B), reduced the frailty of mice.
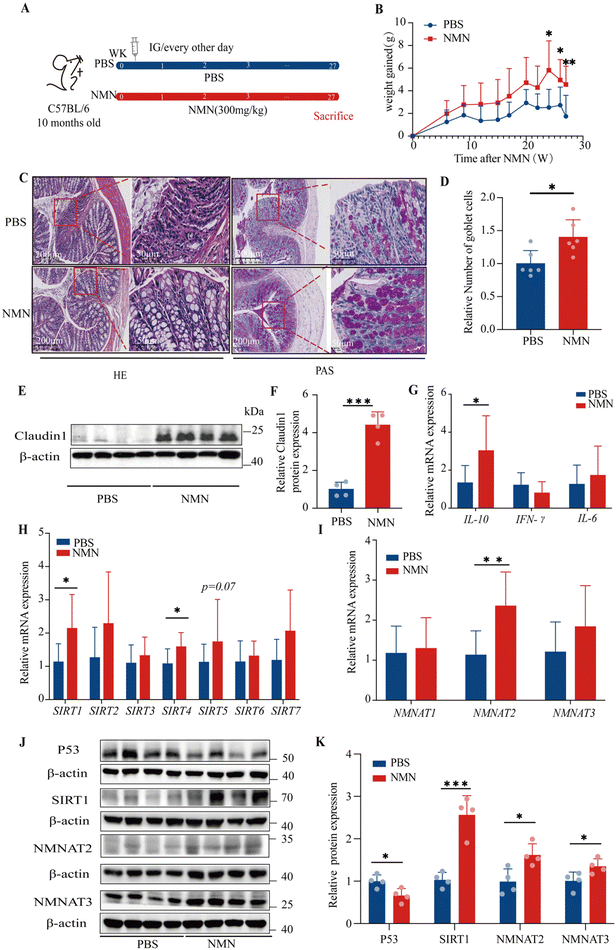 |
| Fig. 2 NMN restored the colon epithelial barrier integrity and the expression of ageing-related genes in ageing mice. (A) C57BL/6 mice were dosed by gavage with vehicle or NMN (300 mg kg−1) every other day until sacrifice, starting at 10 months of age. (B) The weight gained by C57BL/6 mice (n = 7 OR 9). (C) Histological representative picture of a colon sample, H&E representative images and PAS-staining representative images (scale: 50 OR 200 μm). (D) Quantitative analysis of the goblet cells per villus (n = 5 villi/6 mice per group). (E) Western blots for Claudin1 with β-Actin as an internal control (n = 4). (F) Claudin1 protein quantification analyzed using Image J. (G) Relative mRNA levels of the Il-10, IFN-γ and Il-6 genes of the mice colon in the PBS and NMN group (n = 7 OR 9). (H) Relative mRNA levels of the SIRTs gene in the mice colons in the PBS and NMN group (n = 7 OR 9). (I) Relative mRNA levels of NMNAT1, NMNAT2, and NMNAT3 genes of the mice colons in the PBS and NMN group (n = 7 OR 9). (J) Western blots for P53, SIRT1, NMNAT2 and NMNAT3 with β-Actin as an internal control (n = 4). (K) P53, SIRT1, NMNAT2 and NMNAT3 protein quantification analyzed using Image J. *p < 0.05. **p < 0.01 and ***p < 0.001 represent significant differences between the PBS group and the NMN group. | |
After H&E staining, the control mice showed abundant neutrophil and lymphoplasmic cell infiltration in the mucosa and submucosa, while the intrinsic mucosa was damaged; compared with the PBS group, the NMN group showed certain improvement, with fewer acute and chronic inflammatory cells in the lamina propria of the intestinal mucosa, and a clear intestinal wall structure (Fig. 2C). Moreover, a closer observation of Alcian Blue staining showed that the number of goblet cells in the NMN group was higher than that in the PBS group (p < 0.05) (Fig. 2C and D), which represented a significant difference. Therefore, NMN can increase the number of goblet cells and thus improve mucosal barrier function. We examined the expression of markers of the tight junction, including Claudin1, in the colon of mice, as tight junctions are the main components in maintaining the physical barrier of the intestine. NMN increased the protein levels of Claudin1 (p < 0.05) (Fig. 2E and F) as compared with the PBS. Ageing is associated with chronic inflammation. NMN treatment decreased the chronic inflammation by up-regulating the mRNA expression of Il-10 (p < 0.05), while the mRNA expression of IFN-γ and Il-6 had no changes (Fig. 2G). We detected the levels of fluorescein isothiocyanate (FITC)-dextran in the serum of Zmpste24−/− mice, the relative level of FITC in the NMN group was lower than that in the PBS group (p < 0.05) (ESI Fig. S1E†). We detected the levels of lipopolysaccharides (LPS) in the serum of C57BL/6 mice, and the relative LPS level in the NMN group was lower than that in the PBS group (p = 0.052) (ESI Fig. S1F†).
NMN adjusted the activity of genes involved in ageing mice colons
With ageing, the expression of P53 gradually increases, while the levels of SIRT protein in the body gradually decrease. We investigated the effects of NMN supplementation on the gene expression of SIRTs and the results showed that NMN significantly upregulated the gene expression of Sirt1 and Sirt4 (p < 0.05) (Fig. 2H). In this study, we found that NMN supplementation decreased the protein levels of P53 and increased the protein levels of SIRT1 the marker of ageing as compared with the control (p < 0.05) (Fig. 2J and K). However, the levels of SIRT4 protein showed no changes (ESI Fig. S1A and B†). We speculate that mRNA increased and protein levels remained unchanged, which may involve the comprehensive influence of multiple factors such as post-translational modification and protein degradation. The study reported that the overexpression of NMNAT1-3 was able to increase NAD+ levels in various tissues,23–25 thereby preventing age-related declines in NAD+ levels. NMN treatment up-regulated the mRNA expression of NMNAT2 (p < 0.05) and NMNAT3 (Fig. 2I), NMN increased the protein levels of NMNAT2, and NMNAT3 (p < 0.05) (Fig. 2J and K). NMNAT1 mRNA (Fig. 2I) and protein (ESI Fig. S1A and B†) did not change.
NMN promoted the regeneration of intestinal stem cells in the colons of ageing mice
Here, we showed the number and proliferative activity of ISCs, as was the case with ISC function assessed in vivo. The stemness of the intestinal stem cells up-regulated the mRNA Lgr5, β-catenin Wnt5a, Wnt6 and Axin2 (p < 0.05) (Fig. 3A); the number of Lgr5+ ISCs significantly increased in the NMN (Fig. 3B) and NMN increased the protein levels of Lgr5 and β-catenin as compared with PBS (p < 0.05) (Fig. 3C and D). With the establishment of colonic organoids in vivo (Fig. 3E), regarding the number of crypt-like structures showing budding, NMN intervention increased the number of differentiated buds per organoid (p < 0.05) (Fig. 3F and I). For in vitro experiments, colon organoids were supplemented with different concentrations of 100, 150, and 200 mM NMN (Fig. 3G), and the statistical organoid germination showed that NMN200 had the best effect (p < 0.05) (ESI Fig. S1G†). On treatment with NMN in vitro, regarding the numbers of crypt-like structures (Fig. 3H), 200 mM NMN intervention increased the number of differentiated buds per organoid (p < 0.05) (Fig. 3J), and up-regulated the mRNA Lgr5, and β-catenin (p < 0.05) (Fig. 3K).
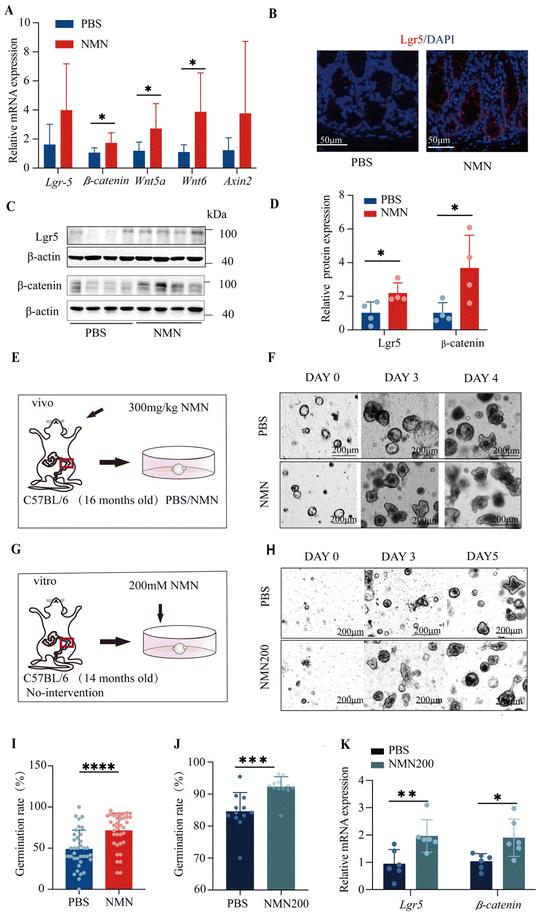 |
| Fig. 3 NMN promoted the regeneration of intestinal stem cells in ageing mice via Lgr5 and Wnt/β-catenin. (A) Relative mRNA levels of the Lgr5, β-catenin, Wnt5a, Wnt6 and Axin2 genes of the mice colon in the PBS and NMN group (n = 7 OR 9). (B) Representative images of immunofluorescence staining of Lgr5 in the colon of C57BL/6 mice (scale: 50 μm). (C) Western blots for Lgr5 and β-catenin with β-actin as an internal control (n = 4). (D) The protein levels of Lgr5 and β-catenin quantification were analyzed using ImageJ. (E) Pattern diagram: Colonic organoids from 16-month-old C57BL/6 mice were dosed by gavage with PBS or NMN every other day. (F) Representative brightfield images of organoid structures derived from the colons of PBS or NMN-treated mice (scale: 200 μm). (G) Pattern diagram: The effect of NMN on the senescence of intestinal epithelial organoids from 14-month-old C57BL/6 mice. (H) Representative brightfield images of organoid structures derived from the colons of ageing mice in the in vitro treatment with 200 mM NMN (scale: 200 μm). (I) The germination rate of organoids derived from the group of PBS and NMN (n = 6) on the last day, count 6 views per hole. (J) The germination rate of organoids derived from the PBS and NMN200 group (n = 6) on the last day, count 2 views per hole. (K) Relative mRNA levels of Lgr5 and β-catenin genes of organoids in the PBS and NMN200 group. *p < 0.05, **p < 0.01, and ***p < 0.001 represent significant differences between the PBS group and the NMN group, and between the PBS group and the NMN200 group. | |
NMN restructured the gut microbiome in ageing mice
We looked at several indicators of alpha diversity to analyse changes in the diversity of the gut microbiota in each group. The Shannon and Simpson indices are relative to the mean and homogeneity, whereas the Chao index primarily reflects the number of OTU species. The results showed that NMN administration increased Shannon's index and indexes for other observed species (Fig. 4A–C); Shannon's was statistically significant (p < 0.05) (Fig. 4A). NMN increased gut species diversity. Principal Coordinate Analysis (PCoA) was performed to assess the comparability of the microbial communities between the two groups. PCoA results (Fig. 4D) showed differences between the NMN and PBS groups, suggesting that the microbial composition was different. These results indicated that NMN affected the microbial composition of the feces of the mice. The PCoA showed that the primary principal component and the secondary principal component accounted for 25.26% and 17.5%, respectively, of the total results of the analysis. At the phylum level (Fig. 4E), NMN supplementation notably increased the relative abundance of Firmicute, Proteobacteria (p < 0.05), Actinobacteria (p = 0.059) and Verrucomicrobiota (p = 0.06) and decreased the relative abundance of Desulfobacterota (p < 0.05). At the genus level (Fig. 4F), the results indicated that NMN supplementation notably boosted the relative abundance of Dubosiella (p < 0.05), Parasutterella (p < 0.05), Bifidobacterium (p < 0.05), Akkermansia (p = 0.06), and Gordonibacter (p < 0.05), and remarkably lowered the relative abundance of Desulfovibrio (p < 0.05) and Bacteroides, as compared with that of mice. At the species level (Fig. 4G), NMN boosted the relative abundance of Bifidobacterium pseudolongum (p < 0.05), Akkermansia muciniphila (p = 0.06), lowering the relative abundance of Alistipes (p < 0.05) compared with the PBS group. An increase in beneficial bacteria in the NMN-treated group is important for gut homeostasis, which can be improved with age.
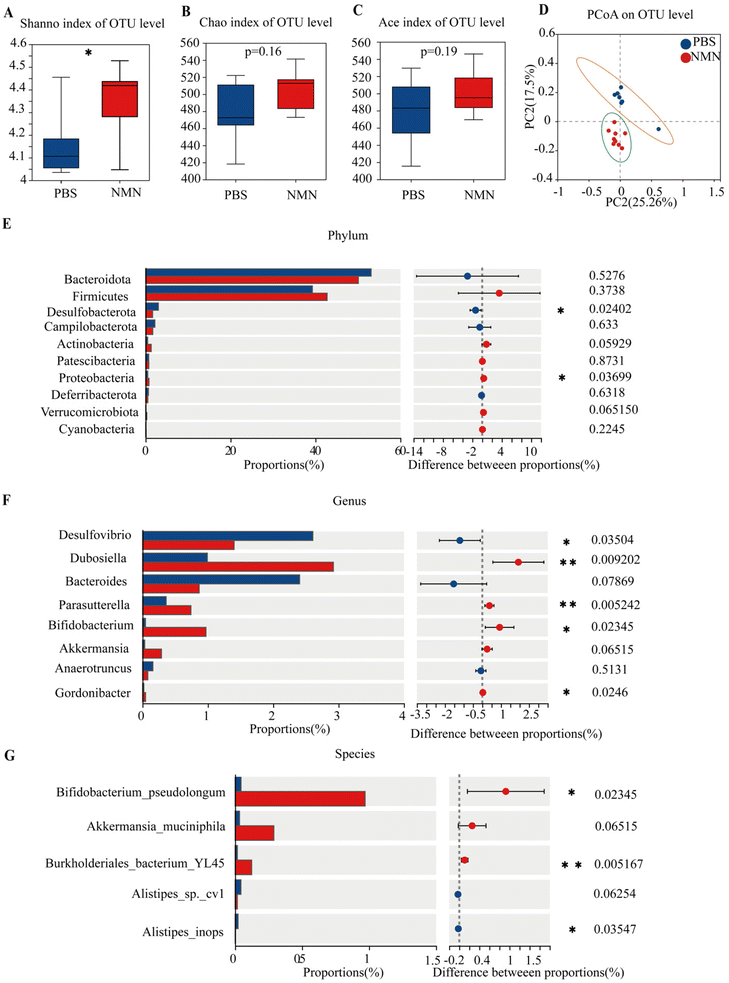 |
| Fig. 4 NMN restructured the gut microbiome in ageing mice. (A) Shannon index of OUT level. (B) Chao index of OUT level. (C) Ace index of OUT level. (D) PCoA analysis based on the weighted UniFrac distance. (E) Relative abundance of intestinal bacteria at the phylum level. (F) Relative abundance of intestinal bacteria at the genus level. (G) Relative abundance of intestinal bacteria at the species level. *p < 0.05, **p < 0.01and ***p < 0.001 represent significant differences between the PBS group and the NMN group. | |
NMN changed the fecal metabolism in ageing mice
To analyse the effects of NMN supplementation on fecal metabolites in mice, untargeted metabolomics was performed. The PLS-DA and OPLS-DA results showed that the metabolite profiling data sets were clustered separately between the NMN group and the PBS group (Fig. 5A and B). The permutation test showed that the OPLS-DA model was reliable without any over-fitting (Fig. 5C). The volcano plot showed significant changes in the fecal metabolite profiles for the NMN group. Compared to the control group, 118 metabolites were upregulated and 79 were downregulated (Fig. 5D). These metabolites were significantly enriched in 20 pathways, such as choline metabolism in cancer (phosphocholine, PC (18:0/0:0), LysoPC (16:0), LysoPC (18:0), DG (20:5), DG (20:2)), MAPK signal pathway (DG (20:5), DG (20:2)), and glycosaminoglycan (chondroitin) (p < 0.05) (Fig. 5E). Metabolites based on significant KEGG pathway enrichment (p < 0.05) (ESI Fig. S2C†), NMN supplementation resulted in a significantly lower concentration of phosphocholine, PC (18:0/0:0), LysoPC (16:0), LysoPC (18:0), and palmitoyl glucuronide, compared to the PBS group, DG(20:5), DG(20:2), chondroitin and galactaric acid were higher in the NMN group as compared to the PBS group. Based on the above sequencing and metabolite results, we conducted a correlation analysis between differential bacteria and metabolites, and the results showed that choline metabolism (phosphocholine, PC (18:0/0:0), LysoPC (16:0), LysoPC (18:0)) was positively correlated with Alistipes, and negatively correlated with Bifidobacterium pseudolongum, Akkermansia muciniphila, Burkholderiales_bacterium_YL45, DG(20:5), DG (20:2), chondroitin and galactaric acid were negatively correlated with Alistipes, and positively correlated with Bifidobacterium pseudolongum, Akkermansia muciniphila, Burkholderiales_bacterium_YL45. Taken together, the results showed that the changed bacterial structure could impact the composition of fecal metabolite constituents.
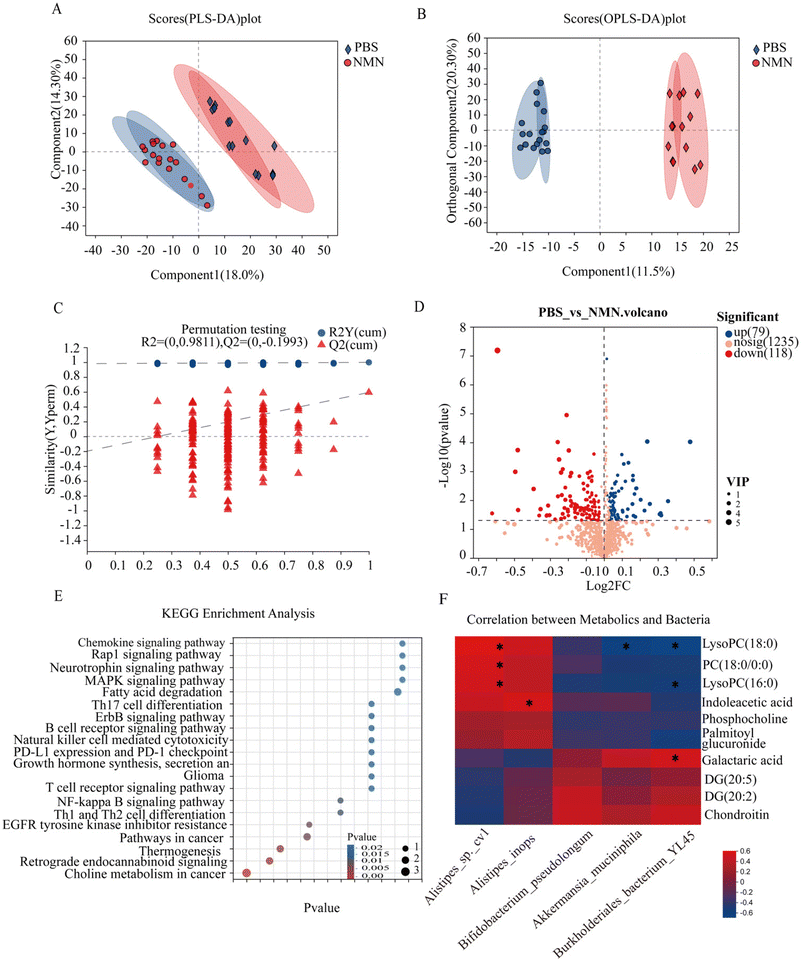 |
| Fig. 5 The impact of NMN supplementation on the fecal metabolome of ageing mice. (A) Score (PLS-DA) plot. (B) Score (OPLS-DA) plot. (C) Permutation test plot. (D) PBS_VS_NMN. Volcano. (E) KEGG enrichment analysis based on significantly altered metabolites. (F) Correlation analysis of differential bacteria and metabolites. (p < 0.05). *p < 0.05, **p < 0.01 and ***p < 0.001 represent significant differences between the PBS group and the NMN group. | |
Discussion
How to maintain healthy ageing is the enduring focus of public concern. In this study, we have shown that NMN consistently extended the healthy and median lifespan of Zmpste24−/− and improved the Zmpste24−/− ageing phenotype. Our study has elaborated on the beneficial effects of NMN in protecting colonic function in ageing mice. These effects include the improvement of intestinal barrier function by regulating goblet cells, the stimulation of tight junctions, the control of the activity of genes involved in ageing, and the regulation of ISCs to protect the intestinal barrier. Additionally, NMN increased the richness and reversed age-related changes in the abundance of the most common bacterial species and metabolites.
The Zmpste24−/− mice are mice that have prematurely aged with slow weight gain, malnutrition and progressive hair loss, with a median survival of about 20 weeks.26 Our study showed that with NMN intervention, the median lifespan of the mice increased from 21.4 weeks to 25.7 weeks, representing an increase of more than 20%. Weight gain was maintained in both models, and we concluded that NMN effectively increased body weight while maintaining overall health. The insufficient impact of the N100 may be attributed to a low dosage or inadequate sample size.
It has also been shown that a chemical barrier is formed by the mucus produced by goblet cells in the intestine.27 One hallmark of healthy ageing may be a balance between pro-inflammatory and anti-inflammatory activity. What sets older adults apart is the ability to maintain (or possibly up-regulate) anti-inflammatory activity, although pro-inflammatory activity is accompanied by an increase in all older adults.10 The increased expression of the anti-inflammatory factor IL-10 in the ageing colon provided evidence for the anti-inflammatory effects of NMN; IL-10 has been reported to have a role in the reduction of intestinal permeability.28 Beneficial microorganisms like Akkermansia muciniphila29 and Bifidobacterium pseudolongum30 have been shown to promote an increase in mucus thickness and improve host tight junctions, reducing permeability. Intestinal permeability increases during ageing,31 and NMN could reverse this process.32 Our data confirmed the agreement with previous data (ESI Fig. S1E and F†). The study findings suggest that NMN had a positive effect on the pathology of intestinal epithelial cells and intestinal permeability, this was achieved by upregulating the expression of intestinal tight junction proteins and the number of goblet cells. Additionally, NMN was found to increase the level of anti-inflammatory factor IL-10, decrease LPS, and increase beneficial bacteria, which promoted the repair of the intestinal mucosal barrier.
SIRT1 is the most studied of the seven human SIRT subtypes and is an important factor in the delay of cell ageing and the extension of the life span of organisms.8 SIRT1 is a NAD+-dependent deacetylase that can deacetylate the tumour suppressor protein P53.9 SIRT4 is another potential target for improving basic metabolism and extending the healthy life span in Drosophila.33 As previously reported, p53 knock-out experiments prolong life.11 NMNAT is an incredible rate-limiting enzyme found in all organisms, it catalyzes a crucial step in the biosynthesis of NAD+ by ATP and NMN33 (ESI Fig. S1H†). There is a previous report on reduced NAD+ content and NMNAT2 protein expression in oocytes of aged mice.24 Studies have shown the ability to increase NAD+ levels in various tissues by overexpressing NMNAT3 in mice, preventing the decline in levels of NAD+ associated with ageing.25 The deletion of NMNAT2 leads to mitochondrial damage as well as a decrease in its substrate product, AMPK coordinates an integrated signalling network that constitutes metabolic pathways and is involved in AD pathogenesis.34 The AMPK pathway serves as a highly integrated stress response system; this system is evolutionarily conserved and adeptly detects changes in NAD+ /NADH ratios, responds by activating a common protective transcriptional response that prevents ageing and promotes longevity,35 and the activation of AMPK is directly related to SIRT1.36,37 Multiple studies have demonstrated increased NAD+/NADH ratios after NMN intervention.38,39 Our findings agree that a pathway likely exists between p53 and NMN, as represented by NMNAT2/NMNAT3 → NAD+ → AMPK → SIRT1 → p53; studies suggest that NMNAT2 and NMNAT3 are the primary regulatory factors in this process. Interestingly, the study identified NMNAT2 as a new downstream target gene of p5340 and speculated that there may be a feedback loop between p53 and NAD+. This may explain the mechanism that regulates the increase in NMNAT after NMN intervention in our study.
ISC marker Lgr5 is a receptor for R-spondin (RSPO), which is known to enhance Wnt signalling in proliferating crypts.41 Lgr5 is the receptor responsible for triggering the Wnt signaling cascade, which induces the transformation of β-catenin into the nucleus.42 Wnt signaling components (including Wnt5a, Wnt6, and Axin2) are required for epithelial homeostasis and are essential for the homeostasis and proliferation of ISCs.43 A decline in organoid formation in the crypts of older mice was also reported, which can be mediated by improved Wnt signaling.12 The decreased Wnt levels at least partially explain the breakdown of the epithelial barrier and reduced goblet cell numbers.44 The restoration of tight cortical junctions and intestinal barrier integrity is accompanied by the restoration of the Wnt-expressing ISC layer. Studies have shown that activation of the Wnt pathway in epithelial cells is partly responsible for the repair of the intestinal mucosal barrier by the anti-inflammatory factor IL-10.45 SIRT1 also deacetylates S6K1, increasing phosphorylation through mTORC1, which increases protein synthesis and the number of ISCs.46 Our study suggests that the protective function of NMN on epithelial integrity seems to be mainly mediated by ISC stimulation.
The composition of gut microbiota in a life cycle may regulate health and disease in ageing populations, and the hallmark of healthy ageing may be core microbiota balance.15,47 Intestinal microbiota plays a key role in the integrity of the intestinal epithelium and enhances the role of the intestinal barrier by influencing the apoptosis and renewal of intestinal epithelial cells through the expression and function of tight junction proteins.16 In the study, age-related gut microbiota dysbiosis was observed, characterised by reduced alpha diversity and altered beta diversity, as well as changes in the abundance of several gut microbiota members.48 A decrease in Firmicutes has been reported in ageing mice.49 Ma et al. found that ageing reduced Actinobacteria in male mice,2 and increased Proteobacteria found in centenarians.50 The phylum Verrucomicrobia, with its members Verrucomicrobiaceae, Akkermansia and Akkermansia muciniphila, was identified as the main biomarker in centenarians.51 It was recently reported that the intervention with Akkermansia muciniphila was an alternative and effective way to maintain a healthy ageing process.52 So far, there have been no reports on ageing and Bifidobacterium pseudolongum but as a probiotic, Bifidobacterium pseudolongum can treat liver cancer, provide anti-tumour immunity, and boost the host's immune response.53–56 In studies examining host frailty as a factor influencing microbiome composition, the Alistipes genus was found to be more abundant in the frailest individuals.57 Although our study showed no significant difference in Akkermansia muciniphila, there have been previous literature reports on an increase in Akkermansia muciniphila after three months of intervention, consistent with the trend of our results. This evidence suggests that NMN may promote intestinal homeostasis and healthy ageing by increasing the abundance of Actinobacteria, Verrucomicrobia, Akkermansia muciniphila and Bifidobacterium pseudolongum. Based on the above, as well as our study, we believe that Bifidobacterium pseudolongum may be the next potential probiotic for ageing.
Choline metabolism is an important part of intracellular phospholipid metabolism.58 It has been reported that capsaicin can treat ageing AD by significantly increasing the relative abundance of Akkermansia muciniphila and decreasing the level of LysoPC (16
:
0).59 The research indicated that ageing might decrease energy production through β-oxidation because of a decrease in NAD+, despite the accumulation of the lipid energy metabolism intermediate LysoPC (18
:
0).60 The study reported that dietary restriction induces age-dependent metabolic changes in Caenorhabditis elegans at the whole-organism level, and these changes suggest a potential association between low levels of phosphocholine and increased life expectancy.61,62 There have been reports that serum choline was reduced in studies on model organisms with extended healthy lifespans.63 Chondroitin describes a fraction of chondroitin sulfate with little or no sulfation. Researchers found that chondroitin is an anti-ageing drug that may help to prolong life or improve health indicators related to ageing.64 It has been reported that chondroitin sulfate, when taken orally with Akkermansia muciniphila, restricted the growth of sulfatase-secreting and sulfate-reducing bacteria, which may help improve osteoarthritis.65 This evidence suggests that NMN may increase the abundance of Akkermansia muciniphila, and affect choline metabolism and glycosaminoglycan metabolism. NMN may affect metabolites in ageing hosts, indicating potential benefits for age-related conditions.
Conclusions
In this study, NMN had the effects of prolonging life and delaying senescence, and demonstrated a protective effect on colon mucosa by controlling the activity of genes involved in ageing, intestinal stem cell differentiation and improving intestinal flora homeostasis, which had a positive effect on intestinal health. However, there is still a lot of work to be explored. First, our study found that NMN regulation could improve intestinal health in many aspects but the specific mechanisms of how NMN regulates the various aspects still need proof. Second, Igor Shats et al. found that bacteria can use their own PncA to connect the amidated NAD+ remediation pathway to the deamidated NAD+ synthesis pathway in mammalian cells and tissues, thereby promoting NAD+ synthesis.66 We speculate whether there is a connection between NMN and bacteria in our research, which may promote the production of NAD +. AMPK enhances SIRT1 activity, resulting in the deacetylation and modulation of the activity of downstream SIRT1 targets that include p53 and autophagy.36,37 The study mentioned that caloric restriction and resveratrol extend the lifespan of Caenorhabditis elegans by igniting autophagy through the activation of SIRT1.67 The activation of AMPK also directly activates autophagy, and the studies unequivocally demonstrate that autophagy plays a pivotal role in maintaining, regulating, and repairing the intestinal epithelial barrier. Furthermore, it is a crucial mechanism that regulates the intestinal barrier function in response to cellular stress through tight connections.68,69 Although autophagy was not explored in our study, the detailed mechanisms between AMPK, SIRT1 and autophagy are still worth our attention and warrant further exploration. Three distinct signalling mechanisms, namely, Notch, Hh and BMP, were identified as playing a role in ISC self-renewal, proliferation, differentiation and villus formation.70 The precise regulation of these signals via NMN activation requires further investigation. In general, our findings suggest that NMN supplementation may be a viable strategy for maintaining healthy ageing in the gut.
Author contributions
YC Yu conceptualized and designed the study. YR Gu, LD Gao and JM He performed the experiments and data curation. M Luo, M Hu, YX Lin and JX Li performed the investigation and revised the manuscript. JM Si performed the supervision. YR Gu and LD Gao performed the visualization, statistical analysis work and drafted the manuscript, TY Hou, and YX Lin helped in editing and revising the manuscript. All authors read and approved the final manuscript.
Conflicts of interest
There are no conflicts to declare.
Acknowledgements
This work was supported by the “Pioneer” and “Leading Goose” R&D Program of Zhejiang (No. 2022C03145), National Natural Science Foundation of China (No. 82300621).
References
- R. D. Molony, A. Malawista and R. R. Montgomery, Reduced dynamic range of antiviral innate immune responses in aging, Exp. Gerontol., 2018, 107, 130–135 CrossRef CAS PubMed.
- J. Ma, Y. Hong, N. Zheng, G. Xie, Y. Lyu, Y. Gu, C. Xi, L. Chen, G. Wu, Y. Li, X. Tao, J. Zhong, Z. Huang, W. Wu, L. Yuan, M. Lin, X. Lu, W. Zhang, W. Jia, L. Sheng and H. Li, Gut microbiota remodeling reverses aging-associated inflammation and dysregulation of systemic bile acid homeostasis in mice sex-specifically, Gut Microbes, 2020, 11, 1450–1474 CrossRef CAS PubMed.
- D. Liu, Y. Ouyang, R. Chen, M. Wang, C. Ai, H. R. El-Seedi, M. M. R. Sarker, X. Chen and C. Zhao, Nutraceutical potentials of algal ulvan for healthy aging, Int. J. Biol. Macromol., 2022, 194, 422–434 CrossRef CAS PubMed.
- Y. Kurashima and H. Kiyono, Mucosal Ecological Network of Epithelium and Immune Cells for Gut Homeostasis and Tissue Healing, Annu. Rev. Immunol., 2017, 35, 119–147 CrossRef CAS PubMed.
- S. Lechuga and A. I. Ivanov, Disruption of the epithelial barrier during intestinal inflammation: Quest for new molecules and mechanisms, Biochim. Biophys. Acta, Mol. Cell Res., 2017, 1864, 1183–1194 CrossRef CAS PubMed.
- A. M. Salazar, R. Aparicio, R. I. Clark, M. Rera and D. W. Walker, Intestinal barrier dysfunction: an evolutionarily conserved hallmark of aging, Dis. Models Mech., 2023, 16, dmm049969 CrossRef CAS PubMed.
- L. Gao, X. Liu, X. Luo, X. Lou, P. Li, X. Li and X. Liu, Antiaging effects of dietary supplements and natural products, Front. Pharmacol., 2023, 14, 1192714 CrossRef CAS PubMed.
- C. Chen, M. Zhou, Y. Ge and X. Wang, SIRT1 and aging related signaling pathways, Mech. Ageing Dev., 2020, 187, 111215 CrossRef CAS PubMed.
- A. L. C. Ong and T. S. Ramasamy, Role of Sirtuin1-p53 regulatory axis in aging, cancer and cellular reprogramming, Ageing Res. Rev., 2018, 43, 64–80 CrossRef CAS PubMed.
- J. Campisi, P. Kapahi, G. J. Lithgow, S. Melov, J. C. Newman and E. Verdin, From discoveries in ageing research to therapeutics for healthy ageing, Nature, 2019, 571, 183–192 CrossRef CAS PubMed.
- D. He, H. Wu, J. Xiang, X. Ruan, P. Peng, Y. Ruan, Y. G. Chen, Y. Wang, Q. Yu, H. Zhang, S. L. Habib, R. A. De Pinho, H. Liu and B. Li, Gut stem cell aging is driven by mTORC1 via a p38 MAPK-p53 pathway, Nat. Commun., 2020, 11, 37 CrossRef CAS PubMed.
- Y. Qi, J. He, Y. Zhang, Q. Ge, Q. Wang, L. Chen, J. Xu, L. Wang, X. Chen, D. Jia, Y. Lin, C. Xu, Y. Zhang, T. Hou, J. Si, S. Chen and L. Wang, Heat-inactivated Bifidobacterium adolescentis ameliorates colon senescence through Paneth-like-cell-mediated stem cell activation, Nat. Commun., 2023, 14, 6121 CrossRef CAS PubMed.
- M. E. Johansson, H. E. Jakobsson, J. Holmén-Larsson, A. Schütte, A. Ermund, A. M. Rodríguez-Piñeiro, L. Arike, C. Wising, F. Svensson, F. Bäckhed and G. C. Hansson, Normalization of Host Intestinal Mucus Layers Requires Long-Term Microbial Colonization, Cell Host Microbe, 2015, 18, 582–592 CrossRef CAS PubMed.
- S. Donati Zeppa, D. Agostini, F. Ferrini, M. Gervasi, E. Barbieri, A. Bartolacci, G. Piccoli, R. Saltarelli, P. Sestili and V. Stocchi, Interventions on Gut Microbiota for Healthy Aging, Cells, 2022, 12, 34 CrossRef PubMed.
- E. N. DeJong, M. G. Surette and D. M. E. Bowdish, The Gut Microbiota and Unhealthy Aging: Disentangling Cause from Consequence, Cell Host Microbe, 2020, 28, 180–189 CrossRef CAS PubMed.
- H. Kayama, R. Okumura and K. Takeda, Interaction Between the Microbiota, Epithelia, and Immune Cells in the Intestine, Annu. Rev. Immunol., 2020, 38, 23–48 CrossRef CAS PubMed.
- X. A. Cambronne and W. L. Kraus, Location, Location, Location: Compartmentalization of NAD(+) Synthesis and Functions in Mammalian Cells, Trends Biochem. Sci., 2020, 45, 858–873 CrossRef CAS PubMed.
- S. Ur Rahman, A. Qadeer and Z. Wu, Role and Potential Mechanisms of Nicotinamide Mononucleotide in Aging, Aging Dis., 2023 DOI:10.14336/ad.2023.0519-1.
- X. Zhu, W. Shen, Y. Wang, A. Jaiswal, Z. Ju and Q. Sheng, Nicotinamide adenine dinucleotide replenishment rescues colon degeneration in aged mice, Signal Transduction Targeted Ther., 2017, 2, 17017 CrossRef PubMed.
- M. Ru, W. Wang, Z. Zhai, R. Wang, Y. Li, J. Liang, D. Kothari, K. Niu and X. Wu, Nicotinamide mononucleotide supplementation protects the intestinal function in aging mice and D-galactose induced senescent cells, Food Funct., 2022, 13, 7507–7519 RSC.
- K. F. Mills, S. Yoshida, L. R. Stein, A. Grozio, S. Kubota, Y. Sasaki, P. Redpath, M. E. Migaud, R. S. Apte, K. Uchida, J. Yoshino and S. I. Imai, Long-Term Administration of Nicotinamide Mononucleotide Mitigates Age-Associated Physiological Decline in Mice, Cell Metab., 2016, 24, 795–806 CrossRef CAS PubMed.
- M. B. Schultz, A. E. Kane, S. J. Mitchell, M. R. MacArthur, E. Warner, D. S. Vogel, J. R. Mitchell, S. E. Howlett, M. S. Bonkowski and D. A. Sinclair, Age and life expectancy clocks based on machine learning analysis of mouse frailty, Nat. Commun., 2020, 11, 4618 CrossRef CAS PubMed.
- X. R. Mao, D. M. Kaufman and C. M. Crowder, Nicotinamide mononucleotide adenylyltransferase promotes hypoxic survival by activating the mitochondrial unfolded protein response, Cell Death Dis., 2016, 7, e2113 CrossRef CAS PubMed.
- X. Wu, F. Hu, J. Zeng, L. Han, D. Qiu, H. Wang, J. Ge, X. Ying and Q. Wang, NMNAT2-mediated NAD(+) generation is essential for quality control of aged oocytes, Aging Cell, 2019, 18, e12955 CrossRef PubMed.
- M. J. Son, Y. Kwon, T. Son and Y. S. Cho, Restoration of Mitochondrial NAD(+) Levels Delays Stem Cell Senescence and Facilitates Reprogramming of Aged Somatic Cells, Stem Cells, 2016, 34, 2840–2851 CrossRef CAS PubMed.
- Y. Wang, K. Shilagardi, T. Hsu, K. O. Odinammadu, T. Maruyama, W. Wu, C. S. Lin, C. B. Damoci, E. D. Spear, J. Y. Shin, W. Hsu, S. Michaelis and H. J. Worman, Abolishing the prelamin A ZMPSTE24 cleavage site leads to progeroid phenotypes with near-normal longevity in mice, Proc. Natl. Acad. Sci. U. S. A., 2022, 119, e2118695119 CrossRef CAS PubMed.
- J. K. Gustafsson and M. E. V. Johansson, The role of goblet cells and mucus in intestinal homeostasis, Nat. Rev. Gastroenterol. Hepatol., 2022, 19, 785–803 CrossRef PubMed.
- F. Meyer, D. Wendling, C. Demougeot, C. Prati and F. Verhoeven, Cytokines and intestinal epithelial permeability: A systematic review, Autoimmun. Rev., 2023, 22, 103331 CrossRef CAS PubMed.
- S. Zhao, W. Liu, J. Wang, J. Shi, Y. Sun, W. Wang, G. Ning, R. Liu and J. Hong, Akkermansia muciniphila improves metabolic profiles by reducing inflammation in chow diet-fed mice, J. Mol. Endocrinol., 2017, 58, 1–14 CAS.
- I. Mangin, F. Dossou-Yovo, C. Lévêque, M. V. Dessoy, O. Sawoo, A. Suau and P. Pochart, Oral administration of viable Bifidobacterium pseudolongum strain Patronus modified colonic microbiota and increased mucus layer thickness in rat, FEMS Microbiol. Ecol., 2018, 94, fiy177 CrossRef PubMed.
- Y. C. Koh, L. H. Kuo, Y. C. Tung, M. Weerawatanakorn and M. H. Pan, Identification of Indicative Gut Microbial Guilds in a Natural Aging Mouse Model, ACS Omega, 2023, 8, 36569–36580 CrossRef CAS PubMed.
- P. Huang, X. Wang, S. Wang, Z. Wu, Z. Zhou, G. Shao, C. Ren, M. Kuang, Y. Zhou, A. Jiang, W. Tang, J. Miao, X. Qian, A. Gong and M. Xu, Treatment of inflammatory bowel disease: Potential effect of NMN on intestinal barrier and gut microbiota, Curr. Res. Food Sci., 2022, 5, 1403–1411 CrossRef CAS PubMed.
- H. N. Jayaram, P. Kusumanchi and J. A. Yalowitz, NMNAT expression and its relation to NAD metabolism, Curr. Med. Chem., 2011, 18, 1962–1972 CrossRef CAS PubMed.
- X. S. Cheng, F. X. Shi, K. P. Zhao, W. Lin, X. Y. Li, J. Zhang, Y. Y. Bu, R. Zhu, X. H. Li, D. X. Duan, X. Y. Ji, J. S. Wei, J. Z. Wang, J. Du and X. W. Zhou, Nmnat2 attenuates amyloidogenesis and up-regulates ADAM10 in AMPK activity-dependent manner, Aging, 2021, 13, 23620–23636 CrossRef CAS PubMed.
- H. G. Keizer, R. Brands and W. Seinen, An AMP Kinase-pathway dependent integrated stress response regulates ageing and longevity, Biogerontology, 2023, 24, 443–455 CrossRef CAS PubMed.
- X. Han, H. Tai, X. Wang, Z. Wang, J. Zhou, X. Wei, Y. Ding, H. Gong, C. Mo, J. Zhang, J. Qin, Y. Ma, N. Huang, R. Xiang and H. Xiao, AMPK activation protects cells from oxidative stress-induced senescence via autophagic flux restoration and intracellular NAD(+) elevation, Aging Cell, 2016, 15, 416–427 CrossRef CAS PubMed.
- C. Cantó, Z. Gerhart-Hines, J. N. Feige, M. Lagouge, L. Noriega, J. C. Milne, P. J. Elliott, P. Puigserver and J. Auwerx, AMPK regulates energy expenditure by modulating NAD+ metabolism and SIRT1 activity, Nature, 2009, 458, 1056–1060 CrossRef PubMed.
- Z. Sun, L. Liu, H. Liang and L. Zhang, Nicotinamide mononucleotide induces autophagy and ferroptosis via AMPK/mTOR pathway in hepatocellular carcinoma, Mol. Carcinog., 2024 DOI:10.1002/mc.23673.
- H. Wang, Y. Sun, C. Pi, X. Yu, X. Gao, C. Zhang, H. Sun, H. Zhang, Y. Shi and X. He, Nicotinamide Mononucleotide Supplementation Improves Mitochondrial Dysfunction and Rescues Cellular Senescence by NAD(+)/Sirt3 Pathway in Mesenchymal Stem Cells, Int. J. Mol. Sci., 2022, 23, 14739 CrossRef CAS PubMed.
- L. Z. Pan, D. G. Ahn, T. Sharif, D. Clements, S. A. Gujar and P. W. Lee, The NAD+ synthesizing enzyme nicotinamide mononucleotide adenylyltransferase 2 (NMNAT-2) is a p53 downstream target, Cell Cycle, 2014, 13, 1041–1048 CrossRef CAS PubMed.
- L. Novellasdemunt, A. Kucharska, C. Jamieson, M. Prange-Barczynska, A. Baulies, P. Antas, J. van der Vaart, H. Gehart, M. M. Maurice and V. S. Li, NEDD4 and NEDD4L regulate Wnt signalling and intestinal stem cell priming by degrading LGR5 receptor, EMBO J., 2020, 39, e102771 CrossRef CAS PubMed.
- C. Leung, S. H. Tan and N. Barker, Recent Advances in Lgr5(+) Stem Cell Research, Trends Cell Biol., 2018, 28, 380–391 CrossRef CAS PubMed.
- T. Takahashi, Roles of nAChR and Wnt signaling in intestinal stem cell function and inflammation, Int. Immunopharmacol., 2020, 81, 106260 CrossRef CAS PubMed.
- N. Asano, A. Takeuchi, A. Imatani, M. Saito, X. Jin, W. Hatta, K. Uno, T. Koike and A. Masamune, Wnt Signaling and Aging of the Gastrointestinal Tract, Int. J. Mol. Sci., 2022, 23, 12210 CrossRef CAS PubMed.
- M. Quiros, H. Nishio, P. A. Neumann, D. Siuda, J. C. Brazil, V. Azcutia, R. Hilgarth, M. N. O'Leary, V. Garcia-Hernandez, G. Leoni, M. Feng, G. Bernal, H. Williams, P. H. Dedhia, C. Gerner-Smidt, J. Spence, C. A. Parkos, T. L. Denning and A. Nusrat, Macrophage-derived IL-10 mediates mucosal repair by epithelial WISP-1 signaling, J. Clin. Invest., 2017, 127, 3510–3520 CrossRef PubMed.
- M. Igarashi and L. Guarente, mTORC1 and SIRT1 Cooperate to Foster Expansion of Gut Adult Stem Cells during Calorie Restriction, Cell, 2016, 166, 436–450 CrossRef CAS PubMed.
- H. J. Zapata and V. J. Quagliarello, The microbiota and microbiome in aging: potential implications in health and age-related diseases, J. Am. Geriatr. Soc., 2015, 63, 776–781 CrossRef PubMed.
- M. A. Jackson, I. B. Jeffery, M. Beaumont, J. T. Bell, A. G. Clark, R. E. Ley, P. W. O'Toole, T. D. Spector and C. J. Steves, Signatures of early frailty in the gut microbiota, Genome Med., 2016, 8, 8 CrossRef PubMed.
- N. H. Rashidah, S. M. Lim, C. F. Neoh, A. B. A. Majeed, M. P. Tan, H. M. Khor, A. H. Tan, S. H. Rehiman and K. Ramasamy, Differential gut microbiota and intestinal permeability between frail and healthy older adults: A systematic review, Ageing Res. Rev., 2022, 82, 101744 CrossRef PubMed.
- E. Biagi, L. Nylund, M. Candela, R. Ostan, L. Bucci, E. Pini, J. Nikkïla, D. Monti, R. Satokari, C. Franceschi, P. Brigidi and W. De Vos, Through ageing, and beyond: gut microbiota and inflammatory status in seniors and centenarians, PLoS One, 2010, 5, e10667 CrossRef PubMed.
- V. Palmas, S. Pisanu, V. Madau, E. Casula, A. Deledda, R. Cusano, P. Uva, A. Loviselli, F. Velluzzi and A. Manzin, Gut Microbiota Markers and Dietary Habits Associated with Extreme Longevity in Healthy Sardinian Centenarians, Nutrients, 2022, 14, 2436 CrossRef CAS PubMed.
- J. Ma, Z. Liu, X. Gao, Y. Bao, Y. Hong, X. He, W. Zhu, Y. Li, W. Huang, N. Zheng, L. Sheng, B. Zhou, H. Chen and H. Li, Gut microbiota remodeling improves natural aging-related disorders through Akkermansia muciniphila and its derived acetic acid, Pharmacol. Res., 2023, 189, 106687 CrossRef CAS PubMed.
- M. Tatsuoka, R. Shimada, F. Ohsaka and K. Sonoyama, Administration of Bifidobacterium pseudolongum suppresses the increase of colonic serotonin and alleviates symptoms in dextran sodium sulfate-induced colitis in mice, Biosci. Microbiota. Food Health, 2023, 42, 186–194 CrossRef CAS PubMed.
- Q. Song, X. Zhang, W. Liu, H. Wei, W. Liang, Y. Zhou, Y. Ding, F. Ji, A. Ho-Kwan Cheung, N. Wong and J. Yu, Bifidobacterium pseudolongum-generated acetate suppresses non-alcoholic fatty liver disease-associated hepatocellular carcinoma, J. Hepatol., 2023, 79, 1352–1365 CrossRef CAS PubMed.
- J. Niu, M. Cui, X. Yang, J. Li, Y. Yao, Q. Guo, A. Lu, X. Qi, D. Zhou, C. Zhang, L. Zhao and G. Meng, Microbiota-derived acetate enhances host antiviral response via NLRP3, Nat. Commun., 2023, 14, 642 CrossRef CAS PubMed.
- L. F. Mager, R. Burkhard, N. Pett, N. C. A. Cooke, K. Brown, H. Ramay, S. Paik, J. Stagg, R. A. Groves, M. Gallo, I. A. Lewis, M. B. Geuking and K. D. McCoy, Microbiome-derived inosine modulates response to checkpoint inhibitor immunotherapy, Science, 2020, 369, 1481–1489 CrossRef CAS PubMed.
- M. J. Claesson, I. B. Jeffery, S. Conde, S. E. Power, E. M. O'Connor, S. Cusack, H. M. Harris, M. Coakley, B. Lakshminarayanan, O. O'Sullivan, G. F. Fitzgerald, J. Deane, M. O'Connor, N. Harnedy, K. O'Connor, D. O'Mahony, D. van Sinderen, M. Wallace, L. Brennan, C. Stanton, J. R. Marchesi, A. P. Fitzgerald, F. Shanahan, C. Hill, R. P. Ross and P. W. O'Toole, Gut microbiota composition correlates with diet and health in the elderly, Nature, 2012, 488, 178–184 CrossRef CAS PubMed.
- K. Glunde, Z. M. Bhujwalla and S. M. Ronen, Choline metabolism in malignant transformation, Nat. Rev. Cancer, 2011, 11, 835–848 CrossRef CAS PubMed.
- J. Li, X. Liao, X. Yin, Z. Deng, G. Hu, W. Zhang, F. Jiang and L. Zhao, Gut Microbiome and Serum Metabolome Profiles of Capsaicin with Cognitive Benefits in APP/PS1 Mice, Nutrients, 2022, 15, 118 CrossRef PubMed.
- N. Son, H. J. Hur, M. J. Sung, M. S. Kim, J. T. Hwang, J. H. Park, H. J. Yang, D. Y. Kwon, S. H. Yoon, H. Y. Chung and H. J. Kim, Liquid chromatography-mass spectrometry-based metabolomic analysis of livers from aged rats, J. Proteome Res., 2012, 11, 2551–2558 CrossRef CAS PubMed.
- C. Pontoizeau, L. Mouchiroud, L. Molin, A. Mergoud-Dit-Lamarche, N. Dallière, P. Toulhoat, B. Elena-Herrmann and F. Solari, Metabolomics analysis uncovers that dietary restriction buffers metabolic changes associated with aging in Caenorhabditis elegans, J. Proteome Res., 2014, 13, 2910–2919 CrossRef CAS PubMed.
- V. Gonzalez-Covarrubias, Lipidomics in longevity and healthy aging, Biogerontology, 2013, 14, 663–672 CrossRef CAS PubMed.
- A. Wijeyesekera, C. Selman, R. H. Barton, E. Holmes, J. K. Nicholson and D. J. Withers, Metabotyping of long-lived mice using 1H NMR spectroscopy, J. Proteome Res., 2012, 11, 2224–2235 CrossRef CAS PubMed.
- C. Y. Ewald, Drug Screening Implicates Chondroitin Sulfate as a Potential Longevity Pill, Front. Aging, 2021, 2, 741843 CrossRef PubMed.
- Q. Wang, S. Q. Huang, C. Q. Li, Q. Xu and Q. P. Zeng, Akkermansia muciniphila May Determine Chondroitin Sulfate Ameliorating or Aggravating Osteoarthritis, Front. Microbiol, 2017, 8, 1955 CrossRef PubMed.
- I. Shats, J. G. Williams, J. Liu, M. V. Makarov, X. Wu, F. B. Lih, L. J. Deterding, C. Lim, X. Xu, T. A. Randall, E. Lee, W. Li, W. Fan, J. L. Li, M. Sokolsky, A. V. Kabanov, L. Li, M. E. Migaud, J. W. Locasale and X. Li, Bacteria Boost Mammalian Host NAD Metabolism by Engaging the Deamidated Biosynthesis Pathway, Cell Metab., 2020, 31, 564–579 CrossRef CAS PubMed.
- E. Morselli, M. C. Maiuri, M. Markaki, E. Megalou, A. Pasparaki, K. Palikaras, A. Criollo, L. Galluzzi, S. A. Malik, I. Vitale, M. Michaud, F. Madeo, N. Tavernarakis and G. Kroemer, The life span-prolonging effect of sirtuin-1 is mediated by autophagy, Autophagy, 2010, 6, 186–188 CrossRef PubMed.
- H. Tai, Z. Wang, H. Gong, X. Han, J. Zhou, X. Wang, X. Wei, Y. Ding, N. Huang, J. Qin, J. Zhang, S. Wang, F. Gao, Z. M. Chrzanowska-Lightowlers, R. Xiang and H. Xiao, Autophagy impairment with lysosomal and mitochondrial dysfunction is an important characteristic of oxidative stress-induced senescence, Autophagy, 2017, 13, 99–113 CrossRef CAS PubMed.
- E. G. Foerster, T. Mukherjee, L. Cabral-Fernandes, J. D. B. Rocha, S. E. Girardin and D. J. Philpott, How autophagy controls the intestinal epithelial barrier, Autophagy, 2022, 18, 86–103 CrossRef CAS PubMed.
- S. Umar, Intestinal stem cells, Curr. Gastroenterol. Rep., 2010, 12, 340–348 CrossRef PubMed.
Footnotes |
† Electronic supplementary information (ESI) available. See DOI: https://doi.org/10.1039/d3fo05221d |
‡ These authors contributed equally to this work and were considered co-first authors. |
|
This journal is © The Royal Society of Chemistry 2024 |