DOI:
10.1039/D3FO03066K
(Paper)
Food Funct., 2024,
15, 676-688
Exopolysaccharide β-(2,6)-levan-type fructans have a molecular-weight-dependent modulatory effect on Toll-like receptor signalling†
Received
26th July 2023
, Accepted 6th December 2023
First published on 18th December 2023
Abstract
Scope: Fructans are a group of dietary fibers which are known to have many beneficial effects including immune-modulating effects. A family of fructans are β-(2,6)-linked levan-type fructans that are known to serve as exopolysaccharides in the cell wall of many species of bacteria including commensal bacteria and probiotics. It is still largely unknown whether and how they can serve as immunomodulating molecules. Results: Microbial β-(2,6)-fructans were found to induce TLR-dependent activation of THP-1 cells, in a dose-dependent fashion. Low molecular weight (Mw), medium Mw and high Mw β-(2,6)-fructans activated both TLR2 and 4 in a dose- and molecular weight-dependent fashion. In addition, it was found that β-(2,6)-fructans were able to inhibit signalling of various TLRs with the strongest effect on TLR5 and 8, which were inhibited by all the β-(2,6)-fructans in a dose- and molecular weight-dependent fashion. The final effect of this activation and inhibition of TLRs on cytokine responses in human dendritic cells (DCs) was minor which may be explained by the counter-activating effects of the different β-(2,6)-linked levan-type fructans on inhibition of TLR signalling in the DCs. Conclusion: A mechanism by which exopolysaccharide levan β-(2,6)-fructans can be immune-modulating is by impacting TLR signalling. This knowledge could lead to food in which exopolysaccharide levan β-(2,6)-fructans are added for preventing disorders where TLR-signalling is modulated.
1. Introduction
Dietary fibers are complex carbohydrates that resist digestion in the upper intestinal tract. During recent years, many beneficial effects for human health have been attributed to enhanced consumption of dietary fibers. For instance, it has been shown that the intake of a fiber-rich diet contributes to better health and lower mortality in individuals suffering from various inflammatory diseases.1–3 Dietary fibers exert their beneficial effect via different routes. One of these is through the stimulation of the growth of beneficial intestinal microbes.4,5 These beneficial microbes can produce fermentation products like short-chain fatty acids (SCFAs) that can positively influence the mucosal immune system and gut barrier function.6 In addition to the microbiota-dependent effects, complex carbohydrates can also directly interact with the intestinal epithelium and immune cells by interacting with their immune receptors such as pattern recognition receptors (PRRs) including Toll-like receptors (TLRs),5,7–9 resulting in immune modulation and thereby influencing intestinal immune responses.
Fructans are a group of complex carbohydrates that are often used as a food supplement to serve as a source of dietary fiber.10 They are made up of D-fructose residues which can be connected via β-(2,1)- or β-(2,6)-linkages to form mostly linear structures.11 Depending on the degree of polymerization, fructans can vary considerably in chain length and molecular weight.11 Fructans with β-(2,1)-linkages are called inulin-type fructans and are found in many plants.11 Fructans with β-(2,6)-linkages are called levan-type fructans and can be found in plants and microbes. Another group of fructans are linked by both β(2 → 1) and β(2 → 6) bonds and are referred to as graminans and are predominantly found in cereals.12–14 Much research has been dedicated to microbiota-stimulating and immune-modulating properties of fructans. For example, Logtenberg et al. showed that inulin-type fructans can stimulate the growth of Bifidobacterium during fermentation with infant microbiota,15 while graminans were shown to have a strong impact on the human gut immune barrier.16–18
Levan-type fructans are the most abundant type of fructans on earth.19 For example, they are produced by a variety of micro-organisms organisms including Gluconobacter albidus, Lactobacillus reuteri, Helicobactoer hepaticus and Bacillus subtilis.11,20 Microbial β-(2,6)-fructans can be found on the outer surface of the bacteria, either loosely bound or secreted and are characterized as exopolysaccharides.11,21 Although less studied than β-(2,1)-inulin-type fructans, microbial β-(2,6)-fructans are also suggested to exert microbiota stimulating and immune-modulating effects.21 For example, microbial β-(2,6)-fructans isolated from B. subtilis were shown to enhance the production of proinflammatory TNF-α and the chemokine IL-8 in human-derived ovarian carcinoma cells.22 Microbial β-(2,6)-fructans isolated from L. reuteri were shown to increase FOXP3+ CD4+ regulatory T-cells in rats,23 and microbial β-(2,6)-fructan isolated from H. hepaticus increased the IL-10/IL-6 ratio in vitro in bone marrow-derived macrophages.24
Although much research has been performed on the possible immunological effects of fructans, the mechanisms underlying their immunomodulatory properties are, especially for β-(2,6)-fructans, less well understood. For β-(2,1)-fructans, several studies have shown that they interact with Toll-like receptors (TLRs), a specific family of pattern recognition receptors (PRRs) present on multiple cell types in the intestine, including epithelial cells and dendritic cells.7 Interaction of β-(2,1)-fructans with TLRs can influence the immunological state of these cells.7 β-(2,1)-fructans were shown to activate multiple TLRs, including TLR2 and TLR4, and the interaction of the fructans with these TLRs was dependent on the molecular weight (Mw) of the fructans.7 Long-chain β-(2,1)-fructans induced a higher activation of TLR2, whereas short-chain β-(2,1)-fructans induced a higher activation of TLR4.7 Unfortunately, not much is known about TLR interaction by microbial β-(2,6)-fructans, however, Xu et al. suggested that β-(2,6)-fructans can also interact with TLR4, as microbial β-(2,6)-fructans isolated from B. subtilis induced Th2 responses in mouse macrophage cells via TLR4 signalling.25 As there is substantial evidence available showing that β-(2,1)-fructans can interact with TLRs, we hypothesized that specific β-(2,6)-fructans could also have distinct effects on TLR signalling.
Therefore, in this study, we investigated whether microbial β-(2,6)-fructans with varying degrees of Mw can activate or inhibit TLR signalling. To this end, we used reporter cell lines expressing different TLRs and incubated them with β-(2,6)-fructans. Subsequently, the β-(2,6)-fructans were tested for their cytokine-inducing effects on dendritic cells. In addition, we studied the effect of β-(2,6)-fructans on an intestinal epithelial cell line, after which the conditioned medium of these cells was incubated with DC to study whether soluble products from these intestinal cells affect DC responses.
2. Materials and methods
2.1 β-Fructans
The low Mw (lMw) β-(2,6)-fructan (P-Levan) was provided by Megazyme (Wicklow, Ireland), and the medium Mw (mMw) β-(2,6)-fructans and high Mw (hMw) β-(2,6)-fructans were kindly provided by Frank Jakob (Technical University of Munich, Germany).
2.2 Molecular weight determination
The molecular weight of the β-fructans was measured by gel permeation chromatography (GPC). The analysis was performed on an Agilent Technologies 1200 Series using three PSS Suprema columns (100, 1000, 3000 Å, 300 × 8 mm × 10 μm), with the temperature set at 40 °C. The eluate was monitored by a refractive index (RI) detector. The mobile phase was 0.05 M NaNO3 at a flow rate of 1 mL min−1. The sample concentration was 2 mg mL−1 and the injection volume was 10 μL. Ethylene glycol 0.5% was used as internal standard. Calibration was performed using a pullulan series (PSS-pulkit-12) with a molecular weight in the range of 1.03–708 kDa.
2.3 HPAEC-PAD analysis
Samples were hydrolyzed using an adapted method based on a previously described procedure.26 In brief, samples were treated with concentrated H2SO4 (1 h, 30 °C) and then hydrolyzed with 2.8 M H2SO4 for 3 h at 100 °C. Samples were diluted 20 times and 10 μL was directly injected for analysis. High-performance anion exchange chromatography (HPAEC) was performed on a Dionex Ultimate 6000 system (Thermo Scientific, Sunnyvale, CA, USA) equipped with a CarboPac PA-1 column (2 mm × 250 mm ID) in combination with a CarboPac PA-1 guard column (2 mm × 50 mm ID) and pulsed amperometric detection (PAD). The system was controlled by Chromeleon 7.2.9 software (Thermo Scientific, Sunnyvale, CA, USA). Elution of monosaccharides was performed at a flow-rate of 0.25 mL min−1 with a multi-step-gradient using the following eluents: A: 0.1 M NaOH, B: 1 M NaOAc in 0.1 M NaOH, C: 0.2 M NaOH, and D: MilliQ water. The gradient used was 16% A, 84% D (20 min), 45% A, 5% B, 50% D (5 min), and 60% A, 40% B (15 min). To regenerate the column, it was flushed for 12 min with 100% C by increasing the flow rate in the first 2 min to 0.35 mL min−1. Finally, the column was equilibrated for 12 min with 16% A, 84% D by decreasing the flow rate in the first 2 min to 0.25 mL min−1.
2.4 Determination of carbohydrate and protein content
The carbohydrate content of the samples was estimated by the phenol-sulphuric acid method, using glucose as a standard.27 For the spectroscopic measurements, the absorbance was measured at 490 nm on an Agilent 8453 UV-Visible Spectrophotometer using a 10 mm quartz cuvette. The protein content of the samples was determined by the Bradford assay, using the Bio-Rad assay reagents (catalogue number 500-0116); bovine serum albumin (BSA) was used to generate a standard curve.
2.5 Culturing of THP-1 and HEK cell lines
To determine the effects of β-fructans on TLR signalling, various THP-1 and HEK reporter cell lines, all purchased from Invivogen (Toulouse, France), were used. These reporter cell lines express Secreted Embryonic Alkaline Phosphatase (SEAP), which is coupled to the nuclear factor κB/Activating protein-1 (NF-κB/AP-1) promotor. Upon activation of the TLRs by a specific agonist, high levels of intracellular NF-κB will lead to the secretion of SEAP which can be quantified.
To assess the TLR dependent effects of β-fructans, two THP-1 acute monocytic leukemia cell lines were cultured; THP1-XBlue™-MD2-CD14, expressing MD2 and CD14 and thus responding to TLR-ligands and the TH1-XBlue™-DefMyD, expressing a truncated, non-functional form of the TLR adaptor Myeloid differentiation primary response gene 88 (MyD88), and therefore unresponsive to TLR activation. Both THP1 cell lines were cultured in RPMI1640 medium (Gibco, Life Technologies, Bleiswijk, The Netherlands), containing 10% heat inactivated FBS (Fetal Bovine Serum, HyClone, Thermo Scientific, Breda, The Netherlands), 2 mM L-glutamine, 1.5 g L−1 sodium bicarbonate (Boom B.V. Meppel, The Netherlands), 4.5 g L−1 glucose, 10 mM HEPES, 1.0 mM sodium pyruvate, 100 μg mL−1 Normocin™ (Invivogen, Toulouse, Fance), and 50 U mL−1 and 50 μg mL−1 Penicillin/Streptomycin. All additives were purchased from Sigma Aldrich (Zwijndrecht), unless indicated otherwise.
To investigate signalling via individual TLRs, 7 human embryonic kidney (HEK) 293 cell lines (HEK-Blue™-hTLRX) were used, each containing an inserted construct of either human TLR2, 3, 4, 5, 7, 8 or 9. The HEK-Blue-hTLR2 cell line, also expresses TLR1 and TLR6. The HEK cells were cultured in DMEM medium (Gibco, Life Technologies, Bleiswijk, The Netherlands), containing 10% heat inactivated FBS, 2 mM L-glutamine, 4.5 g L−1 glucose, 50 U mL−1 and 50 mg mL−1 penicillin/streptomycin and 100 mg mL−1 Normocin. All reporter cell lines were cultured for 3 passages before they were maintained in cell medium containing selective antibiotics, as described before.28
2.6 Reporter cell line stimulation and inhibition assays and Quanti-blue analysis
THP-1 and HEK cells were seeded into a flat-bottom 96-well plate at a cell density following the manufacturer's protocol (ESI Table 1†). To determine the activation of TLR by β-fructans, cells were stimulated for 24 hours (37 °C, 5% CO2) with either 0.5, 1 or 2 mg mL−1 β-fructan or the relevant ligand as a positive control (ESI Table 1†). Although all samples tested negative for LPS, we still excluded activation of TLR4 due to contamination with LPS by the addition of 100 μg mL−1 polymyxin B to all samples apart from the agonist. For inhibitory properties of β-fructans on the TLRs, cells were stimulated for 1 hour with different concentrations of β-fructans, before exposing cells to the appropriate agonist for 24 hours.
After activation or inhibition, 20 μL of supernatant was mixed with 180 μL of Quanti-Blue in a flat bottom 96-well plate. The plate was incubated for 1 hour (37 °C, 5% CO2) before measuring the absorbance (655 nm) using a VersaMax microplate reader (Molecular Devices GmbH, Biberach an der Riss, Germany) to determine SEAP activity, which represents activation of NF-κB/AP-1. Results are displayed as fold-change compared to the negative control or positive control, for TLR activation and inhibition respectively. As all experiments were done in triplicate and repeated at least 5 times, the mean of the positive or negative controls within each separate experiment was set to 1.
2.7 Stimulation of dendritic cells with β-fructans and Caco-spent medium
2.7.1 Cell culture and stimulation.
Dendritic cells (DCs) generated from umbilical cord blood CD34 + progenitor cells (hematopoietic stem cells) were purchased from MatTek Corporation (Ashland, MA, USA). DCs were thawed and seeded into 96-well plates at a density of 7 × 104 cells per well and cultured under normal conditions (37 °C, 21% O2 and 5% CO2) for 24 h according to manufacturer's instructions. After 24 h of culturing, cells were attached to the culture plate after which the medium could be replaced.
To investigate direct stimulation of DCs by β-fructans, DCs were incubated with 200 μL per well DC-MM culture medium (Ashland, MA, USA) containing 0.5 mg mL−1 of β-fructans for 48 h. This concentration was selected as the lowest concentration that showed interaction with TLRs. After incubation, supernatants were collected and stored at −20 °C until further analysis. All experiments were repeated five times.
To determine whether intestinal epithelial cells produce soluble signals that may skew the response of DCs, we stimulated intestinal epithelial cells with β-fructans and collected the medium as has been described before.29 This Caco-spent medium (CSM) was then used to stimulated DCs. In order to obtain this CSM, Caco-2 cells were seeded in a Transwell system (0.33 cm2, pore size 0.4 μm; Corning, Kennebunk, USA) at a density of 20
000 cells per well and cultured under normal conditions for 21 days to reach a stable trans-epithelial electrical resistance of >400 Ohm cm2. Medium was changed every second day. Caco-2 cells were then incubated with 10 mg mL−1 β-fructans on the apical site for 24 hours, after which the basolateral medium was collected and stored at −80 °C (ESI Fig. 1†). The Caco-SM was diluted in DC-MM culture medium at a ratio of 1
:
10. DCs were then incubated with 200 μL per well for 48 hours. After incubation, supernatants were collected and stored at −20 °C until further analysis. All experiments were repeated five times.
2.7.2 Assessment of cytokine production.
A magnetic Luminex Assay (R&D systems, Bio-Techne, Minneapolis, USA) was used to determine the levels of MCP-1/CLL2, MIP-1α/CCL3, IL-1β, IL-6, TNFα and IL-10 in the DC supernatant. The assay was performed according to manufacturer's protocol. In brief, cytokine standards were resuspended and serial dilutions were prepared. An antibody magnetic bead mix was added into the wells of a 96-well plate. Standards and samples were added and incubated overnight at 4 °C while shaking. After washing the plate three times, detection antibodies were added and incubated for 30 min at RT while shaking. After incubation, the plate was washed again and incubated with streptavidin-PE for 30 min at RT while shaking. Finally, after the plate was washed again, 100 μL of wash buffer was added to each well. Subsequently, the plate was analyzed using a Luminex 200 System. The data obtained were analyzed using the Luminex xPONENT software. Data were transformed to relative values of the control, which was set to 1.
2.8 Statistical analysis
All statistical tests were performed using Prism 9.1.0 software (GraphPad, San Diego, CA, USA). Outliers were removed after testing using a ROUT outlier test (Q = 1%). Normal distribution was tested with the Kolmogorov–Smirnov test (p > 0.05). In case of the TLR data, if normally distributed, statistical significance was determined with a one-way ANOVA with Dunnett's multiple comparisons test. If data was not normally distributed Kruskal–Wallis test was performed with a Dunn's multiple comparisons test.
For the data of the cytokine production of DCs, samples with cytokine levels that were under the detection level of the Luminex kit were interpreted as 1
:
10 of the lower detection level (ESI Table 2†). Again, outliers were removed after testing using a ROUT outlier test (Q = 1%).
The data of cytokine production was normally distributed and analyzed using a One-way ANOVA or a mixed-effects model, with the Geisser-Greenhouse correction, and Dunnett's test (to assess differences between the control and the experimental groups) was used post hoc. Significance was set at p < 0.001***, p < 0.01** and p < 0.05*, trends were set at p < 0.1.
3. Results
3.1 Characterization of the tested β-(2,6)-fructans
As determined by gel permeation chromatography (GPC) mMw and hMw β-(2,6)-fructans have a molecular weight in the gigadalton (109 Da) range (Table 1, ESI Fig. 2†), while the IMw β-(2,6)-fructan has a significantly smaller size of 12.5 kDa [Megazyme 9013-95-0].
Table 1 Structural analysis of the samples used in this study
Sample |
M
w (kDa) distributiona |
Monosaccharide compositionb |
Carbohydrate content estimationc (%) |
Protein contentd (%) |
Gal |
Glc |
Fru |
M
w = Molecular weight as determined by AF4-MALLS (Ua-Arak) and by SEC (Megazyme) and confirmed by GPC (ESI Fig. 2†).
The monosaccharide composition of the β-(2,6) fructans was determined by HPAEC-PAD analysis. Gal = galactose, Glc = glucose, Fru = fructose.
Carbohydrate content was determined using the Dubois method (Dubois et al., 195627). Values are the average of three measurements, using a glucose standard curve as a reference.
Values are the average of three measurements.
M
w average data is taken from the product sheet [Megazyme 9013-95-0].
M
w data is taken from Ua-Arak et al., 2017.
|
lMw β-(2,6)-fructan |
12.5e |
− |
+ |
+ |
68 ± 0.3 |
0 |
mMw β-(2,6)-fructan |
405.8 × 103 f |
− |
− |
+ |
86 ± 2 |
0 |
hMw β-(2,6)-fructan |
1986.2 × 103 f |
− |
− |
+ |
75 ± 7 |
0 |
The monosaccharide composition of the fructans was analyzed by HPEAC-PAD. lMw β-(2,6)-fructan contained mostly fructose and, in lower amount, glucose, whereas only fructose was detectable for mMw β-(2,6)-fructan and hMw β-(2,6)-fructan (Table 1).
The carbohydrate content was estimated using the Dubois method27 and was 68%, 86% and 75% for lMw β-(2,6)-fructan, mMw β-(2,6)-fructan, and hMw β-(2,6)-fructan respectively (Table 1).
3.2 TLR-induced activation of NF-κB/AP-1 in THP-1 cell lines by β-(2,6)-fructans is dependent on the presence of the TLR adapter MyD88
To investigate the involvement of TLRs in the immune modulatory properties of β-(2,6)-fructans, it was first determined whether the β-(2,6)-fructans can activate THP1-MD2 CD14 cells. These cells express all TLRs. To this end, THP1-MD2 CD14 cells were exposed to 0.5 mg mL−1, 1 mg mL−1 and 2 mg mL−1 of either type of β-(2,6)-fructans. These concentrations were similar to those used in previous TLR studies.7 NF-κB/AP-1 expression was determined as a measure for TLR activation (Fig. 1a).
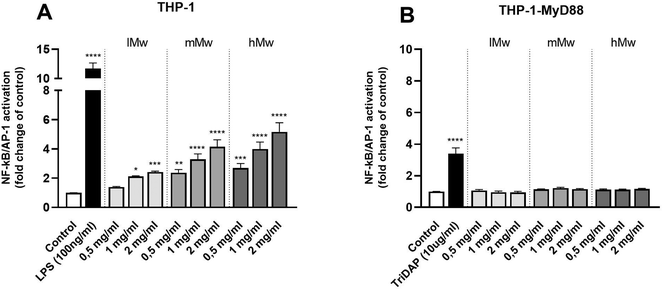 |
| Fig. 1 NF-κB/AP-1 activation in THP-1 MD2-CD14 (A) and THP-1 defMyD88 reporter cells (B) by β-(2,6)-fructans. Cells were incubated with 0.5, 1 or 2 mg mL−1 β-fructan. Data are expressed as mean ± standard error of the mean (SEM) (n = 5). For normally distributed data, statistical significance was determined using a One-way ANOVA test with a Dunnett's multiple comparisons post-test. For not-normally distributed data, a Kruskal–Wallis test followed by a Dunn's multiple comparisons test post hoc was done (*P < 0.05, **p < 0.01, ***p < 0.001, ****p < 0.0001). | |
β-(2,6)-Fructans had a strong TLR-activating effect. All concentrations of lMw, mMw, and hMw β-(2,6)-fructans induced NF-κB/AP-1 expression. We observed a 2.4-fold (p < 0.1), 4.1-fold (p < 0.0001) and 5.1-fold increase (p < 0.0001) for the 2 mg mL−1 concentrations compared to unstimulated control for lMW, mMw and hMw respectively. The induction of NFκB/AP-1 expression by lMw, mMw and hMw β-(2,6)-fructans was concentration dependent (Fig. 1a).
To determine whether the observed activation of NF-κB/AP-1 was TLR dependent, the β-(2,6)-fructans were also tested on THP-1 myD88-deficient cells. These cells are deficient in MyD88 which is an essential adapter molecule for TLR2, 4, 5, 7, 8, and 9 signalling. None of the β-fructan structures were able to induce a statistically significant activation of these cells illustrating the TLR dependency of NFκB/AP-1 activation (Fig. 1b).
3.3 β-(2,6)-Fructans activate TLR2 and 4 in a dose- and molecular weight-dependent fashion
Next, β-fructans were tested for their ability to activate or inhibit specific TLRs. To this end, HEK293 cells expressing either human TLR2, in combination with TLR1 and TLR6, or TLR3, 4, 5, 7, 8, or 9 as single TLR were stimulated with the β-fructans. The NFκB/AP-1 expression was determined and expressed as fold changes compared to the negative control.
The different β-(2,6)-fructans used in this study (lMw, mMw and hMw) all induced significant activation of TLR2 and 4 in a dose- and molecular weight-dependent fashion (Fig. 2a and c). For TLR2, the lMw, mMw and hMw β-(2,6)-fructans at a concentration of 2 mg mL−1 induced a 5.6-fold (p < 0.0001), 8.7-fold (p < 0.0001) and a 11.1-fold (p < 0.0001) increase in receptor activation respectively. For TLR4, the lMw, mMw and hMw β-(2,6)-fructans at a concentration of 2 mg mL−1 induced a 4.3-fold (p < 0.0001), 4.9-fold (p < 0.0001) and a 2.3-fold (p < 0.0001) increase in receptor activation respectively. The other TLRs, TLR3, 5, 7, 8 and 9 were not significantly activated by the β-(2,6)-fructans.
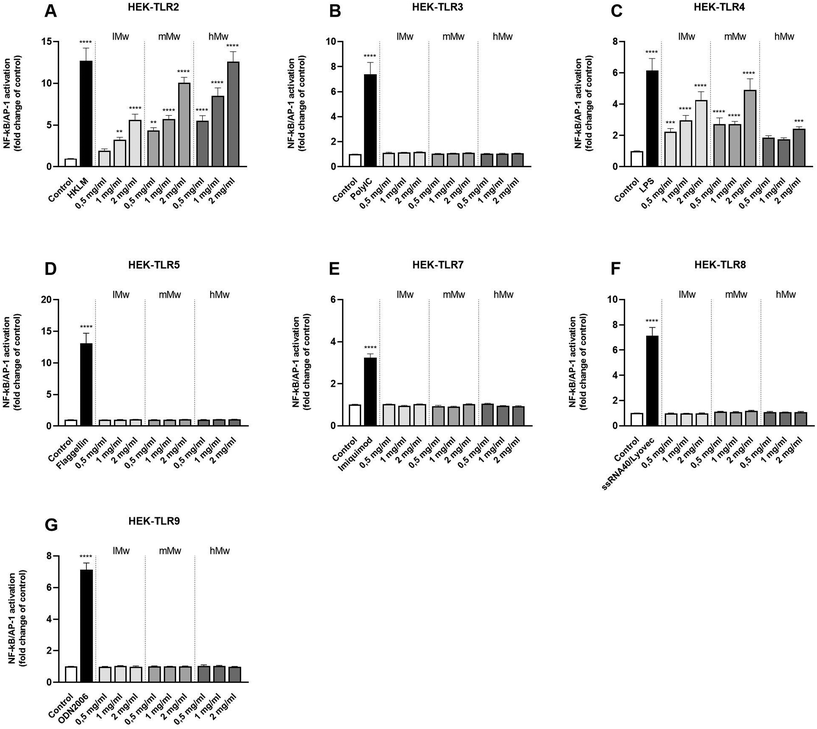 |
| Fig. 2 Activation of HEK reporter cell lines expressing individual TLRs (A–G) by β-(2,6)-fructans. HEK cell lines were stimulated with 0.5, 1 and 2 mg mL−1 β-fructans. Values are expressed as mean ± standard error of the mean (SEM) (n = 5). Statistical significance was determined using a one-way ANOVA with a Dunnet's multiple comparisons test for normally distributed data and a Kruskal–Wallis test with a Dunn's multiple comparisons test for not normally distributed data (**p < 0.01, ***p < 0.001, ****p < 0.0001). | |
3.4 β-(2,6)-Fructans inhibit signalling of various TLRs
To determine the inhibitory properties of β-fructans on TLR signalling, HEK cells were pre-incubated with β-fructans for 1 hour before stimulating them with their respective TLR agonists. Inhibition of β-(2,6)-fructans was quantified by comparing the NF-κB/AP-1 expression in presence of the fructans to the control containing only the agonist (Fig. 3).
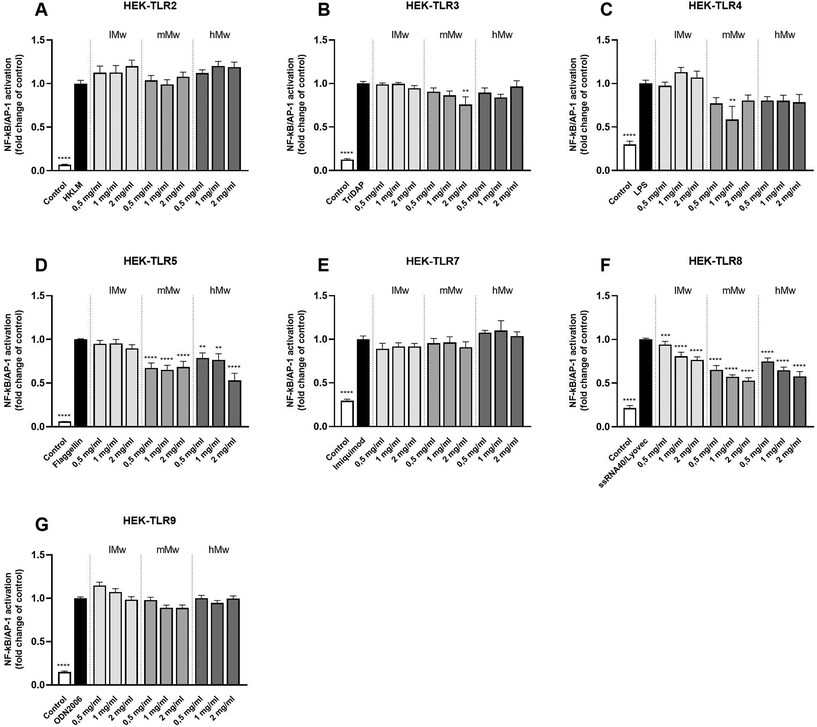 |
| Fig. 3 Inhibition of individual TLRs β-(2,6)-fructans. HEK cell lines expressing individual TLRs (A–G) were pre-incubated with 0.5, 1 or 2 mg mL−1 β-(2,6)-fructans before stimulation with their agonists. Values are expressed as mean ± standard error of the mean (SEM) (n = 3–5). Statistical significance was determined using a one-way ANOVA with a Dunnet's multiple comparisons test for normally distributed data and a Kruskal–Wallis test with a Dunn's multiple comparisons test for not normally distributed data (**p < 0.01, ***p < 0.001, ****p < 0.0001). | |
β-(2,6)-Fructans showed strong inhibitory properties on different TLRs in a Mw dependent fashion. mMw β-(2,6)-fructans, at a concentration of 2 mg mL−1 inhibited TLR3 to 0.76-fold (p < 0.01). The 1 mg mL−1 concentration of the mMw β-(2,6)-fructan also inhibited TLR4 to 0.59-fold (p < 0.01). Also, the mMW and hMw β-(2,6)-fructans inhibited TLR5. This effect was seen for all concentrations. The 2 mg mL−1 concentrations of the mMw and hMw β-(2,6)-fructans inhibited TLR5 up to 0.68-fold (p < 0.0001) and 0.53-fold (p < 0.0001) respectively. TLR8 was inhibited by all concentrations of the lMw, mMw, hMw β-(2,6)-fructans up to a 0.77-fold (p < 0.0001), 0.53-fold (p < 0.0001) and 0.58-fold (p < 0.0001) respectively.
3.5 Stimulation of dendritic cell with β-(2,6)-fructans directly or indirectly and induced cytokine production
Dendritic cells are key players in the gut mucosal immune system and are distributed along the intestinal epithelium.30 Therefore, we investigated whether the β-(2,6)-fructans could also influence cytokine production of DCs. To this end, we incubated DCs for 48 h in the presence and absence of the β-fructans and determined cytokine release. However, only the production of MIP1α/CCL3 was significantly reduced by the incubation with mMw β-(2,6)-fructans. No further significant differences were observed (Fig. 4).
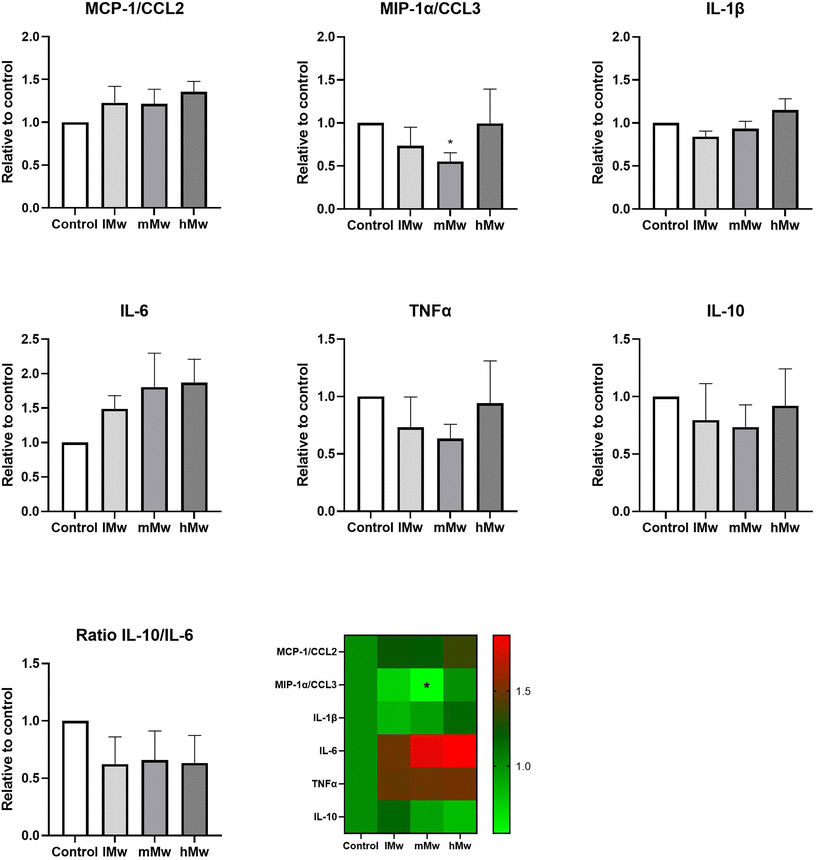 |
| Fig. 4 Cytokine production by dendritic cells incubated with β-(2,6)-fructans. Dendritic cells were incubated with 0.5 mg mL−1 of β-fructans for 48 h. Subsequently, cytokine levels in the supernatant were determined using Luminex. In addition, the ratio for IL-10/IL-6 was determined. Values are expressed as mean ± standard error of the mean (SEM) (n = 5). Statistical significance was determined using a One-way ANOVA test followed by a Dunnett's multiple comparison test. | |
To investigate whether the β-fructans could influence DC cytokine production via intestinal epithelial cell derived factors, DC were incubated with β-(2,1)- and β-(2,6)-fructans Caco-spentd medium (1
:
10 diluted) for 48 h. These incubations also did not induce significant differences in DC cytokine production (Fig. 5).
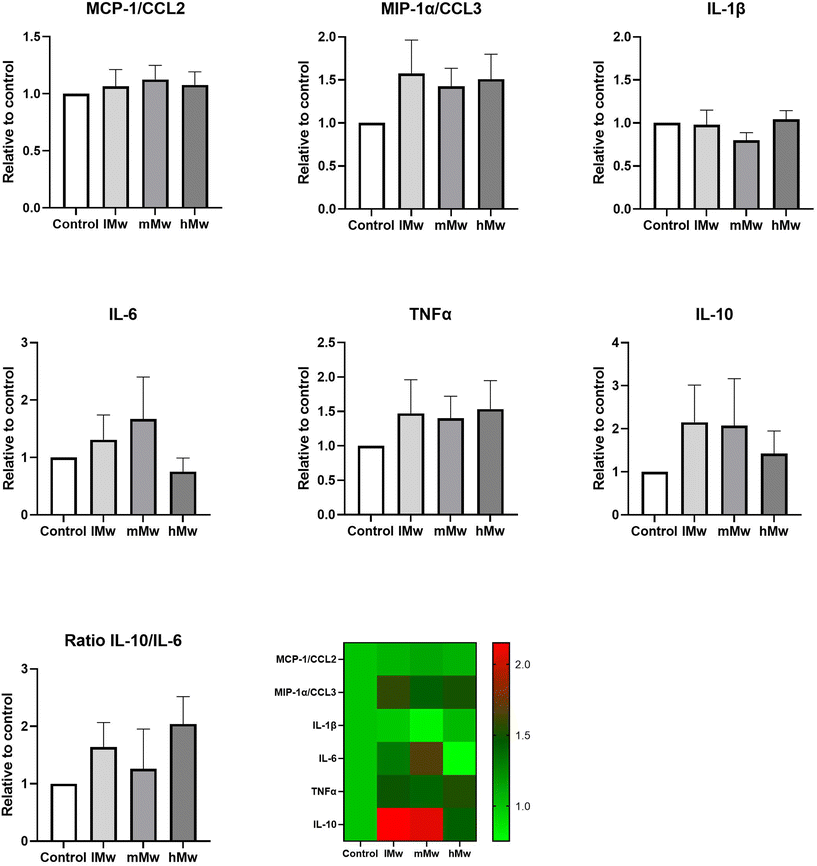 |
| Fig. 5 Cytokine production by dendritic cells incubated with Caco-spent medium of β-(2,6)-fructans. Dendritic cells were incubated with of β-(2,6)-fructans CSM. Values are expressed as mean ± standard error of the mean (SEM) (n = 5). Statistical significance was determined using a mixed effects analysis followed by a Dunnett's multiple comparison test. No significant differences were found. | |
4. Discussion and conclusion
Fructans are complex carbohydrates that exert many beneficial effects on the human health. β-(2,1)-fructans are extensively investigated for their effect on the immune response and have been shown to interact with TLRs to elicit their response.21 Although β-(2,6)-fructans presented similar effects on the immune response in human in vitro studies,22,24,25 the mechanisms underlying the immunomodulatory function of β-(2,6)-fructans are not well understood. Since it was shown that β-(2,1)-fructans elicit their immunomodulatory response through TLR interaction,7,29 we hypothesized that similar mechanisms might be involved in the immunomodulatory effects of β-(2,6)-fructans. Therefore, this study was undertaken to determine the potential immunomodulatory effects of exopolysaccharide β-(2,6)-levan-type fructans and the mechanisms involved.
Levans are the most abundant fructans on earth and are a pertinent component of both plants and microbes that humans consume on a daily basis.11 They are also part of commensal bacteria. Among these are Gluconobacter albidus, Lactobacillus reuteri, Helicobactoer hepaticus, Bacillus subtilis and Bifidobacterium longum,11,20 but also Streptococcus salivarius in the oral cavity,31 and Lactobacillus reuteri.32 The levans on these organisms can be found either in the cytoplasm or integrated into the cell wall as capsular exopolysaccharide.33–35 They can be either attached to other macromolecules in the cell membrane as glycolipid or glycoprotein or be secreted to exert their beneficial effects.21 Many functions have been attributed to exopolysaccharides such as levans which include biological functions such as forming a pertinent anchoring site for commensal bacteria and by that contributing to biofilm formation.36,37
Levan-type fructans have been recognized for their immunomodulatory effect,38–41 but mechanisms are still largely unknown. They have been shown to modulate cytokine production by immune cells in vitro39–42 which suggests direct interaction of fructans with immune cells. During recent years we and other have shown that pattern recognition receptors such as TLRs are important targets for glycosidic structures such as fructan.8,43 Here we show, to the best of our knowledge for the first time, that levan-type β-(2,6)-fructans influence the signalling of specific immune receptors in a molecular weight-dependent and thus structure dependent fashion. We found that levan-type β-(2,6)-fructans have strong stimulating effects for TLR2 and TLR4. More specifically, we observed that the lMw, mMw and hMw β-(2,6)-fructans could stimulate both receptors in a Mw- and concentration-dependent fashion. In addition, we also found that all β-(2,6)-fructans inhibited TLR8 and that mMw and hMW β-(2,6)-fructans inhibited TLR5. As we used specific reporter cell lines, we know these effects are dependent on the NF-κB/AP1 pathway. However, when β-(2,6)-fructans were incubated with DCs, no effect on cytokine secretion was observed.
TLR2 is a rather versatile receptor containing large areas of binding surfaces with many insertions and β-sheets included 19 leucine rich repeats, to which many molecules might bind.28 All three molecular weight β-(2,6)-fructans were able to stimulate TLR2 signalling but this was gradually stronger with the higher molecular weight levans. This could be explained by the clustering of different TLR2 receptors that have a stronger impact when combinedly activated than individual receptors. Similar activation patterns of activation with clustering of receptors have been reported for β-glucans that interact with the PRR Dectin-1.44 This could possibly be further investigated using 3D-modelling techniques, however, at this moment, both the 3D-structure of the ligand binding-site as well as the 3D-structure of the area around the binding-site are still unknown.
The pattern of activation of TLR4 was different from that of TLR2. The two lower molecular weights β-(2,6)-fructans gradually increased activation in a concentration-dependent fashion while it was lower at higher molecular weights. This might be related to the pertinent differences in structure between TLR2 and TLR4. TLR4 contains 22 leucine-rich repeats that are suggested to be responsible for the specificity of ligand binding.45 An important difference with TLR2 is however that the β-sheet of the central domain has smaller radii and larger twist angles.46 Our data suggest that longer molecules might be less readily able to bind to the ligand binding site and might therewith be less immune stimulating. The finding that β-(2,6)-fructans, as exopolysaccharide can activate TLR4, corroborates the findings of Xu et al., who previously demonstrated that β-(2,6)-fructans isolated from B. subtilis exert activating effect on TLR4.25
Besides activation, also inhibition of TLR signalling was observed by β-(2,6)-fructans. Especially β-(2,6)-fructans exhibited strong inhibitory effects on TLR5. TLR5 is composed of a single-domain LRR structure that consists of an N-terminal β-hairpin capping motif and 13 complete LRR modules and two residues from LRR14.47 The concave surface is less regular than other TLRs and has a variation of helices and extended structures and specialized in recognizing flagellin. Little is known about how molecular structures such as mMW and hMW β-(2,6)-fructans are capable of inhibiting TLR5. Many pathogens, including flagellin-carrying organisms, use β-(2,6)-fructans as exopolysaccharides in their cell wall. It is, therefore, possible that the larger structures tested in this study bind to the ligand binding sites and are large enough to interfere with the activation of the receptor by the applied agonist flagellin of S. typhimurium.
Also, the endosomal TLR8 was inhibited by β-(2,6)-fructans. Although intracellularly located this finding might still have biological implications as the gastrointestinal tract is lined with cells that have phagocytic capacity. This includes intraepithelial dendritic cells as well as cells located in the Peyer patches such as M-cells, macrophages, and monocytes. Activation and inhibition of TLR8 activation is a multi-step process. For TLR8 activation the formation of an apo TLR8 dimer is needed and requires a proteolytic cleavage that induces a conformational change of TLR8 when the ligand is bound.48 For activation of TLR8 the ligand, i.e., ssRNA, requires the formation of an activated dimer with the uridine part of ssRNA in the receptor.49,50 This is most likely where the β-(2,6)-fructans interferes with interaction with the receptors with van der Waals bonds and hydrogen bridges as the conformation and ability to bind in the agonist-activated TLR8 is different from that of the inactivated TLR8.43,48,51
Finally, we determined the combined outcome of activation of TLR2 and 4 and inhibition of TLR5 and 8 by incubating the different levans with dendritic cells. Also, TLR8 inhibition might be involved as phagocytosis of the fructans might occur in this set-up. However, only minor changes in cytokine levels were observed. Only for DCs directly stimulated with mMw fructans a significant reduction in MIP1α/CCL3 production was observed compared to the control. CCL3 is a member of the CC chemokine family and involved in the recruitment and activation of polymorphonuclear leukocytes after binding to receptors such as CCR1, CCR4, and CCR5. In addition, some trends towards enhanced MIP1α/CCL3, TNF-α and a molecular weight-dependent lowering of IL-10 were observed for the CSM-stimulated DCs, but there were no statistically significant differences compared to the untreated controls. This is most likely due to the counteracting effects of TLR2 and TLR4 stimulation and the inhibition of TLR5 and 8. Also, the addition of CSM to potentiate the responses of DCs to the stimuli1,8 did not significantly change the cytokine responses of the fructans.
Our study contributes to enhanced knowledge of the mechanisms that might be involved in immunomodulation induced by exopolysaccharides such as β-(2,6)-fructans. The molecules have a molecular weight-dependent effect on TLR signalling. The final effect on DC cytokine responses was a minor change in proinflammatory cytokines likely due to counteracting effects of activation and inhibition of TLRs. This however should not be interpreted as a suggestion that exopolysaccharides cannot be immune modulating. Exopolysaccharides are highly diverse in composition and different results might be expected from exopolysaccharides from other bacterial sources.21 Also, TLR expression is different under diseased and non-diseased conditions which might also impact the biological effects of exopolysaccharides such as of levans. Our study also demonstrates that fructans such as levans can impact TLR signalling just like has been shown for other fructans such as inulin-type and graminan-like fructans. It therewith opens new venues to use β-(2,6)-fructans as an immunomodulating component in disorders where TLR signalling is involved such as in allergies, mucositis and other intestinal disorders.46,52–56
Author contributions
R. A., conceptualization; methodology; formal analysis; writing – original draft. M. M. P. O., conceptualization; methodology; investigation; writing – original draft. M. F., conceptualization; methodology; investigation; writing – original draft. C. F., investigation; writing – review & editing. B. J. H., investigation; writing – review & editing. M. M. F., investigation; writing – review & editing; supervision. M. T. C. W., conceptualization; resources; writing – original draft; supervision; funding acquisition. P. V., conceptualization; resources; writing – original draft; supervision; funding acquisition.
Conflicts of interest
The authors declare no conflict of interest.
Acknowledgements
This research was performed in the public-private partnership ‘CarboBiotics’ coordinated by the Carbohydrate Competence Center (CCC, https://www.cccresearch.nl). CarboBiotics is jointly financed by participating industrial partners Cooperatie Avebe U.A., FrieslandCampina Nederland B.V., Nutrition Sciences N.V., and allowances of The Dutch Research Council (NWO).
References
- M. Bermudez-Brito, M. M. Faas and P. de Vos, Modulation of dendritic-epithelial cell responses against Sphingomonas Paucimobilis, by dietary fibers, Sci. Rep., 2016, 6, 30277 CrossRef.
- R. Fernandes, V. A. do Rosario, M. C. Mocellin, M. G. Kuntz and E. B. S. M. Trindade, Effects of inulin-type fructans, galacto-oligosaccharides and related synbiotics on inflammatory markers in adult patients with overweight or obesity: a systematic review, Clin. Nutr., 2017, 36(5), 1197–1206 CrossRef PubMed.
- B. Wilson and K. Whelan, Prebiotic inulin-type fructans and galacto-oligosaccharides: definition, specificity, function and application in gastrointestinal disorders, J. Gastroenterol. Hepatol., 2017, 32, 64–68 CrossRef CAS.
- P. Cronin, S. A. Joyce, P. W. O'Toole and E. M. O'Connor, Dietary fibre modulates the gut microbiota, Nutrients, 2021, 13(5), 1655 CrossRef CAS PubMed.
- R. Akkerman, M. M. Faas and P. de Vos, Non-digestible carbohydrates in infant formula as substitution for human milk oligosaccharides: effects on microbiota and gut maturation, Crit. Rev. Food Sci. Nutr., 2018, 59(3), 1486–1497 Search PubMed.
- D. P. Venegas, M. K. De la Fuente, G. Landskron, M. J. González, R. Quera, G. Dijkstra, H. J. M. Harmsen, K. N. Faber and M. A. Hermoso, Short chain fatty acids (SCFAs)-mediated gut epithelial and immune regulation and its relevance for inflammatory bowel disease, Front. Immunol., 2019, 10, 277 CrossRef CAS.
- L. Vogt, U. Ramasamy, D. Meyer, G. Pullens, K. Venema, M. M. Faas, H. A. Schols and P. de Vos, Immune modulation by different types of β2–1-fructans is Toll-like receptor dependent, PLoS One, 2013, 8(7), e68367 CrossRef.
- M. Bermudez-Brito, N. M. Sahasrabudhe, C. Rösch, H. A. Schols, M. M. Faas and P. de Vos, Resistant starches differentially stimulate Toll-like receptors and attenuate proinflammatory cytokines in dendritic cells by modulation of intestinal epithelial cells, Mol. Nutr. Food Res., 2015, 59, 1–13 Search PubMed.
- N. M. Sahasrabudhe, J. Dokter-Fokkens and P. de Vos, Particulate β-glucans synergistically activate TLR4 and Dectin-1 in human dendritic cells, Mol. Nutr. Food Res., 2016, 60(11), 2514–2522 CrossRef PubMed.
- D. L. Ackerman, K. M. Craft and S. D. Townsend, Infant food applications of complex carbohydrates: structure, synthesis, and function, Carbohydr. Res., 2017, 437, 16–27 CrossRef PubMed.
- I. D. Young, D. Latousakis and N. Juge, The immunomodulatory properties of β-2,6 fructans: a comprehensive review, Nutrients, 2021, 13(4), 1309 CrossRef PubMed.
- M. G. López, N. A. Mancilla-Maraali and G. Mendoza-Diaz, Molecular structures of fructans from Agave tequilana Weber var. azul, J. Agric. Food Chem., 2003, 51, 7835–7840 CrossRef.
- N. A. Mancilla-Maraali and M. G. Lopez, Water-soluble carbohydrates and fructan structure patterns from Agave and Dasylirion species, J. Agric. Food Chem., 2006, 54, 7832–7839 CrossRef PubMed.
- W. Praznik, R. Lóppert, J. M. C. Rubio, K. Zangger and A. Huber, Structure of fructo-oligosaccharides from leaves and stem of Agave tequilana Weber, var. azul, Carbohydr. Res., 2013, 381, 64–73 CrossRef PubMed.
- M. J. Logtenberg, R. Akkerman, R. An, G. D. A. Hermes, B. J. de Haan, M. M. Faas, E. G. Zoetendal, H. A. Schols and P. de Vos, Fermentation of chicory fructo-oligosaccharides and native inulin by infant fecal microbiota attenuates pro-inflammatory responses in immature dendritic cells in an infant-age-dependent and fructan-specific way, Mol. Nutr. Food Res., 2020, 64(13), 2000068 CrossRef PubMed.
- C. Fernández-Lainez, M. J. Logtenberg, X. Tang, H. A. Schols, G. López-Velázquez and P. de Vos, β(2→1) chicory and β(2→1)-β(2→6) agave fructans protect the human intestinal barrier function in vitro in a stressor-dependent fashion, Food Funct., 2022, 13(12), 6737–6748 RSC.
- C. Fernández-Lainez, R. Akkerman, M. M. P. Oerlemans, M. J. Logtenberg, H. A. Schols, L. A. Silva-Lagos, G. López-Velázquez and P. de Vos, β(2→6)-Type fructans attenuate proinflammatory responses in a structure dependent fashion via Toll-like receptors, Carbohydr. Polym., 2022, 277, 118893 CrossRef PubMed.
- C. Fernández-Lainez, M. aan de Stegge, L. A. Silva-Lagos, G. López-Velázquez and P. de Vos, β(2 → 1)-β(2 → 6) branched graminan-type fructans and β(2 → 1) linear fructans impact mucus-related and endoplasmic reticulum stress-related genes in goblet cells and attenuate inflammatory responses in a fructan dependent fashion, Food Funct., 2023, 14(3), 1338–1348 RSC.
- E. T. Öner, L. Hernández and J. Combie, Review of Levan polysaccharide: from a century of past experiences to future prospects, Biotechnol. Adv., 2016, 34(5), 827–844 CrossRef PubMed.
- R. Srikanth, C. H. S. S. Sundhar Reddy, G. Siddartha, M. J. Ramaiah and K. B. Uppuluri, Review on production, characterization and applications of microbial levan, Carbohydr. Polym., 2015, 120, 102–114 CrossRef PubMed.
- M. M. P. Oerlemans, R. Akkerman, M. Ferrari, M. T. C. Walvoort and P. de Vos, Benefits of bacteria-derived exopolysaccharides on gastrointestinal microbiota, immunity and health, J. Funct. Foods, 2021, 76, 104289 CrossRef.
- A. Magri, M. R. Oliveira, C. Baldo, C. A. Tischer, D. Sartori, M. S. Mantovani and M. A. P. C. Celligoi, Production of fructooligosaccharides by Bacillus subtilis natto CCT7712 and their antiproliferative potential, J. Appl. Microbiol., 2020, 128(5), 1414–1426 CrossRef PubMed.
- I. M. Sims, S. A. Frese, J. Walter, D. Loach, M. Wilson, K. Appleyard, J. Eason, M. Livingston, M. Baird, G. Cook and G. W. Tannock, Structure and functions of exopolysaccharides produced by gut commensal Lactobacillus reuteri 100-23, ISME J., 2011, 5, 1115–1124 CrossRef.
- C. Danne, G. Ryzhkov, M. Martinez-López, N. E. Ilott, F. Franchini, F. Cuskin, E. C. Lowe, S. J. Bullers, J. S. C. Artur and F. A. Powrie, A large polysaccharide produced by Helicobacter hepaticus induces an anti-inflammatory gene signature in macrophages, Cell Host Microbe, 2017, 22(6), 733–745 CrossRef PubMed.
- Q. Xu, T. Yajima, W. Li, K. Saito, Y. Ohshima and Y. Yoshikai, Levan (beta-2,6-fructan), a major fraction of fermented soybean mucilage, displays immunostimulating properties via Toll-like receptor 4 signalling: Induction of interleukin-12 production and suppression of T-helper type 2 response and immunoglobulin E production, Clin. Exp. Allergy, 2006, 36, 94–101 CrossRef PubMed.
- E. Jurak, M. A. Kabel and H. Gruppen, Carbohydrate composition of compost during composting and mycelium growth of Agaricus bisporus, Carbohydr. Polym., 2014, 101, 281–288 CrossRef.
- M. Dubois, K. A. Gilles, J. K. Hamilton, P. A. Rebers and F. Smith, Colorimetric method for determination of sugars and related substances, Anal. Chem., 1956, 28, 350–356 CrossRef.
- M. B. G. Kiewiet, R. Dekkers, M. Gros, R. J. J. van Neerven, A. Groeneveld, P. de Vos and M. M. Faas, Toll-like receptor mediated activation is possibly involved in immunoregulating properties of cow's milk hydrolysates, PLoS One, 2017, 12(6), e0178191 CrossRef.
- A. F. P. Lépine, R. H. J. de Hilster, H. Leemhuis, L. Oudhuis, P. L. Buwalda and P. de Vos, Higher chain lenght distribution in debrached type-3 resistant starches (RS3) increases TLR signaling and supports dendritic cell cytokine production, Mol. Nutr. Food Res., 2019, 63, e1801007 CrossRef PubMed.
- H. Tezuka and T. Ohteki, Regulation of IgA production by intestinal dendritic cells and related cells, Front. Immunol., 1891, 2019, 10 Search PubMed.
- G. Begić, I. J. Badovinac, L. Karleuša, K. Kralik, O. C. Peloza, D. Kuiš and I. Gobin, Streptococcus salivarius as an important factor in dental biofilm homeostasis: influence on Streptococcus mutans, and Aggregatibacter actinomycetemcomitans in mixed biofilm, Int. J. Mol. Sci., 2023, 24(8), 7249 CrossRef.
- S. A. F. T. Van Hijum, K. Bonting, M. J. E. C. van der Maarel and L. Dijkhuizen, Purification of a novel fructosyltransferase from Lactobacillus reuteri, strain 121 and characterization of the levan produced, FEMS Microbiol. Lett., 2001, 205(2), 323–328 CrossRef.
- N. T. Porter and E. C. Martens, The critical roles of polysaccharides in gut microbial ecology and physiology, Annu. Rev. Microbiol., 2017, 71, 349–369 CrossRef PubMed.
- C. P. Neff, M. E. Rhodes, K. Arnolds, C. B. Collins, J. Donnelly, N. Nusbacher, P. Jedlicka, J. M. Schneider, M. D. McCarter, M. Shaffer, S. K. Mazmanian, B. E. Palmer and C. A. Lozupone, Diverse intestinal bacteria contain putative zwitterionic capsular polysaccharides with anti-inflammatory properties, Cell Host Microbe, 2016, 20(4), 535–547 CrossRef.
- B. F. Cress, J. A. Englaender, W. He, D. Kasper, R. J. Linhardt and M. A. G. Koffas, Masquerading microbial pathogens: capsular polysaccharides mimic host-tissue molecules, FEMS Microbiol. Rev., 2014, 38(4), 660–697 CrossRef.
- H. C. Flemming and J. Wingender, The biofilm matrix, Nat. Rev. Microbiol., 2010, 8, 623–633 CrossRef PubMed.
-
P. Gupta, P. A. Pruthi and V. Pruthi, Introduction to biofilm engineering, in Role of exopolysaccharides in biofilm formation, ACS Publications, Washington, DC, USA, 2019, pp. 17–57 Search PubMed.
- S. S. Ferreira, C. P. Passos, P. Madureira, M. Vilanova and M. A. Coimbra, Structure-function relationships of immunostimulatory polysaccharides: a review, Carbohydr. Polym., 2015, 132, 378–396 CrossRef.
- M. Meijerink, C. Rösch, N. Taverne, K. Venema, H. Gruppen, H. A. Schols and J. M. Wells, Structure dependent immunomodulation by sugar beet arabinans via a SYK tyrosine kinase-dependent singaling pathway, Front. Immunol., 2018, 9, 1972 CrossRef PubMed.
- C. Hidalgo-Cantabrana, P. López, M. Gueimonde, C. G. de los Reyes-Gavilán, A. Suárez, A. Margolles and P. Ruas-Madiedo, Immune modulation capability of exopolysaccharides synthesized by lactic acid bacteria and Bifidobacteria, Probiotics Antimicrob. Proteins, 2012, 4, 227–237 CrossRef CAS PubMed.
- M. Yin, Y. Zhang and H. Li, Advances in research on immunoregulation of macrophages by plant polysaccharides, Front. Immunol., 2019, 10, 145 CrossRef CAS.
- N. Castro-Bravo, J. M. Wells, A. Margolles and P. Ruas-Madiedo, Interactions of surface exopolysaccharides from Bifidobacterium and lactobacillus within the intestinal environment, Front. Microbiol., 2018, 9, 2426 CrossRef.
- S. Figuearo-Lozano, R. L. Valk-Weeber, S. S. Van Leeuwen, L. Dijkhuizen and P. de Vos, Dietary N-glycans from bovine lactoferrin and TLR modulation, Mol. Nutr. Food Res., 2018, 62(2), 1700389 CrossRef PubMed.
- N. M. Sahasrabudhe, H. A. Schols, M. M. Faas and P. de Vos, Arabinoxylan activates Dectin-1 and modulates particulate beta-glucan induced Dectin-1 activation, Mol. Nutr. Food Res., 2015, 60, 458–467 CrossRef PubMed.
- H. Tsukamoto, I. Ukai, Y. Yamagata, S. Takeuchi, K. Kubota, S. Kozakai, N. Suzuki, M. Kimoto and Y. Tomioka, Leucine-rich repeat 2 of human Toll-like receptor 4 contains the binding site for inhibitory monoclonal antibodies, FEBS Lett., 3893–3898, 2015, 589 Search PubMed.
- J. Y. Kim, S. M. Seo, H. W. Kim, W. J. Lee and Y. K. Choi, Protective role of the Toll-like receptor 5 agonist KMRC011 against murine colitis induced by Citrobacter rodentium and dextran sulfate sodium, J. Microbiol. Biotechnol., 2023, 33(1), 35–42 CrossRef CAS.
- S. I. Yoon, O. Kurnasov, V. Natarajan, M. Hong, A. V. Gudkov, A. L. Osterman and I. A. Wilson, Structural basis of TLR5-flagellin recognition and signaling, Science, 2012, 335(6070), 859–864 CrossRef CAS PubMed.
- M. Geyer, K. Pelka and E. Latz, Synergistic activation of Toll-like receptor 8 by two RNA degradation products, Nat. Struct. Mol. Biol., 2015, 22(2), 99–101 CrossRef CAS PubMed.
- I. Botos, D. M. Segal and D. R. Davies, The structural biology of toll-like receptors, Structure, 2011, 19(4), 447–459 CrossRef CAS PubMed.
- H. Tanji, U. Ohto, T. Shibata, M. Taoka, Y. Yamauchi, T. Isobe, K. Miyake and T. Shimizu, Toll-like receptor 8 senses degradation products of single stranded RNA, Nat. Struct. Mol. Biol., 2015, 22(2), 109–115 CrossRef CAS.
- Q. Yin, T. Fu, J. Li and H. Wu, Structural biology of innate immunity, Annu. Rev. Immunol., 2015, 33, 393–416 CrossRef CAS PubMed.
- Y. Zhao and R. Li, HMGB1 is a promising therapeutic target for asthma, Cytokine, 2023, 165, 156171 CrossRef CAS.
- M. Liu, F. Liu, Y. Pan, Y. Xiong, X. Zeng, L. Zheng, H. Zhao, Y. Li and D. Liu, Oxymartrine ameliorated experimental colitis via mechanisms involving inflammatory DCs, gut microbiota and TLR/NF-κB pathway, Int. Immunopharmacol., 2023, 115, 109612 CrossRef CAS PubMed.
- M. Liu, S. Thijssen, W. E. Hennink, J. Garssen, C. F. van Nostrum and L. E. M. Willemsen, Oral pretreatment with β-lactoglobulin derived peptide and CpG co-encapsulated in PLGA nanoparticles prior to sensitization attenuates cow's milk allergy, Front. Immunol., 2023, 13, 1053107 CrossRef PubMed.
- M. Beukema, É. Jermendi, T. Koster, K. Kitaguchi, B. J. de Haan, M. A. van den Berg, M. M. Faas, H. A. Schols and P. de Vos, Attenuation of doxorubicin-induced small intestinal mucocitis by pectins is dependent on pectin's methyl-ester number and distribution, Mol. Nutr. Food Res., 2021, 65(18), e2100222 CrossRef.
- N. M. Sahasrabudhe, M. Beukema, L. Tian, B. Troost, J. Scholte, E. Bruininx, G. Bruggeman, M. A. van den Berg, A. Scheurink, H. A. Schols, M. M. Faas and P. de Vos, Dietary fiber pectin directly blocks Toll-like receptor 2-1 and prevents doxorubicin-induced ileitis, Front. Immunol., 2018, 9, 383 CrossRef PubMed.
|
This journal is © The Royal Society of Chemistry 2024 |