DOI:
10.1039/D4FB00084F
(Review Article)
Sustainable Food Technol., 2024,
2, 1297-1364
Advancements in sustainable food packaging: from eco-friendly materials to innovative technologies
Received
19th March 2024
, Accepted 30th June 2024
First published on 1st July 2024
Abstract
The demand for sustainable food packaging solutions has escalated in response to growing environmental concerns and consumer preferences for eco-friendly products. This review delves into the realm of sustainable food packaging materials and methods, exploring their necessity, applications, and impact on advancing sustainability goals. The review begins by examining commonly used materials in food packaging and their negative environmental impacts, particularly focusing on issues like pollution and non-biodegradability. It then highlights the urgent need for eco-friendly alternatives, emphasizing the necessity to transition towards sustainable materials to mitigate ecological harm. A historical timeline contextualizes the evolution of food packaging materials, leading into an exploration of various sustainable options, from general examples to advanced technologies like bio-nanocomposites and antimicrobial packaging. Greener fabrication processes and recent advancements in sustainable materials are highlighted, showcasing innovative approaches such as hybrid nanoparticle coatings and multifunctional bio-nanocomposite films. Furthermore, the review discusses the role of chemical methods in improving packaging properties and examines recent developments in sustainable food packaging, including allicin-loaded nanofibrous films and humidity-adjustable gelatin hydrogel films. The concluding remarks emphasize the significance of these advancements in mitigating environmental impact and enhancing food safety, while also outlining future outlooks for continued innovation in sustainable food packaging.
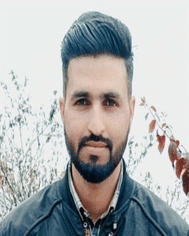 Shokat Hussain | Shokat Hussain completed his BSc from Govt. Post Graduate College Rajouri. He received his MSc in Chemistry from the University of Jammu, BEd from the Central University of Kashmir and BLIS from the IGNOU. Currently, he is pursuing his PhD in Materials Chemistry/Catalysis under the supervision of Dr Shrikant S. Maktedar at Materials Chemistry & Engineering Research Laboratory, Department of Chemistry, National Institute of Technology Srinagar, J&K. |
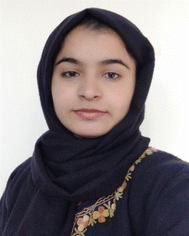 Raheela Akhter | Raheela Akhter completed her BSc from Govt. Degree College for Women Sopore. She received her MSc in Chemistry from the University of Kashmir, Hazratbal, Srinagar and also BEd from the University of Kashmir, Hazratbal, Srinagar. Currently, she is pursuing her PhD in Materials Chemistry/Catalysis under the supervision of Dr Shrikant S. Maktedar at Materials Chemistry & Engineering Research Laboratory, Department of Chemistry, National Institute of Technology Srinagar, J&K. |
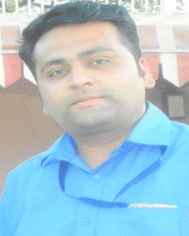 Shrikant S. Maktedar | Dr Shrikant S. Maktedar is Assistant Professor in the Department of Chemistry, National Institute of Technology (Ministry of Education, Government of India), Srinagar, J&K (UT), India. His research group focuses on the study of advanced materials for energy, environment & healthcare sustainability. Also, the challenges in the area of Food, Fertilizers and Fuels are being taken up to resolve the issues. Furthermore, in line with the current research objectives, the group passionately addressing the emerging challenges in the society through real-time prototypes. |
Sustainability spotlight
In a world where sustainability is paramount, the quest for eco-friendly solutions in every aspect of our lives, including food packaging, has gained significant momentum. Our review aims to shed light on the diverse array of sustainable food packaging materials available today, evaluating their environmental impact, functionality, and potential for widespread adoption. We delve into the realm of sustainable food packaging materials, exploring innovations ranging from biodegradable polymers derived from renewable resources to compostable alternatives that minimize environmental harm. By critically assessing the life cycle analysis, carbon footprint, and recyclability of these materials, we offer insights into their efficacy in mitigating the ecological footprint of food packaging. Through our comprehensive review, we illuminate the path towards a more sustainable future for food packaging, where innovative materials harmonize with environmental stewardship, paving the way for a greener, healthier planet.
|
1. Introduction
Amidst a rapidly growing global population and increasing urbanization, the significance of food packaging has grown exponentially. This sector represents an ever-evolving industry constantly pressured to innovate and deliver more efficient solutions. Serving as a crucial barrier against external factors and microbial contamination, food packaging ensures the safe and reliable delivery of consumables to consumers. Consequently, the processed food industry places significant emphasis on the development of packaging materials with desirable traits such as appealing aesthetics, hygienic properties, durability, and eco-friendliness. Despite the prevalence of plastics due to their lightweight nature, impermeability to gases and moisture, cost-effectiveness, and transparency, their use poses a range of drawbacks including non-biodegradability, poor heat resistance, and the presence of toxic softeners, contributing to environmental pollution and associated detriments.1–4
Research studies discussed the pivotal role of food trade in global food security and emphasized the significance of transportation within food supply chains. By utilizing a global multi-region accounting framework, the carbon footprint of food-miles is estimated, revealing that transport contributes significantly to total food-system emissions. Findings suggest that global food-miles correspond to about 3.0 Gt CO2e, significantly higher than previous estimates, with vegetable and fruit transportation contributing a substantial portion. To address environmental concerns, the text advocates for a transition towards plant-based diets and increased local production, particularly in affluent nations.5 The escalating global burden of cancer, projected to reach 28.4 million cases by 2040, with cancer surpassing cardiovascular disease as a leading cause of death in many high-income nations. It emphasizes the role of an unhealthy diet, particularly ultra-processed foods (UPFs), as a key modifiable risk factor for cancer. UPFs, characterized by their high energy density and poor nutritional quality, have been increasingly consumed worldwide, especially in countries like the UK and US. Despite growing evidence linking UPF consumption to adverse health outcomes such as obesity and type 2 diabetes, limited research exists on their association with cancer. The study aims to comprehensively assess the relationship between UPF consumption and cancer risk in a large British cohort, considering factors such as nutritional composition and potential carcinogenic contaminants from industrial processing.6 A rise of 10% in the consumption of ultra-processed foods was linked to a notable increase of over 10% in the likelihood of developing both overall and breast cancer. Additional research is necessary to gain deeper insights into how different aspects of food processing, including nutritional content, additives, packaging materials, and newly formed contaminants, contribute to these correlations.7
For more than fifty years, the global rise in wealth has consistently led to increased use of resources and emissions of pollutants at a faster rate than technological advancements have been able to reduce them. Affluent individuals worldwide bear the greatest responsibility for environmental impacts and are crucial to any efforts aimed at restoring safer environmental conditions. We provide an overview of the evidence and propose potential solutions. Achieving sustainability requires not only technological progress but also significant changes in lifestyles. However, existing societal structures, economies, and cultures promote consumption growth, while competitive market economies prioritize growth, hindering the necessary societal transformations. In understanding the true sustainability of food products, it's imperative to recognize the significance of evaluating them within the broader context of business models. Sustainability assessments must adopt a holistic perspective, taking into account the entire supply chain from production to consumption. This approach ensures that environmental, social, and economic factors are considered comprehensively. Moreover, acknowledging the risk of greenwashing, we pledge to uphold transparency in our evaluations, avoiding the promotion of unsustainable practices under the guise of sustainability. Furthermore, we are committed to addressing systemic issues within the food industry that hinder genuine sustainability efforts. By tackling these challenges head-on, we strive to foster a food system that is truly sustainable for both present and future generations.8,9
In the contemporary world, the discourse on sustainability has gained unprecedented momentum, extending its influence across various domains. This includes a pronounced focus on the packaging industry, a critical player in the modern consumer-driven economy. As the global community strives to minimize waste and reduce its ecological impact, a notable shift towards sustainable packaging materials has emerged. This review aims to comprehensively explore the advancements in this field, highlighting the progress that has steered the community toward a more environmentally conscious paradigm. A pivotal breakthrough in sustainable packaging lies in the development of biodegradable plastics, derived from renewable sources like corn starch or sugarcane. Materials such as polylactic acid (PLA) and polyhydroxyalkanoates (PHA) have surfaced as viable alternatives, offering biodegradability without compromising on performance or functionality. Compostable packaging primarily composed of natural fibers such as paper, cardboard, or bagasse, has gained significant traction, promising to enrich the soil with valuable nutrients as it degrades. Similarly, the innovative use of mycelium, the network of fungi, has led to the creation of mushroom packaging, boasting impressive insulation and shock-absorbing properties. These nature-inspired alternatives open up exciting prospects for sustainable and organic packaging solutions. Moreover, an increased emphasis on recycling has fueled advancements in using recycled and recyclable materials, promoting a circular economy and reducing the demand for virgin resources.8–12
Sustainable food packaging materials we are going to discuss in this review refer to materials used in packaging production that embody the principles of sustainability, integrating economic, social, and environmental considerations throughout their life cycle, from creation to disposal, across all stages of the supply chain. By applying the concept of sustainability to packaging production, these materials aim to achieve safe, healthy, market-efficient, and cost-effective packaging solutions. Sustainable packaging is characterized by the utilization of renewable energy sources, the use of renewable or recyclable materials, and the adoption of clean production technologies and best practices. It is designed to optimize the use of materials and energy and is capable of being efficiently recovered and reused in multiple production cycles.2
Through an in-depth analysis of these materials, this review intends to shed light on their benefits, limitations, and transformative potential within the packaging industry. By embracing these sustainable packaging solutions, people and packaging industry can collectively work towards reducing waste generation, conserving resources, and preserving the planet earth for future generations. This exploration is enriched with valuable insights into the driving forces behind the development of smart packaging (Fig. 1),10 along with a comparative illustration of active and intelligent packaging (Fig. 2).10
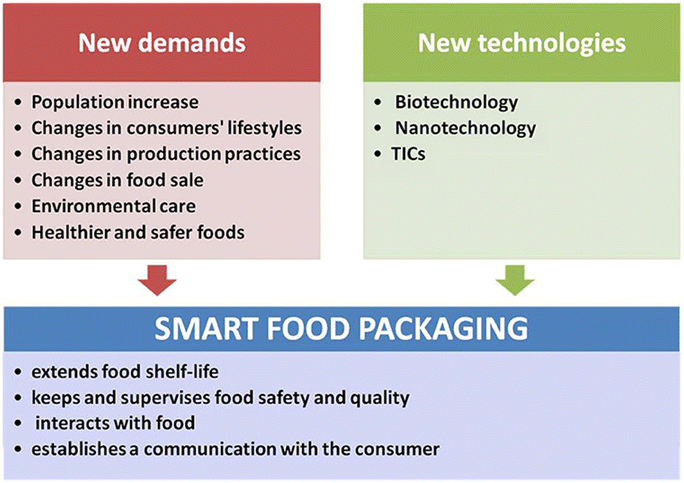 |
| Fig. 1 Driving forces for the development of smart packaging.10 | |
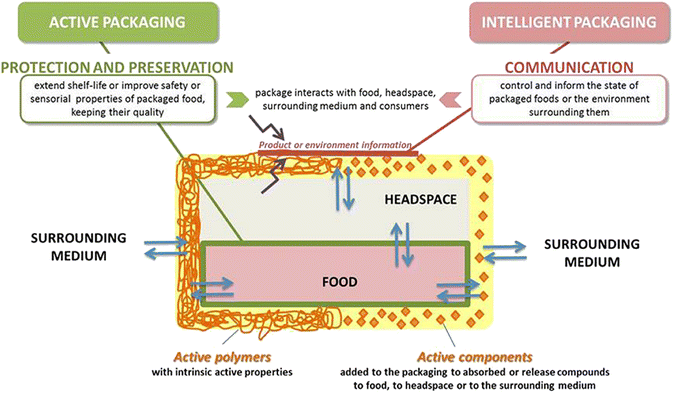 |
| Fig. 2 Active and intelligent packaging.10 | |
2. NON sustainable to sustainable food packaging. why?
2.1. Commonly used materials for food packaging
There are several types of materials commonly used in food packaging, including:13,14
Plastic: plastic is one of the most commonly used food packaging materials. It is lightweight, versatile, and cost-effective. However, some plastics may contain harmful chemicals that can leach into food.
Glass: glass is a nonporous, inert material that is ideal for food packaging because it doesn't interact with food or alter its flavor. However, it is heavy and can be easily broken, making it less practical for some applications.
Paper and cardboard: paper and cardboard are widely used in food packaging, particularly for dry goods such as cereals and crackers. They are biodegradable and recyclable, but may not provide as much protection from moisture and air as other materials.
Metal: metal packaging, such as aluminum cans and tin cans, is commonly used for food and beverage products. It provides a strong barrier against oxygen and light, but can be heavy and may not be suitable for all applications.
Composites: composite materials, such as laminates and co extrusions, are often used in food packaging field for the combination of properties of different materials. For example, a composite material may combine the strength of plastic with the barrier properties of aluminum.
Overall, the choice of food packaging material will depend on the specific needs of the product, as well as considerations such as cost, sustainability, and regulatory requirements.13,14 A general representation of classification of various food packaging systems is shown in Fig. 3.15
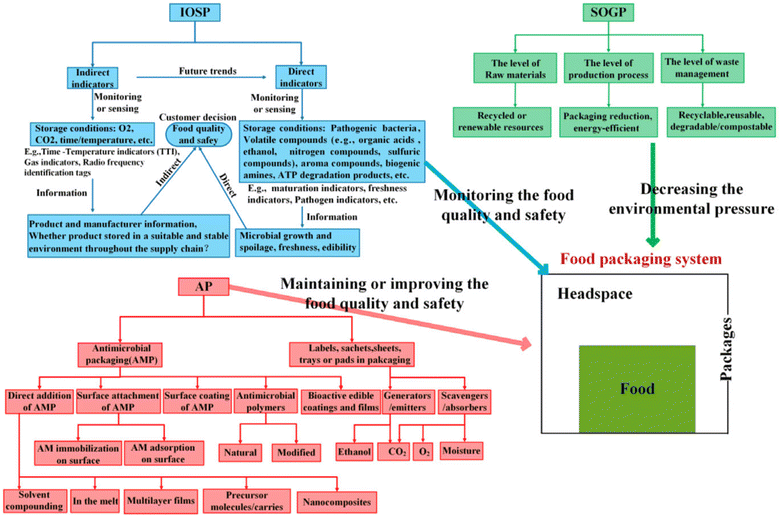 |
| Fig. 3 Classification of food packaging systems (SOGP → sustainable or green packaging, IOSP → intelligent or smart packaging, AP → active packaging).15 Reproduced (adapted) with permission from ref. 15. Copyright [2018] [John Wiley and Sons]. | |
2.2. Negative impacts of non-sustainable food packaging materials on the environment
Non-sustainable food packaging can have significant negative impacts on the environment. Here are some examples:16,17
Waste generation: non-sustainable food packaging materials such as plastic, polystyrene, and aluminum can take hundreds of years to break down, contributing to the accumulation of waste in landfills and oceans. This waste can cause harm to wildlife, pollute waterways, and emit greenhouse gases.
Energy consumption: the production of non-sustainable food packaging materials requires significant amounts of energy, which can contribute to climate change. For example, the production of plastic packaging requires the extraction of fossil fuels, which are a major contributor to greenhouse gas emissions.
Water pollution: the manufacturing process for non-sustainable food packaging materials can also produce wastewater that can pollute local water sources. This can harm aquatic life and make water unsafe for human consumption.
Deforestation: the production of non-sustainable food packaging materials such as paper and cardboard can contribute to deforestation. This can have negative impacts on biodiversity, soil health, and carbon storage.
Chemical pollution: non-sustainable food packaging materials may contain harmful chemicals such as bisphenol A (BPA) or phthalates, which can leach into food and harm human health.
In nut shell, non-sustainable food packaging can have significant negative impacts on the human health and environment. It is important to develop and promote sustainable food packaging alternatives that minimize these impacts.
Hazardous chemicals: the extensive use of chemicals in various materials used for food contact in Europe, including paper wraps, plastic packaging, glass, metal containers, and bamboo kitchenware. Despite intentional chemical usage, there are also unintended chemicals present, numbering in the tens of thousands, with only a fraction studied or known. This implies that chemicals leaching from these materials could be a significant and inadequately regulated source of food contamination. While there's considerable documentation regarding chemicals of concern in paper and board food packaging, knowledge about chemicals in other plant-based food contact materials is limited. Recent investigations have revealed troubling levels of per- and polyfluoroalkyl substances (PFAS), chloropropanols, and pesticide residues in these materials.
Per- and polyfluoroalkyl substances (PFAS) are synthetic chemicals known as ‘forever’ chemicals due to their persistence in the environment and tendency to accumulate in food chains. Exposure to PFAS has been associated with severe health effects such as cancer, developmental toxicity, and immunotoxicity. These chemicals are commonly used to impart water, grease, and stain resistance to food packaging, clothing, and various consumer products. PFAS can enter paper and plant-based materials intentionally through additives or coatings, or unintentionally as residues from precursor compounds or background contamination. Migration of PFAS from grease-resistant paper packaging into food is well-documented. Concerns about the impact of PFAS on human health and the environment are growing globally. The EU's recent Chemicals Strategy for Sustainability aims to ban all non-essential uses of PFAS and reduce exposure from food, water, air, and other sources. Denmark has already implemented a ban on the intentional use of PFAS in paper and fibrous materials for food contact, unless a functional barrier preventing migration into food is employed.
Chloropropanols, including 3-monochloropropane-1,2-diol (3-MCPD) and 1,3-dichloropropan-2-ol (1,3-DCP), are a group of chemical pollutants known for their carcinogenic properties. These compounds have been detected in various processed foods and food ingredients such as hydrolyzed vegetable protein, soy sauce, cereals, and smoked foods. Additionally, chloropropanols can be generated as process contaminants during the production of paper and board. For instance, paper made with wet-strength resins containing epichlorohydrin may contain 3-MCPD and 1,3-DCP. Previous studies have identified these contaminants in products like paper straws, but their prevalence in other fibrous materials like bagasse is not well-documented.
Pesticide residues can be found in plant-based food contact items either as remnants from pesticides used during the cultivation of natural materials like sugarcane and palm trees, or from treatments applied during subsequent processing, such as anti-fungal treatments. These pesticides, including herbicides, fungicides, insecticides, and others, are used to protect crops but can also harm biodiversity and pose health risks such as cancer, birth defects, and neurological issues. Despite the EU's stringent regulations on pesticide use in food and establishment of maximum residue levels, there's no explicit regulation on their presence in food packaging materials. In a study by Öko-Test in June 2018, it was found that disposable tableware made from palm tree leaves contained traces of the banned pesticide DDT alongside biological contaminants.18
2.3. Need and applications of sustainable and eco-friendly food packaging materials
There is a growing need for sustainable packaging materials in the modern era due to several reasons. Firstly, traditional packaging materials such as plastic, which are widely used, have negative impacts on the environment. Plastic takes hundreds of years to decompose, and it pollutes the land and oceans, harming wildlife and ecosystems. Moreover, the extraction of raw materials and the manufacturing processes required to produce traditional packaging materials contribute to greenhouse gas emissions, which worsen climate change. In response to these challenges, sustainable packaging materials are becoming increasingly important. These materials are designed to be environmentally friendly throughout their life cycle, from production to disposal. Sustainable packaging materials can be made from renewable resources, such as plant-based materials, or they can be recyclable, compostable, or biodegradable. They help to reduce waste, conserve resources, and reduce greenhouse gas emissions. The demand for sustainable packaging is growing due to increasing public awareness of environmental issues, regulatory pressure, and the need for businesses to meet sustainability goals. Sustainable packaging also provides opportunities for businesses to differentiate themselves and appeal to consumers who are increasingly eco-conscious.
Food packaging materials serve several purposes beyond just protecting the food product during transportation and storage. Some common applications of food packaging materials include:11,12
Preservation: packaging materials such as vacuum-sealed bags, airtight containers, and metal cans help preserve food products by preventing oxidation, moisture loss, and contamination by microorganisms.
Convenience: packaging materials such as single-serving packages, resalable bags, and easy-open lids make it easy for consumers to handle and use food products.
Branding and marketing: packaging materials with attractive designs and logos help promote brand recognition and influence consumer purchasing decisions.
Information and labeling: packaging materials can also provide important information such as nutritional information, ingredients, and expiration dates, which can help consumers, make informed choices.
Safety and security: packaging materials can protect food products from physical damage during transportation and storage, and tamper-evident packaging can help prevent food tampering and contamination.
Sustainability: packaging materials can be made from renewable or recyclable materials, reducing the environmental impact of packaging waste.
Extended shelf life: modified atmosphere packaging (MAP) and active packaging can extend the shelf life of perishable food products by controlling the atmosphere inside the package and inhibiting the growth of microorganisms.
Overall, food packaging materials play a crucial role in ensuring the safety, quality, and convenience of food products for consumers.19,20
The various properties of materials which make them suitable for food packaging applications are represented in Fig. 4.21 Herein, water barrier refers to water vapor barrier of cellulose based food packaging using double layer coatings and cellulose nanofibers. Water barriers in food packaging are crucial for preserving product quality and extending shelf life by preventing moisture from entering or leaving the package. Common materials include plastic films like polyethylene and polypropylene, aluminum foil, wax-coated and plastic-coated papers, and biodegradable options like PLA. Advanced solutions include multilayer laminates, active packaging with moisture absorbers, and innovative coatings such as silicon oxide and nanocomposites. These barriers are essential for maintaining the freshness, safety, and nutritional value of food products, reducing spoilage and waste throughout the supply chain.22
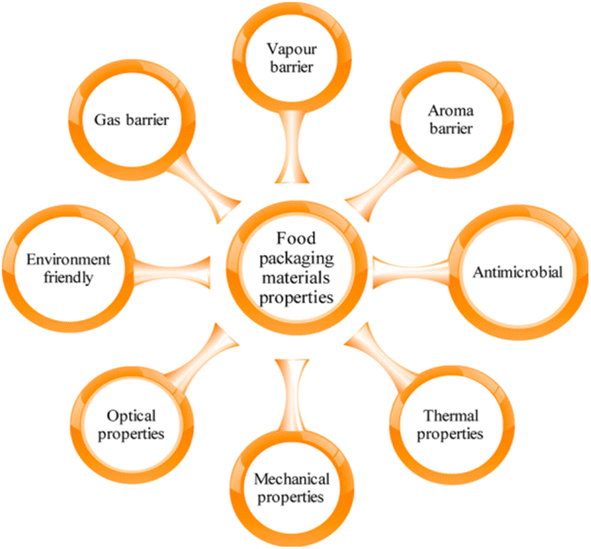 |
| Fig. 4 Quality determining properties of food packaging materials.21 Reproduced (adapted) with permission from ref. 21. Copyright [2018] [ACS]. | |
2.4. Need for sustainability in food packaging materials
There is a growing need for sustainability in food packaging for several reasons:23
Environmental impact: traditional food packaging, such as plastic, often ends up in landfills or pollutes the environment, causing harm to wildlife and ecosystems.
Resource depletion: many traditional materials for food packaging are made from non-renewable resources, such as petroleum, which are becoming increasingly scarce and expensive.
Consumer demand: consumers are becoming more aware of the impact of their purchasing decisions on the environment, and are demanding more sustainable options.
Regulatory requirements: Governments around the world are implementing regulations to reduce waste and promote sustainability, which includes food packaging.
To address these challenges, the food industry is increasingly turning to more sustainable packaging solutions, such as biodegradable, compostable, and recyclable materials. By using sustainable packaging, the food industry can reduce waste, conserve resources, and meet the growing demand for environmentally responsible products.23
2.5. A brief timeline of food packaging materials
Here is a brief timeline of food packaging materials:24
➢ Early history: people used natural materials such as leaves, animal skins, and woven baskets to store and transport food.
➢ 1800s: paper and glass began to be used for food packaging.
➢ Early 1900s: tin cans were invented, making it easier to transport and store food.
➢ Mid-1900s: plastic packaging became more popular due to its versatility and cost-effectiveness.
➢ 1960s: the first recyclable plastic, polyethylene terephthalate (PET), was invented.
➢ 1970s: concerns about the environmental impact of packaging led to the development of biodegradable materials.
➢ 1990s: compostable packaging materials were introduced, made from renewable resources like cornstarch and sugarcane.
➢ 2000s: innovations in packaging included the active and intelligent packaging development, which can help preserve food freshness and safety.
➢ 2010s: the use of sustainable materials like bamboo, mushroom, and seaweed for food packaging gained popularity.
➢ Nowadays: today, there is a growing trend towards the use of more sustainable packaging solutions, such as biodegradable, compostable, and recyclable materials, as the food industry aims to reduce waste and promote sustainability.
2.6. General examples of sustainable food packaging materials
To address these challenges, the food industry is increasingly turning to more sustainable packaging solutions, such as biodegradable, compostable, and recyclable materials. Some examples of sustainable food packaging materials include:25
Bioplastics: these are plastics made from renewable resources like corn, sugarcane, and potatoes, which can biodegrade in the environment.
Paper-based materials: these are made from sustainable materials like bamboo, wheat straw, and recycled paper.
Plant-based materials: these are made from natural materials like seaweed, mushroom, and sugarcane waste.
Compostable materials: these are designed to break down in a composting environment, leaving no harmful residue.
By using sustainable packaging, the food industry can reduce waste, conserve resources, and meet the growing demand for environmentally responsible products.
Table 1 represents some important food packaging materials & their functionalities.
Table 1 Important food packaging materials and their functionalities
Packaging material |
Functionality |
References |
Poly glycolic acid (PGA) |
Barrier property |
26
|
Nanofiller blended biopolymer |
Barrier property and hydrophobicity |
27
|
Plastic films |
Printability, barrier property and sealing |
28
|
Cellophane |
Excellent elasticity and mechanical property |
29
|
PLA reinforced with cellulose nanowhiskers |
Water vapor and oxygen barrier properties |
30
|
Chitosan reinforced with bacterial cellulose micro- and nanofibers |
Bactericidal, mechanical, water vapor barrier and bacteriostatic |
30
|
Paper |
Printability |
31
|
Paper processed in biopolymer coating |
Good barrier property |
32
|
Paper sheet containing polyaniline (PANI) and polystyrene (PS), in the presence of dispersed bagasse pulp fibers |
Antibacterial property |
29
|
Inorganic nanoparticle |
Antimicrobial |
29
|
Organic nanoparticle |
Good tensile strength, mechanical property, environmental friendly, barrier property and reduced cost |
29
|
Bio nanocomposite |
Good mechanical, heat resistance property and barrier property |
29
|
3. Advanced food packaging materials and methods
3.1. Polymer packaging materials
Polymer packaging materials are of four important types as shown in Fig. 5.33
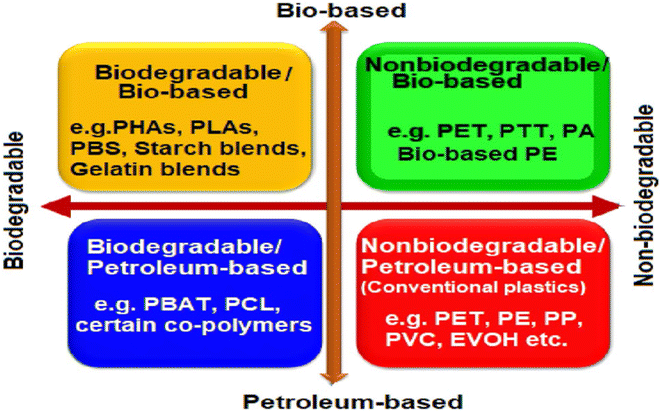 |
| Fig. 5 Different types of polymer packaging materials.33 Reproduced (adapted) with permission from ref. 33. Copyright [2020] [Elsevier]. | |
Bioplastics serve as a preferable substitute for petrochemical-based plastics due to the increased harmful impacts associated with the latter (Fig. 6).34 The benefits of bioplastics, such as a reduced carbon footprint, support for rural economies, and use of renewable resources, confer it with distinct advantages. Conversely, the non-biodegradable nature, limited renewability, and adverse health implications stemming from the emission of hazardous gases, among other characteristics, render petrochemical-based plastics less desirable.
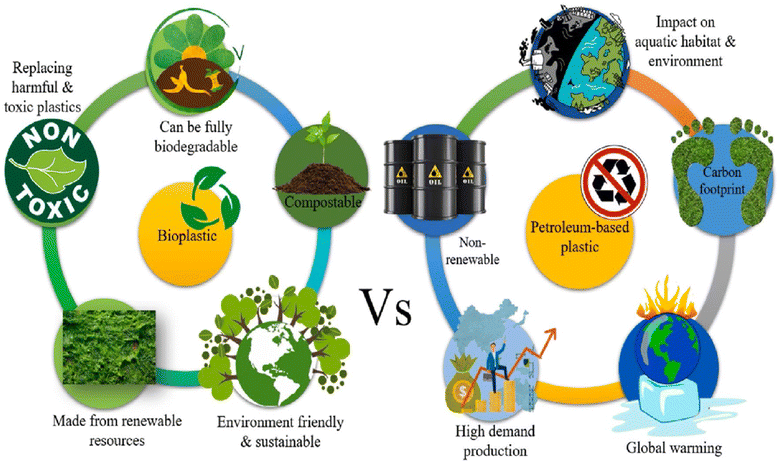 |
| Fig. 6 Comparison between bioplastic and petroleum-based plastics.34 Reproduced (adapted) with permission from ref. 34. Copyright [2022] [Elsevier]. | |
Bioplastics are presented as a greener option to traditional petroleum-based plastics. Despite their low market share, expected growth suggests increasing popularity. The term encompasses materials made from renewable sources, those designed to degrade naturally, or both. However, it's unclear if plant-based blends qualify. Bioplastics are marketed as sustainable, but scientific support for their environmental benefits is limited. Some biodegradable plastics do not degrade effectively in natural environments because they require specific conditions, such as high temperatures and controlled microbial activity, which are not typically present outside industrial composting facilities. As a result, they may persist longer than expected, fragment into microplastics, or leave toxic residues. Assessment often focuses on production and end-of-life aspects, neglecting chemical safety during use. Understanding chemical composition and toxicity is crucial as human exposure will rise with increased usage. Plastics contain various intentionally added compounds like plasticizers and stabilizers, alongside unintentional substances. These chemicals can migrate into the environment, posing risks to humans and ecosystems.35
3.1.1. Petroleum based packaging materials.
Petroleum-derived polymers hold a significant and predominant role in the domain of food packaging, thus experiencing widespread usage in this sector. Alongside favorable attributes including ease of processing and excellent mechanical and barrier properties, the major detrimental concerns of these polymers lie in their lack of degradability and the emission of greenhouse gases.36 Among the commonly utilized petroleum-based polymers in food packaging are polypropylene (PP), poly (vinyl chloride) (PVC), polyethylene (PE), polyamides (PA), polyurethane (PU), expanded polystyrene, polystyrene (PS), and poly (ethylene terephthalate) (PET).37
3.1.2. Bio-based packaging materials.
These polymers originate from living organisms, including plants and microbes, and are created through metabolic engineering processes and renewable resources, such as food waste, wood, agricultural waste, polysaccharides, or lignocelluloses38 (Fig. 7).39
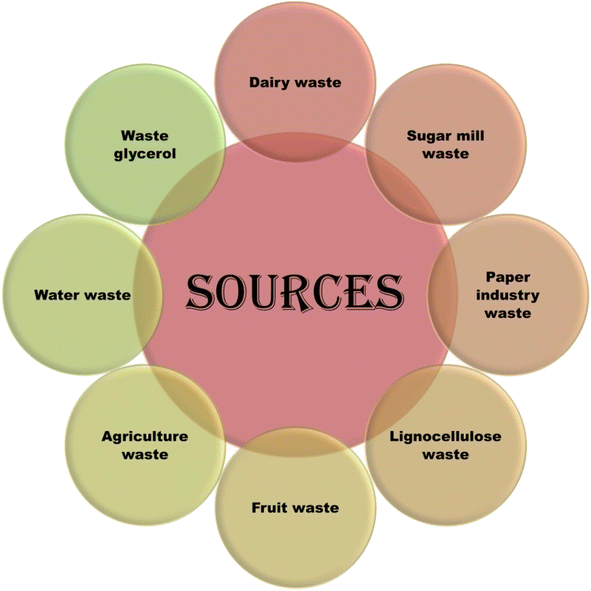 |
| Fig. 7 Substrate sources for biopolymer production.39 Reproduced (adapted) with permission from ref. 39. Copyright [2022] [Springer Nature]. | |
Biodegradability, biocompatibility, and other similar characteristics of such polymers make them ideal for food contacts. Biobased polymers are divided into three groups on the basis of methods of synthesis: microbially originated polymers, protein based polymers and wood based polymers.
Table 2 represents the active/intelligent agent blended biobased materials.39
Table 2 Active/intelligent agent blended biobased materials
Biobased nanomaterials |
Active/intelligent agent |
References |
Nanocellulose |
Flavonoid silymarin |
40
|
Nanocellulose |
Ferulic acid & derivatives |
41
|
Bacterial nanocellulose |
Sulfobetaine methacrylate |
42
|
Nanofibrillated cellulose (NFC) |
Tannins |
43
|
Cellulose nanocrystal (CNC) |
Wheat gluten incorporating TiO2 nanoparticles |
44
|
AgNP/BNC-PVA |
Ag NPs |
45
|
Bacterial nanocellulose |
Methyl red |
46
|
Sugarcane bagasse nanocellulose hydrogel |
Zn2+ cross-linking |
47
|
3.1.2.1. Microbially originated polymers.
This category of polymers can be produced through the utilization of a fermentation process. Enzymatic catalysts facilitate the fermentation process, utilizing various renewable feedstocks as substrates.48 Microorganisms are capable of biosynthesizing a range of biopolymers, including but not limited to polyhydroxyalkanoates, xanthan, polysaccharides, dextrans, oligosaccharides, pullulan, organic acids, glucans, cellulose, gellan, hyaluronic acid, alginate, levan, cyanophycin, and poly (gamma-glutamic acid).49 Below, there is discussion of some significant polymers derived from microorganisms.
3.1.2.1.1 Polyhydroxyalkanoates (PHA).
PHA was initially discovered by Beijernick and his associates, and it is manufactured through bacterial cell fermentation. Among the various categories of PHA, including polyhydroxybutyrate, polyhydroxyvalerate, polyhydroxyhexanoate, and polyhydroxyoctanoate, PHB stands out as the most preferred option due to its widespread acceptance and durability, closely resembling conventional plastics.50 The Gram-negative bacterium Alcaligenes eutrophus synthesizes PHB as an energy reserve in the presence of a high carbon supply, with limited quantities of phosphorus, oxygen, nitrogen, and sulfur. This process accounts for approximately 70 to 80% of the bacterial cell's dry weight, depending on the microbial colony and the carbon source.51 Various renewable resources, including waste materials from food such as domestic waste, maltose, fats, xylose, frying oil, starch, crude glycerol, fructose, as well as gases and n-alcohol, serve as viable sources for PHA synthesis.
The formation of PHA polymers involves the linking of several monomers, resulting in unique properties such as biodegradability and characteristics similar to those of conventional plastics.52 Owing to its high biodegradability and other similar attributes, PHA is particularly well-suited for use in short-term packaging applications.
3.1.2.1.2 Polylactic acid.
PLAs can be produced from aliphatic monomers, such as lactic acid, either through chemical synthesis or starch fermentation. The fermentation process can utilize various carbohydrate-rich sources like kitchen waste, wheat, sugarcane, or corn. The synthesis of PLA involves several key steps, including lactic acid production, the formation of lactide monomers, and subsequent polymerization.53 PLA's popularity in food packaging can be attributed to its non-toxic nature, optimal mechanical strength, renewability, and biodegradability. Additionally, its production emits fewer carbon emissions, consumes less energy, and generates minimal waste. Despite its limitations in heat resistance, ongoing research efforts over the past decades have focused on enhancing its properties and reducing costs. Blending PLA with cellulose has been explored as a strategy to improve its heat resistance.54 Furthermore, the incorporation of various fillers and polymers has been investigated to enhance the performance of the final product while minimizing costs. Additionally, the addition of nanofillers, such as silica and talc, and nanoadditives can lead to further improvements in PLA's physical and chemical properties.
Recent investigations have demonstrated that PLA possesses antimicrobial activity. Surface coatings of PLA integrated with substances like silver nanoparticles, cellulose nanocrystals, sophorolipid, and lysozyme exhibit preventive effects against microorganisms such as Staphylococcus aureus, Escherichia coli, Listeria monocytogenes, Salmonella spp, and Micrococcus lysodeikticus. These coatings have also demonstrated the potential to extend the shelf life of perishable fruits.55
3.1.2.1.3 Exopolysaccharides.
These long-chain polysaccharides can be generated through the bacterial fermentation of various sources, including dairy waste, carbohydrate-rich food waste, sugarcane juice, potato peel, coconut waste, and sugarcane molasses. While lactic acid bacteria (LAB) have historically been employed for enhancing the functional properties of food in terms of prebiotics and probiotics, their role has expanded significantly with the growing emphasis on safe food consumption and technological advancements. LAB are now extensively utilized for the production of exopolysaccharides, contributing to improvements in food texture and secure packaging. In addition to Gram-negative and Gram-positive bacteria, exopolysaccharides can also be synthesized by fungi, yeast, and blue–green algae.56
Exopolysaccharides produced by LAB are instrumental in formulating edible coatings or films for food products, owing to their favorable structural integrity, as well as their smooth and lustrous surface. Integrating exopolymers with other nanocomposites not only enhances productivity from LAB but also imparts antioxidant and antimicrobial properties.57 The preparation of composite EPS films achieved through the incorporation of sodium carboxymethylcellulose (CMC) with lactic acid bacteria (Lactobacillus plantarum), results in films with reduced moisture absorption capacity and improved antioxidant activity.58,59 Kafiran, distinguished by its biodegradability and antimicrobial properties, is gaining notable attention within the spectrum of exopolysaccharides.
3.1.2.2. Wood based polymers.
Lignocellulosic polysaccharides serve as the primary source for the production of these polymers, comprising approximately 40 to 50% cellulose, 20 to 30% hemicellulose, and 20 to 25% lignin (Fig. 8).60 Wood fibers have diversified applications not only in wood products but also in non-wood products, assorted panel boards, and paper products.60
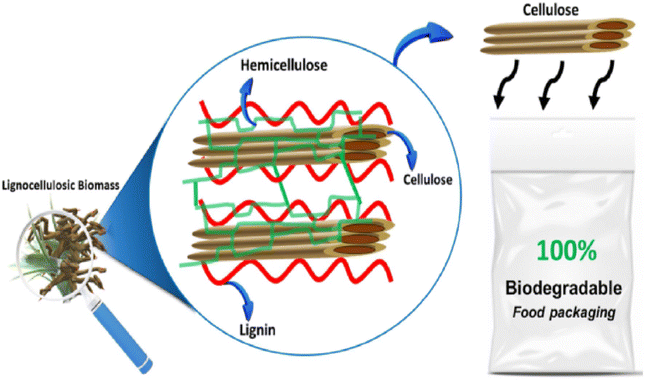 |
| Fig. 8 Lignocelluloses biomass-composition.60 | |
Some important wood based polymers are discussed below.
3.1.2.2.1 Cellulose & hemicellulose.
To harness the multifaceted attributes of cellulose, such as its capacity to augment color, enhance mechanical properties, bolster barrier properties, and improve dyeing, various materials can be introduced alongside cellulose nanoparticles.60 The combination of chitin with cellulose nanocrystals yields a homogenous surface, establishes a robust percolating network, and serves as fillers to achieve optimal tensile strength.61 When nanocellulose and alginate polymers form nanocomposite films, the resultant product exhibits commendable tensile strength, although increasing the concentration of cellulose nanomaterials renders the film transparent.62
For the development of biodegradable films with low water vapor permeability, carboxymethyl cellulose (CMC) extracted from sugarcane bagasse can be amalgamated with gelatin, glycerol, and agar.63Fig. 9 (ref. 39) illustrates the progression of the development of CMC-blended bioplastics.
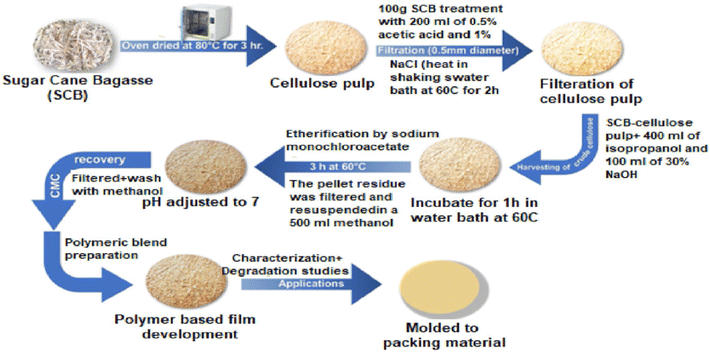 |
| Fig. 9 Flow of development of CMC bioplastics.39 Reproduced (adapted) with permission from ref. 39. Copyright [2022] [Springer Nature]. | |
Cellulose monomers play an important role in the production of polymer as represented in Fig. 10.64
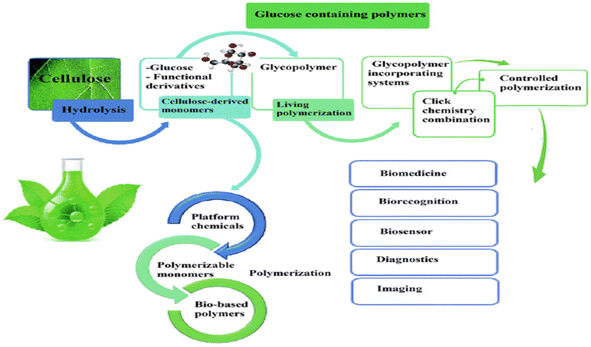 |
| Fig. 10 Possible applications of cellulose monomers for polymer production.64 Reproduced (adapted) with permission from ref. 64. Copyright [2018] [RSC]. | |
Hemicellulose is regarded as one of the potentially more environmentally friendly alternatives to plastics for food packaging. Methods such as hot water extraction, alkali extraction, acid extraction, and steam explosion are commonly employed for the extraction of hemicellulose from plant cells. Notably, the two-step alkali extraction-delignification method yields the highest output at 84%. With its low molecular weight and hydroxyl functional group, hemicellulose can be utilized in film production using the casting and drying method, making it suitable for food packaging.65Fig. 11 (ref. 39) illustrates the process of film formation from hemicellulose solution.
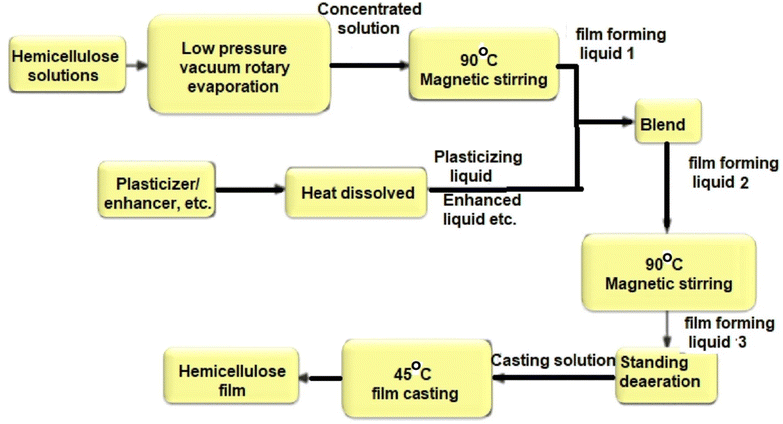 |
| Fig. 11 Flow chart of hemicellulose film formation.39 Reproduced (adapted) with permission from ref. 39. Copyright [2022] [Springer Nature]. | |
3.1.2.2.2 Chitosan/chitin.
Because of the existence of amino and hydroxyl groups on chitosan, it exhibits antimicrobial properties against a range of bacterial species, including both Gram-negative and Gram-positive types. Films derived from the combination of chitosan with diverse materials demonstrate outstanding antioxidant and antimicrobial activities suitable for food packaging.66,67 The distinctive properties of chitosan enable its application in the production of food packaging films and coatings.
3.1.2.2.3 Lignins.
Roughly eighty million tons of the available lignin are annually consumed in the paper-making industry, with only 2% utilized in processing and the remaining 98% wasted as fuel. Up to 90% of the technical lignin produced comes from kraft lignin and lignosulfonates, acquired through the delignification method.68 The hydrophilic nature and the presence of hydroxyl groups on lignin polymers facilitate its incorporation with polylactic acids, starch, and amino acids, enhancing mechanical properties and reducing moisture absorption characteristics. When used as a reinforcing material blended with starch extracted from sago palm, lignin contributes to improved barrier properties and seal strength in packaging films.69 Additionally, lignin can function as a stabilizer and plasticizer alongside PLA, cellulose, and PLB polymers, promoting the production of biocompatible and biodegradable bioplastics.70
3.1.2.3. Protein based polymers.
3.1.2.3.1 Gelatin & collagen.
Gelatin is derived from the partial hydrolysis of collagen, resulting in an odorless protein characterized by a random polypeptide chain. Its exceptional functional properties render it a valuable biopolymer within the food packaging industry. The two primary types of gelatin, type A and type B, are distinguished by their processing methods. Type A gelatin, with an isoelectric point between pH 8 to 9, is acquired through collagen treatment with acids. Conversely, type B gelatin, with an isoelectric point between pH 4 to 5, is obtained by collagen treatment with alkali, leading to the conversion of asparagine and glutamine residues into their acids, thereby achieving higher viscosity. Type A gelatin is sourced from pig skin, while type B gelatin is sourced from bones and beef skin.69
Films produced from gelatin tend to exhibit high moisture absorption, necessitating various enhancements to modify its hydrophilic nature. Crosslinking modifications, facilitated by the addition of natural extracts, have been shown to enhance gel strength in comparison to pure gelatin film.70 The antimicrobial and antioxidant properties of gelatin can be amplified by blending it with other materials, including zinc oxide nanoparticles, chitosan, and tea polyphenols, among others.71
3.1.2.3.2 Soy protein.
The soy-based film, renowned for its desirable properties in food packaging applications, boasts exceptional texture-forming capabilities, strong adhesiveness, and effective fiber binding. These films are derived from soy protein isolates, which contain approximately 90% protein content.72 Soy protein isolates can be precipitated from diverse soy sources, including crude soybean, soy milk, or soy flour. Various sources of soy yield soy-based films with distinct functional and mechanical properties, as well as different molecular weights. Incorporating different substances into soy-based films can contribute to enhancing their physical and mechanical characteristics.73 For instance, the addition of stearic acid and cysteine leads to improvements in several properties of soy-based films, such as reduced water vapor permeability and water absorption capacity, along with enhanced tensile strength.74 Beyond soy proteins, pea proteins, zein proteins, and globulin proteins are also viable options for food packaging applications.74
3.1.2.3.3 Casein & whey proteins.
Both casein and whey proteins are derived from milk subsequent to cheese production. Owing to intermolecular hydrogen and electrostatic bonds, casein can be employed to create films within aqueous solutions. However, the hydrophilic nature of these films renders them susceptible to moisture. By introducing polysaccharides, genipin, lipids, wax, and glutaraldehyde via crosslinking, the chemical and physical properties of these films can be significantly enhanced, resulting in reduced moisture absorption capacity and prolonged shelf life.75 Whey protein concentrates and whey protein isolates, both rich sources of sulfur-containing amino acids such as cysteine and methionine, are utilized in the production of whey-based food packaging. Plasticized whey-based films can be developed by heating the whey protein isolate solution for denaturation over a few minutes at temperatures ranging from 80 to 100 °C. A more uniform WPC film can be achieved by treating the solution at 75 °C for 30 minutes under an alkaline pH of 6.6. Furthermore, exposing the film to UV treatment within an alkaline pH range of 7 to 9 can enhance uniformity, tensile strength, and elasticity. The addition of lipids, such as fatty acids, waxes, or plant oils, serves to mitigate the hydrophilic nature of the film.76
Petroleum-based packaging materials have been the backbone of the packaging industry due to their durability and cost-effectiveness. However, their widespread use contributes to plastic pollution, resource depletion, and the persistence of non-biodegradable waste. As environmental concerns mount, the shift towards bio-based packaging materials has gained momentum. Bio-based packaging materials are derived from renewable sources such as plants and agricultural waste. They offer a more sustainable alternative by reducing the reliance on fossil fuels and decreasing carbon emissions during production. These materials can also be engineered for biodegradability or compostability, addressing the problem of packaging waste that lingers in the environment. Polylactic acid (PLA) is a prime example of a bio-based material. Derived from corn starch, PLA offers transparency and moderate barrier properties. It can be used in various applications, from food containers to beverage cups. Polyhydroxyalkanoates (PHA) are another promising bio-based option. Produced by microorganisms, PHA is biodegradable and exhibits diverse mechanical properties. These materials have the potential to revolutionize the packaging landscape by offering both functionality and sustainability.
3.2. Bio-nanocomposites in food packaging
3.2.1. Biopolymers.
Biopolymers, commonly referred to as biodegradable plastics, are polymeric materials that undergo the metabolism of naturally occurring organisms as part of their degradation process.77,78 However, their utilization in the industrial sector is limited due to their inadequate mechanical and barrier properties. The challenges of performance, processing, and cost are common issues associated with biopolymers regardless of their origin.79–81 In addition to these factors, various limitations further restrict their applications, such as low heat distortion temperature, brittleness, high gas and vapor permeability, and poor resistance to prolonged processing.82–86 Despite these constraints, they find application in food packaging materials due to unique packaging-related enhancements. Fig. 12 (ref. 87) provides a schematic representation of the classification of biopolymers, while Fig. 13 (ref. 87) illustrates the life cycle of biopolymer packaging materials.
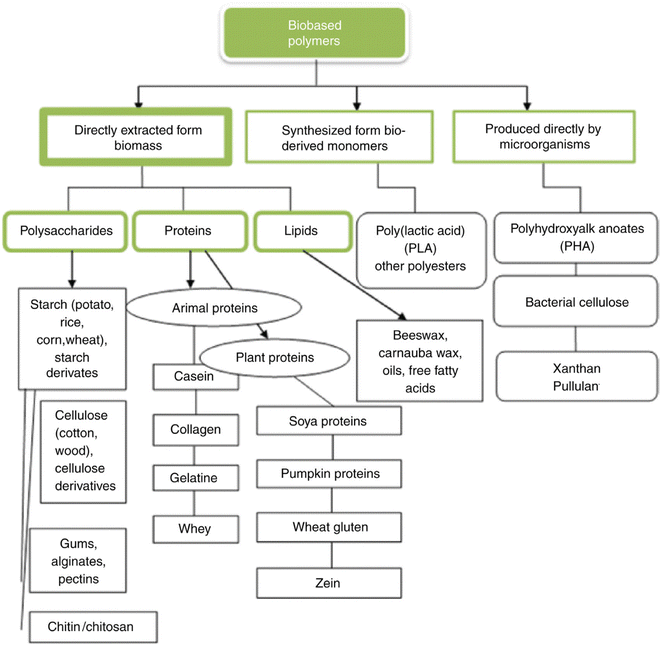 |
| Fig. 12 Classification of biopolymers.87 Reproduced (adapted) with permission from ref. 87. Copyright [2018] [Elsevier]. | |
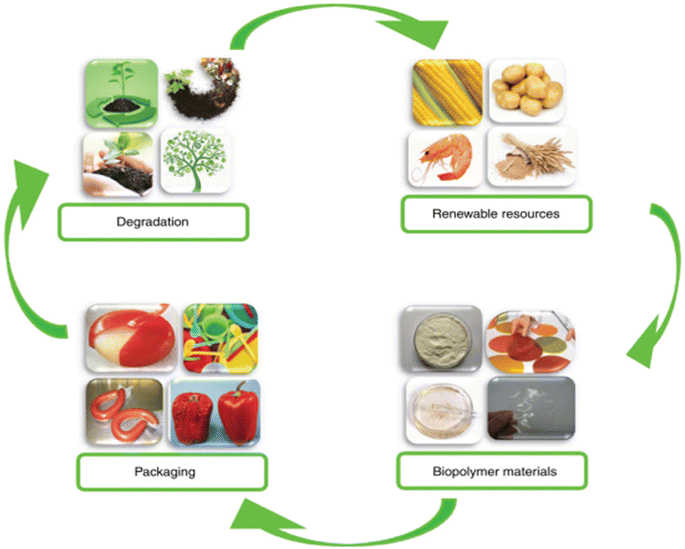 |
| Fig. 13 Life cycle of biopolymer packaging materials.87 Reproduced (adapted) with permission from ref. 87. Copyright [2018] [Elsevier]. | |
3.2.2. Biopolymer-based nanocomposites.
Nanocomposite technology stands out as one of the pivotal technologies that effectively addresses the inherent limitations of biopolymer-based packaging materials.88 When compared to neat polymers and their conventional composites, nanocomposites demonstrate improved properties, including enhanced mechanical strength, superior barrier properties, and improved heat resistance.89–92 For instance, the incorporation of montmorillonite clay in nylon enhances its mechanical and thermal properties.93 Utilizing such nanocomposites in food packaging applications result in advancements in food technology processes, allowing for better endurance during thermal processing, storage, and transportation.90,94 The preparation and characterization of various types of biodegradable polymer nanocomposites constitute an area of significant research importance, with numerous research groups actively engaged in this field. These biodegradable polymer nanocomposites, known as bio-nanocomposites, exhibit various unique properties that cater to a wide range of applications.95 The presence of layered silicates within plays a crucial role in enhancing the desirable properties of both natural and synthetic biodegradable polymers, while preserving their biodegradability. The inclusion of clay layers in these polymers significantly bolsters their barrier properties by obstructing the molecular pathway, resulting in a more convoluted diffusive path.87Fig. 14 illustrates the antimicrobial mechanisms of action of nanocomposites designed for food packaging.96
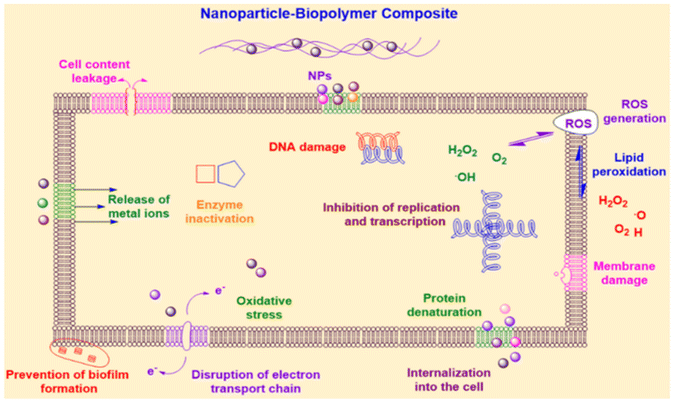 |
| Fig. 14 Antimicrobial mechanisms of action of nanocomposites designed for food packaging.96 | |
Bio-nanocomposites represent a fusion of biopolymers and nanomaterials, resulting in materials that combine the benefits of both worlds. Biopolymers, sourced from renewable resources, reduce the environmental impact associated with conventional plastics.
3.3. Carbon nanostructures in food packaging
3.3.1. Carbon dots.
Fluorescent carbons, also known as carbon dots, exhibit a multitude of unique and exceptional properties, including high chemical stability, facile synthetic methods, and water solubility, among others. Their exceptional antibacterial properties can be attributed to the diverse functional groups present in them, such as –OH, –NH, and –COOH, alongside a carbon center. For instance, Raina et al.97 demonstrated the antibacterial effect of Ag@CD against Escherichia coli and Staphylococcus aureus bacteria, utilizing Cannabis sativa leaves for the synthesis of carbon dots. Other researchers have employed the hydrothermal method to assess the antibacterial potential of N-doped CDs, utilizing Osmanthus leaves as a precursor for CD synthesis.98
Additionally, CDs can serve as effective and environmentally friendly active packaging materials. This application was verified through various experiments, including the fabrication of antimicrobial bacterial nanocellulose (BNC) sheets using CDs. Incorporating small-sized CDs (4–5 nm) derived from white mulberry into the BNC resulted in the production of nanopaper with strong antimicrobial and UV-blocking properties against L. Monocytogenes.99 Similarly, CDs obtained from lactic acid bacteria were utilized by Kousheh et al.100 to create a UV-protective and antimicrobial bacterial nanocomposite film, exhibiting notable mechanical and antimicrobial activities against L. monocytogenes and E. coli.
The potential applications of carbon dots as additives, coating agents, and active and intelligent agents for food packaging purposes are illustrated in Fig. 15.101 Furthermore, Fig. 16 (ref. 101) delineates the ultraviolet light barrier mechanism of carbon dots-based biodegradable packaging, highlighting their role in safeguarding food products from ultraviolet radiation.
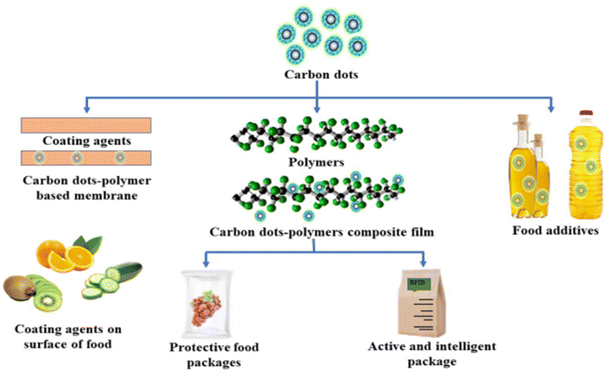 |
| Fig. 15 Potential applications of carbon dots as additives, coating agent, and active and intelligent agents for food packaging applications.101 | |
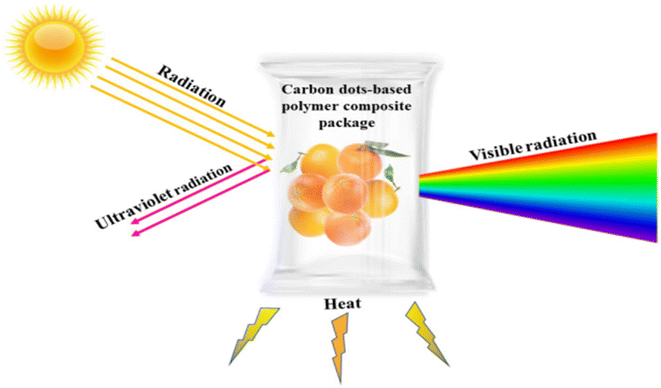 |
| Fig. 16 Ultraviolet light barrier mechanism of carbon dots based on biodegradable packaging illustrating food product's protection from ultraviolet radiation.101 | |
3.3.2. Carbon nanotubes.
One-dimensional carbon nanomaterials are divided into two categories: single-walled carbon nanotubes (SWCNTs) and multi-walled carbon nanotubes (MWCNTs).102 SWCNTs consist of a single layer of graphene rolled into a cylinder, while MWCNTs are characterized by a coaxial arrangement of multiple concentric cylindrical shells around a central hollow core, held together by van der Waals forces between adjacent layers.103 Extensive research has investigated the antibacterial properties of carbon nanotubes. For instance, Ag-doped TiO2 nanoparticles with SWCNTs and MWCNTs coatings were synthesized, and their antibacterial efficacy against S. aureus and E. coli was studied, revealing that SWCNTs-TiO2/Ag exhibited greater toxicity than MWCNTs-TiO2/Ag.104
Moreover, carbon nanotubes have found applications in food packaging materials. Incorporating CNTs into bionanocomposites of poly(3-hydroxybutyrate-co-3-hydroxyvalerate) has significantly enhanced the mechanical and thermal properties, as well as the tensile strength and Young's modulus, of the resulting films.105 These improved properties have contributed to their widespread use as food preservatives and packaging materials. Additionally, films made using carbon nanotubes and isothiocyanate have been employed for the packaging of shredded and cooked chicken meat.106 CNTs have also been utilized in the production of garlic-microparticle-coated gelatin/MWCNT nanocomposite films for use as food packaging material.107
3.3.3. Graphene-based nanomaterials.
Numerous research studies have focused on examining the antibacterial properties of nanomaterials based on graphene. For instance, the antibacterial effects of graphene oxide (GO) nanosheets against both Gram-negative and Gram-positive bacteria have been investigated, revealing distinct zones of inhibition in comparison to commercially available antibiotics.108 Notably, the zones of inhibition demonstrated by GO were as follows: E. coli, 39 mm; S. aureus, 38 mm; K. pneumoniae, 41 mm; P. aeruginosa, 38 mm; S. marcescens, 39 mm; and P. mirabilis, 27 mm. Enhanced antibacterial activity was observed in GO synthesized via the modified Hummers' method, surpassing the effectiveness of all the tested commercial antibiotics. Other studies on the antimicrobial properties of graphene-based nanomaterials have similarly presented promising findings.109–111
In another study by Vi et al., the antibacterial activity of Ag-GO against S. aureus and E. coli was investigated, revealing that Ag-GO exhibited 73% inhibition against E. coli and 98.5% inhibition against S. aureus when compared to pristine GO and silver nanoparticles.110Fig. 17 illustrates the different zones of inhibition.110
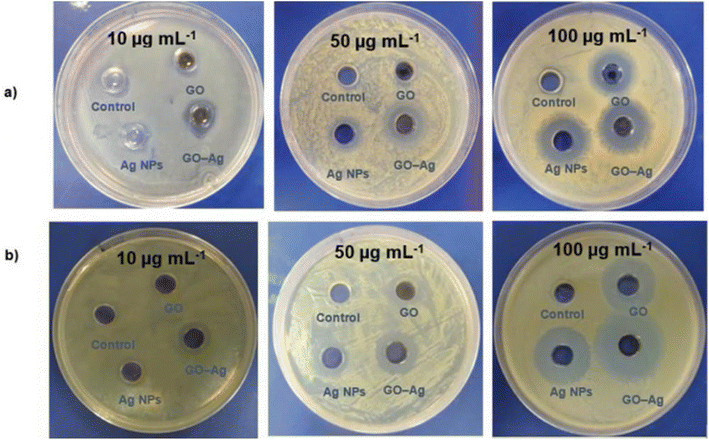 |
| Fig. 17 Zone of inhibitions displayed by control, GO, Ag NPs, and GO-Ag against (a) E. coli and (b) S. aureus.110 | |
In contemporary times, graphene-based nanomaterials have also found utilization as biofiller materials. For instance, GO/polylactic acid films111,112 have been employed as food packaging materials, demonstrating antibacterial activity against S. aureus and E. coli. Likewise, Ag–ZnO-rGO/polyethylene nanocomposite films,113 MTAC-rGO-EVOH films,114 starch-reduced graphene oxide/polyiodide nanocomposite films,115 thermally exfoliated graphene oxide reinforced polycaprolactone-based bactericidal nanocomposite films,116 and various others are extensively employed in the food packaging industry, showcasing antibacterial properties alongside their potential as effective food packaging materials. The process of forming films using carbon-based nanomaterials is illustrated schematically in Fig. 18.117
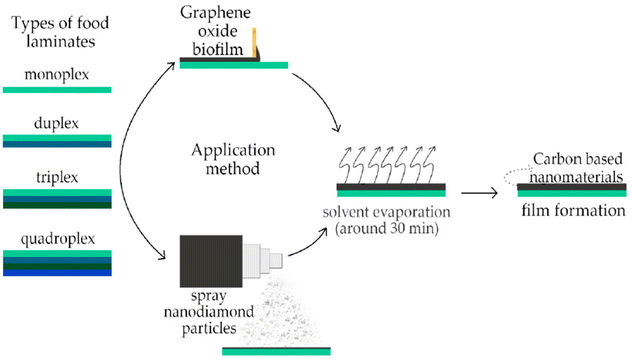 |
| Fig. 18 Schematic representation of the carbon based nanomaterials film formation.117 | |
Nanomaterials, such as carbon nanotubes and graphene, enhance mechanical properties and offer unique functionalities. The integration of nanomaterials into bio-based matrices improves packaging strength, barrier properties, and even sensor capabilities. Carbon nanotubes, known for their exceptional mechanical strength, reinforce packaging materials without compromising their biodegradability. Graphene, with its high electrical conductivity, opens doors to smart packaging that can monitor food quality in real time. These innovations promote sustainability by enabling packaging manufacturers to create thinner and lighter materials while maintaining desired properties. Reduced material consumption translates to lower resource use and transportation costs, thereby minimizing the environmental footprint of packaging.
Fig. 19 represents applications of nanoparticles in food packaging118 and various nanomaterials which are used in food packaging industry are discussed below.
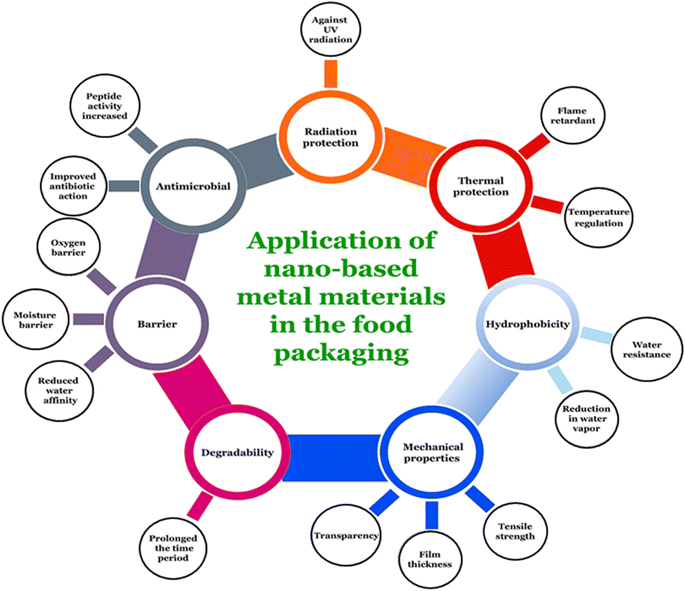 |
| Fig. 19 Applications and characteristics of nano-based metal-materials in food packaging.118 | |
3.4. Nanomaterials in food packaging
3.4.1. Nanocellulose.
Cellulose, a polysaccharide abundantly present in nature and a key component of plant cell walls, plays a critical role in providing structural integrity to plant cells. Nanocellulose, with a nano-sized cellulose structure, can exist as either nanocrystals or nanofibers, characterized by a diameter of less than 100 nm and lengths of a few micrometers.119 Chemical and mechanical extraction processes enable the retrieval of nanocelluloses from plants, facilitating the creation of dense, impermeable surfaces for molecules owing to their remarkable hydrogen bonding capability. This unique property of nanocellulose contributes to its excellent barrier properties.120 The widespread use of nanocellulose in the paper and composite industries serves to enhance uniformity, biodegradability, strength, and mechanical properties.121 Incorporating nanocellulose into biopolymers presents challenges due to the interfacial disparities between the two constituents; however, successful integration leads to improved mechanical, thermal, and barrier properties.122
3.4.2. Nano starch.
The intricate polysaccharide starch is comprised of two polymers, namely amylose and amylopectin, and can be generated through diverse physical and chemical treatments involving the breakdown of starch granules.123 These nanoparticles possess a substantial surface area per unit volume and have at least one dimension smaller than 300 nm.124 Employed as nanofillers, starch nanoparticles/starch nanocrystals serve to enhance the biodegradability, thermal and water impermeability, strength, flexibility, and barrier properties of composites.125,126
3.4.3. Protein nanoparticles.
Protein-based nanoparticles significantly contribute to bolstering the barrier properties, such as water resistance and strength, of food packaging materials.127 Incorporating peanut protein nanoparticles into protein-starch-based biocomposites was observed to enhance their strength, moisture barrier properties, and temperature resistance.128 Similarly, the incorporation of zein nanoparticles into whey isolate-based films was found to enhance their moisture barrier and mechanical properties.129
3.4.4. Silver nanoparticles.
Silver nanoparticles (AgNPs) play a crucial role as antimicrobial agents in food packaging, contributing to the enhancement of food shelf life. They exhibit antibacterial activity against a variety of Gram-negative and Gram-positive bacteria, in addition to possessing antioxidant properties. Due to these distinct features, researchers have developed environmentally friendly, cost-effective, and straightforward processes for their synthesis.130 AgNPs serve as antimicrobial agents in both biodegradable and non-biodegradable polymers for the production of active food packaging films.131 When incorporated into food packaging materials after necessary modifications so as make them safe and non-toxic, silver nanoparticles gradually release into food products, acting against microbes and mitigating food toxicity.132,133 They can also be embedded in active packaging films and coatings, which then release onto the food surface, effectively inhibiting microbial growth and extending food shelf life, as the food surface is considered a hotspot for microbial activity initiation. These techniques have the potential to reduce the use of preservatives, which may be harmful, contributing to the extension of shelf life and maintenance of food quality.134
3.4.5. Zinc oxide nanoparticles.
Zinc, an essential micronutrient, holds a significant role in the formulation of dietary supplements and fortified foods.135 When present in the form of ZnO nanoparticles, it generates Zn2+ ions and reactive oxygen species (ROS), which can harm cell organelles and lead to cell death. This property makes it a valuable antibacterial agent effective against a wide range of bacteria such as E. coli, Listeria monocytogens, Staphylococcus aureus, Salmonella enteritids, among others.136 When incorporated into the polymer matrix of composite films, these nanoparticles contribute to the enhancement of the mechanical, antimicrobial, and barrier properties of the films.136,137 Their ready availability in highly pure form on the market makes it easy to study their effects by integrating them into various polymer matrices.137
3.4.6. Titanium dioxide nanoparticles.
TiO2 nanoparticles, white-colored metal oxides, are utilized in various food industry applications, including food additives, and are also employed as nanocomposites in packaging.138 Their incorporation into food packaging polymers serves to block UV radiations while simultaneously enhancing the mechanical, chemical, and barrier properties of the resulting food packaging films. Due to their distinctive characteristics such as color stability, high index of refraction, and brightness, TiO2 NPs are also used as coloring agents in various processed foods.139,140 Additionally, they exhibit antimicrobial properties as they generate free radicals and reactive oxygen species (ROS) upon interaction with bacterial cells, leading to cell death.141 Owing to their environmentally friendly nature, chemical stability, cost-effectiveness, and non-toxicity, they are effective photocatalysts.138 When exposed to sunlight, TiO2 facilitates the photodegradation of ethylene, making it useful as an oxygen and ethylene scavenger.142
3.4.7. Nanoclay.
Nanoclay, a layered mineral silicate composed of octahedral and/or tetrahedral sheets, contributes to the enhancement of the physical and barrier properties of plastic food packaging materials.143 Its inclusion in these materials results in improved barrier and mechanical properties of thermoplastics, while also enhancing the biodegradability of synthetic polymers.144–147
The suitability of nanomaterials for food packaging applications is underscored by their various properties, as depicted in Fig. 20.146
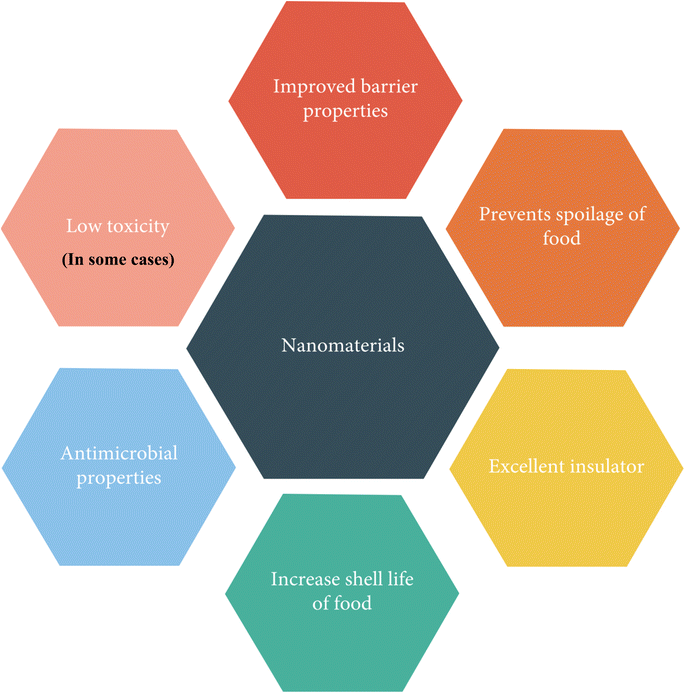 |
| Fig. 20 Properties of nanomaterials.146 | |
Nanoclays and other nanomaterials offer a range of advantages, including enhanced mechanical strength, barrier properties, and thermal stability when incorporated into various products. They can improve the durability and performance of materials such as packaging, construction materials, and automotive components. Additionally, nanomaterials often enable the development of novel functionalities, such as antimicrobial properties or self-healing capabilities, which can contribute to product innovation and sustainability efforts. Moreover, nanotechnology holds promise for advancements in fields like medicine and renewable energy, with potential applications in drug delivery systems, diagnostics, and efficient energy storage.
However, alongside these benefits, there are also notable concerns associated with the use of nanomaterials. One significant issue is the potential environmental impact, particularly when nanomaterials are released during the biodegradation of products containing them. Questions arise regarding the effects of nanoparticles on soil microorganisms and ecosystems, including their potential toxicity and long-term consequences. Furthermore, the behavior and fate of nanoparticles in the environment are still not fully understood, raising uncertainties about their ecological footprint and potential risks to human health through exposure pathways.
Additionally, the manufacturing processes involved in producing nanomaterials may have environmental implications, such as energy consumption, resource depletion, and waste generation. Concerns have been raised about the possible release of harmful by-products or contaminants during the synthesis and disposal of nanomaterials. Furthermore, there are regulatory and ethical considerations surrounding the use of nanotechnology, including questions about safety standards, risk assessment methodologies, and public perception.
Overall, while nanomaterials offer exciting possibilities for technological advancement and innovation, it is essential to carefully weigh their potential benefits against the associated risks and challenges. Effective risk management strategies, comprehensive environmental assessments, and transparent communication are crucial for ensuring the responsible development and utilization of nanotechnology in a manner that promotes sustainability and minimizes adverse impacts on human health and the environment.148
The impact of nanomaterials on microorganisms in soil is a complex and multifaceted issue that requires careful consideration. Some studies suggest that certain nanoparticles may have antimicrobial properties, potentially inhibiting the growth or activity of soil microorganisms. This could disrupt crucial ecological processes such as nutrient cycling and soil fertility, ultimately affecting plant health and ecosystem stability. On the other hand, nanomaterials might also interact with soil microorganisms in ways that enhance their growth or metabolic activity, leading to beneficial effects such as improved soil structure or enhanced degradation of organic pollutants.
However, the specific effects of nanomaterials on soil microorganisms can vary widely depending on factors such as nanoparticle composition, size, surface properties, and environmental conditions. Additionally, there is still limited research on the long-term consequences of nanoparticle exposure on soil microbial communities and ecosystem functioning. It is crucial to conduct further studies to better understand the mechanisms underlying nanoparticle–microorganism interactions and to assess the potential risks and benefits associated with the widespread use of nanomaterials in agriculture, construction, and other sectors. Ultimately, sustainable management practices and regulatory measures may be necessary to minimize any adverse impacts on soil microbial communities while maximizing the potential benefits of nanotechnology.149
Nanomaterials have ushered in a new era of tailored packaging solutions with improved functionalities. Nano cellulose, extracted from plant sources, enhances packaging strength and reduces oxygen permeability. Nano starch, derived from agricultural waste, improves mechanical properties and offers biodegradability. Furthermore, nanomaterials like protein nanoparticles, silver nanoparticles, zinc oxide nanoparticles, and titanium dioxide nanoparticles provide unique benefits. Protein nanoparticles can improve packaging strength and act as carriers for bioactive compounds. Silver nanoparticles offer antimicrobial properties, which can extend the shelf life of perishable foods. Zinc oxide and titanium dioxide nanoparticles contribute to UV-blocking and antimicrobial effects, reducing the need for synthetic additives. These advancements promote sustainability by enhancing packaging performance and reducing the reliance on synthetic materials and additives, thus aligning with consumer demands for cleaner and safer packaging.
3.5. Antimicrobial packaging
One of the primary factors contributing to the spoilage of food products is the undesirable proliferation of pathogenic and spoilage microorganisms. To counter this issue, one of the crucial strategies involves the use of antimicrobial materials, such as active substances like absorbent pads and emission sachets, among others. Most antimicrobial active encapsulation systems are formulated using compounds such as triclosan, chlorine dioxide, glucose oxidase, silver, silver zeolite, natamycin, ethanol vapor emissions, allyl isothiocyanate, and sulfur as active components.150,151 Notably, silver, platinum, gold, and copper are some of the key examples of antimicrobial agents.
Fig. 21 illustrates the coating of polypropylene/polyethylene (PP/PE) films with other polymers (cellulose fibers—CF) and their loading with antibacterial agents (nisin).152 Various techniques related to antimicrobial packaging are outlined below.
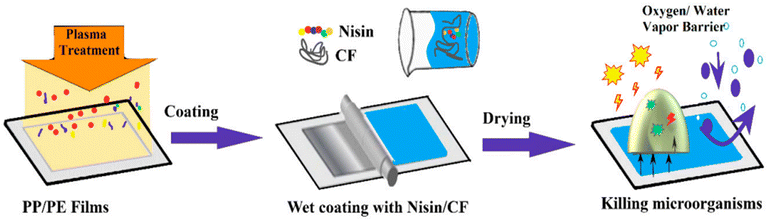 |
| Fig. 21 Coating of polypropylene/polyethylene (PP/PE) films with other polymers (cellulose fibers—CF) and loading with antibacterial agents (nisin).152 | |
3.5.1. Packaging with CO2 emitters.
CO2 is both water-soluble and lipid-soluble in food. This leads to the creation of carbonic acid, which subsequently causes the acidification of food products. Its role in the food industry is multifaceted, serving to preserve food quality and extend shelf life. The effects of CO2 on food are complex, involving alterations to bacterial cell membranes, inhibition of bacterial enzymes, and changes in cytoplasmic pH. These actions result in the extension of the lag phase and the suppression of the growth of numerous spoilage microorganisms.153
In the domain of CO2 emission technologies, baking soda (NaHCO3) and organic acids stand out as two common active ingredients. Additionally, citric acid can serve as a CO2 emitter. Combinations of various active substances, such as the amalgamation of ascorbic acid and iron carbonate, can also yield CO2 emission, along with the simultaneous consumption of O2 in a 1
:
1 ratio.154–156
Fig. 22 illustrates commonly used antimicrobial agents in food packaging applications.157
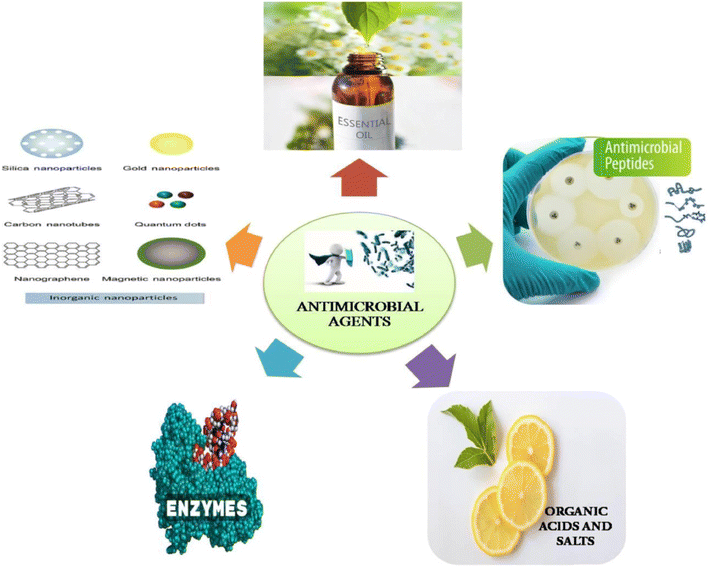 |
| Fig. 22 Commonly used antimicrobial agents in food packaging applications.157 Reproduced (adapted) with permission from ref. 157. Copyright [2021] [Elsevier]. | |
Antimicrobial substances, such as disinfectants and antibiotic drugs, are crucial for preventing and treating infections but face challenges due to antimicrobial resistance, with nearly 5 million deaths attributed to this issue in 2019. The use of disinfectants and cleaning products is increasingly recognized as a contributor to antimicrobial resistance alongside human and veterinary medicine. Certain microorganisms develop tolerance to these substances, particularly at sublethal concentrations, through mechanisms like spore formation and changes in cell membrane composition. Pseudomonas species, notably Pseudomonas aeruginosa, pose significant challenges due to their efflux pumps and ability to tolerate disinfectants like benzalkonium chloride (BAC). Resistance can extend to clinically relevant antibiotics and is influenced by environmental factors, such as the presence of other microorganisms. Studies have shown that exposure to disinfectants can enrich antimicrobial resistance genes in soil microbial communities, highlighting the need for cautious use of these substances. Moreover, antimicrobial resistance exacerbates concerns, particularly in drug-resistant pathogens like P. aeruginosa, with potential links to increased antibiotic resistance observed following the COVID-19 pandemic. These findings underscore the importance of judicious disinfectant use and vigilant monitoring to mitigate the spread of antimicrobial resistance. The potential consequences of using antimicrobial materials to prevent food waste and extend shelf life, it becomes evident that the focus on food safety must be balanced with consideration for broader environmental impacts. While antimicrobial materials play a crucial role in inhibiting the growth of harmful microorganisms, they can inadvertently affect beneficial microorganisms present in food, the human gut, and soil ecosystems. These beneficial microorganisms are essential for processes such as fermentation, digestion, and nutrient cycling, contributing to both human health and environmental sustainability. Therefore, the indiscriminate use of antimicrobial materials may disrupt these delicate microbial ecosystems, potentially leading to unintended consequences for both human and environmental health.
Moreover, the impact of antimicrobial materials extends beyond their immediate application in food packaging to their entire life cycle. Concerns have been raised regarding the biodegradability of these materials, particularly in ambient environments where conditions may differ from controlled laboratory settings. Various studies have highlighted discrepancies between biodegradability claims and actual environmental degradation rates, indicating the need for more rigorous evaluation and regulation of such materials. False certifications and claims regarding biodegradability not only mislead consumers but also contribute to environmental pollution and the accumulation of non-biodegradable waste.
Therefore, it is imperative to adopt a more holistic approach to food packaging design, one that considers the potential impacts of antimicrobial materials on microbial ecosystems, human health, and environmental sustainability throughout their entire life cycle. This approach involves not only evaluating the efficacy of antimicrobial materials in preventing food waste and extending shelf life but also assessing their long-term environmental consequences, including their biodegradability and potential for unintended ecological disruption. By integrating considerations for microbial ecology, environmental degradation, and regulatory oversight, we can develop more sustainable solutions for food packaging that prioritize both food safety and environmental stewardship.158,159
Antimicrobial packaging tackles two critical aspects of food preservation: extending shelf life and reducing the need for chemical additives. Packaging integrated with carbon dioxide emitters or scavengers modifies the package atmosphere, inhibiting microbial growth and delaying spoilage. Antioxidant agents incorporated into packaging materials prevent oxidative reactions, maintaining food quality. By employing these technologies, antimicrobial packaging reduces the likelihood of premature spoilage, thereby minimizing food waste. This aligns with sustainability goals by enhancing the efficiency of the food supply chain and reducing the environmental impact associated with discarded food. Biosensors integrated into packaging materials introduce a new dimension of functionality by allowing real-time monitoring of food quality.
3.6. Biosensor in food packaging
It is well understood that a wide array of chemical sensors is accessible for implementation in food packaging. However, in the case of biosensors, the situation differs significantly, with only a limited range finding application as intelligent food packaging materials. Among the most frequently utilized biosensors for active packaging are those designed for the direct detection of bacteria, necessitating contact with the food products (or the fluid form of food), as well as sensors for identifying volatile chemicals produced during the degradation of food products, among other functions.160 Some of the most commonly employed biosensors in food packaging are listed below.
3.6.1. Fluorescent and microfluidics biosensors.
Utilizing a solid polymer matrix in a biosensor centered on fluorescence enables the immobilization of bioluminescent or fluorescent dyes. Biosensor devices are constructed with a thin film incorporating a polymer coating of a distinct color. The luminous sensor's sensing coating operates in the presence of molecular oxygen emitted from the headspace of the package, employing a straightforward diffusion technique that facilitates a flexible light washout. The calibration curve's effects on various luminescence parameters aid in the determination of oxygen concentration.161 In this reversible technique, the fluorescence-based oxygen sensor does not yield any by-products or consume dyes during the process. Moreover, the fluorescent-based biosensors generate an array of colors upon encountering food pathogens. They can also function as electronic noses or tongues, contributing to a reduction in pathogen detection time from hours to days. Their compact size is advantageous in detecting minute substances in large volumes over time.162
3.6.2. Electrochemical biosensors.
Presently, electrochemical biosensors are extensively employed for assessing food quality based on their functionalities. Based on biological recognition, electrochemical biosensors primarily fall into two categories: affinity-based biosensors and biocatalytic sensors. Biocatalytic biosensors are utilized for identifying target biomolecules, employing redox enzymes, tissue slices, or entire cells as recognition materials in the process.163 In affinity-based sensors, recognition materials include antibodies, aptamers, and antibody fragments. The distinctive properties and benefits of biocatalytic biosensor devices, such as their uncomplicated design, ease of use, cost-effectiveness, compact size, and frequently absent additional equipment, make them readily adaptable with packaging materials.164 Additionally, these biosensors demonstrate exceptional specificity for the target substance, obviating the need for pretreatment or separation processes. Advantages of electrochemical biosensors include lower detection limits, reduced background signal, and a straightforward sensor approach.
One prominent instance of an electrochemical biosensor for foods documented in the literature is the biosensor based on single-walled carbon nanotubes (SWCNT) for detecting food microbes.165,166 Other examples of electrochemical biosensors involve a biosensor relying on diamine oxidase for amine measurements in packed food present in the atmosphere, as well as a biosensor centered on DNA for detecting potential carcinogens in food samples.167
3.6.3. Gas sensors.
Gas sensors are instrumental in detecting gas leaks in packaging and assessing food quality. They also aid in identifying the presence of spoilage gases, such as basic oxygen, nitrogen compounds, and carbon dioxide, which are emitted during food spoilage.168 Moreover, they can be employed for detecting pesticides like carbamides in fruits and vegetables, serving as a rapid, sensitive alternative for testing the rancidity of meat products. A gas sensor typically comprises three components: the sensing electrode, the counter electrode, and the reference electrode. This sensor operates based on the detection of CO2 compounds within food containers. It exhibits robust functionality even in hazardous environments and specifically targets gas molecules, remaining unaffected by electromagnetic interferences. These distinct characteristics of gas sensors position them as pivotal choices for the food packaging industry.169Fig. 23 illustrates the principles of ECL biosensors, ECL luminophores, and their ECL applications in food analysis.170 We can incorporate such sensors in food packaging materials for advanced applications.
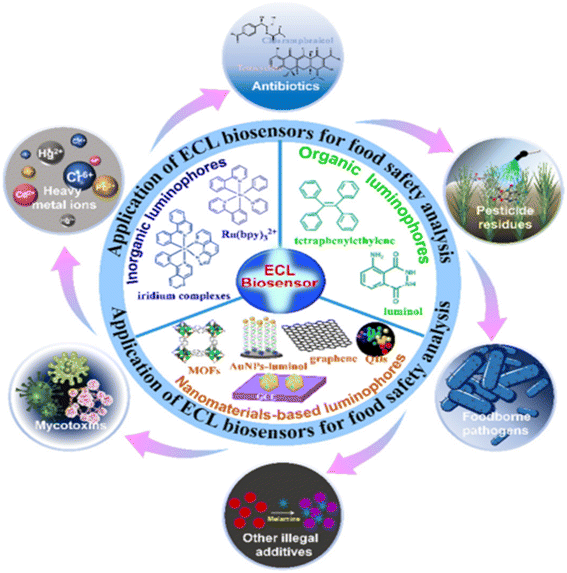 |
| Fig. 23 Principles of ECL biosensors, ECL luminophores, and their ECL applications in food analysis.170 | |
Fluorescent and microfluidics biosensors detect specific spoilage indicators, providing consumers with timely information about food freshness. Electrochemical biosensors gauge freshness based on parameters like pH and gas emissions. These biosensors empower consumers to make informed decisions about the safety and quality of their food purchases. By reducing instances of consuming spoiled food, biosensors contribute to sustainability by reducing food waste and encouraging responsible consumption.
3.7. Chitosan-based films for food packaging
Chitosan-based films have been the subject of extensive research for several years due to their distinctive and significant applications in the food industry, contributing to the reduction of environmental impacts.171–173 These films serve as effective food packaging materials, aiding in the extension of the shelf life of food.174–189 Various types of chitosan-based films are utilized in the realm of the food packaging industry, offering diverse applications.
3.7.1. Chitosan/biopolymer-based films.
Due to their distinct and significant characteristics, naturally derived biopolymers are suitable for blending with chitosan to create chitosan/biopolymer films. These biopolymers, which include polysaccharides, organic acids, extracts, proteins, among others, offer attributes such as nontoxicity, biocompatibility, and biodegradability. The resulting films have found significant applications in the food packaging industry.190
3.7.2. Chitosan/polysaccharides-based films.
Composite films with chitosan can be created by combining polysaccharides, resulting in functional films. Starch, a renewable polysaccharide derived from plants, is one of the most important options due to its widespread availability, low cost, and film-forming capability. The resulting composite films with chitosan exhibit distinct properties, including notable antioxidant activity, improved water vapor barrier properties, and reduced bacterial adhesion on the packaging, making them highly effective for food packaging applications.191–193 Numerous researchers have reported that cellulose/chitosan films offer enhanced mechanical properties, which can be attributed to the hydrogen bonds between the nanocellulose and chitosan. These films also demonstrate excellent antimicrobial, gas barrier, optical, and bioactive properties, alongside sustainability.194–198 Additionally, the chitosan/carboxymethyl film, formed through electrostatic interactions, has been shown to extend the shelf life of wheat bread and cheese.199,200 Other cellulose materials, such as chitosan/alginate, chitosan/pectin, and chitosan/cyclodextrin, can also be utilized to synthesize composite films with chitosan, offering diverse applications and properties.201–211
3.7.3. Chitosan/protein-based films.
An array of proteins sourced from plants, animals, and microorganisms can be blended with chitosan to create films. These chitosan/protein films possess distinct functional groups that enable them to be applied in various areas, including food packaging.212–225 Proteins derived from animals, in particular, have garnered significant interest in research due to their notable properties such as film-forming ability, biocompatibility, and high nutritive value. Several proteins exhibit these unique attributes, allowing them to be combined with chitosan to produce films with diverse functionalities in food packaging. Notable examples include chitosan/caseinate, chitosan/collagen, chitosan/lysozyme, chitosan/gelatin, chitosan/kidney bean protein, chitosan/quinoa protein, chitosan/nisin, and chitosan/ε-polylysine/nisin films.212–225 These chitosan-based films serve various purposes in the food packaging industry, acting as antimicrobial agents, extending the shelf life of food, and improving food quality, among other functions.
3.7.4. Chitosan/extracts-based films.
Chitosan can be combined with extracts from bee secretions, such as beeswax and propolis, to produce films for food packaging.226 Chitosan/beeswax films are known for their environmentally friendly nature and their ability to regulate pathogenic microorganisms while preserving food quality.227,228 Natural and non-toxic extracts from plants have been utilized in the food industry for decades, particularly in food preservation. When incorporated into chitosan films, these plant extracts significantly enhance various properties, including antimicrobial activity (e.g., honeysuckle flower and citrus extracts),229,230 antioxidant activity (e.g., clove eugenol and maqui berry extracts),231,232 barrier performance (e.g., thyme extract),233 mechanical property (e.g., tannic acid),234 thermal stability (e.g., young apple polyphenols),235 and color property (e.g., carvacrol).236 Furthermore, the free radical-initiated grafting of gallic acid onto chitosan through carbodiimide (as shown in Fig. 24)237,238 significantly enhances various film properties, including increased tensile strength, antioxidant capacity, and antimicrobial activity, as well as decreased oxygen and water vapor permeability.237–241 This makes it an excellent candidate for multifunctional packaging applications in the food industry.
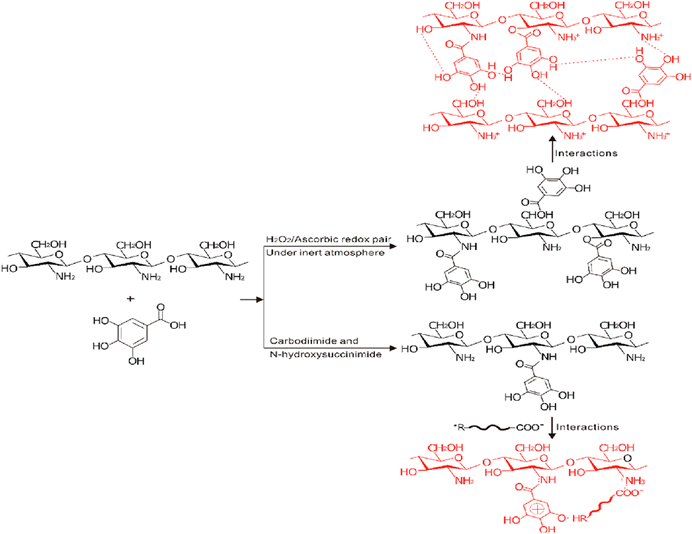 |
| Fig. 24 Grafting of gallic acid onto chitosan.196 Reproduced (adapted) with permission from ref. 196. Copyright [2014] [ACS]. | |
Plant-derived essential oils, known for their aromatic and volatile nature, can be extracted and incorporated into chitosan films, significantly enhancing their properties. For instance, films containing chitosan and cinnamon oil extract demonstrated remarkable antimicrobial efficacy, making them promising candidates for active food packaging.242 Numerous other essential oils have also been shown to enhance various aspects of chitosan films, including barrier properties, mechanical properties, and antioxidant activity.243,244
3.7.5. Chitosan/synthetic polymers-based films.
When chitosan is combined with synthetic polymers to create films, there is a notable enhancement in the properties and applications of the resulting films. Incorporating poly(vinyl alcohol), known for its robust mechanical strength, into chitosan facilitates the fabrication of films.245 Higher concentrations of poly(vinyl alcohol) contribute to plasticization, increased elasticity, elevated tensile strength, and improved oxygen and water barrier properties in the films, which also exhibit potential antimicrobial activity in food packaging applications.245,246 Incorporating or immobilizing certain bioactive materials in chitosan/poly(vinyl alcohol) films can imbue them with unique attributes such as mechanical durability and fire resistance, thereby broadening their potential applications.247 Additionally, various other synthetic materials can be blended with chitosan films to enhance their characteristics and applications in the food packaging industry. Examples include chitosan/poly(lactic acid) films,248,249 chitosan/salicylic acid films, chitosan/fumaric acid films,250 chitosan/low-density polyethylene films,251,252 chitosan/poly(ethylene oxide) films,253 chitosan/allyl isothiocyanate films, and chitosan/polycaprolactone films.254,255Fig. 25 illustrates composite films with lactic acid oligomer-grafted chitosan as a nanofiller, demonstrating significant and promising enhancements in properties of food packaging materials, including tensile strength and thermal properties, through the use of such nanofillers.256
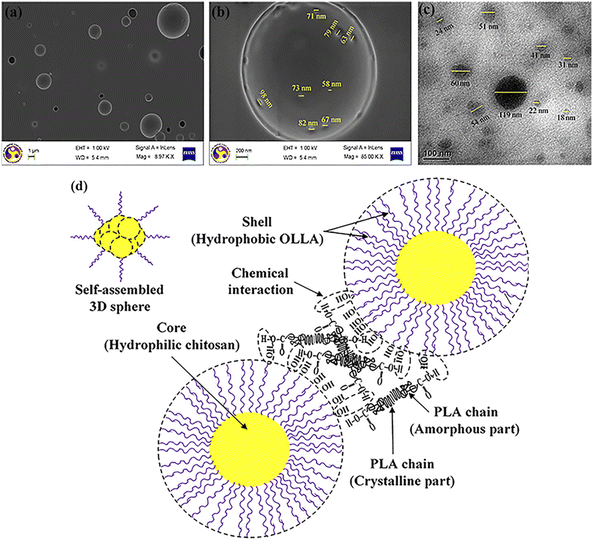 |
| Fig. 25 FESEM images of PLA/OLLA-g-CH (5%) bionanocomposite film (a) at 8.97 KX, (b) at higher magnification ∼85 KX, (c) TEM topography of PLA/OLLA-g-CH (5%) bionanocomposite film and (d) schematic representation of interaction between matrix and filler.256 Reproduced (adapted) with permission from ref. 256. Copyright [2016] [ACS]. | |
3.7.6. Chitosan/inorganic materials-based films.
One of the most important characteristics of chitosan is their high chelating ability which makes it to be used for the synthesis of a large number of chitosan–inorganic complexes based films (Fig. 26). Such films can be widely used in food packaging applications.197
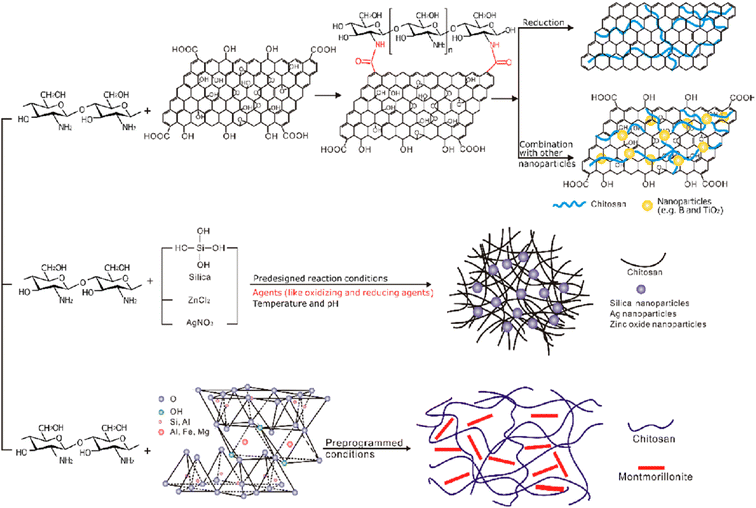 |
| Fig. 26 Preparation of chitosan–inorganic complexes.197 Reproduced (adapted) with permission from ref. 197. Copyright [2013] [Elsevier]. | |
Given the antimicrobial effects of silver nanoparticles on various pathogenic microorganisms, they can be integrated into chitosan films for active use in food packaging. Employing green chemistry methodologies allows for the even distribution of chitosan and silver nanoparticles within the polymer matrix.257,258 These films have numerous applications in edible food packaging due to the improved hydrophilic nature, antimicrobial activity, biocompatibility, non-toxicity, and degradability of chitosan and silver nanoparticles films.259,260 Zinc oxide nanofillers are another material that enhances the biological and physicochemical properties, such as transparency, antimicrobial characteristics, and mechanical strength of composite films.261,262 They prevent the growth of food pathogens and extend the shelf life of food, making them highly useful in the food industry.263
Graphene oxide and expanded graphite stacks also significantly enhance the properties of composite films compared to pristine chitosan films. These composite films, comprising graphene oxide, expanded graphite stacks, and chitosan, demonstrate improved mechanical capacity, oxygen barrier properties, and antimicrobial characteristics, making them valuable for food packaging.264–267 The barrier properties, including oxygen, carbon dioxide, and water vapor properties, are greatly improved in chitosan/montmorillonite composite films.268–271 Additionally, chitosan/montmorillonite composite films exhibit enhanced mechanical properties and improved flame-retardant characteristics, rendering them suitable for food packaging applications.272–274 Incorporating various other inorganic materials into chitosan films also enhances their properties. Examples of these materials include silicon materials, titanium dioxide, nanomagnesium oxide, cloisite 20 A, CaCO3, CaCl2, cerium(IV), and zinc(II).266,275–279 These chitosan/inorganic complexes based composite films possess unique and crucial attributes and applications, such as reducing the food decay rate, enhancing thermal stability, strengthening chemical resistance, and more, making them significant candidates for food packaging applications.280–285
3.7.7. Chitosan/chitosan derivatives-based films.
Chitosan, which contains a significant number of hydroxyl and amino groups, can be transformed into chitosan derivatives (Fig. 27), possessing additional properties compared to regular chitosan.286 Combining chitosan with these derivatives allows the creation of edible films, offering a wide array of distinctive applications in the realm of food packaging.287–295
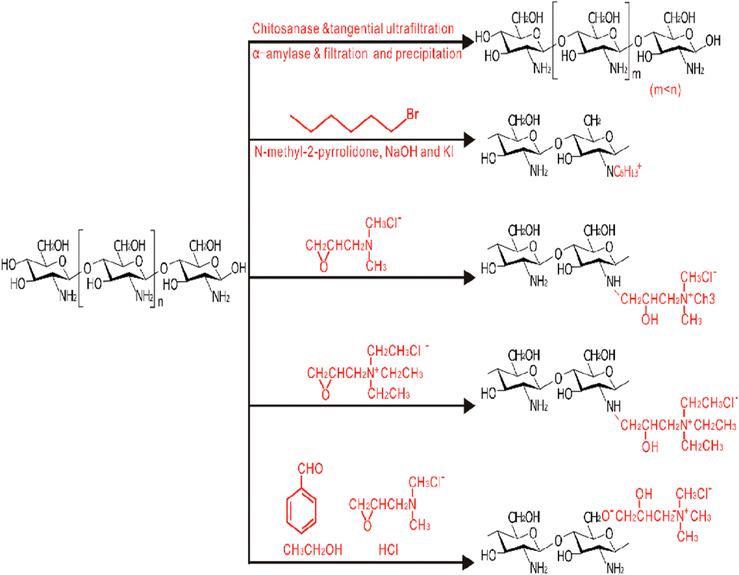 |
| Fig. 27 Chitosan derivative development of fabrication of chitosan/chitosan derivatives-based films.197 Reproduced (adapted) with permission from ref. 197. Copyright [2013] [Elsevier]. | |
Fig. 28 represents different methods of fabrication of chitosan-based films.
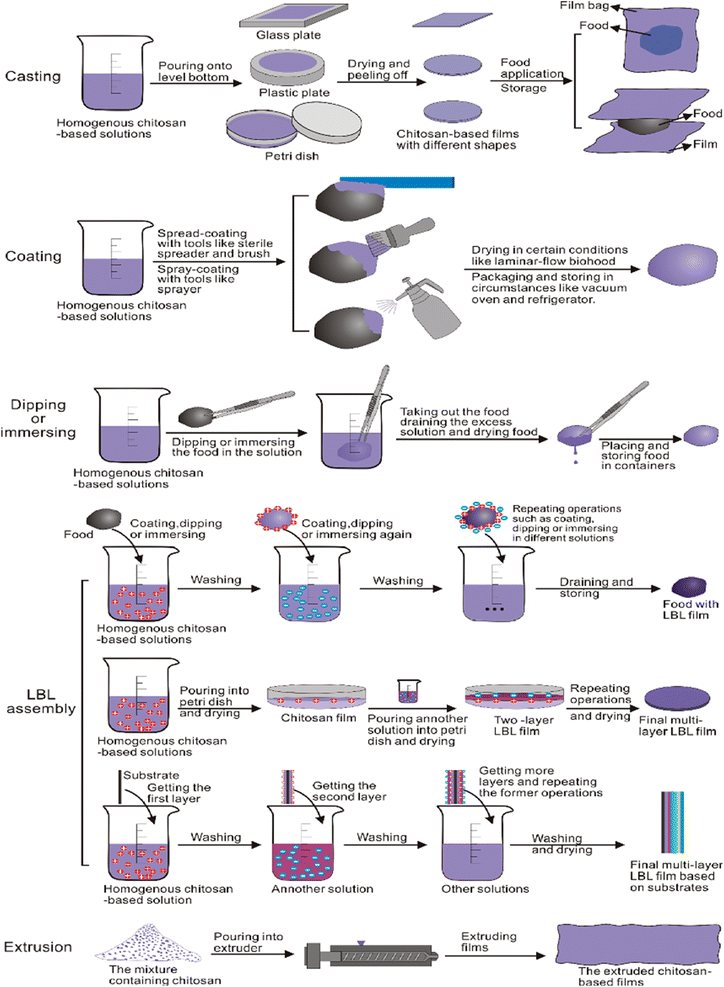 |
| Fig. 28 Different methods of fabrication of chitosan-based films.197 Reproduced (adapted) with permission from ref. 197. Copyright [2013] [Elsevier]. | |
Fig. 29 represents the antimicrobial mechanism of chitosan films.296–310
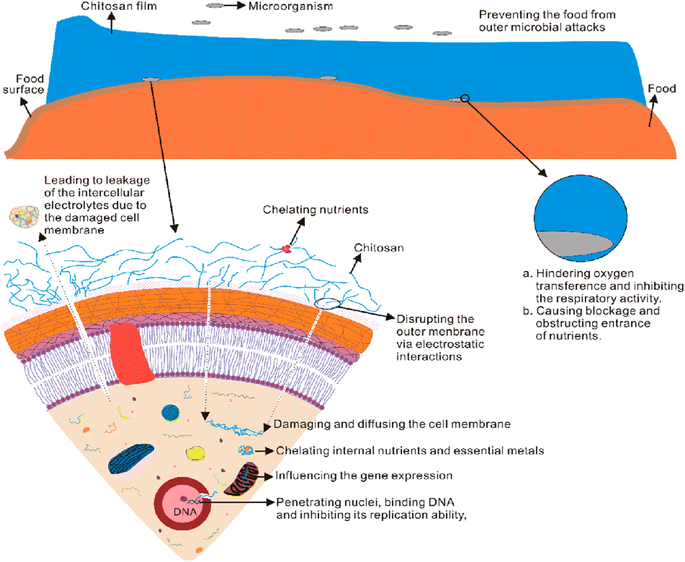 |
| Fig. 29 Antimicrobial mechanism of chitosan films.197 Reproduced (adapted) with permission from ref. 197. Copyright [2013] [Elsevier]. | |
Fig. 30 represents sensing film application as a sticker sensor for pork & fish freshness.311
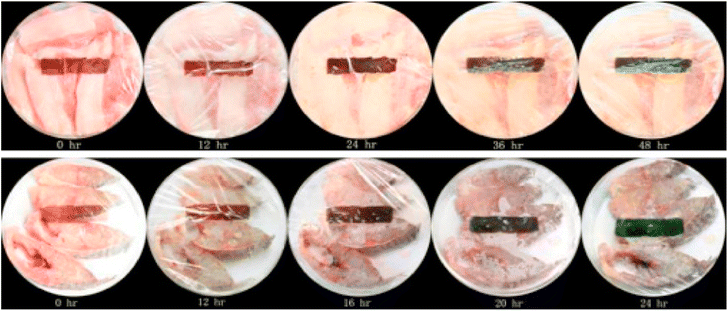 |
| Fig. 30 Sensing film application as a sticker sensor for pork & fish freshness.311 Reproduced (adapted) with permission from ref. 311. Copyright [2014] [Elsevier]. | |
Chitosan, a biopolymer derived from shellfish exoskeletons, has emerged as a versatile material for food packaging. Chitosan-based films offer a range of functionalities, from biodegradability to antimicrobial properties. These films can be tailored to meet specific packaging needs and contribute to sustainability on multiple fronts. Pure chitosan films exhibit biodegradability and biocompatibility, making them suitable for single-use applications. Chitosan/biopolymer-based films combine chitosan with other natural polymers, enhancing mechanical strength and barrier properties. Chitosan/protein-based films can act as carriers for bioactive compounds, enhancing food safety and quality. Inorganic materials can be incorporated into chitosan films to introduce novel functionalities like UV-blocking. The antimicrobial properties of chitosan are particularly valuable in food packaging. By reducing the growth of spoilage microorganisms, chitosan-based films extend the shelf life of packaged products. This contributes to sustainability by minimizing food waste and enhancing the efficiency of the food supply chain.
Some other important biobased polymers in food packaging applications are presented in Table 3.
Table 3 Important biobased polymers in food packaging applications
Biopolymer used |
Additive added |
Synthesis method |
Food packaged |
Main characteristics |
References |
Polylactic acid |
Nano-zinc oxide (ZnONPs), pomegranate peel extract |
Solvent-casting method |
Cherry tomatoes |
Increased UV barrier, WVP, elongation at break, good antibacterial and antioxidant activity |
312
|
Polylactic acid |
Zein, polyethylene glycol (plasticizer) |
Blended method |
None used |
Improved mechanical and water barrier properties |
313
|
Polylactic acid |
Cerium lactate (Ce-LA) |
Precipitation method |
None used |
Increased crystallinity and enhanced antibacterial properties |
314
|
Yam starch |
Bentonite |
Bentonite |
None used |
Increased tensile strength, high degradability |
315
|
Starch-based bioplastics |
2,2,6,6-Tetramethylpiperidine 1-oxy-oxidized cellulose nanofibers and zinc oxide nanoparticles |
Not clearly defined (possibly gel-formation) |
None used |
Improved tensile strength (up to 24.54 MPa), water resistance, thermal resistance, biodegradability |
316
|
Yam starch |
Glycerol (plasticizer), citric acid |
Gel formation |
Fish |
High tensile strength (24.60 MPa), smart pH auto color detection |
317
|
Pectin |
Spent coffee grounds extracts, PHA (coating) |
Dipping method |
Mashed carrot |
Improved water vapor permeability |
318
|
Alginate |
Nitrogen carbon dots (NCDs), layered clay |
Casting method |
Banana |
Improved mechanical property and reduced surface wettability |
319
|
Protein and pectin (yellow passion fruit peel) |
None mentioned |
Casting method |
None used |
Improved strength and elasticity, improved thermal stability, low water permeability |
320
|
Alginate |
Citrus lemon extract |
In situ method |
None used |
Good antibiofilm effect |
321
|
3.8. Hydrogel based food packaging
Hydrogel-based food packaging is an emerging area of research, encompassing various applications such as antimicrobial activity, antioxidant properties, and coatings, all of which contribute to enhancing the shelf life and overall quality of food products.322 Hydrogels, three-dimensional polymeric materials with the capacity to absorb and swell in fluids, owe their water-absorbing ability to the presence of hydrophilic groups like –COOH, –OH, –CONH–, –CONH2, and SO3H.323 These hydrogels can be derived from natural or synthetic polymers, held together by either physical or chemical bonds, and their 3D structure enables them to endure the pressure generated during swelling. If they can absorb fluid of over 100% of their dry weight, they are referred to as superabsorbent hydrogels.323–325 Hydrogels can be classified based on parameters like biodegradability, crosslinking type, physical appearance, electric charge, polymer composition, responsiveness to stimuli, and more. When dissolved in solvents, they can function as colloidal gels with distinctive rheological and viscoelastic properties. Natural materials like mucilages, proteins, gums, and starch can be utilized in the formation of hydrogels, with gums and mucilages serving as protective substances produced by plants in response to environmental stress and microbial threats.326,327Fig. 31 illustrates the various methods of hydrogel synthesis,328 while Fig. 32 depicts different polymerization techniques utilized in the creation of hydrogels.329
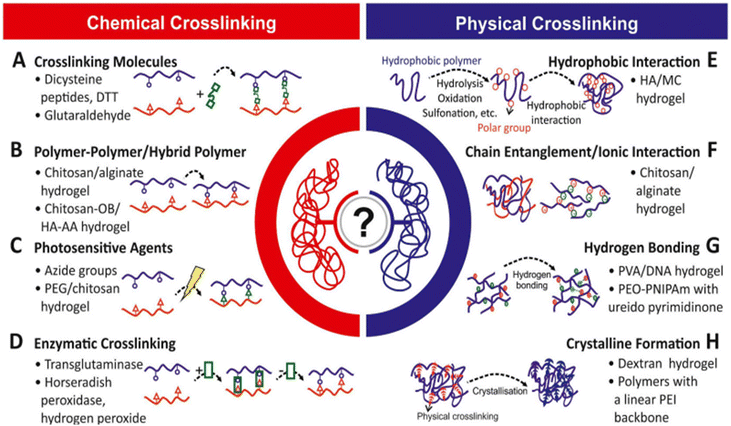 |
| Fig. 31 Methods of hydrogel synthesis: (A) crosslinking molecules, (B) polymer–polymer/hybrid polymer, (C) photosensitive agents, (D) enzymatic crosslinking, (E) hydrophobic interaction, (F) chain entanglement/ionic interaction, (G) hydrogen bonding, and (H) crystalline formation.328 | |
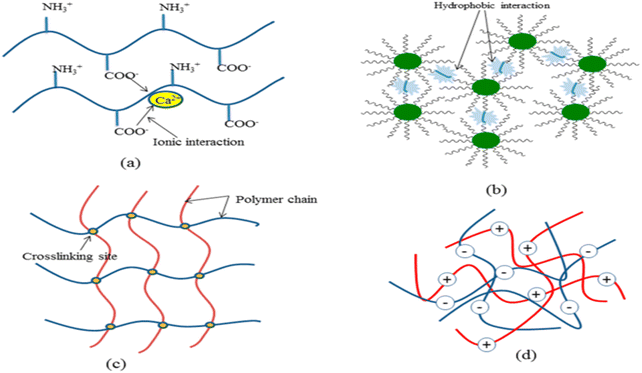 |
| Fig. 32 Different polymerization techniques for the synthesis of hydrogels: (a) ionic interaction, (b) hydrophobic interaction, (c) cross-linking junction by cooling, and (d) complex coacervate.329 Reproduced (adapted) with permission from ref. 329. Copyright [2018] [ACS]. | |
Some of the most important uses of hydrogels in food packaging are discussed below.
3.8.1. Absorbent pads.
Hydrogels are utilized in active food packaging systems as moisture absorbents for food items with high moisture content. They are particularly suitable for products that release exudates during storage. By absorbing these exudates, hydrogel-based absorbents reduce the spoilage rate of packaged food, maintaining a low internal moisture level within the package. The effectiveness of an absorbent pad hinges on its capacity to hold exudates within its 3D structure, thereby preserving the structural integrity of the packaging and extending the shelf life of the enclosed product. Typically, an absorbent pad comprises two layers: an outer layer and an inner layer. The outer layer serves to prevent direct contact between the food and the absorbent material's inner layer. Tiny openings or holes in the outer layer facilitate the flow of exudates into the inner absorbent layer. As illustrated in Fig. 33,324,330 the absorbent material swells upon absorption, enabling it to retain the exudate within its 3D structure.
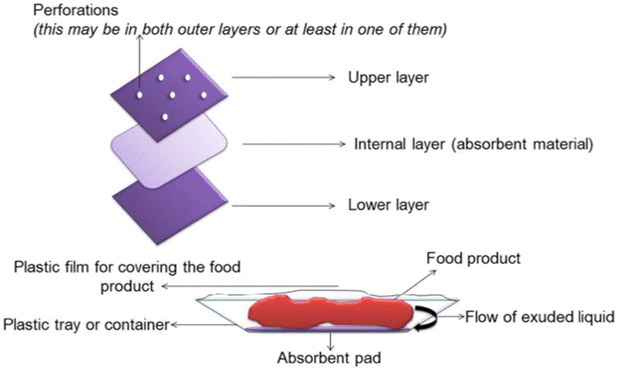 |
| Fig. 33 Absorbent pad.330 Reproduced (adapted) with permission from ref. 330. Copyright [2016] [Elsevier]. | |
3.8.2. Colorimetric indicator.
Hydrogel-based colorimetric indicators for food packaging use water-absorbing polymeric materials combined with natural extracts to monitor food quality. Composed of ingredients like gelatin, citric acid, N,N-dimethyl acrylamide, and natural pigments such as Basilicum extract, these hydrogels change color in response to spoilage or contamination, providing a visual indication of food freshness. This technology enhances food safety by extending shelf life through antimicrobial and antioxidant properties, while promoting environmental sustainability as the materials are biodegradable and reduce reliance on synthetic dyes. Additionally, using natural pigments decreases health risks associated with synthetic dyes. Despite challenges in scalability, cost, regulatory approval, and maintaining stability, hydrogel-based colorimetric indicators offer a promising solution for intelligent food packaging, aligning with goals of sustainability and consumer safety.331,332 Lu et al. reported a sugarcane bagasse nanocellulose-based hydrogel as a freshness indicator for chicken, as depicted in Fig. 34.333
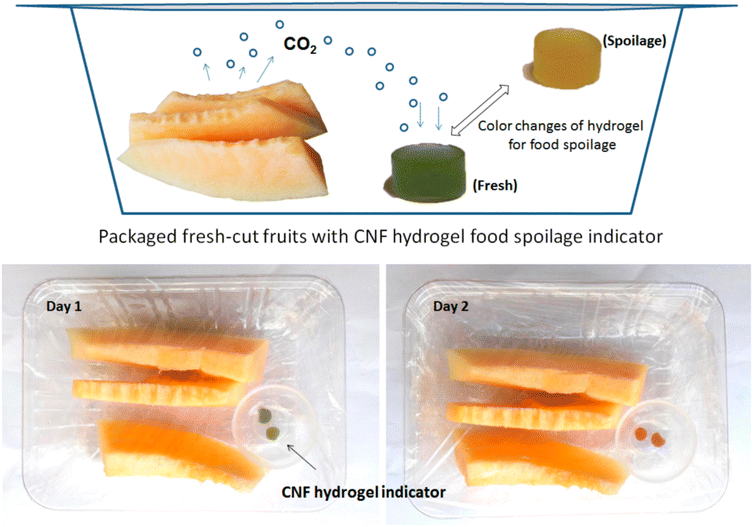 |
| Fig. 34 Cellulose nanofibrils hydrogel.333 | |
3.8.3. Antimicrobial property.
Hydrogels, when combined with antimicrobial agents like bioactive compounds or nanoparticles, exhibit antimicrobial properties. Alpaslan investigated the efficacy of Basilicum extract's antimicrobial activity against E. coli, B. subtilis, and S. aureus, finding notable antimicrobial effectiveness.332 Similarly, Wang and Rhim developed an agar/alginate/collagen ternary blend incorporated with grapefruit seed extract and silver nanoparticles. Their hydrogel film was tested against Listeria monocytogenes and Escherichia coli, demonstrating greater activity against the latter compared to the former.324,334–336
3.8.4. Antioxidant property.
The addition of antioxidant materials to hydrogel films enhances their antioxidant properties. An instance of this is the use of ferulic acid-based antioxidant film to prevent butter oxidation.337 Additionally, the incorporation of Basilicum extracts into hydrogels significantly improves their antioxidant activity.332
3.8.5. Biodegradable packaging.
The biodegradation of some hydrogels can be done because of their biopolymeric nature as they contain bio-polysaccharides in their constituents and their structures contain glycosidic bonds degradation of which can be done by enzymatic action.338 One of the most important and widely used biopolymers for the development of hydrogel matrices is chitosan. Important linkages which are targeted by enzymatic action include N-acetyl-glucosamine-N-acetyl-glucosamine, glucosamine–glucosamine and glucosamine-N-acetyl-glucosamine. The enzymes which are responsible for chitosan biodegradation include lysozyme and bacterial enzymes. The degree of deacetylation determines the extent of chitosan degradation, since low biodegradation results from a high level of deacetylation.339
3.8.6. Other potential applications of hydrogels in food industry.
Table 4 (ref. 344) shows some other potential applications of hydrogels in the food industry.
Table 4 Potential applications of hydrogels in the food industry
Main goal |
Main characteristic |
Hydrogel composition |
Reference |
Food freshness indicator |
Generated information regarding food freshness based on metabolites production in the food |
Poly(N,N-dimethyl acrylamide-co-methacryloyl sulfadimethoxine) (poly(DMA-co-SDM)) hydrogels incorporated with methacryloyl sulfadimethoxine monomer (SDM) with a pH-responsive group |
340
|
Stability and retention of volatiles substances |
Flavor encapsulation (nanoemulsions in hydrogels) |
Flavored nanoemulsions incorporated in low methoxyl (LM) pectin & whey protein isolate (WPI) at pH 4.0 |
341
|
Controlled release occurs by pH modification |
Orange oil, medium-chain triglyceride (MCT) oil, & WPI (stable nanoemulsions) |
Improvement of bioavailability of lipophilic compounds |
Incorporation of lipophilic bioactive compounds (e.g. β-carotene) in food matrix, improving their bioavailability |
Hydrogels based on polysaccharides (starch and xanthan gum) to incorporate β-carotene emulsion |
342
|
Method for aflatoxin B1 detection |
Detection of aflatoxin B1 in food sample when the hydrogel causes collapse of the network, and occurs the release of urease into the analyzed solution |
DNA hydrogel |
343
|
Hydrogels, three-dimensional networks of hydrophilic polymers, have found applications in food packaging that promote sustainability. Absorbent pads made from hydrogels manage excess moisture within food packages, preventing spoilage and maintaining food quality. By controlling moisture levels, these pads minimize microbial growth and the potential for mold formation. Colorimetric indicators incorporated into hydrogels offer a visual indication of food freshness. These indicators react to specific compounds released during food spoilage, providing consumers with a simple and intuitive tool to assess food quality. This reduces unnecessary food waste and empowers consumers to make informed choices. Hydrogels can also possess antimicrobial and antioxidant properties. This contributes to food preservation by minimizing microbial growth and oxidative reactions. Additionally, the biodegradability of hydrogels aligns with sustainability goals, ensuring that packaging waste does not linger in the environment.
3.9. Biodegradable plastics in food packaging
Environmentally friendly biodegradable plastics make up just 1% of the yearly production and readily decompose in the environment through a fragmentation process.345 The different stages of plastic degradation are depicted in Fig. 35,346 while Fig. 36 illustrates the typical mechanism of plastic degradation under aerobic conditions.347
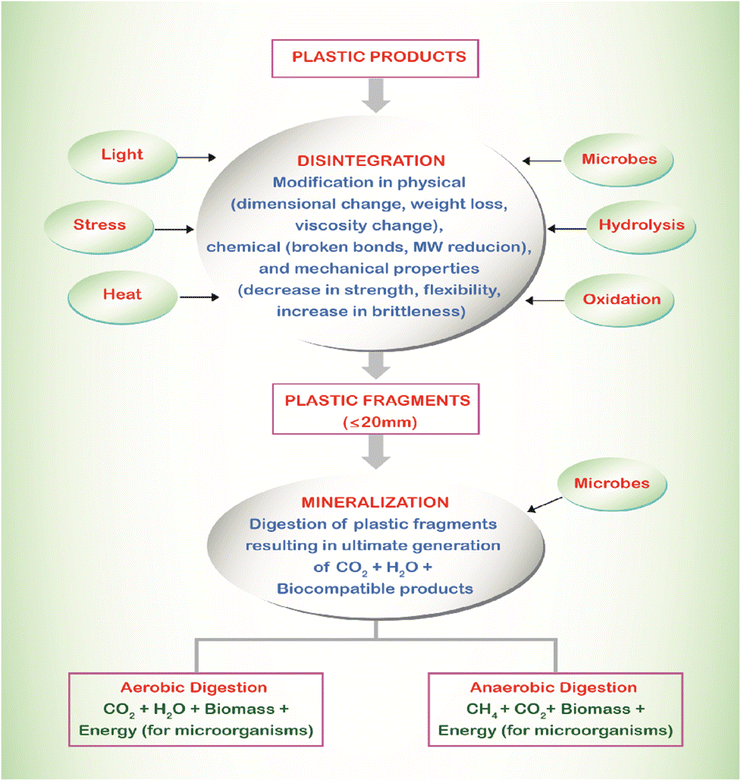 |
| Fig. 35 Schematic representation of plastic degradation.346 Reproduced (adapted) with permission from ref. 346. Copyright [2006] [Elsevier]. | |
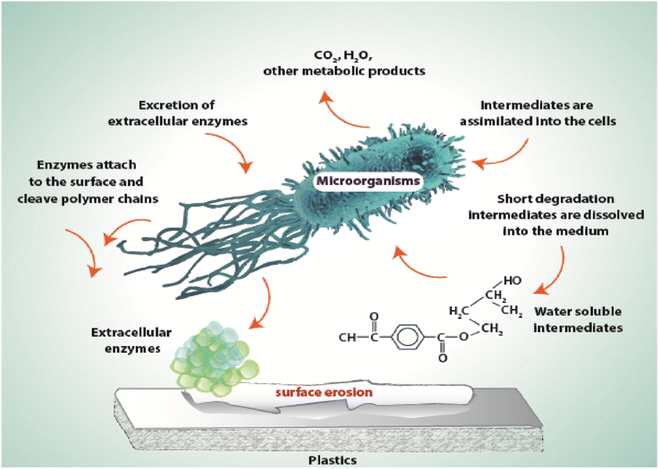 |
| Fig. 36 General mechanism of plastic degradation under aerobic conditions.347 Reproduced (adapted) with permission from ref. 347. Copyright [2005] [John Wiley and Sons]. | |
The factors which affect the degradation of plastics include chemical characteristics; raw ingredients used and the finished product's design beside climatic conditions such as temperature and location (Fig. 37).
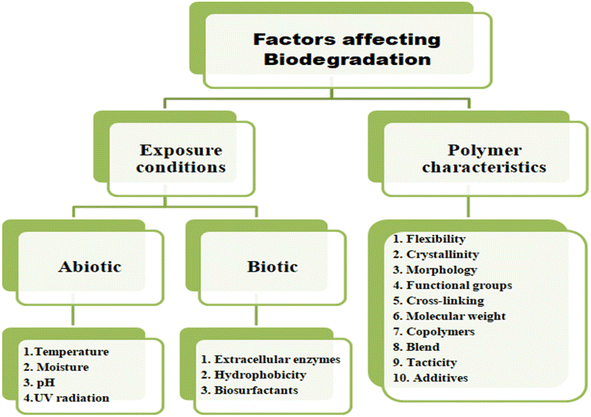 |
| Fig. 37 Factors affecting biodegradation. | |
The determination of the biodegradability of packaging materials can rely on the following characteristics:348
(a) To achieve up to 90% biodegradability, the product should consist of 50% organic mass.
(b) It should not contain heavy metals beyond the permissible health limits.
(c) The CO2 amount should allow for permeability both inside and outside the packaging material.
(d) A low oxygen permeability coefficient contributes to an increased shelf life of food.349
(e) It should possess high tensile strength, which can be enhanced by incorporating nanoparticles like PCL and PLA.350
(f) A smooth surface can be achieved by introducing biosurfactants such as polysaccharide-lipid complexes, phospholipids, and lipoproteins.351
(g) Additives, including colorants, agricultural waste, cellulose nanofibers, nanocomposites, dialdehyde starch, silica, edible oils, natural rubber, and soy protein, can improve biodegradability.352,353
The biodegradable plastics are categorized into two groups, as illustrated in Fig. 38.354
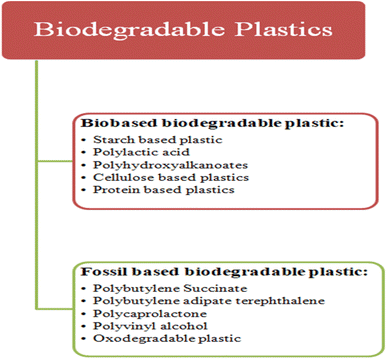 |
| Fig. 38 Classification of biodegradable plastics. | |
Fig. 39 represents various approaches of barrier biodegradable packaging.355
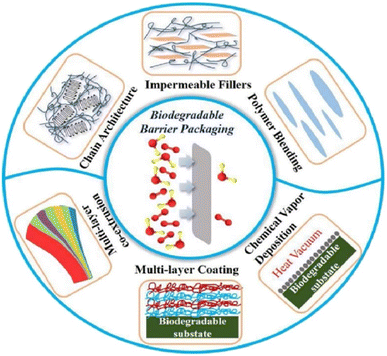 |
| Fig. 39 High barrier biodegradable packaging approaches.355 Reproduced (adapted) with permission from ref. 355. Copyright [2021] [Elsevier]. | |
Biodegradable plastics have become a crucial component in the food packaging sector, not only providing appealing packaging but also extending the shelf life of food products. These plastics are used in various forms of packaging, including net coverings for fruits, rigid beverage bottles, and egg trays. Different biodegradable polymers offer diverse applications in the food processing and packaging industry, with their combination often leading to material enhancement.356 For instance, the combination of cassava starch and sugarcane bagasse, along with other polysaccharides like orange bagasse and corn husk, has demonstrated greater resistance and rigidity in trays compared to EPA trays. Similarly, a high-quality food packaging material can be achieved through the blending of PHA with PHB.357
Incorporating PHA/ZnO and poly(3-hydroxybutyrate-co-3-hydroxyvalerate) with wood fiber improves tensile strength and elasticity, resulting in the formation of a thin film suitable for packaging junk food and wrapped meals.358 The blending of alginate and cellulose also yields notable properties and applications. In the contemporary context, biodegradable films are extensively used as substitutes for plastic (PE) in packaging materials. Additives, including enzymes facilitating the breakdown of plastic materials, are often introduced during the film formation process. These biodegradable films exhibit desirable properties that make them superior to PE films.359 These include controlled respiration allowance, acting as effective barriers, maintaining structural integrity, and preventing and reducing microbiological spoilage. Fig. 40 provides an illustration of the polyethylene biodegradation process.360
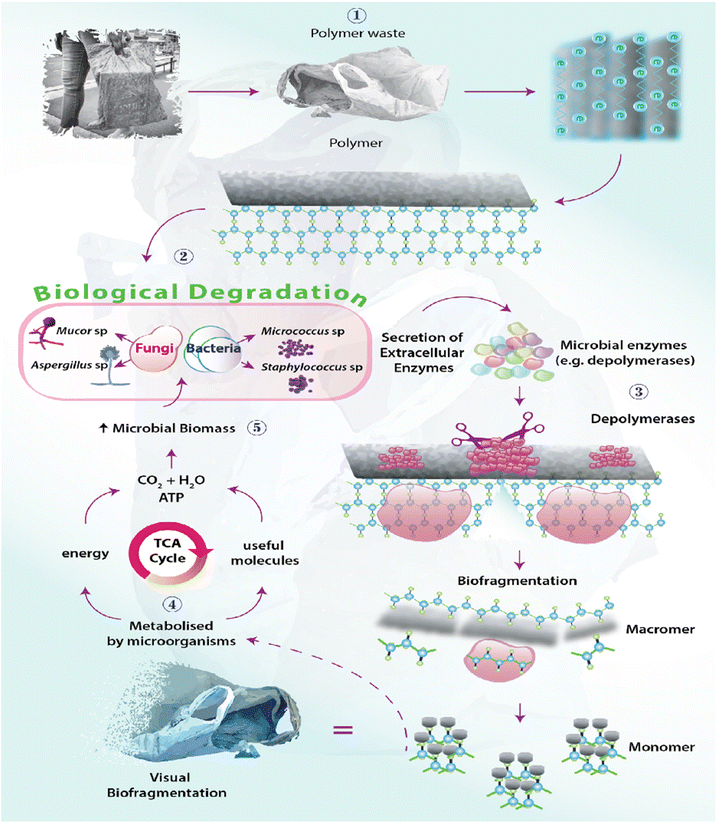 |
| Fig. 40 Biodegradation of polyethylene.360 Reproduced (adapted) with permission from ref. 360. Copyright [2021] [Elsevier]. | |
3.9.1 Biodegradable vs. shelf-life.
Finding a delicate balance between the objectives of biodegradability and extended shelf life in food packaging poses a complex challenge. Biodegradable materials offer a promising avenue for reducing environmental impact by breaking down into harmless compounds over time, thus minimizing long-term pollution and waste accumulation. These materials typically utilize organic compounds derived from renewable sources such as plant-based polymers or bioplastics, which decompose naturally through biological processes. However, the inherent properties of biodegradable materials often result in a trade-off with shelf life. Due to their susceptibility to degradation, biodegradable packaging may have a shorter lifespan compared to conventional packaging materials. This limitation raises concerns about the potential for premature product spoilage and increased food waste if items deteriorate before reaching consumers.
Conversely, packaging materials engineered for extended shelf life prioritize food preservation and longevity, aiming to minimize spoilage and maximize product freshness. These materials often incorporate synthetic polymers or additives that provide barriers against moisture, oxygen, and microbial contamination, thereby prolonging the shelf life of packaged goods. While effective in preserving food quality and reducing waste at the consumer level, these materials present challenges in terms of environmental sustainability. Their resistance to degradation means they persist in the environment long after their intended use, contributing to pollution, litter, and ecosystem harm.
Achieving a harmonious convergence between biodegradability and extended shelf life requires innovative strategies that reconcile conflicting priorities. This could involve the development of biodegradable materials with enhanced durability and protective properties, effectively extending their useful lifespan without compromising their ability to break down naturally. Alternatively, integrating shelf-life-extending technologies, such as modified atmosphere packaging or active packaging systems, into biodegradable materials may offer a viable solution by preserving product freshness while still allowing for eventual decomposition.
Furthermore, comprehensive life cycle assessments are essential for evaluating the environmental impacts of different packaging options holistically. These assessments consider not only the end-of-life disposal of packaging but also the energy consumption, resource use, and emissions associated with manufacturing, transportation, and usage stages. By adopting a holistic approach that considers the entire life cycle of packaging materials, including their biodegradability, shelf life, and environmental footprint, stakeholders can make informed decisions that promote both sustainability and food safety. Ultimately, striking a balance between biodegradability and shelf life requires careful consideration of diverse factors, including consumer preferences, regulatory requirements, technological advancements, and environmental stewardship goals.361
The proliferation of plastic waste has sparked interest in biodegradable plastics as a sustainable alternative. Biodegradable plastics are derived from renewable resources like cornstarch or sugarcane. They are designed to break down more readily in the environment, reducing the persistence of plastic waste. By transitioning from conventional plastics to biodegradable alternatives, the packaging industry addresses the issue of plastic pollution. Biodegradable plastics can be composted alongside organic waste, minimizing the load on landfills and contributing to a circular economy.
3.10. Essential oil-based pickering emulsions for food packaging
Essential oils, characterized by their low molecular weight, high volatility, and lipophilic nature, constitute a fraction of materials with varying components depending on factors such as climate, growth stage, plant material, harvest, and health, among others. These components can undergo interconversion through dehydrogenation, oxidation, isomerization, and cyclization triggered chemically or enzymatically.362 Vegetable or edible oils primarily consist of triacylglycerides (96%), alongside antioxidants, free fatty acids, tocopherols, phospholipids, waxes, and phytosterols.363 Owing to their diverse bioactive composition, essential oils find extensive use in food systems. They contribute to the enhancement of food quality and the reduction of food shelf life resulting from microbial contamination and lipid oxidation processes. Utilizing essential oils from cinnamon, cloves, thyme, rosemary, and oregano has been effective in extending the shelf life of various food products, including vegetables, meat, and fish.364
However, the direct application of essential oils in food is restricted due to their intense flavor and high volatility. To expand their usage in food products, these limitations can be overcome through the development of Pickering Emulsions (PEs) based on various essential oils using different Pickering particles. Notably, PE formulations of marjoram oil, clove, tea tree oil, cinnamon, cardamom, oregano, peppermint oil, rosemary oil, and cedarwood are extensively discussed, as summarized in Table 5.373
Table 5 Essential oil-based Pickering emulsions in food packaging
Essential oil |
Pickering particle |
Fabrication method |
Applications |
Reference |
Cinnamon |
Zein–pectin composite nanoparticles |
High speed homogenization |
Antimicrobial agent in apple slices |
365
|
Cinnamon oil & corn oil |
Sodium starch octenyl succinate (SSOS) |
Homogenization |
Active biodegradable films with improved antimicrobial & antioxidant activities |
366
|
Marjoram |
Whey protein isolate (WPI)/inulin |
Ultrasonication |
Active pectin film as a new active packaging system |
367
|
Clove |
Zein colloidal particles |
High speed homogenization and ultrasonication |
Chitosan based edible film with antimicrobial properties |
368
|
Clove |
Cellulose nanofiber (CNF) |
High speed homogenization |
Gelatin/agar active film with improved antioxidant activity and their application as active food packaging |
369
|
Peppermint |
Chitosan decorated silica nanoparticles |
Homogenization |
PE based composite microcapsules using HPMC which is a promising strategy for antibacterial applications |
370
|
Oregano |
Zein–pectin nanoparticle |
High speed homogenization |
Strawberries preserved in konjac glucomannan active packaging films |
371
|
Oregano |
ZnO nanoparticle |
High-shear homogenization |
Cellulose nanofibril film with antioxidant & antimicrobial activity |
372
|
Fig. 41 illustrates the incorporation of gelatin/agar-based films with Pickering emulsion374 and SSOS-based Pickering emulsion films infused with cinnamon essential oil.375 Additionally, Fig. 42 represents the depiction of oregano essential oil Pickering emulsion stabilized by CNCs376 and the formation of peppermint oil-loaded composite microcapsule based on chitosan-decorated silica nanoparticle stabilized Pickering emulsion templating.370
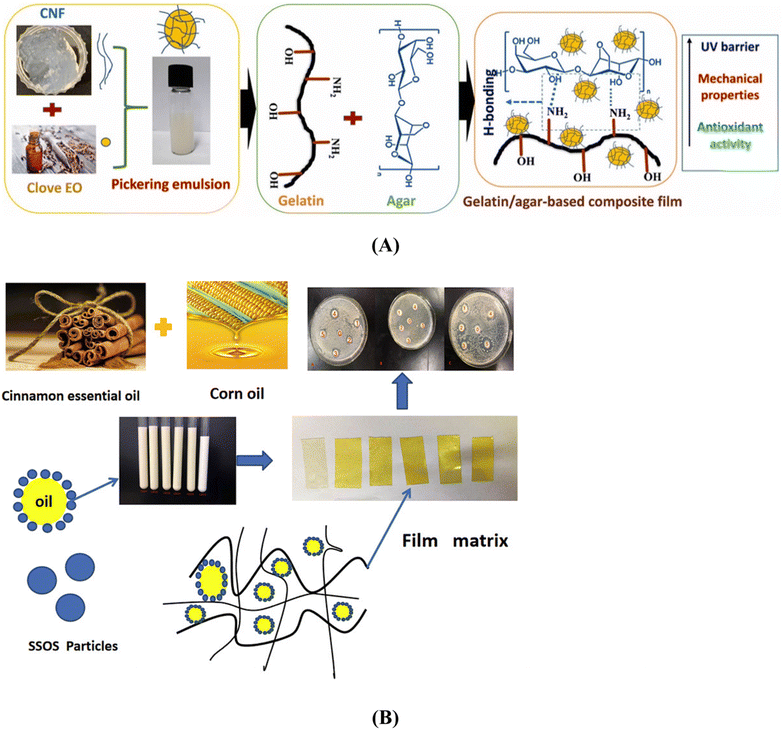 |
| Fig. 41 (A) Pickering emulsion added gelatin/agar-based films,374 reproduced (adapted) with permission from ref. 374. Copyright [2021] [Elsevier], & (B) SSOS-based Pickering emulsion films incorporated with cinnamon essential oil.375 Reproduced (adapted) with permission from ref. 375. Copyright [2020] [Elsevier]. | |
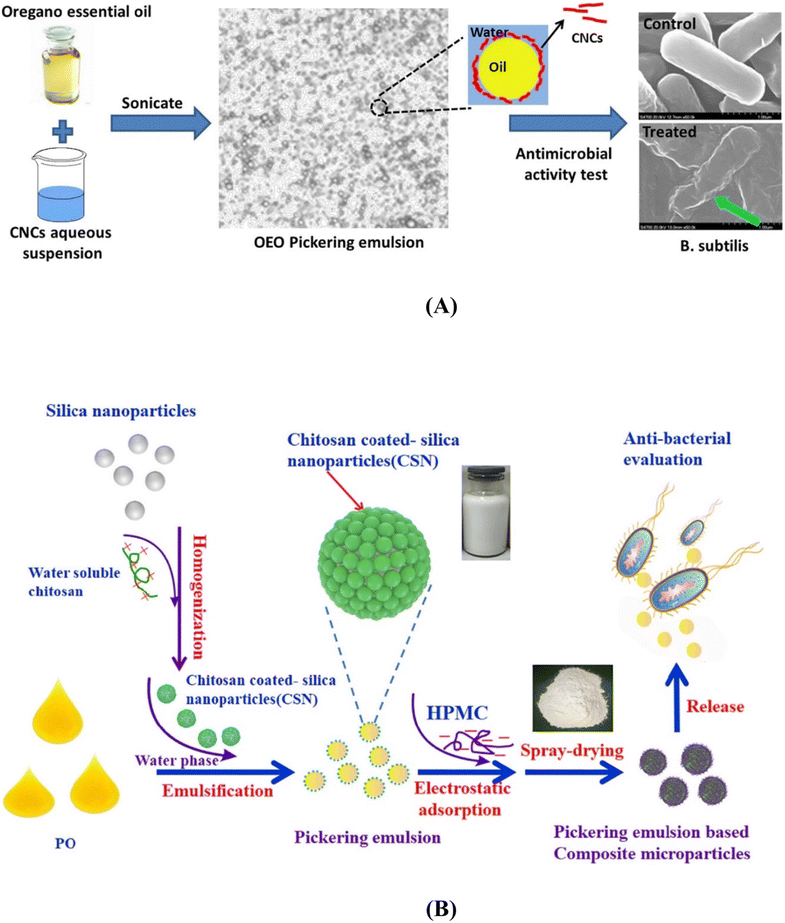 |
| Fig. 42 Schematic representation of (A) oregano essential oil Pickering emulsion stabilized by CNCs,376 reproduced (adapted) with permission from ref. 376. Copyright [2018] [Elsevier], & (B) peppermint oil-loaded composite microcapsule based on chitosan-decorated silica nanoparticle stabilized Pickering emulsion templating.370 Reproduced (adapted) with permission from ref. 370. Copyright [2021] [Elsevier]. | |
In nutshell, the non-toxic and biodegradable nature of essential oil based Pickering emulsions and their fabrication by using natural Pickering particles made them suitable to be used in biomedicine, food, cosmetics, etc. applications. Their applications in the food industry include as preservative, antimicrobial and antioxidant agent. Conventional packaging materials are non-biodegradable and for overcoming this issue, EO-loaded PE are incorporated into biodegradable packaging films for the generation of active and edible films. Such films are found to be used widely in food packaging for the improvement of shelf life of food and other similar applications some of them still need to be explored further.373,377
Pickering emulsions, stabilized by solid particles, offer a novel avenue for sustainable food packaging. Essential oil-based Pickering emulsions combine the antimicrobial properties of essential oils with stable emulsions, reducing the need for synthetic emulsifiers. These emulsions can be applied as coatings to packaging materials, providing antimicrobial protection to packaged foods. By incorporating essential oils into packaging, manufacturers can minimize the need for synthetic antimicrobial agents. This aligns with sustainability goals by utilizing natural compounds to enhance food safety and quality. Additionally, Pickering emulsions promote efficient resource utilization by providing a stable platform for essential oil delivery.
4. Greener fabrication processes, underlying toxicity, environmental ramifications and techno-economic assessments of sustainable food packaging materials
4.1. Greener processes in fabrication
Adopting greener processes in the fabrication of sustainable food packaging materials is a pivotal step towards reducing the environmental impact of packaging production. Traditional manufacturing methods often rely heavily on fossil fuels and energy-intensive processes, leading to significant greenhouse gas emissions and resource depletion. To address this, companies are increasingly turning to eco-friendly practices.
One key aspect is the integration of renewable energy sources into manufacturing facilities. Solar panels and wind turbines can provide a substantial portion of the energy required for production, drastically reducing reliance on non-renewable sources. Additionally, optimizing manufacturing processes for efficiency can significantly cut down on energy consumption. Techniques such as lean manufacturing and advanced automation minimize waste, energy use, and carbon emissions.
Circular economy principles also play a vital role in greener fabrication. Closed-loop systems that encourage the recycling and repurposing of materials within the production process help minimize waste generation. This approach reduces the need for virgin resources and lowers the overall environmental impact. Byproducts and waste streams from one process can be used as inputs for another, creating a more sustainable and interconnected production ecosystem.378
4.2. Underlying toxicity
The consideration of toxicity in sustainable food packaging materials is paramount to ensure both consumer safety and environmental well-being. Some conventional packaging materials contain additives or chemicals that can leach into food products, potentially causing health concerns for consumers. These substances can also pose a threat to the environment when they are released into ecosystems. To address this, manufacturers are increasingly adopting materials that have been rigorously tested and approved for food contact. Materials such as food-grade stainless steel, glass, and certain types of plastics undergo extensive assessments to ensure they do not release harmful substances. This not only protects consumers but also prevents contamination of the food supply chain. Furthermore, advancements in material science have led to the development of biodegradable and compostable packaging materials. These materials break down naturally over time, reducing the accumulation of non-biodegradable waste in landfills and oceans. However, it's important to conduct thorough testing to ensure that these materials break down safely without releasing harmful compounds.379
4.3. Environmental ramifications
Evaluating the environmental ramifications of packaging materials goes beyond their immediate impact and considers their lifecycle effects. Traditional packaging materials often contribute to carbon emissions, deforestation, and habitat destruction. Sustainable alternatives aim to mitigate these issues. One key consideration is the carbon footprint of packaging materials. Materials that require significant energy for extraction, production, and transportation contribute more to climate change. Sustainable options, such as plant-based bioplastics or recycled materials, often have a lower carbon footprint. Additionally, sustainable materials like bamboo and jute grow rapidly and require fewer resources compared to traditional materials like wood or cotton. Biodiversity is another critical concern. Unsustainable packaging materials, particularly those derived from resource-intensive processes, can contribute to habitat loss and disruption of ecosystems. Choosing materials that have minimal impact on biodiversity helps preserve fragile ecosystems and maintain ecological balance.380
4.4. Techno-economic assessments
Techno-economic assessments provide a comprehensive understanding of the financial and practical feasibility of sustainable food packaging materials. Businesses need to consider not only the initial costs of production but also the long-term benefits and potential risks. Incorporating the entire lifecycle of the packaging material is crucial. This includes evaluating the costs associated with raw material extraction, manufacturing, transportation, use, and end-of-life disposal. While some sustainable materials may have higher upfront costs, they could lead to significant savings over time due to reduced energy consumption, waste management, and potential regulatory compliance. Anticipating regulatory changes is also vital. As governments and organizations increasingly prioritize sustainability, regulations surrounding packaging materials may evolve. Materials that align with these changing regulations will be more future-proof and less likely to incur unforeseen compliance costs.381
In nutshell, each of these factors—greener fabrication processes, underlying toxicity, environmental ramifications, and techno-economic assessments—plays a pivotal role in shaping the landscape of sustainable food packaging materials. A holistic approach that considers these facets ensures that packaging choices are not only environmentally responsible but also economically viable and safe for consumers. As technology and awareness continue to advance, the potential for even more innovative and sustainable packaging solutions is promising.
5. Applications of chemical methods/chemistry in the improvement of packaging properties of materials
Chemical methods play a vital role in improving the properties of food packaging materials, enhancing their performance, and addressing specific requirements of the food industry. These methods aim to modify the chemical composition or structure of packaging materials to achieve desired characteristics. Here are some common chemical methods used for improving the properties of food packaging.
5.1. Crosslinking
Crosslinking involves chemically linking polymer chains to increase the strength, durability, and thermal stability of the packaging material. Crosslinked polymers exhibit enhanced mechanical properties, better resistance to chemical degradation, and reduced gas permeability. This method is commonly used for polyethylene (PE) and polypropylene (PP) materials in food packaging.
5.2. Polymer blending
By blending different polymers, food packaging materials can benefit from a combination of desirable properties. For example, blending polyethylene with ethylene-vinyl alcohol (EVOH) can improve the gas barrier properties of the packaging, making it suitable for oxygen-sensitive food products.
5.3. Coating and lamination
Chemical coatings or laminations are applied to the surface of food packaging materials to modify their properties. Coatings can improve barrier properties, enhance printability, and add heat sealability. Lamination involves bonding multiple layers to create a composite material with specific functionalities.
5.4. Barrier coatings
Barrier coatings are applied to packaging materials to enhance their resistance to moisture, gases, and aroma migration. These coatings may include nanocomposites, metal oxides, or silicones, depending on the specific requirements.
5.5. Antimicrobial coatings
To prevent microbial growth and food spoilage, antimicrobial coatings can be applied to food packaging materials. These coatings incorporate agents like silver nanoparticles or natural antimicrobial compounds to inhibit the growth of bacteria and fungi.
5.6. Active packaging
Active packaging involves incorporating specific additives or compounds into the packaging material that interact with the food product to extend its shelf life or improve quality. For example, oxygen scavengers can be added to absorb oxygen, preventing oxidative degradation of food.
5.7. Nanotechnology
Nanotechnology offers innovative approaches to modify food packaging materials at the nanoscale level. Nanocomposites, nanolayers, or nanoparticles can be incorporated into packaging materials to improve mechanical strength, gas barrier properties, and UV resistance.
5.8. Biodegradable additives
To enhance the biodegradability of traditional plastics, biodegradable additives can be incorporated during polymerization. These additives allow the packaging material to break down more efficiently in the environment after disposal.
5.9. UV stabilization
UV stabilizers can be added to packaging materials to protect them from degradation caused by exposure to ultraviolet (UV) radiation. This is especially important for packaging materials used for products intended for outdoor storage or display.
The selection of appropriate chemical methods depends on the specific requirements of the food product, the packaging application, and the desired properties. It is essential to consider food safety regulations and ensure that the chemical modifications do not introduce any harmful substances into the food. Proper testing and evaluation are necessary to ensure the performance and safety of chemically improved food packaging materials.382
6. Recent advances in sustainable food packaging materials
6.1. Hybrid nanoparticle-based biopolymer electrospin coating for intelligent food packaging and shelf-life improvement of chapattis
Pomegranate peel has a great potential to act as a valuable source of antioxidants and bioactive compounds. It highlights various studies that have explored its beneficial properties, including its antioxidant, anticarcinogenic, antibacterial, antifungal, and anti-inflammatory activities. Researchers have investigated the use of pomegranate peel extracts in food packaging to improve shelf life and inhibit microbial growth. Nanoparticles, when combined with biopolymers in packaging materials, can enhance their properties, although research in this area is limited. Consequently, a study was conducted to develop biodegradable food packaging using a mixture of TiO2, ZnO nanoparticles, and pomegranate peel powder. The coated packaging was evaluated for its ability to preserve cooked food, employing various chemical tests to assess shelf life, oxidation kinetics, and microbial activity. The results suggest that the active mixture significantly improves the shelf life of food items, indicating its potential for enhancing food packaging efficiency (Fig. 43).383
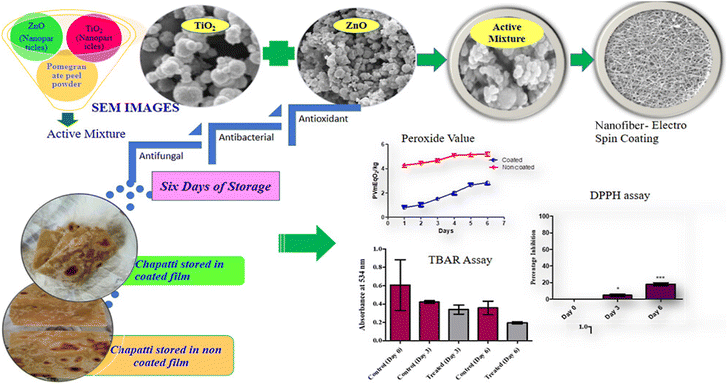 |
| Fig. 43 Schematic representation of hybrid nanoparticle-based biopolymer electrospin coating for intelligent food packaging and shelf-life improvement of chapattis.383 Reproduced (adapted) with permission from ref. 383. Copyright [2023] [ACS]. | |
6.2. Glycerol-added chitosan films for food packaging
Chitosan, derived from chitin found in crustaceans and fungi, is a versatile polymer with various applications due to its unique properties such as being nontoxic, biodegradable, and antimicrobial. It is utilized in biomedical products, wound dressings, cosmetics, water treatment, fertilizers, and animal husbandry. In the food industry, it serves as a packaging material or coating to enhance the shelf life of products. To improve the mechanical properties of chitosan films, plasticizers like glycerol are often added. Glycerol, in particular, significantly enhances the flexibility and strength of these films, which is crucial for maintaining product quality during storage and transportation. However, while the effects of plasticizers on tensile and tear strengths have been studied, little attention has been paid to their impact on burst strength. This study focuses on investigating the influence of glycerol as a plasticizer on the mechanical, chemical, morphological, optical, and thermal properties of chitosan films. The research includes characterizing the glass transition temperature, transparency, Young's modulus, elongation, tensile strength, and burst strength of the films. The findings demonstrate the significant role of glycerol in enhancing various properties of chitosan films, making them suitable for food packaging applications (Fig. 44).384
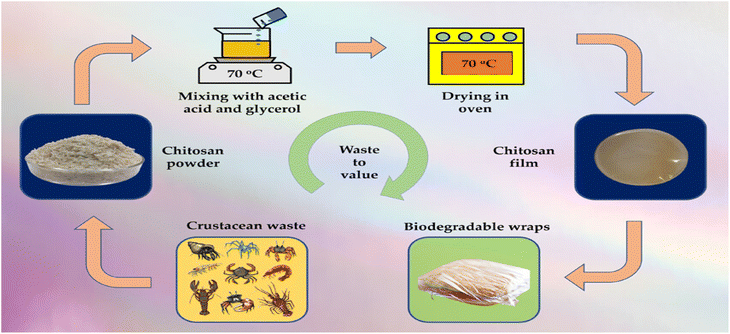 |
| Fig. 44 Schematic representation of synthesis and use of glycerol-added chitosan films for food packaging.384 Reproduced (adapted) with permission from ref. 384. Copyright [2023] [ACS]. | |
6.3. Allicin-loaded electrospun PVP/PVB nanofibrous films with superior water absorption and water stability for antimicrobial food packaging
Poly(vinyl pyrrolidone) (PVP) is widely used in food packaging due to its excellent spinnability and safety. However, its high hydrophilicity and water solubility limit its application in packaging high-moisture food items. Cross-linking is a common method to address this issue, but many cross-linking agents pose safety concerns. Genipin, a safer alternative, is expensive. To make PVP more suitable for food packaging, researchers explored blending it with the safe and cost-effective polymer PVB. In this study, PVP/PVB-allicin (PB64-A) nanofibrous films were produced via electrospinning, with allicin incorporated as an antibacterial agent. PVB, known for its hydrophobic nature and biocompatibility, was blended with PVP to enhance water stability. The antibacterial efficacy of PB64-A films against E. coli and S. aureus was assessed, and their potential as antimicrobial food packaging for chicken breast was evaluated. This approach offers a safe and economical method to improve the water stability of PVP nanofibrous films for food packaging applications (Fig. 45).385
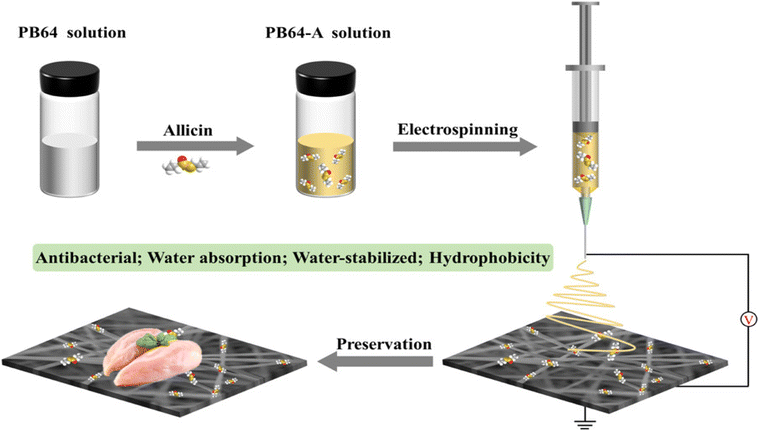 |
| Fig. 45 Schematic representation of synthesis and use of allicin-loaded electrospun PVP/PVB nanofibrous films for food packaging.385 Reproduced (adapted) with permission from ref. 385. Copyright [2022] [ACS]. | |
6.4. Poly(vinyl chloride) derived food packaging materials with antioxidative and anticancer properties
Autoxidation, a natural process involving unsaturated fatty acids and oxygen, leads to spoilage through the formation of reactive radicals and peroxides. Oxygen in the headspace of plastic bottles can initiate autoxidation, deteriorating cooking oils even before use. Adding UV radiation barrier compounds to packaging materials reduces the rate of autoxidation by limiting UV light penetration. Various natural compounds, such as tannic acid and catechin, have been identified as effective in reducing autoxidation. Vanillic acid, caffeic acid, coumaric acid, naringin, and cinnamic acid, among others, exhibit antioxidant and antimicrobial properties, contributing to health benefits and food preservation. In previous studies, novel derivatives of PVC and chlorinated polypropylene were synthesized using natural compounds for active food packaging applications. In this study, PVC derivatives functionalized with vanillic, caffeic, coumaric, and cinnamic acids, along with naringin, were synthesized and characterized. The inhibitory effects of these polymers on the autoxidation of linseed oil were investigated, highlighting their potential as antioxidants and anticarcinogens (Fig. 46).386
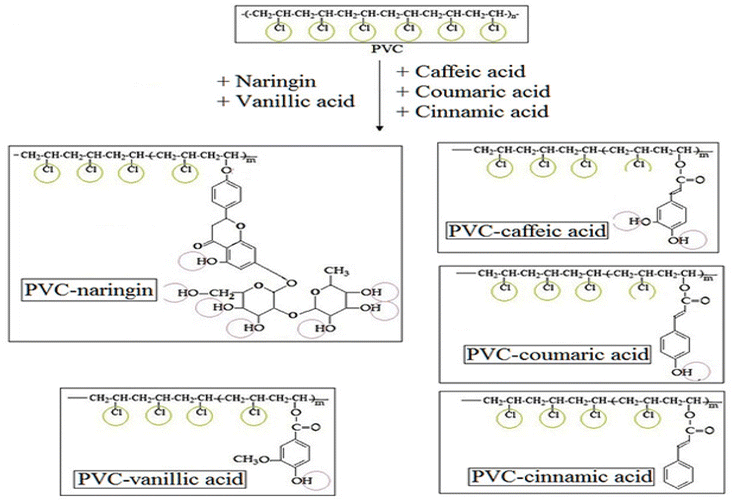 |
| Fig. 46 Schematic representation of poly(vinyl chloride) derived food packaging materials.386 Reproduced (adapted) with permission from ref. 386. Copyright [2023] [ACS]. | |
6.5. Multifunctional bionanocomposite films based on chitosan/polyvinyl alcohol with ZnO NPs and Carissa carandas extract anthocyanin for smart packaging materials
Polyvinyl alcohol (PVA) is a synthetic polymer known for its biodegradability, water solubility, and compatibility. It is widely used in food packaging due to its transparent, odorless, and adhesive nature. Blending PVA with chitosan (CS) forms composite films with enhanced mechanical strength and barrier properties against oxygen and water, making them ideal for food packaging applications. Anthocyanins, natural pigments found in plants and fruits, are utilized as pH indicators in intelligent food packaging systems due to their color-changing ability at different pH levels. Incorporating anthocyanins into polymer matrices extends food shelf life and enhances packaging. To improve antibacterial, mechanical, and barrier properties of these films, nanoparticles like nano-SiO2, gold, silver, and zinc oxide (ZnO) are added. Among these, nano-ZnO is preferred for its cost-effectiveness, antibacterial properties, and safety in food packaging. In this study, composite films of CS/PVA blended with natural extracts and ZnO nanoparticles were developed and characterized for their physicochemical properties. The incorporation of both natural extract and ZnO nanoparticles improved the properties of the films, making them suitable for various applications including food packaging. The study explored the effects of ZnO nanoparticles and anthocyanin composition on the properties of CS/PVA films, including their morphology, mechanical strength, pH sensitivity, cell viability, and antioxidant properties. The films demonstrated potential as smart packaging materials, particularly in maintaining the freshness of fish fillets (Fig. 47).387
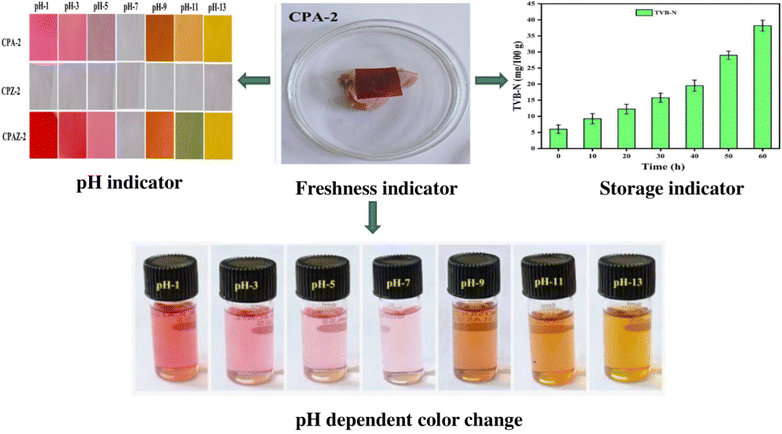 |
| Fig. 47 Schematic representation of the use of bionanocomposite films based on chitosan/polyvinyl alcohol with ZnO NPs and Carissa carandas extract anthocyanin for smart packaging materials.387 Reproduced (adapted) with permission from ref. 387. Copyright [2023] [ACS]. | |
6.6. Sodium alginate–Aloe vera hydrogel films enriched with organic fibers
Biopolymers sourced from renewable materials, such as algae, are valuable for hydrogel production. Algal polysaccharides, notably alginate from brown seaweed, are recognized for their biodegradability and compatibility. However, pure alginate films lack mechanical strength and functional properties necessary for food packaging. Blending alginate with other polymers like Aloe vera, gum tragacanth (GT), and hydroxypropyl methylcellulose (HPMC) enhances their properties. Aloe vera, rich in water and active compounds, offers therapeutic and functional benefits. Gum tragacanth, extracted from Astragalus plants, is an anionic polysaccharide used in the food industry as a thickener and emulsifier. Hydroxypropyl methylcellulose, a biodegradable polymer, forms transparent, flexible, and oil-resistant films suitable for food packaging. This study builds upon previous research on alginate–Aloe vera composite films for food packaging. The optimal concentrations of alginate and Aloe vera were determined in earlier studies. Here, gum tragacanth and HPMC were added to reinforce these films. The study aims to compare the reinforcing effects of gum tragacanth and HPMC and their impact on the physicochemical and functional properties of alginate–Aloe vera films (Fig. 48).388
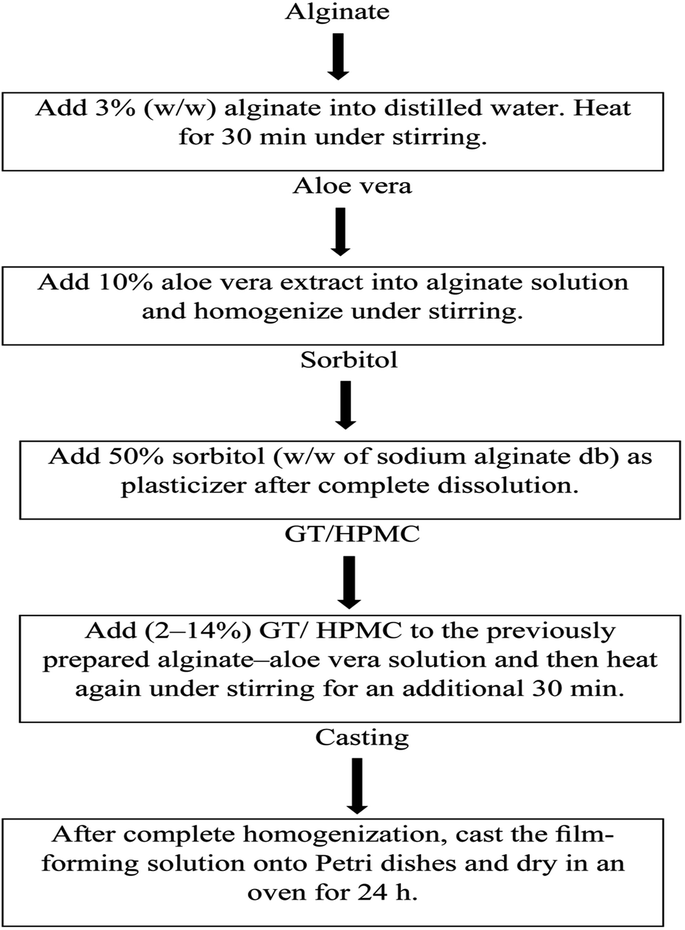 |
| Fig. 48 Flow diagram showing the preparation of the alginate–Aloe vera films.388 | |
6.7.
In situ crosslinked Schiff base biohydrogels containing Carica papaya peel extract: application in the packaging of fresh berries
Papaya (Carica papaya L.), a widely cultivated tropical fruit, is rich in bioactive compounds beneficial for human health. The significant amount of waste generated from papaya harvesting, mainly in the form of peels, has prompted researchers to explore its potential as a valuable resource. Papaya peels (PPs) have traditionally been used in various applications such as cattle feed and nutraceutical supplements. However, recent efforts have focused on valorizing PPs to develop value-added products, including biofuels, dietary fibers, biomaterials, and corrosion inhibitors. Starch and chitosan, important biopolymers in the food processing industry, suffer from poor mechanical properties when used individually. To address this limitation, researchers have combined these biopolymers to create hydrogels with enhanced properties. Typically, hydrogel synthesis involves chemical crosslinking reagents, but there is a growing interest in green chemistry approaches. In this study, in situ crosslinked Schiff base hydrogels were prepared without external crosslinkers, utilizing periodate oxidation to functionalize starch and create aldehyde groups for crosslinking with chitosan. The synthesized Schiff base hydrogels of starch and chitosan, designated as CsDAS hydrogel, were explored as potential food packaging materials. The incorporation of C. papaya peel extract into the hydrogels aimed to develop an active food packaging system. The study evaluated the hydrogel properties and their effectiveness in extending the shelf life of berries. This research contributes to the development of sustainable and functional food packaging materials derived from renewable resources (Fig. 49).389
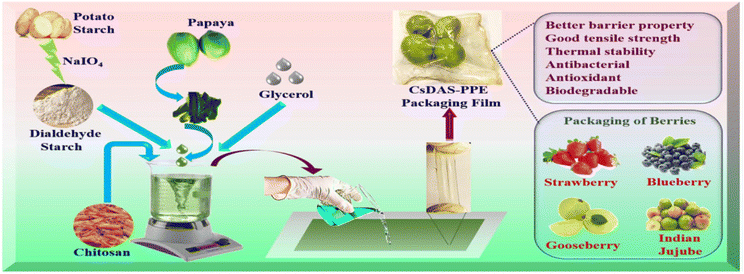 |
| Fig. 49 Diagrammatic depiction of the fabrication of chitosan (Cs)–dialdehyde starch (DAS) Schiff base hydrogels containing C. papaya peel extract (CsDAS–PPE hydrogel) as novel packaging materials for fresh berries.389 | |
6.8. Gallic acid functionalized chitosan/pullulan active bio-films for the preservation and shelf-life extension of green chillies
Researchers have been exploring the development of food packaging systems by incorporating natural polyphenols such as citric acid, dextran, ascorbic acid, and gallic acid (GA) to enhance the multifunctional properties of the films. Additionally, the use of additives like green deep eutectic solvents (DES) in chitosan (CS) based films has shown improvements in solubility, tensile stability, and flexibility. Incorporating GA into CS films has been particularly effective in enhancing antimicrobial and antioxidant properties, as well as improving tensile strength and elongation at break, especially when formulated with gelatin. Furthermore, GA-grafted CS films have shown enhanced water vapor barrier and thermal stability. Studies have demonstrated the extension of the shelf-life of mushrooms by up to 24 days when packed with dextran-integrated CS film. These findings highlight the significant impact of using green components as additives on the functional properties of polymeric film matrices. The present study aims to develop novel CS/PL (polylactic acid) based active bio-films functionalized with different percentages of GA. The physico-mechanical and functional properties of these composite films were investigated and compared with those of pristine CS and CS/PL films. Notably, there have been no previous studies utilizing CS/PL film matrices with GA. Additionally, the research aims to evaluate the practical packaging efficiency of the developed film in preserving and extending the shelf-life of green chillies at room temperature (Fig. 50).390
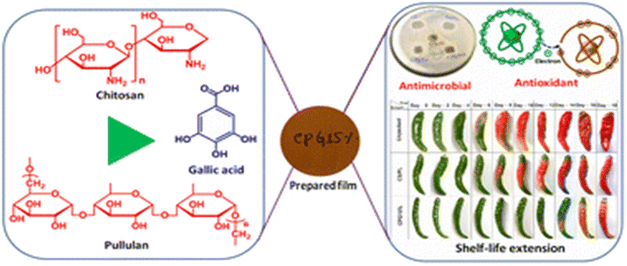 |
| Fig. 50 Gallic acid functionalized chitosan/pullulan active bio-films for the preservation and shelf-life extension of green chillies.390 | |
6.9. Humidity-adjustable functional gelatin hydrogel/ethyl cellulose bilayer films
In recent years, protein films have emerged as promising materials for food packaging due to their favorable mechanical properties, cost-effectiveness, stability, renewability, and abundance. Gelatin (GEL), derived from animal processing waste, is widely used in the food industry for its excellent film-forming behavior and water-holding capacity. However, pure GEL films suffer from poor mechanical properties, thermal stability, and high water solubility, limiting their application in food packaging. Strategies such as blending with polysaccharides, essential oils, or nanofillers have been employed to overcome these drawbacks. Yet, composite films lack the ability to regulate humidity within packaging systems effectively. To address this issue, a bilayer film approach is proposed, comprising a hydrophilic internal layer and a hydrophobic external layer. Hydrogel, with its ability to absorb and retain water, serves as the internal layer to regulate humidity, while ethyl cellulose (EC) is utilized as the external layer for moisture barrier properties. Tannic acid (TA) is explored as a cross-linker for GEL hydrogel due to its cost-effectiveness and non-toxic nature. The incorporation of metallic nanoparticles, such as silver nanoparticles (AgNPs), into the film matrix provides antibacterial activity. In situ synthesis and immobilization of AgNPs within the GEL hydrogel network are proposed to minimize toxicity and aggregation issues. In this study, a bilayer film combining TA cross-linked GEL hydrogel as the inner layer and EC as the outer layer was developed. AgNPs were synthesized in situ and immobilized within the GEL hydrogel network to introduce antibacterial activity. The physicochemical properties, migration in food simulants, and biodegradability of the bilayer film were investigated. Additionally, the potential application of the developed bilayer film for mushrooms preservation packaging was evaluated. This research addresses the need for multifunctional food packaging materials with internal humidity regulation and antibacterial activity, which is crucial for ensuring food safety and extending shelf-life (Fig. 51).391
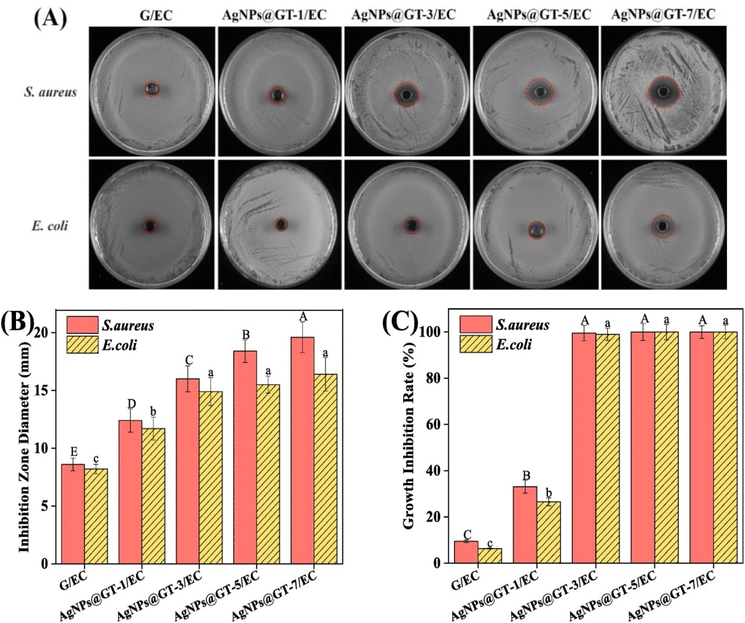 |
| Fig. 51 Antibacterial activities of different film samples against S. aureus and E. coli: (A) antibacterial photographs, (B) inhibition zone diameter and (C) growth inhibition rate.391 Reproduced (adapted) with permission from ref. 391. Copyright [2024] [Elsevier]. | |
6.10. Covalent organic framework-based nanofibrous films with temperature-responsive release of thymol for active food packaging
Nanofibers are pivotal in active food packaging, with a focus on achieving rapid preparation methods for large-scale applications. Solution blow molding (SBS) has emerged as a promising technique, utilizing high-speed airflow for nanofiber production, with applications in various fields. Compared to electrospinning, SBS offers advantages such as high yield, shorter fabrication time, safety, and suitability for large-scale production. Poly(ε-caprolactone) (PCL) is commonly used for biodegradable packaging nanofibers due to its excellent mechanical properties, biocompatibility, and loading capacity. This study aimed to develop temperature-responsive active food packaging using SBS. Covalent organic frameworks (COFs) were synthesized via asymmetric monomer exchange (AME) for encapsulating a compound (THY), and then loaded into PCL nanofibrous films for controlled release of THY. The morphology, crystal structure, and physical properties of COFs and nanofibrous films were characterized. The release profiles of THY at different temperatures were investigated, along with the biocompatibility and antibacterial activities of the nanofibrous films (Fig. 52).392
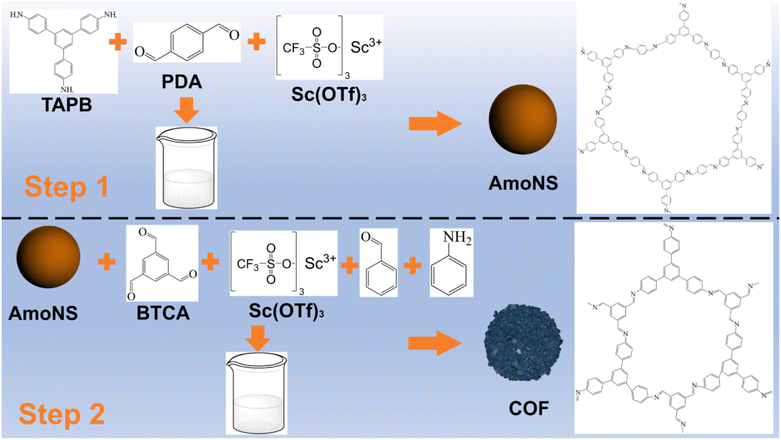 |
| Fig. 52 The synthesis mold of AmoNS and COFs.392 Reproduced (adapted) with permission from ref. 392. Copyright [2023] [Elsevier]. | |
6.11. Condensed tannins, a viable solution to meet the need for sustainable and effective multifunctionality in food packaging
Condensed tannins (CT) are prevalent natural phenolic polymers, ranking second only to lignin in abundance across the plant kingdom. They are widely distributed, with the highest concentrations found in the bark and heartwood of various tree species like mimosa, quebracho, and oak. Additionally, CTs are present in nuts, fruits, seeds, leaves, twigs, and stems of certain leguminous plants. Berries, persimmons, bananas, apples, cocoa, and grape seeds are notable sources of CT. In recent years, CTs have garnered attention as multifunctional additives in the food packaging sector. Their biocompatibility and antimicrobial properties make them effective against food spoilage, while their unique chemical properties offer advantages for functional packaging design. CTs can reinforce packaging polymer matrices, enhancing mechanical properties, UV and thermal stability, and gas permeability. Moreover, they can act as functional additives to prolong food shelf life by delaying deterioration processes. CTs' antioxidant properties stabilize materials commonly used in food packaging, such as polyolefins and polylactic acid, against thermal and photo-induced oxidation. Their ability to interact with polymer matrices improves mechanical properties and barrier properties crucial for food freshness and protection from oxidative deterioration. CTs also exhibit antimicrobial and antifungal activities against foodborne pathogens, enhancing the oxidative stability of lipid-rich foods during storage. They can prevent enzymatic browning in fruit smoothies by inhibiting polyphenol oxidases' activity and improve frying oil quality by removing toxic carbonyl species. Incorporation of CT into food packaging materials is typically achieved through techniques like extrusion, solvent casting, or vacuum filtration, without requiring chemical linkage to the polymer. Characterization methods such as microscopy, spectroscopy, and mechanical tests assess CT's effects on the packaging material's properties and performance. Several innovative applications of CT in food packaging have emerged, such as PLA films incorporating CT from pecan nut shells, whey protein-based edible films with antimicrobial properties, and chitosan-based composite films with enhanced antioxidant activity and barrier properties. Overall, CT-functionalized materials hold significant potential for improving food packaging's safety, quality, and sustainability, with various patents reflecting ongoing research and development efforts in this area.393Fig. 53 represents some important food packaging applications of CT.
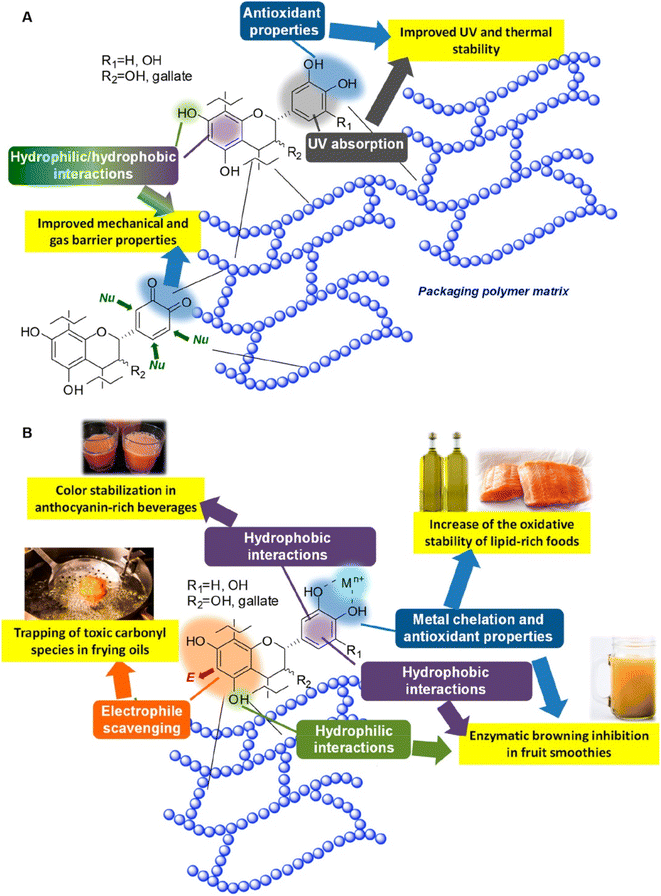 |
| Fig. 53 Exploitation of CT chemical properties in food packaging: (A) role of CT for reinforcement of the packaging polymer matrix and (B) role of CT as functional additives able to delay the onset of deterioration processes and prolong the shelf life of food.393 | |
6.12. Photothermal controlled antibacterial Ta4C3Tx–AgNPs/nanocellulose bioplastic food packaging
Currently, various antibacterial agents, including antibiotics, biological extracts, natural polymers, and metal nanoparticles, are utilized. However, the misuse of antibiotics has led to bacterial resistance, and biological extracts and natural polymers often lack strong antibacterial properties. Consequently, metal nanoparticles, particularly silver nanoparticles (AgNPs), have emerged as preferred antimicrobial agents due to their high efficiency, broad spectrum, long-term efficacy, stability, and low toxicity. Despite AgNPs' effectiveness, direct incorporation into biomass packaging materials can lead to excessive release, posing potential health and environmental risks, while also failing to provide long-lasting antibacterial effects. To address these challenges, researchers have turned to two-dimensional (2D) nanomaterials, such as MXene, for immobilizing AgNPs. MXene's large surface area makes it an ideal template for immobilizing AgNPs, preventing agglomeration and cumulative toxicity. Moreover, tantalum carbide (Ta4C3Tx) MXene exhibits excellent photothermal conversion properties, enabling controlled AgNPs release under near-infrared light. However, the binding between antibacterial agents and cellulose in composite packaging may be weak, affecting packaging performance. Quaternized chitosan (QCS), a derivative of chitosan, offers a potential solution due to its green reducing properties and structural similarity to cellulose. By serving as a reducing agent for in situ AgNPs preparation and a binder between MXene and nanocellulose, QCS facilitates the integration of antimicrobials into the packaging matrix. In this study, single-layer Ta4C3Tx nanosheets were prepared from Ta4AlC3 and combined with AgNPs to form Ta4C3Tx@AgNPs. This antimicrobial agent was then compounded with nanocellulose to produce nanocellulose-based bioplastic packaging (CTa–Ag) with enhanced mechanical and barrier properties. Additionally, CTa–Ag exhibited photothermal conversion capabilities, enabling controlled release of antibacterial agents while reducing cumulative toxicity.394Fig. 54 represents fabrication of CTa–Ag packaging.
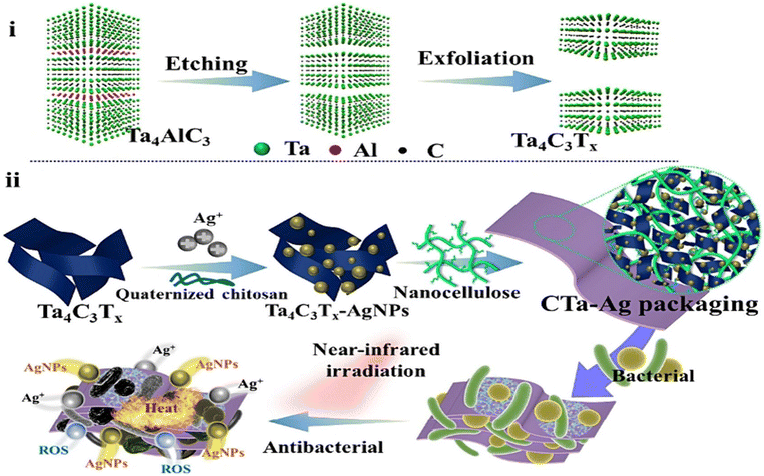 |
| Fig. 54 Schematic illustration of (i) Ta4C3Tx preparation from Ta4AlC3, and (ii) fabrication of CTa–Ag packaging.394 Reproduced (adapted) with permission from ref. 394. Copyright [2024] [Elsevier]. | |
6.13. Novel pectin-based nanocomposite film for active food packaging applications
Synthetic plastics, while commonly used for food packaging due to their affordability and performance, pose environmental and health concerns. Consequently, there's a growing demand for eco-friendly alternatives like biodegradable biopolymers such as gluten, gelatin, chitosan, starch, and alginate. Pectin, derived from plant cell walls, is a popular choice for packaging films due to its biodegradability, gelation properties, and non-toxic nature. However, its moderate barrier properties and high hydrophilicity can limit its use. To enhance its functionality, pectin films can be blended with other polymers or supplemented with nanosized fillers to improve their physicochemical, barrier, thermal, and mechanical properties. Crystalline nanocellulose (CNC), derived from lignocellulosic and cellulosic waste materials, is one such filler known for its high surface area, mechanical strength, and availability. Zinc oxide nanoparticles (ZnO NPs) offer antimicrobial and UV-blocking properties, approved for use in food packaging. The combination of ZnO NPs and CNC to reinforce pectin-based films, however, has not been extensively studied. In this study, active ZnO NPs/CNC/pectin-based films were prepared and characterized for their physicochemical, morphological, thermal, barrier, and mechanical properties. Antimicrobial activity was assessed both in vitro and in cheese samples stored at low temperatures. Additionally, cytotoxicity and migration of Zn2+ from the films to cheese were evaluated to ensure safety and suitability for food packaging applications.395Fig. 55 represents ZnO/CNC composite and pectin-based nanocomposite films preparation.
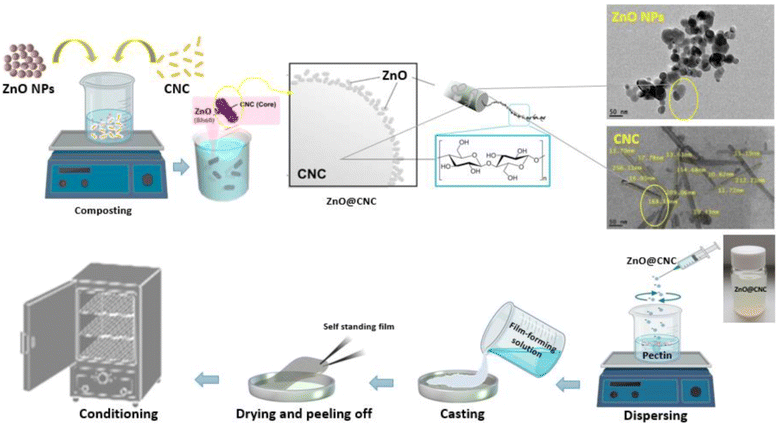 |
| Fig. 55 Schematic diagram for the preparation of ZnO/CNC composite and pectin-based nanocomposite films.395 | |
7. Concluding remarks and outlook
In conclusion, the use of sustainable food packaging materials has become increasingly important in recent years due to the negative impact of traditional packaging on the environment. The need for sustainable packaging is driven by concerns about plastic pollution, greenhouse gas emissions, and the depletion of natural resources. Sustainable food packaging materials can be made from a variety of sources, such as biodegradable plastics, plant-based materials, and recycled materials. These materials offer benefits such as reduced waste, decreased carbon footprint, and the ability to be recycled or composted. While there is no single solution to the problem of unsustainable food packaging, the adoption of sustainable packaging practices can help mitigate the environmental impact of packaging. It is essential to continue researching and investing in sustainable food packaging solutions to create a more sustainable future for the food industry and the planet. The future perspectives of sustainable food packaging materials are promising, with a growing interest in developing and adopting innovative solutions that are environmentally friendly, economically viable, and socially responsible. One of the main drivers of sustainable food packaging materials is the need to reduce waste and increase recycling. New materials and technologies are being developed to enable more efficient recycling and composting, such as biodegradable and compostable plastics, and plant-based materials. Another promising trend is the use of renewable and biodegradable materials, such as cellulose, starch, and lignin, which can be sourced from agricultural waste or other by-products, reducing the reliance on fossil fuels and virgin materials. Furthermore, the use of reusable and refillable packaging is gaining popularity, particularly in the food service industry, where it can significantly reduce waste and costs. The future also holds potential for advanced materials that are smart and interactive, such as sensors that can detect food spoilage, extend shelf life, or improve freshness, while reducing food waste and promoting food safety. Overall, the future perspectives of sustainable food packaging materials are exciting, and the continued research, development, and adoption of innovative solutions will play a critical role in creating a more sustainable and circular economy for the food industry.
The food packaging industry is constantly evolving to meet the changing demands of consumers, regulators, and the environment. Some future research opportunities in this field are:
Sustainable packaging materials: the use of eco-friendly packaging materials such as biodegradable plastics, paper, and other sustainable alternatives is increasing. Further research can focus on the development of new materials that are more environmentally friendly, cost-effective, and have improved barrier properties.
Smart packaging: smart packaging can help to improve food safety, enhance shelf-life, and provide better consumer information. Further research can focus on the development of new technologies that can help to reduce food waste, improve food quality, and enhance the consumer experience.
Nanotechnology: the use of nanotechnology in food packaging can provide improved barrier properties, better control of moisture and oxygen levels, and improved antimicrobial properties. Further research can focus on the development of new nanomaterials that can help to reduce food waste, improve food quality, and enhance the safety of packaged foods.
Food contact materials: research can focus on the development of new materials that can improve the safety and quality of food contact materials. This can include the development of new coatings, adhesives, and sealants that are more durable, resistant to degradation, and have improved barrier properties.
Packaging design: packaging design can play a crucial role in improving the functionality, usability, and aesthetics of food packaging. Further research can focus on the development of new packaging designs that can improve the convenience, portability, and sustainability of food packaging.
Certainly! While the outlined research directions are promising, it's essential to ensure a balanced approach that addresses not just technological advancements but also societal needs and ethical considerations. One direction to consider could be:
Social and cultural implications of packaging innovation: understanding how consumers perceive and interact with new packaging materials and technologies is crucial for successful adoption. Research in this area could explore consumer attitudes, preferences, and behaviors towards sustainable packaging, smart packaging features, and nanotechnology applications. This could involve interdisciplinary approaches integrating psychology, sociology, and marketing to ensure that packaging innovations align with consumer values and expectations while also promoting sustainability and safety.
By considering the social and cultural dimensions alongside technological advancements, researchers can ensure that future developments in the food packaging industry are not only effective but also socially responsible and widely accepted by consumers. This approach can help bridge the gap between innovation and consumer adoption, ultimately leading to more impactful and sustainable solutions in the field of food packaging.
Data availability
Data will be made available on reasonable request.
Conflicts of interest
There are no conflicts to declare.
Acknowledgements
SH is highly thankful for the fellowship under CSIR-JRF Scheme [File No.: 09/0984(15809)2022-EMR-I]. RA is highly thankful to Ministry of Education (MoE), for financial assistance through Institute fellowship. SSM is grateful to the Director, National Institute of Technology, Srinagar, J&K for basic infrastructural and laboratory facilities.
References
- C. Vasile and M. Baican, Progresses in Food Packaging, Food Quality, and Safety—Controlled-Release Antioxidant and/or Antimicrobial Packaging, Molecules, 2021, 26, 1263 CrossRef CAS PubMed.
- K. R. Dörnyei, I. Uysal-Unalan, V. Krauter, R. Weinrich, L. Incarnato, I. Karlovits, G. Colelli, P. Chrysochou, M. C. Fenech, M. K. Pettersen, E. Arranz, B. Marcos, V. Frigerio, A. Apicella, S. Yildirim, F. Poças, M. Dekker, L. Johanna, V. Coma and M. Corredig, Sustainable food packaging: An updated definition following a holistic approach, Front. Sustainable Food Syst., 2023, 7, 1119052, DOI:10.3389/fsufs.2023.1119052.
- D. Enescu, M. A. Cerqueira, P. Fucinos and L. M. Pastrana, Recent advances and challenges on applications of nanotechnology in food packaging. A literature review, Food Chem. Toxicol., 2019, 134, 110814 CrossRef CAS PubMed.
- R. Porta, The Plastics Sunset and the Bio-Plastics Sunrise, Coatings, 2019, 9, 526 CrossRef CAS.
- M. Li, N. Jia, M. Lenzen, A. Malik, L. Wei, Y. Jin and D. Raubenheimer, Global food-miles account for nearly 20% of total food-systems emissions, Nat. Food, 2022, 3(6), 445–453, DOI:10.1038/s43016-022-00531-w.
- K. Chang, M. J. Gunter, F. Rauber, R. B. Levy, I. Huybrechts, N. Kliemann, C. Millett and E. P. Vamos, Ultra-processed food consumption, cancer risk and cancer mortality: a large-scale prospective analysis within the UK Biobank, eClinicalMedicine, 2023, 56, 101840, DOI:10.1016/j.eclinm.2023.101840.
- T. Fiolet, B. Srour, L. Sellem, E. Kesse-Guyot, B. Allès, C. Méjean, M. Deschasaux, P. Fassier, P. Latino-Martel, M. Beslay, S. Hercberg, C. Lavalette, C. A. Monteiro, C. Julia and M. Touvier, Consumption of ultra-processed foods and cancer risk: results from NutriNet-Santé prospective cohort, BMJ, 2018, 360, k322, DOI:10.1136/bmj.k322.
- C. J. A. Bradshaw, P. R. Ehrlich, A. Beattie, G. Ceballos, E. Crist, J. Diamond, R. Dirzo, A. H. Ehrlich, J. Harte, M. E. Harte, G. Pyke, P. H. Raven, W. J. Ripple, F. Saltré, C. Turnbull, M. Wackernagel and D. T. Blumstein, Underestimating the Challenges of Avoiding a Ghastly Future, Front. Conserv. Sci., 2021, 1, 615419, DOI:10.3389/fcosc.2020.615419.
- T. Wiedmann, M. Lenzen, L. T. Keyßer and J. K. Steinberger, Scientists' warning on affluence, Nat. Commun., 2020, 11(1), 3107, DOI:10.1038/s41467-020-16941-y.
- P. R. Salgado, L. Di Giorgio, Y. S. Musso and A. N. Mauri, Recent Developments in Smart Food Packaging Focused on Biobased and Biodegradable Polymers, Front. Sustainable Food Syst., 2021, 5, 630393, DOI:10.3389/fsufs.2021.630393.
- R. Akhter, S. Hussain and S. S. Maktedar, Advanced graphene-based (photo & electro) catalysts for sustainable & clean energy technologies, New J. Chem., 2024, 48, 437–505 RSC.
- R. Akhter and S. S. Maktedar, MXenes: A comprehensive review of synthesis, properties, and progress in supercapacitor applications, J. Materiomics, 2023, 9, 1196–1241 CrossRef.
- K. Marsh and B. Bugusu, Food Packaging?Roles, Materials, and Environmental Issues, J. Food Sci., 2007, 72, R39–R55 CrossRef CAS PubMed.
- I. D. Ibrahim, Y. Hamam, E. R. Sadiku, J. M. Ndambuki, W. K. Kupolati, T. Jamiru, A. A. Eze and J. Snyman, Need for Sustainable Packaging: An Overview, Polymers, 2022, 14, 4430 CrossRef CAS PubMed.
- J.-W. Han, L. Ruiz-Garcia, J.-P. Qian and X.-T. Yang, Food Packaging: A Comprehensive Review and Future Trends, Compr. Rev. Food Sci. Food Saf., 2018, 17, 860–877 CrossRef PubMed.
- B. Kuswandi, Environmental friendly food nano-packaging, Environ. Chem. Lett., 2017, 15, 205–221 CrossRef CAS.
-
E. Pongrácz, The environmental impacts of packaging, Environmentally Conscious Materials and Chemicals Processing, 2007, pp. 237–278 Search PubMed.
-
Towards Safe and Sustainable Food Packaging, Becu. Bureau Européen Des Unions De Consommateurs Aisbl, 2021, May 27, Retrieved March 18, 2024, from https://www.beuc.eu/sites/default/files/publications/beuc-x-2021-050_towards_safe_and_sustainable_fcm._report.pdf Search PubMed.
- V. Guillard, S. Gaucel, C. Fornaciari, H. Angellier-Coussy, P. Buche and N. Gontard, The Next Generation of Sustainable Food Packaging to Preserve Our Environment in a Circular Economy Context, Front. Nutr., 2018, 5, 121, DOI:10.3389/fnut.2018.00121.
- F. Versino, F. Ortega, Y. Monroy, S. Rivero, O. V. López and M. A. García, Sustainable and Bio-Based Food Packaging: A Review on Past and Current Design Innovations, Foods, 2023, 12, 1057 CrossRef CAS PubMed.
- A. Ali and S. Ahmed, Recent Advances in Edible Polymer Based Hydrogels as a Sustainable Alternative to Conventional Polymers, J. Agric. Food Chem., 2018, 66, 6940–6967 CrossRef CAS PubMed.
- M. Z. Al-Gharrawi, J. Wang and D. W. Bousfield, Improving water vapor barrier of cellulose based food packaging using double layer coatings and cellulose nanofibers, Food Packag. Shelf Life, 2022, 33, 100895 CrossRef CAS.
- I. D. Ibrahim, Y. Hamam, E. R. Sadiku, J. M. Ndambuki, W. K. Kupolati, T. Jamiru, A. A. Eze and J. Snyman, Need for Sustainable Packaging: An Overview, Polymers, 2022, 14, 4430 CrossRef CAS PubMed.
- B. E. Teixeira-Costa and C. T. Andrade, Natural Polymers Used in Edible Food Packaging—History, Function and Application Trends as a Sustainable Alternative to Synthetic Plastic, Polysaccharides, 2021, 3, 32–58 CrossRef.
- J. Nilsen-Nygaard, E. N. Fernández, T. Radusin, B. T. Rotabakk, J. Sarfraz, N. Sharmin, M. Sivertsvik, I. Sone and M. K. Pettersen, Current status of biobased and biodegradable food packaging materials: Impact on food quality and effect of innovative processing technologies, Compr. Rev. Food Sci. Food Saf., 2021, 20, 1333–1380 CrossRef PubMed.
-
K. Outi, Catabolism of Biomass-Derived Sugars in Fungi and Metabolic Engineering as a Tool for Organic Acid Production, VTT Technical Research Centre of Finland, 2013, 978-951-38-8100-9 Search PubMed.
- A. M. Abdelgawad, M. E. El-Naggar, S. M. Hudson and O. J. Rojas, Fabrication and characterization of bactericidal thiol-chitosan and chitosan iodoacetamide nanofibres, Int. J. Biol. Macromol., 2017, 94, 96–105 CrossRef CAS PubMed.
-
T. Koutchma, Advanced Technologies for Meat Processing, ed. L. M. L. Nollet, and F. Toldra, Taylor & Francis, CRC Press LLC, Boca Raton, FL, 2006, p. 483, $159.95, Hardback, ISBN:10:1574445871Food Microbiol., 2007, 24, 801– Search PubMed.
- A. M. Youssef and S. M. El-Sayed, Bionanocomposites materials for food packaging applications: Concepts and future outlook, Carbohydr. Polym., 2018, 193, 19–27 CrossRef CAS PubMed.
-
S. Ahmadzadeh and A. M. Khaneghah, Role of Green Polymers in Food Packaging, Encyclopedia of Renewable and Sustainable Materials, 2020, pp. 305–319 Search PubMed.
- S. P. Mishra, A.-S. Manent, B. Chabot and C. Daneault, Production of nanocellulose from native cellulose – Various options utilizing ultrasound, BioResources, 2011, 7, 422–436 Search PubMed.
- K. Khwaldia, E. Arab-Tehrany and S. Desobry, Biopolymer Coatings on Paper Packaging Materials, Compr. Rev. Food Sci. Food Saf., 2010, 9, 82–91 CrossRef CAS PubMed.
- M. Asgher, S. A. Qamar, M. Bilal and H. M. N. Iqbal, Bio-based active food packaging materials: Sustainable alternative
to conventional petrochemical-based packaging materials, Food Res. Int., 2020, 137, 109625 CrossRef CAS PubMed.
- J. W. Roy Chong, X. Tan, K. S. Khoo, H. S. Ng, W. Jonglertjunya, G. Y. Yew and P. L. Show, Microalgae-based bioplastics: Future solution towards mitigation of plastic wastes, Environ. Res., 2022, 206, 112620 CrossRef CAS PubMed.
- L. Zimmermann, A. Dombrowski, C. Völker and M. Wagner, Are bioplastics and plant-based materials safer than conventional plastics? In vitro toxicity and chemical composition, Environ. Int., 2020, 145, 106066, DOI:10.1016/j.envint.2020.106066.
- A. C. Mendes and G. A. Pedersen, Perspectives on sustainable food packaging:– is bio-based plastics a solution?, Trends Food Sci. Technol., 2021, 112, 839–846 CrossRef CAS.
- F. Luzi, L. Torre, J. Kenny and D. Puglia, Bio- and Fossil-Based Polymeric Blends and Nanocomposites for Packaging: Structure–Property Relationship, Materials, 2019, 12, 471 CrossRef CAS PubMed.
- V. Menon and M. Rao, Trends in bioconversion of lignocellulose: Biofuels, platform chemicals & biorefinery concept, Prog. Energy Combust. Sci., 2012, 38, 522–550 CrossRef CAS.
- A. Agarwal, B. Shaida, M. Rastogi and N. B. Singh, Food Packaging Materials with Special Reference to Biopolymers-Properties and Applications, Chem. Afr., 2022, 6, 117–144 CrossRef.
- Y.-H. Tsai, Y.-N. Yang, Y.-C. Ho, M.-L. Tsai and F.-L. Mi, Drug release and antioxidant/antibacterial activities of silymarin-zein nanoparticle/bacterial cellulose nanofiber composite films, Carbohydr. Polym., 2018, 180, 286–296 CrossRef CAS PubMed.
- C. Moreirinha, C. Vilela, N. H. C. S. Silva, R. J. B. Pinto, A. Almeida, M. A. M. Rocha, E. Coelho, M. A. Coimbra, A. J. D. Silvestre and C. S. R. Freire, Antioxidant and antimicrobial films based on brewers spent grain arabinoxylans, nanocellulose and feruloylated compounds for active packaging, Food Hydrocolloids, 2020, 108, 105836 CrossRef CAS.
- C. Vilela, C. Moreirinha, E. M. Domingues, F. M. L. Figueiredo, A. Almeida and C. S. R. Freire, Antimicrobial and Conductive Nanocellulose-Based Films for Active and Intelligent Food Packaging, Nanomaterials, 2019, 9, 980 CrossRef CAS PubMed.
- A. L. Missio, B. D. Mattos, D. d. F. Ferreira, W. L. E. Magalhães, D. A. Bertuol, D. A. Gatto, A. Petutschnigg and G. Tondi, Nanocellulose-tannin films: From trees to sustainable active packaging, J. Cleaner Prod., 2018, 184, 143–151 CrossRef CAS.
- N. A. El-Wakil, E. A. Hassan, R. E. Abou-Zeid and A. Dufresne, Development of wheat gluten/nanocellulose/titanium dioxide nanocomposites for active food packaging, Carbohydr. Polym., 2015, 124, 337–346 CrossRef CAS PubMed.
- W. Wang, Z. Yu, F. K. Alsammarraie, F. Kong, M. Lin and A. Mustapha, Properties and antimicrobial activity of polyvinyl alcohol-modified bacterial nanocellulose packaging films incorporated with silver nanoparticles, Food Hydrocolloids, 2020, 100, 105411 CrossRef CAS.
- B. Kuswandi, Jayus, R. Oktaviana, A. Abdullah and L. Y. Heng, A Novel On-Package Sticker Sensor Based on Methyl Red for Real-Time Monitoring of Broiler Chicken Cut Freshness, Packag. Technol. Sci., 2013, 27, 69–81 CrossRef.
- P. Lu, Y. Yang, R. Liu, X. Liu, J. Ma, M. Wu and S. Wang, Preparation of sugarcane bagasse nanocellulose hydrogel as a colourimetric freshness indicator for intelligent food packaging, Carbohydr. Polym., 2020, 249, 116831 CrossRef CAS PubMed.
- B. Johnston, I. Radecka, D. Hill, E. Chiellini, V. Ilieva, W. Sikorska, M. Musioł, M. Zięba, A. Marek, D. Keddie, B. Mendrek, S. Darbar, G. Adamus and M. Kowalczuk, The Microbial Production of Polyhydroxyalkanoates from Waste Polystyrene Fragments Attained Using Oxidative Degradation, Polymers, 2018, 10, 957 CrossRef PubMed.
- A. B. Sathya, V. Sivasubramanian, A. Santhiagu, C. Sebastian and R. Sivashankar, Production of Polyhydroxyalkanoates from Renewable Sources Using Bacteria, J. Polym. Environ., 2018, 26, 3995–4012 CrossRef CAS.
- A. B. Sathya, V. Sivasubramanian, A. Santhiagu, C. Sebastian and R. Sivashankar, Production of Polyhydroxyalkanoates from Renewable Sources Using Bacteria, J. Polym. Environ., 2018, 26, 3995–4012 CrossRef CAS.
-
D. Plackett and I. Siro, Polyhydroxyalkanoates (PHAs) for Food Packaging, Woodhead Publishing Ltd, 2011, vol. 498–526 Search PubMed.
- M. Asgher, S. A. Qamar, M. Bilal and H. M. N. Iqbal, Bio-based active food packaging materials: Sustainable alternative to conventional petrochemical-based packaging materials, Food Res. Int., 2020, 137, 109625 CrossRef CAS PubMed.
- S. Thakur, J. Chaudhary, P. Singh, W. F. Alsanie, S. A. Grammatikos and V. K. Thakur, Synthesis of Bio-based monomers and polymers using microbes for a sustainable bioeconomy, Bioresour. Technol., 2022, 344, 126156 CrossRef CAS PubMed.
- E. Balla, V. Daniilidis, G. Karlioti, T. Kalamas, M. Stefanidou, N. D. Bikiaris, A. Vlachopoulos, I. Koumentakou and D. N. Bikiaris, Poly(lactic Acid): A Versatile Biobased Polymer for the Future with Multifunctional Properties—From Monomer Synthesis, Polymerization Techniques and Molecular Weight Increase to PLA Applications, Polymers, 2021, 13, 1822 CrossRef CAS PubMed.
- V. A. I. Silveira, B. M. Marim, A. Hipólito, M. C. Gonçalves, S. Mali, R. K. T. Kobayashi and M. A. P. C. Celligoi, Characterization and antimicrobial properties of bioactive packaging films based on polylactic acid-sophorolipid for the control of foodborne pathogens, Food Packag. Shelf Life, 2020, 26, 100591 CrossRef.
- E. Korcz and L. Varga, Exopolysaccharides from lactic acid bacteria: Techno-functional application in the food industry, Trends Food Sci. Technol., 2021, 110, 375–384 CrossRef CAS.
- L. Marangoni Júnior, R. P. Vieira and C. A. R. Anjos, Kefiran-based films: Fundamental concepts, formulation strategies and properties, Carbohydr. Polym., 2020, 246, 116609 CrossRef PubMed.
- M. Moradi, J. T. Guimarães and S. Sahin, Current applications of exopolysaccharides from lactic acid bacteria in the development of food active edible packaging, Curr. Opin. Food Sci., 2021, 40, 33–39 CrossRef CAS.
- E. A. E. Sakr, M. I. Massoud and S. Ragaee, Food wastes as natural sources of lactic acid bacterial exopolysaccharides for the functional food industry: A review, Int. J. Biol. Macromol., 2021, 189, 232–241 CrossRef CAS PubMed.
- U. Qasim, A. I. Osman, A. H. Al-Muhtaseb, C. Farrell, M. Al-Abri, M. Ali, D.-V. N. Vo, F. Jamil and D. W. Rooney, Renewable cellulosic nanocomposites for food packaging to avoid fossil fuel plastic pollution: a review, Environ. Chem. Lett., 2020, 19, 613–641 CrossRef.
- Z. Yu, Y. Ji, V. Bourg, M. Bilgen and J. C. Meredith, Chitin- and cellulose-based sustainable barrier materials: a review, Emergent Mater., 2020, 3, 919–936 CrossRef CAS.
- G. Lavrič, A. Oberlintner, I. Filipova, U. Novak, B. Likozar and U. Vrabič-Brodnjak, Functional Nanocellulose, Alginate and Chitosan Nanocomposites Designed as Active Film Packaging Materials, Polymers, 2021, 13, 2523 CrossRef PubMed.
- J. S. Yaradoddi, N. R. Banapurmath, S. V. Ganachari, M. E. M. Soudagar, N. M. Mubarak, S. Hallad, S. Hugar and H. Fayaz, Biodegradable carboxymethyl cellulose based material for sustainable packaging application, Sci. Rep., 2020, 10, 21960, DOI:10.1038/s41598-020-78912-z.
- H. Shaghaleh, X. Xu and S. Wang, Current progress in production of biopolymeric materials based on cellulose, cellulose
nanofibers, and cellulose derivatives, RSC Adv., 2018, 8, 825–842 RSC.
-
M. Brienzo, Hemicellulose Biorefinery: A Sustainable Solution for Value Addition to Bio-Based Products and Bioenergy, Springer Nature, 2022 Search PubMed.
- R. Priyadarshi and J.-W. Rhim, Chitosan-based biodegradable functional films for food packaging applications, Innovative Food Sci. Emerging Technol., 2020, 62, 102346 CrossRef CAS.
- H. Wang, J. Qian and F. Ding, Emerging Chitosan-Based Films for Food Packaging Applications, J. Agric. Food Chem., 2018, 66, 395–413 CrossRef CAS PubMed.
- J. Yang, Y. Ching and C. Chuah, Applications of Lignocellulosic Fibers and Lignin in Bioplastics: A Review, Polymers, 2019, 11, 751 CrossRef CAS PubMed.
- Q. Luo, M. A. Hossen, Y. Zeng, J. Dai, S. Li, W. Qin and Y. Liu, Gelatin-based composite films and their application in food packaging: A review, J. Food Eng., 2022, 313, 110762 CrossRef CAS.
- R. A. S. N. Ranasinghe, W. L. I. Wijesekara, P. R. D. Perera, S. A. Senanayake, M. M. Pathmalal and R. A. U. J. Marapana, Functional and Bioactive Properties of Gelatin Extracted from Aquatic Bioresources – A Review, Food Rev. Int., 2020, 38, 812–855 CrossRef.
- H. Wang, F. Ding, L. Ma and Y. Zhang, Edible films from chitosan-gelatin: Physical properties and food packaging application, Food Biosci., 2021, 40, 100871 CrossRef CAS.
- H. Tian, G. Guo, X. Fu, Y. Yao, L. Yuan and A. Xiang, Fabrication, properties and applications of soy-protein-based materials: A review, Int. J. Biol. Macromol., 2018, 120, 475–490 CrossRef CAS PubMed.
- H. Chen, J. Wang, Y. Cheng, C. Wang, H. Liu, H. Bian, Y. Pan, J. Sun and W. Han, Application of Protein-Based Films and Coatings for Food Packaging: A Review, Polymers, 2019, 11, 2039 CrossRef CAS PubMed.
- Q. Ye, Y. Han, J. Zhang, W. Zhang, C. Xia and J. Li, Bio-based films with improved water resistance derived from soy protein isolate and stearic acid via bioconjugation, J. Cleaner Prod., 2019, 214, 125–131 CrossRef CAS.
- S. Chhikara and D. Kumar, Edible Coating and Edible Film as Food Packaging Material: A Review, J. Packag. Technol. Res., 2021, 6, 1–10 Search PubMed.
- M.-I. Socaciu, M. Fogarasi, C. A. Semeniuc, S. A. Socaci, M. A. Rotar, V. Mureşan, O. L. Pop and D. C. Vodnar, Formulation and Characterization of Antimicrobial Edible Films Based on Whey Protein Isolate and Tarragon Essential Oil, Polymers, 2020, 12, 1748 CrossRef CAS PubMed.
- A. Sorrentino, G. Gorrasi and V. Vittoria, Potential perspectives of bio-nanocomposites for food packaging applications, Trends Food Sci. Technol., 2007, 18, 84–95 CrossRef CAS.
- Anonymous and Xu's, Definition and classification of degradable plastics, http://www.08607559825-blog.com/definition-classification-degradableplastics.html, accessed February 2013.
- J. K. Pandey, A. P. Kumar, M. Misra, A. K. Mohanty, L. T. Drzal and R. Palsingh, Recent Advances in Biodegradable Nanocomposites, J. Nanosci. Nanotechnol., 2005, 5, 497–526 CrossRef CAS PubMed.
- G. Scott, ‘Green’ polymers, Polym. Degrad. Stab., 2000, 68, 1–7 CrossRef CAS.
- M. Trznadel, Biodegradable polymer materials, Polimery, 1995, 40, 485–492 CrossRef CAS.
- J. M. Krochta and C. De Mulder-Johnston, Edible and biodegradable polymer films: challenges and opportunities, Food Technol., 1997, 51, 61–74 Search PubMed.
- R. K. Bharadwaj, Modeling the Barrier Properties of Polymer-Layered Silicate Nanocomposites, Macromolecules, 2001, 34, 9189–9192 CrossRef CAS.
- L. E. Nielsen, Models for the Permeability of Filled Polymer Systems, J. Macromol. Sci., Part A: Chem., 1967, 1, 929–942 CrossRef CAS.
- H. C. Koh, J. S. Park, M. A. Jeong, H. Y. Hwang, Y. T. Hong, S. Y. Ha and S. Y. Nam, Preparation and gas permeation properties of biodegradable polymer/layered silicate nanocomposite membranes, Desalination, 2008, 233, 201–209 CrossRef CAS.
- A. Sorrentino, M. Tortora and V. Vittoria, Diffusion behavior in polymer-clay nanocomposites, J. Polym. Sci., Part B: Polym. Phys., 2005, 44, 265–274 CrossRef.
-
S. Z. Popović, V. L. Lazić, N. M. Hromiš, D. Z. Šuput and S. N. Bulut, Biopolymer Packaging Materials for Food Shelf-Life Prolongation, Biopolymers for Food Design, 2018, pp. 223–277 Search PubMed.
- A. Arora and G. W. Padua, Review: Nanocomposites in Food Packaging, J. Food Sci., 2010, 75, R43–R49 CrossRef CAS PubMed.
- A. Sorrentino, G. Gorrasi and V. Vittoria, Potential perspectives of bio-nanocomposites for food packaging applications, Trends Food Sci. Technol., 2007, 18, 84–95 CrossRef CAS.
- S. Sinha Ray and M. Okamoto, Polymer/layered silicate nanocomposites: a review from preparation to processing, Prog. Polym. Sci., 2003, 28, 1539–1641 CrossRef.
- S. Ray, S. Y. Quek, A. Easteal and X. D. Chen, The Potential Use of Polymer-Clay Nanocomposites in Food Packaging, Int. J. Food Eng., 2006, 2, 5, DOI:10.2202/1556-3758.1149.
- E. P. Giannelis, Polymer Layered Silicate Nanocomposites, Adv. Mater., 1996, 8, 29–35 CrossRef CAS.
- J. W. Cho and D. R. Paul, Nylon 6 nanocomposites by melt compounding, Polymer, 2001, 42, 1083–1094 CrossRef CAS.
- E. Thostenson, C. Li and T. Chou, Nanocomposites in context, Compos. Sci. Technol., 2005, 65, 491–516 CrossRef CAS.
- S. Sinharay and M. Bousmina, Biodegradable polymers and their layered silicate nanocomposites: In greening the 21st century materials world, Prog. Mater. Sci., 2005, 50, 962–1079 CrossRef.
- N. Basavegowda and K.-H. Baek, Advances in Functional Biopolymer-Based Nanocomposites for Active Food Packaging Applications, Polymers, 2021, 13, 4198 CrossRef CAS PubMed.
- S. Raina, A. Thakur, A. Sharma, D. Pooja and A. P. Minhas, Bactericidal activity of Cannabis sativa phytochemicals from leaf extract and their derived Carbon Dots and Ag@Carbon Dots, Mater. Lett., 2020, 262, 127122 CrossRef CAS.
- Y. Song, F. Lu, H. Li, H. Wang, M. Zhang, Y. Liu and Z. Kang, Degradable Carbon Dots from Cigarette Smoking with Broad-Spectrum Antimicrobial Activities against Drug-Resistant Bacteria, ACS Appl. Bio Mater., 2018, 1, 1871–1879 CrossRef CAS PubMed.
- F. Salimi, M. Moradi, H. Tajik and R. Molaei, Optimization and characterization of eco-friendly antimicrobial nanocellulose sheet prepared using carbon dots of white mulberry ( Morus alba L.), J. Sci. Food Agric., 2020, 101, 3439–3447 CrossRef PubMed.
- S. A. Kousheh, M. Moradi, H. Tajik and R. Molaei, Preparation of antimicrobial/ultraviolet protective bacterial nanocellulose film with carbon dots synthesized from lactic acid bacteria, Int. J. Biol. Macromol., 2020, 155, 216–225 CrossRef CAS PubMed.
- L. K. Deepika and K. K. Gaikwad, Carbon dots for food packaging applications, Sustainable Food Technol., 2023, 1, 185–199 RSC.
- S. Iijima, Helical microtubules of graphitic carbon, Nature, 1991, 354, 56–58 CrossRef CAS.
- M. S. Dresselhaus, G. Dresselhaus and R. Saito, Physics of carbon nanotubes, Carbon, 1995, 33, 883–891 CrossRef CAS.
- M. R. Mohammad, D. S. Ahmed and M. K. A. Mohammed, Synthesis of Ag-doped TiO2 nanoparticles coated with carbon nanotubes by the sol–gel method and their antibacterial activities, J. Sol-Gel Sci. Technol., 2019, 90, 498–509 CrossRef CAS.
- H.-Y. Yu, Z.-Y. Qin, B. Sun, X.-G. Yang and J.-M. Yao, Reinforcement of transparent poly(3-hydroxybutyrate-co-3-hydroxyvalerate) by incorporation of functionalized carbon nanotubes as a novel bionanocomposite for food packaging, Compos. Sci. Technol., 2014, 94, 96–104 CrossRef CAS.
- M. V. Dias, N. d. F. F. Soares, S. V. Borges, M. M. de Sousa, C. A. Nunes, I. R. N. de Oliveira and E. A. A. Medeiros, Use of allyl isothiocyanate and carbon nanotubes in an antimicrobial film to package shredded, cooked chicken meat, Food Chem., 2013, 141, 3160–3166 CrossRef CAS PubMed.
- A. Pattanshetti, N. Pradeep, V. Chaitra and V. Uma, Synthesis of multi-walled carbon nanotubes (MWCNTs) from plastic waste & analysis of garlic coated gelatin/MWCNTs nanocomposite films as food packaging material, SN Appl. Sci., 2020, 2, 730, DOI:10.1007/s42452-020-2442-8.
- M. T. H. Aunkor, T. Raihan, S. H. Prodhan, H. S. C. Metselaar, S. U. F. Malik and A. K. Azad, Antibacterial activity of graphene oxide nanosheet against multidrug resistant superbugs isolated from infected patients, R. Soc. Open Sci., 2020, 7, 200640 CrossRef CAS PubMed.
-
I. Rago, A. Bregnocchi, E. Zanni, A. G. D'Aloia, F. De Angelis, M. Bossu, G. De Bellis, A. Polimeni, D. Uccelletti and M. S. Sarto, Antimicrobial activity of graphene nanoplatelets against Streptococcus mutans, 2015 IEEE 15th International Conference on Nanotechnology, IEEE-NANO, DOI:10.1109/nano.2015.7388945.
- T. Vi, S. Kumar, J.-H. Pang, Y.-K. Liu, D. Chen and S. Lue, Synergistic Antibacterial Activity of Silver-Loaded Graphene Oxide towards Staphylococcus Aureus and Escherichia Coli, Nanomaterials, 2020, 10, 366 CrossRef CAS PubMed.
- D. K. Gupta, R. S. Rajaura, N. D. Jasuja and K. Sharma, Synthesis And Characterization Of Graphene Oxide Nanoparticles And Their Antibacterial Activity, J. Environ. Sci. Technol., 2015, 1, 16–24 Search PubMed.
- Y. A. Arfat, J. Ahmed, M. Ejaz and M. Mullah, Polylactide/graphene oxide nanosheets/clove essential oil composite films for potential food packaging applications, Int. J. Biol. Macromol., 2018, 107, 194–203 CrossRef CAS PubMed.
- A. Naskar, H. Khan, R. Sarkar, S. Kumar, D. Halder and S. Jana, Anti-biofilm activity and food packaging application of room temperature solution process based polyethylene glycol capped Ag-ZnO-graphene nanocomposite, Mater. Sci. Eng., C, 2018, 91, 743–753 CrossRef CAS PubMed.
- H. Wang, M. Chen, C. Jin, B. Niu, S. Jiang, X. Li and S. Jiang, Antibacterial [2-(Methacryloyloxy) ethyl] Trimethylammonium Chloride Functionalized Reduced Graphene Oxide/Poly(ethylene-co-vinyl alcohol) Multilayer Barrier Film for Food Packaging, J. Agric. Food Chem., 2018, 66, 732–739 CrossRef CAS PubMed.
- K. B. Narayanan, G. T. Park and S. S. Han, Antibacterial properties of starch-reduced graphene oxide–polyiodide nanocomposite, Food Chem., 2021, 342, 128385 CrossRef CAS PubMed.
- N. Malik, Thermally exfoliated graphene oxide reinforced polycaprolactone-based bactericidal nanocomposites for food packaging applications, Mater. Technol., 2020, 37, 345–354 CrossRef.
- K. Mitura, J. Kornacka, E. Kopczyńska, J. Kalisz, E. Czerwińska, M. Affeltowicz, W. Kaczorowski, B. Kolesińska, J. Frączyk, T. Bakalova, L. Svobodová and P. Louda, Active Carbon-Based Nanomaterials in Food Packaging, Coatings, 2021, 11, 161 CrossRef CAS.
- A. Kumar, A. Choudhary, H. Kaur, S. Mehta and A. Husen, Metal-based nanoparticles, sensors, and their multifaceted application in food packaging, J. Nanobiotechnol., 2021, 19, 256, DOI:10.1186/s12951-021-00996-0.
- A. Sharma, M. Thakur, M. Bhattacharya, T. Mandal and S. Goswami, Commercial application of cellulose nano-composites – A review, Biotechnol. Rep., 2019, 21, e00316 CrossRef PubMed.
- A. Ferrer, L. Pal and M. Hubbe, Nanocellulose in packaging: Advances in barrier layer technologies, Ind. Crops Prod., 2017, 95, 574–582 CrossRef CAS.
- B. Naseer, G. Srivastava, O. S. Qadri, S. A. Faridi, R. U. Islam and K. Younis, Importance and health hazards of nanoparticles used in the food industry, Nanotechnol. Rev., 2018, 7, 623–641 CrossRef CAS.
-
M. P. Arrieta, E. Fortunati, N. Burgos, M. A. Peltzer, J. López and L. Peponi, Nanocellulose-Based Polymeric Blends for Food Packaging Applications, Multifunctional Polymeric Nanocomposites Based on Cellulosic Reinforcements, 2016, pp. 205–252 Search PubMed.
- D. Morán, G. Gutiérrez, M. C. Blanco-López, A. Marefati, M. Rayner and M. Matos, Synthesis of Starch Nanoparticles and Their Applications for Bioactive Compound Encapsulation, Appl. Sci., 2021, 11, 4547 CrossRef.
- M. Yu, N. Ji, Y. Wang, L. Dai, L. Xiong and Q. Sun, Starch-based nanoparticles: Stimuli responsiveness, toxicity, and interactions with food components, Compr. Rev. Food Sci. Food Saf., 2020, 20, 1075–1100 CrossRef PubMed.
- C. Dularia, A. Sinhmar, R. Thory, A. K. Pathera and V. Nain, Development of starch nanoparticles based composite films from non-conventional source - Water chestnut (Trapa bispinosa), Int. J. Biol. Macromol., 2019, 136, 1161–1168 CrossRef CAS PubMed.
- P. H. Campelo, A. S. Sant'Ana and M. T. Pedrosa Silva Clerici, Starch nanoparticles: production methods, structure, and properties for food applications, Curr. Opin. Food Sci., 2020, 33, 136–140 CrossRef.
- M. Zubair and A. Ullah, Recent advances in protein derived bionanocomposites for food packaging applications, Crit. Rev. Food Sci. Nutr., 2019, 60, 406–434 CrossRef PubMed.
- X. Li, N. Ji, C. Qiu, M. Xia, L. Xiong and Q. Sun, The effect of peanut protein nanoparticles on characteristics of protein- and starch-based nanocomposite films: A comparative study, Ind. Crops Prod., 2015, 77, 565–574 CrossRef CAS.
- P. Oymaci and S. A. Altinkaya, Improvement of barrier and mechanical properties of whey protein isolate based food packaging films by incorporation of zein nanoparticles as a novel bionanocomposite, Food Hydrocolloids, 2016, 54, 1–9 CrossRef CAS.
- A. K. Biswal and P. K. Misra, Biosynthesis and characterization of silver nanoparticles for prospective application in food packaging and biomedical fields, Mater. Chem. Phys., 2020, 250, 123014 CrossRef CAS.
- M. Carbone, D. T. Donia, G. Sabbatella and R. Antiochia, Silver nanoparticles in polymeric matrices for fresh food packaging, J. King Saud Univ., Sci., 2016, 28, 273–279 CrossRef.
- S. S. Ahmad, O. Yousuf, R. U. Islam and K. Younis, Silver nanoparticles as an active packaging ingredient and its toxicity, Packag. Technol. Sci., 2021, 34, 653–663 CrossRef CAS.
- A. Istiqola and A. Syafiuddin, A review of silver nanoparticles in food packaging technologies: Regulation, methods, properties, migration, and future challenges, J. Chin. Chem. Soc., 2020, 67, 1942–1956 CrossRef CAS.
- K. Kraśniewska, S. Galus and M. Gniewosz, Biopolymers-Based Materials Containing Silver Nanoparticles as Active Packaging for Food Applications–A Review, Int. J. Mol. Sci., 2020, 21, 698 CrossRef PubMed.
- D. J. McClements and H. Xiao, Is nano safe in foods? Establishing the factors impacting the gastrointestinal fate and toxicity of organic and inorganic food-grade nanoparticles, npj Sci. Food, 2017, 1, 6, DOI:10.1038/s41538-017-0005-1.
- I. Kim, K. Viswanathan, G. Kasi, S. Thanakkasaranee, K. Sadeghi and J. Seo, ZnO Nanostructures in Active Antibacterial Food Packaging: Preparation Methods, Antimicrobial Mechanisms, Safety Issues, Future Prospects, and Challenges, Food Rev. Int., 2020, 38, 537–565 CrossRef.
- M. Abbas, M. Buntinx, W. Deferme and R. Peeters, (Bio)polymer/ZnO Nanocomposites for Packaging Applications: A Review of Gas Barrier and Mechanical Properties, Nanomaterials, 2019, 9, 1494 CrossRef CAS PubMed.
- L. C. Mohr, A. P. Capelezzo, C. R. D. M. Baretta, M. A. P. M. Martins, M. A. Fiori and J. M. M. Mello, Titanium dioxide nanoparticles applied as ultraviolet radiation blocker in the polylactic acid bidegradable polymer, Polym. Test., 2019, 77, 105867 CrossRef CAS.
- E. Baranowska-Wójcik, D. Szwajgier, P. Oleszczuk and A. Winiarska-Mieczan, Effects of Titanium Dioxide Nanoparticles Exposure on Human Health—a Review, Biol. Trace Elem. Res., 2019, 193, 118–129 CrossRef PubMed.
- Ş. Sungur, P. Kaya and M. Koroglu, Determination of titanium dioxide nanoparticles used in various foods, Food Addit. Contam.: Part Bc, 2020, 13, 260–267 CrossRef PubMed.
- G. D. Venkatasubbu, R. Baskar, T. Anusuya, C. A. Seshan and R. Chelliah, Toxicity mechanism of titanium dioxide and zinc oxide nanoparticles against food pathogens, Colloids Surf., B, 2016, 148, 600–606 CrossRef CAS PubMed.
- U. Siripatrawan and P. Kaewklin, Fabrication and characterization of chitosan-titanium dioxide nanocomposite film as ethylene scavenging and antimicrobial active food packaging, Food Hydrocolloids, 2018, 84, 125–134 CrossRef CAS.
- F. Guo, S. Aryana, Y. Han and Y. Jiao, A Review of the Synthesis and Applications of Polymer–Nanoclay Composites, Appl. Sci., 2018, 8, 1696 CrossRef.
- P. Chaudhary, F. Fatima and A. Kumar, Relevance of Nanomaterials in Food Packaging and its Advanced Future Prospects, J. Inorg. Organomet. Polym. Mater., 2020, 30, 5180–5192 CrossRef CAS PubMed.
- J. Bandyopadhyay and S. S. Ray, Are nanoclay-containing polymer composites safe for food packaging applications?—An overview, J. Appl. Polym. Sci., 2018, 136, 47214 CrossRef.
- U. Chadha, P. Bhardwaj, S. K. Selvaraj, K. Arasu, S. Praveena, A. Pavan, M. Khanna, P. Singh, S. Singh, A. Chakravorty, B. Badoni, M. Banavoth, P. Sonar and V. Paramasivam, Current Trends and Future Perspectives of Nanomaterials in Food Packaging Application, J. Nanomater., 2022, 2022, 1–32 CrossRef.
- C. Madhush, I. Munaweera and N. Kottegod, Functional Nanomaterials As Smart Food Packaging: A Brief Review, Afr. J. Agric. Food Sci., 2021, 4, 58–78 Search PubMed.
- A. Ashfaq, N. Khursheed, S. Fatima, Z. Anjum and K. Younis, Application of nanotechnology in food packaging: Pros and Cons, J. Agric. Food Res., 2022, 7, 100270, DOI:10.1016/j.jafr.2022.100270.
- H. Onyeaka, P. Passaretti, T. Miri and Z. T. Al-Sharify, The safety of nanomaterials in food production and packaging, Curr. Res. Food Sci., 2022, 5, 763–774, DOI:10.1016/j.crfs.2022.04.005.
- A. Bhardwaj, T. Alam and N. Talwar, Recent advances in active packaging of agri-food products: a review, J. Postharvest Technol., 2019, 7, 33–62 Search PubMed.
- A. Mousavi Khaneghah, S. M. B. Hashemi and S. Limbo, Antimicrobial agents and packaging systems in antimicrobial active food packaging: An overview of approaches and interactions, Food Bioprod. Process., 2018, 111, 1–19 CrossRef CAS.
- P. Lu, M. Guo, Z. Xu and M. Wu, Application of Nanofibrillated Cellulose on BOPP/LDPE Film as Oxygen Barrier and Antimicrobial Coating Based on Cold Plasma Treatment, Coatings, 2018, 8, 207 CrossRef.
- C. Vilela, M. Kurek, Z. Hayouka, B. Röcker, S. Yildirim, M. D. C. Antunes, J. Nilsen-Nygaard, M. K. Pettersen and C. S. R. Freire, A concise guide to active agents for active food packaging, Trends Food Sci. Technol., 2018, 80, 212–222 CrossRef CAS.
- K. V. P. Kumar, J. S. W and B. A. Kumari, Active packaging systems in food packaging for enhanced shelf life, J. Pharmacogn. Phytochem., 2018, 7, 2044–2204 CAS.
- Y.-T. Hung, L. A. McLandsborough, J. M. Goddard and L. J. Bastarrachea, Antimicrobial polymer coatings with efficacy against pathogenic and spoilage microorganisms, LWT–Food Sci. Technol., 2018, 97, 546–554 CrossRef CAS.
- C. Ruan, Y. Zhang, J. Wang, Y. Sun, X. Gao, G. Xiong and J. Liang, Preparation and antioxidant activity of sodium alginate and carboxymethyl cellulose edible films with epigallocatechin gallate, Int. J. Biol. Macromol., 2019, 134, 1038–1044 CrossRef CAS PubMed.
- R. Chawla, S. Sivakumar and H. Kaur, Antimicrobial edible films in food packaging: Current scenario and recent nanotechnological advancements- a review, Carbohydr. Polym. Technol. Appl., 2021, 2, 100024 CAS.
- W. A. Arnold, A. Blum, J. Branyan, T. A. Bruton, C. C. Carignan, G. Cortopassi, S. Datta, J. DeWitt, A. C. Doherty, R. U. Halden, H. Harari, E. M. Hartmann, T. C. Hrubec, S. Iyer, C. F. Kwiatkowski, J. LaPier, D. Li, L. Li, J. G. Muñiz Ortiz and G. Zheng, Quaternary Ammonium Compounds: A Chemical Class of Emerging Concern, Environ. Sci. Technol., 2023, 57(20), 7645–7665, DOI:10.1021/acs.est.2c08244.
- T. F. Nelson, R. Baumgartner, M. Jaggi, S. M. Bernasconi, G. Battagliarin, C. Sinkel, A. Künkel, H. P. E. Kohler, K. McNeill and M. Sander, Biodegradation of poly(butylene succinate) in soil laboratory incubations assessed by stable carbon isotope labelling, Nat. Commun., 2022, 13(1), 5691, DOI:10.1038/s41467-022-33064-8.
- A. Sobhan, K. Muthukumarappan and L. Wei, Biosensors and biopolymer-based nanocomposites for smart food packaging: Challenges and opportunities, Food Packag. Shelf Life, 2021, 30, 100745 CrossRef CAS.
- J. Yang, M. Shen, Y. Luo, T. Wu, X. Chen, Y. Wang and J. Xie, Advanced applications of chitosan-based hydrogels: From biosensors to intelligent food packaging system, Trends Food Sci. Technol., 2021, 110, 822–832 CrossRef CAS.
- W. Nawrot, K. Drzozga, S. Baluta, J. Cabaj and K. Malecha, A Fluorescent Biosensors for Detection Vital Body Fluids' Agents, Sensors, 2018, 18, 2357 CrossRef PubMed.
- L. El Harrad, I. Bourais, H. Mohammadi and A. Amine, Recent Advances in Electrochemical Biosensors Based on Enzyme Inhibition for Clinical and Pharmaceutical Applications, Sensors, 2018, 18, 164 CrossRef PubMed.
- K. A. Al-Maqdi, M. Bilal, A. Alzamly, H. M. N. Iqbal, I. Shah and S. S. Ashraf, Enzyme-Loaded Flower-Shaped Nanomaterials: A Versatile Platform with Biosensing, Biocatalytic, and Environmental Promise, Nanomaterials, 2021, 11, 1460 CrossRef CAS PubMed.
- J. Riu and B. Giussani, Electrochemical biosensors for the detection of pathogenic bacteria in food, TrAC, Trends Anal. Chem., 2020, 126, 115863 CrossRef CAS.
- A. Sobhan, J.-H. Oh, M.-K. Park and J. Lee, Reusability of a single-walled carbon nanotube-based biosensor for detecting peanut allergens and Y. enterocolitica, Microelectron. Eng., 2020, 225, 111281 CrossRef CAS.
-
F. Patra and R. K. Duary, in Biosensors in Food Safety and Quality, CRC Press, 1st edn, 2022, p. 24 Search PubMed.
- J.-W. Han, L. Ruiz-Garcia, J.-P. Qian and X.-T. Yang, Food Packaging: A Comprehensive Review and Future Trends, Compr. Rev. Food Sci. Food Saf., 2018, 17, 860–877 CrossRef PubMed.
- A. Agarwal, B. Shaida, M. Rastogi and N. B. Singh, Food Packaging Materials with Special Reference to Biopolymers-Properties and Applications, Chem. Afr., 2022, 6, 117–144 CrossRef.
- J. Zhou, X. Lv, J. Jia, Z. Din, S. Cai, J. He, F. Xie and J. Cai, Nanomaterials-Based Electrochemiluminescence Biosensors for Food Analysis: Recent Developments and Future Directions, Biosensors, 2022, 12, 1046 CrossRef CAS PubMed.
- L. A. M. van den Broek, R. J. I. Knoop, F. H. J. Kappen and C. G. Boeriu, Chitosan films and blends for packaging material, Carbohydr. Polym., 2015, 116, 237–242 CrossRef CAS PubMed.
- L. Sun, J. Sun, L. Chen, P. Niu, X. Yang and Y. Guo, Preparation and characterization of chitosan film incorporated with thinned young apple polyphenols as an active packaging material, Carbohydr. Polym., 2017, 163, 81–91 CrossRef CAS PubMed.
- P. Cazón, G. Velazquez, J. A. Ramírez and M. Vázquez, Polysaccharide-based films and coatings for food packaging: A review, Food Hydrocolloids, 2017, 68, 136–148 CrossRef.
- A. Verlee, S. Mincke and C. V. Stevens, Recent developments in antibacterial and antifungal chitosan and its derivatives, Carbohydr. Polym., 2017, 164, 268–283 CrossRef CAS PubMed.
- K. K. Kuorwel, M. J. Cran, J. D. Orbell, S. Buddhadasa and S. W. Bigger, Review of Mechanical Properties, Migration, and Potential Applications in Active Food Packaging Systems Containing Nanoclays and Nanosilver, Compr. Rev. Food Sci. Food Saf., 2015, 14, 411–430 CrossRef CAS.
- G. Romanazzi, E. Feliziani, S. B. Baños and D. Sivakumar, Shelf life extension of fresh fruit and vegetables by chitosan treatment, Crit. Rev. Food Sci. Nutr., 2016, 57, 579–601 CrossRef PubMed.
- M. Azmana, S. Mahmood, A. R. Hilles, A. Rahman, M. A. B. Arifin and S. Ahmed, A review on chitosan and chitosan-based bionanocomposites: Promising material for combatting global issues and its applications, Int. J. Biol. Macromol., 2021, 185, 832–848 CrossRef CAS PubMed.
- S. R. Kanatt, M. S. Rao, S. P. Chawla and A. Sharma, Effects of chitosan coating on shelf-life of ready-to-cook meat products during chilled storage, LWT–Food Sci. Technol., 2013, 53, 321–326 CrossRef CAS.
- A. Wan, Q. Xu, Y. Sun and H. Li, Antioxidant Activity of High Molecular Weight Chitosan and N,O-Quaternized Chitosans, J. Agric. Food Chem., 2013, 61, 6921–6928 CrossRef CAS PubMed.
- F. Ding, H. Deng, Y. Du, X. Shi and Q. Wang, Emerging chitin and chitosan nanofibrous materials for biomedical applications, Nanoscale, 2014, 6, 9477–9493 RSC.
- H. Wang, T. Guo and H. Li, Evaluation of viscosity and printing quality of chitosan-based flexographic inks: The effect of chitosan molecular weight, J. Appl. Polym. Sci., 2016, 133, 43997, DOI:10.1002/app.43997.
- H. Wang, J. Qian and F. Ding, Recent advances in engineered chitosan-based nanogels for biomedical applications, J. Mater. Chem. B, 2017, 5, 6986–7007 RSC.
- I. Leceta, P. Guerrero, I. Ibarburu, M. T. Dueñas and K. de la Caba, Characterization and antimicrobial analysis of chitosan-based films, J. Food Eng., 2013, 116, 889–899 CrossRef CAS.
- N. B. Gol, P. R. Patel and T. V. R. Rao, Improvement of quality and shelf-life of strawberries with edible coatings enriched with chitosan, Postharvest Biol. Technol., 2013, 85, 185–195 CrossRef CAS.
- M. Z. Treviño-Garza, S. García, M. del Socorro Flores-González and K. Arévalo-Niño, Edible Active Coatings Based on Pectin, Pullulan, and Chitosan Increase Quality and Shelf Life of Strawberries (Fragaria ananassa), J. Food Sci., 2015, 80, M1823–M1830 CrossRef PubMed.
- S. Vimaladevi, S. K. Panda, K. A. M. Xavier and J. Bindu, Packaging performance of organic acid incorporated chitosan films on dried anchovy (Stolephorus indicus), Carbohydr. Polym., 2015, 127, 189–194 CrossRef CAS PubMed.
- P. Fernández-Saiz, G. Sánchez, C. Soler, J. M. Lagaron and M. J. Ocio, Chitosan films for the microbiological preservation of refrigerated sole and hake fillets, Food Control, 2013, 34, 61–68 CrossRef.
- A. Günlü, S. Sipahioǧlu and H. Alpas, The effect of high hydrostatic pressure on the muscle proteins of rainbow trout (Oncorhynchus mykissWalbaum) fillets wrapped with chitosan-based edible film during cold storage (4±1°C), High Pressure Res., 2014, 34, 122–132 CrossRef.
- N. Erkan, Use Of Natural Preservatives In Seafood: Plant Extracts, Edible Film And Coating, J. Food Heath Sci., 2015, 1, 33–49, DOI:10.3153/jfhs15004.
- H. Wang, J. Qian and F. Ding, Emerging Chitosan-Based Films for Food Packaging Applications, J. Agric. Food Chem., 2018, 66, 395–413 CrossRef CAS PubMed.
- E. Talón, K. T. Trifkovic, V. A. Nedovic, B. M. Bugarski, M. Vargas, A. Chiralt and C. González-Martínez, Antioxidant edible films based on chitosan and starch containing polyphenols from thyme extracts, Carbohydr. Polym., 2017, 157, 1153–1161 CrossRef PubMed.
- S. Woranuch and R. Yoksan, Eugenol-loaded chitosan nanoparticles: II. Application in bio-based plastics for active packaging, Carbohydr. Polym., 2013, 96, 586–592 CrossRef CAS PubMed.
- S. Alix, A. Mahieu, C. Terrie, J. Soulestin, E. Gerault, M. G. J. Feuilloley, R. Gattin, V. Edon, T. Ait-Younes and N. Leblanc, Active pseudo-multilayered films from polycaprolactone and starch based matrix for food-packaging applications, Eur. Polym. J., 2013, 49, 1234–1242 CrossRef CAS.
- W. Xiao, J. Xu, X. Liu, Q. Hu and J. Huang, Antibacterial hybrid materials fabricated by nanocoating of microfibril bundles of cellulose substance with titania/chitosan/silver-nanoparticle composite films, J. Mater. Chem. B, 2013, 1, 3477 RSC.
- M. Bansal, G. S. Chauhan, A. Kaushik and A. Sharma, Extraction and functionalization of bagasse cellulose nanofibres to Schiff-base based antimicrobial membranes, Int. J. Biol. Macromol., 2016, 91, 887–894 CrossRef CAS PubMed.
- A. Khan, S. Salmieri, C. Fraschini, J. Bouchard, B. Riedl and M. Lacroix, Genipin Cross-Linked Nanocomposite Films for the Immobilization of Antimicrobial Agent, ACS Appl. Mater. Interfaces, 2014, 6, 15232–15242 CrossRef CAS PubMed.
- F. Li, P. Biagioni, M. Finazzi, S. Tavazzi and L. Piergiovanni, Tunable green oxygen barrier through layer-by-layer self-assembly of chitosan and cellulose nanocrystals, Carbohydr. Polym., 2013, 92, 2128–2134 CrossRef CAS PubMed.
- J. Sundaram, J. Pant, M. J. Goudie, S. Mani and H. Handa, Antimicrobial and Physicochemical Characterization of Biodegradable, Nitric Oxide-Releasing Nanocellulose–Chitosan Packaging Membranes, J. Agric. Food Chem., 2016, 64, 5260–5266 CrossRef CAS PubMed.
- A. M. Youssef, S. M. EL-Sayed, H. S. EL-Sayed, H. H. Salama and A. Dufresne, Enhancement of Egyptian soft white cheese shelf life using a novel chitosan/carboxymethyl cellulose/zinc oxide bionanocomposite film, Carbohydr. Polym., 2016, 151, 9–19 CrossRef CAS PubMed.
- N. Noshirvani, B. Ghanbarzadeh, R. Rezaei Mokarram and M. Hashemi, Novel active packaging based on carboxymethyl cellulose-chitosan-ZnO NPs nanocomposite for increasing the shelf life of bread, Food Packag. Shelf Life, 2017, 11, 106–114 CrossRef.
- A. Acevedo-Fani, L. Salvia-Trujillo, R. Soliva-Fortuny and O. Martín-Belloso, Modulating Biopolymer Electrical Charge to Optimize the Assembly of Edible Multilayer Nanofilms by the Layer-by-Layer Technique, Biomacromolecules, 2015, 16, 2895–2903 CrossRef CAS PubMed.
- E. Poverenov, S. Danino, B. Horev, R. Granit, Y. Vinokur and V. Rodov, Layer-by-Layer Electrostatic Deposition of Edible Coating on Fresh Cut Melon Model: Anticipated and Unexpected Effects of Alginate–Chitosan Combination, Food Bioprocess Technol., 2013, 7, 1424–1432 CrossRef.
- M. P. Souza, A. F. M. Vaz, M. A. Cerqueira, J. A. Texeira, A. A. Vicente and M. G. Carneiro-da-Cunha, Effect of an Edible Nanomultilayer Coating by Electrostatic Self-Assembly on the Shelf Life of Fresh-Cut Mangoes, Food Bioprocess Technol., 2014, 8, 647–654 CrossRef.
- M. A. da Silva, B. T. Iamanaka, M. H. Taniwaki and T. G. Kieckbusch, Evaluation of the Antimicrobial Potential of Alginate and Alginate/Chitosan Films Containing Potassium Sorbate and Natamycin, Packag. Technol. Sci., 2012, 26, 479–492 CrossRef.
- P. D. Hoagland and N. Parris, Chitosan/Pectin Laminated Films, J. Agric. Food Chem., 1996, 44, 1915–1919 CrossRef CAS.
- V. B. V. Maciel, C. M. P. Yoshida and T. T. Franco, Chitosan/pectin polyelectrolyte complex as a pH indicator, Carbohydr. Polym., 2015, 132, 537–545 CrossRef CAS PubMed.
- M. E. Martiñon, R. G. Moreira, M. E. Castell-Perez and C. Gomes, Development of a multilayered antimicrobial edible coating for shelf-life extension of fresh-cut cantaloupe (Cucumis melo L.) stored at 4 °C, LWT–Food Sci. Technol., 2014, 56, 341–350 CrossRef.
- M. V. Lorevice, C. G. Otoni, M. R. de Moura and L. H. C. Mattoso, Chitosan nanoparticles on the improvement of thermal, barrier, and mechanical properties of high- and low-methyl pectin films, Food Hydrocolloids, 2016, 52, 732–740 CrossRef CAS.
- X. Sun, S. Sui, C. Ference, Y. Zhang, S. Sun, N. Zhou, W. Zhu and K. Zhou, Antimicrobial and Mechanical Properties of β-Cyclodextrin Inclusion with Essential Oils in Chitosan Films, J. Agric. Food Chem., 2014, 62, 8914–8918 CrossRef CAS PubMed.
- L. Higueras, G. López-Carballo, P. Hernández-Muñoz, R. Catalá and R. Gavara, Antimicrobial packaging of chicken fillets based on the release of carvacrol from chitosan/cyclodextrin films, Int. J. Food Microbiol., 2014, 188, 53–59 CrossRef CAS PubMed.
- V. Rizzi, P. Fini, F. Fanelli, T. Placido, P. Semeraro, T. Sibillano, A. Fraix, S. Sortino, A. Agostiano, C. Giannini and P. Cosma, Molecular interactions, characterization and photoactivity of Chlorophyll a/chitosan/2-HP-β-cyclodextrin composite films as functional and active surfaces for ROS production, Food Hydrocolloids, 2016, 58, 98–112 CrossRef CAS.
- K. Khwaldia, A. H. Basta, H. Aloui and H. El-Saied, Chitosan–caseinate bilayer coatings for paper packaging materials, Carbohydr. Polym., 2014, 99, 508–516 CrossRef CAS PubMed.
- M. Ahmad, N. P. Nirmal, M. Danish, J. Chuprom and S. Jafarzedeh, Characterisation of composite films fabricated from collagen/chitosan and collagen/soy protein isolate for food packaging applications, RSC Adv., 2016, 6, 82191–82204 RSC.
- M. Yuceer and C. Caner, Antimicrobial lysozyme-chitosan coatings affect functional properties and shelf life of chicken eggs during storage, J. Sci. Food Agric., 2013, 94, 153–162 CrossRef PubMed.
- F. Liu, J. Antoniou, Y. Li, J. Yi, W. Yokoyama, J. Ma and F. Zhong, Preparation of Gelatin Films Incorporated with Tea Polyphenol Nanoparticles for Enhancing Controlled-Release Antioxidant Properties, J. Agric. Food Chem., 2015, 63, 3987–3995 CrossRef CAS PubMed.
- S. F. Hosseini, M. Rezaei, M. Zandi and F. Farahmandghavi, Fabrication of bio-nanocomposite films based on fish gelatin reinforced with chitosan nanoparticles, Food Hydrocolloids, 2015, 44, 172–182 CrossRef CAS.
- S. F. Hosseini, M. Rezaei, M. Zandi and F. Farahmandghavi, Preparation and Characterization of Chitosan Nanoparticles-Loaded Fish Gelatin-Based Edible Films, J. Food Process Eng., 2015, 39, 521–530 CrossRef.
- S. F. Hosseini, M. Rezaei, M. Zandi and F. Farahmandghavi, Development of bioactive fish gelatin/chitosan nanoparticles composite films with antimicrobial properties, Food Chem., 2016, 194, 1266–1274 CrossRef CAS PubMed.
- S. F. Hosseini, M. Rezaei, M. Zandi and F. F. Ghavi, Preparation and functional properties of fish gelatin–chitosan blend edible films, Food Chem., 2013, 136, 1490–1495 CrossRef PubMed.
- D. Kowalczyk, M. Kordowska-Wiater, J. Nowak and B. Baraniak, Characterization of films
based on chitosan lactate and its blends with oxidized starch and gelatin, Int. J. Biol. Macromol., 2015, 77, 350–359 CrossRef CAS PubMed.
- W. Ma, C.-H. Tang, X.-Q. Yang and S.-W. Yin, Fabrication and characterization of kidney bean (Phaseolus vulgaris L.) protein isolate–chitosan composite films at acidic pH, Food Hydrocolloids, 2013, 31, 237–247 CrossRef CAS.
- L. Abugoch, C. Tapia, D. Plasencia, A. Pastor, O. Castro-Mandujano, L. López and V. H. Escalona, Shelf-life of fresh blueberries coated with quinoa protein/chitosan/sunflower oil edible film, J. Sci. Food Agric., 2015, 96, 619–626 CrossRef PubMed.
- N. Caro, E. Medina, M. Díaz-Dosque, L. López, L. Abugoch and C. Tapia, Novel active packaging based on films of chitosan and chitosan/quinoa protein printed with chitosan-tripolyphosphate-thymol nanoparticles via thermal ink-jet printing, Food Hydrocolloids, 2016, 52, 520–532 CrossRef CAS.
- M. Imran, A. Klouj, A.-M. Revol-Junelles and S. Desobry, Controlled release of nisin from HPMC, sodium caseinate, poly-lactic acid and chitosan for active packaging applications, J. Food Eng., 2014, 143, 178–185 CrossRef CAS.
- Z. Song, F. Li, H. Guan, Y. Xu, Q. Fu and D. Li, Combination of nisin and ε-polylysine with chitosan coating inhibits the white blush of fresh-cut carrots, Food Control, 2017, 74, 34–44 CrossRef CAS.
- E. Velickova, E. Winkelhausen, S. Kuzmanova, V. D. Alves and M. Moldão-Martins, Impact of chitosan-beeswax edible coatings on the quality of fresh strawberries (Fragaria ananassa cv Camarosa) under commercial storage conditions, LWT–Food Sci. Technol., 2013, 52, 80–92 CrossRef CAS.
- E. Barrera, J. Gil, A. Restrepo, K. Mosquera and D. Durango, A coating of chitosan and propolis extract for the postharvest treatment of papaya (Carica papaya L. cv. Hawaiiana), Rev. Fac. Nac. Agron. Medellin, 2015, 68, 7667–7678 CrossRef.
- U. Siripatrawan and W. Vitchayakitti, Improving functional properties of chitosan films as active food packaging by incorporating with propolis, Food Hydrocolloids, 2016, 61, 695–702 CrossRef CAS.
- L. Wang, Q. Wang, J. Tong and J. Zhou, Physicochemical Properties of Chitosan Films Incorporated with Honeysuckle Flower Extract for Active Food Packaging, J. Food Process Eng., 2015, 40, e12305 CrossRef.
- L. Iturriaga, I. Olabarrieta, A. Castellan, C. Gardrat and V. Coma, Active naringin-chitosan films: Impact of UV irradiation, Carbohydr. Polym., 2014, 110, 374–381 CrossRef CAS PubMed.
- S. Woranuch and R. Yoksan, Eugenol-loaded chitosan nanoparticles: II. Application in bio-based plastics for active packaging, Carbohydr. Polym., 2013, 96, 586–592 CrossRef CAS PubMed.
- E. Genskowsky, L. A. Puente, J. A. Pérez-Álvarez, J. Fernandez-Lopez, L. A. Muñoz and M. Viuda-Martos, Assessment of antibacterial and antioxidant properties of chitosan edible films incorporated with maqui berry (Aristotelia chilensis), LWT–Food Sci. Technol., 2015, 64, 1057–1062 CrossRef CAS.
- E. Talón, K. T. Trifkovic, V. A. Nedovic, B. M. Bugarski, M. Vargas, A. Chiralt and C. González-Martínez, Antioxidant edible films based on chitosan and starch containing polyphenols from thyme extracts, Carbohydr. Polym., 2017, 157, 1153–1161 CrossRef PubMed.
- S. Rivero, M. A. García and A. Pinotti, Heat Treatment To Modify the Structural and Physical Properties of Chitosan-Based Films, J. Agric. Food Chem., 2011, 60, 492–499 CrossRef PubMed.
- L. Sun, J. Sun, L. Chen, P. Niu, X. Yang and Y. Guo, Preparation and characterization of chitosan film incorporated with thinned young apple polyphenols as an active packaging material, Carbohydr. Polym., 2017, 163, 81–91 CrossRef CAS PubMed.
- J. F. Rubilar, R. M. S. Cruz, H. D. Silva, A. A. Vicente, I. Khmelinskii and M. C. Vieira, Physico-mechanical
properties of chitosan films with carvacrol and grape seed extract, J. Food Eng., 2013, 115, 466–474 CrossRef CAS.
- P. Guo, J. D. Anderson, J. J. Bozell and S. Zivanovic, The effect of solvent composition on grafting gallic acid onto chitosan via carbodiimide, Carbohydr. Polym., 2016, 140, 171–180 CrossRef CAS PubMed.
- C. Wu, J. Tian, S. Li, T. Wu, Y. Hu, S. Chen, T. Sugawara and X. Ye, Structural properties of films and rheology of film-forming solutions of chitosan gallate for food packaging, Carbohydr. Polym., 2016, 146, 10–19 CrossRef CAS PubMed.
- M. Xie, B. Hu, Y. Wang and X. Zeng, Grafting of Gallic Acid onto Chitosan Enhances Antioxidant Activities and Alters Rheological Properties of the Copolymer, J. Agric. Food Chem., 2014, 62, 9128–9136 CrossRef CAS PubMed.
- S. B. Schreiber, J. J. Bozell, D. G. Hayes and S. Zivanovic, Introduction of primary antioxidant activity to chitosan for application as a multifunctional food packaging material, Food Hydrocolloids, 2013, 33, 207–214 CrossRef CAS.
- X. Sun, Z. Wang, H. Kadouh and K. Zhou, The antimicrobial, mechanical, physical and structural properties of chitosan–gallic acid films, LWT–Food Sci. Technol., 2014, 57, 83–89 CrossRef CAS.
- L. Wang, F. Liu, Y. Jiang, Z. Chai, P. Li, Y. Cheng, H. Jing and X. Leng, Synergistic Antimicrobial Activities of Natural Essential Oils with Chitosan Films, J. Agric. Food Chem., 2011, 59, 12411–12419 CrossRef CAS PubMed.
- F. M. Pelissari, M. V. E. Grossmann, F. Yamashita and E. A. G. Pineda, Antimicrobial, Mechanical, and Barrier Properties of Cassava Starch−Chitosan Films Incorporated with Oregano Essential Oil, J. Agric. Food Chem., 2009, 57, 7499–7504 CrossRef CAS PubMed.
- T. Jiang, L. Feng and X. Zheng, Effect of Chitosan Coating Enriched with Thyme Oil on Postharvest Quality and Shelf Life of Shiitake Mushroom (Lentinus edodes), J. Agric. Food Chem., 2011, 60, 188–196 CrossRef PubMed.
- H. Wang, R. Zhang, H. Zhang, S. Jiang, H. Liu, M. Sun and S. Jiang, Kinetics and functional effectiveness of nisin loaded antimicrobial packaging film based on chitosan/poly(vinyl alcohol), Carbohydr. Polym., 2015, 127, 64–71 CrossRef CAS PubMed.
- A. Giannakas, M. Vlacha, C. Salmas, A. Leontiou, P. Katapodis, H. Stamatis, N.-M. Barkoula and A. Ladavos, Preparation, characterization, mechanical, barrier and antimicrobial properties of chitosan/PVOH/clay nanocomposites, Carbohydr. Polym., 2016, 140, 408–415 CrossRef CAS PubMed.
- X. Feng, X. Wang, W. Xing, B. Yu, L. Song and Y. Hu, Simultaneous Reduction and Surface Functionalization of Graphene Oxide by Chitosan and Their Synergistic Reinforcing Effects in PVA Films, Ind. Eng. Chem. Res., 2013, 52, 12906–12914 CrossRef CAS.
- A. K. Pal and V. Katiyar, Nanoamphiphilic Chitosan Dispersed Poly(lactic acid) Bionanocomposite Films with Improved Thermal, Mechanical, and Gas Barrier Properties, Biomacromolecules, 2016, 17, 2603–2618 CrossRef CAS PubMed.
- P. Bie, P. Liu, L. Yu, X. Li, L. Chen and F. Xie, The properties of antimicrobial films derived from poly(lactic acid)/starch/chitosan blended matrix, Carbohydr. Polym., 2013, 98, 959–966 CrossRef CAS PubMed.
- Y. Zhang, M. Zhang and H. Yang, Postharvest chitosan-g-salicylic acid application alleviates chilling injury and preserves cucumber fruit quality during cold storage, Food Chem., 2015, 174, 558–563 CrossRef CAS PubMed.
- H. Wang, D. Chen and C. Chuai, Mechanical and Barrier Properties of LLDPE/Chitosan Blown Films for Packaging, Packag. Technol. Sci., 2015, 28, 915–923 CrossRef CAS.
- A. P. Martínez-Camacho, M. O. Cortez-Rocha, A. Z. Graciano-Verdugo, F. Rodríguez-Félix, M. M. Castillo-Ortega, A. Burgos-Hernández, J. M. Ezquerra-Brauer and M. Plascencia-Jatomea, Extruded films of blended chitosan, low density polyethylene and ethylene acrylic acid, Carbohydr. Polym., 2013, 91, 666–674 CrossRef PubMed.
- I. Kohsari, Z. Shariatinia and S. M. Pourmortazavi, Antibacterial electrospun chitosan-polyethylene oxide nanocomposite mats containing ZIF-8 nanoparticles, Int. J. Biol. Macromol., 2016, 91, 778–788 CrossRef CAS PubMed.
- S. Alix, A. Mahieu, C. Terrie, J. Soulestin, E. Gerault, M. G. J. Feuilloley, R. Gattin, V. Edon, T. Ait-Younes and N. Leblanc, Active pseudo-multilayered films from polycaprolactone and starch based matrix for food-packaging applications, Eur. Polym. J., 2013, 49, 1234–1242 CrossRef CAS.
- M. Guo, T. Z. Jin, M. P. Yadav and R. Yang, Antimicrobial property and microstructure of micro-emulsion edible composite films against Listeria, Int. J. Food Microbiol., 2015, 208, 58–64 CrossRef CAS PubMed.
- A. K. Pal and V. Katiyar, Nanoamphiphilic Chitosan Dispersed Poly(lactic acid) Bionanocomposite Films with Improved Thermal, Mechanical, and Gas Barrier Properties, Biomacromolecules, 2016, 17, 2603–2618 CrossRef CAS PubMed.
- G. López-Carballo, L. Higueras, R. Gavara and P. Hernández-Muñoz, Silver Ions Release from Antibacterial Chitosan Films Containing in Situ Generated Silver Nanoparticles, J. Agric. Food Chem., 2012, 61, 260–267 CrossRef PubMed.
- A. M. Youssef, M. S. Abdel-Aziz and S. M. El-Sayed, Chitosan nanocomposite films based on Ag-NP and Au-NP biosynthesis by Bacillus Subtilis as packaging materials, Int. J. Biol. Macromol., 2014, 69, 185–191 CrossRef CAS PubMed.
- M. Kaur, A. Kalia and A. Thakur, Effect of biodegradable chitosan-rice-starch nanocomposite films on post-harvest quality of stored peach fruit, Starch/Staerke, 2017, 69, 1600208 CrossRef.
- A. M. Youssef, H. Abou-Yousef, S. M. El-Sayed and S. Kamel, Mechanical and antibacterial properties of novel high performance chitosan/nanocomposite films, Int. J. Biol. Macromol., 2015, 76, 25–32 CrossRef CAS PubMed.
- S. Sanuja, A. Agalya and M. J. Umapathy, Synthesis and characterization of zinc oxide–neem oil–chitosan bionanocomposite for food packaging application, Int. J. Biol. Macromol., 2015, 74, 76–84 CrossRef CAS PubMed.
- L. Al-Naamani, S. Dobretsov and J. Dutta, Chitosan-zinc oxide nanoparticle composite coating for active food packaging applications, Innovative Food Sci. Emerging Technol., 2016, 38, 231–237 CrossRef CAS.
- A. M. Youssef, S. M. EL-Sayed, H. S. EL-Sayed, H. H. Salama and A. Dufresne, Enhancement of Egyptian soft white cheese shelf life using a novel chitosan/carboxymethyl cellulose/zinc oxide bionanocomposite film, Carbohydr. Polym., 2016, 151, 9–19 CrossRef CAS PubMed.
- P. Tzeng, B. Stevens, I. Devlaming and J. C. Grunlan, Polymer–Graphene Oxide Quadlayer Thin-Film Assemblies with Improved Gas Barrier, Langmuir, 2015, 31, 5919–5927 CrossRef CAS PubMed.
- N. Yan, F. Capezzuto, M. Lavorgna, G. G. Buonocore, F. Tescione, H. Xia and L. Ambrosio, Borate cross-linked graphene oxide–chitosan as robust and high gas barrier films, Nanoscale, 2016, 8, 10783–10791 RSC.
- W. Xu, W. Xie, X. Huang, X. Chen, N. Huang, X. Wang and J. Liu, The graphene oxide and chitosan biopolymer loads TiO2 for antibacterial and preservative research, Food Chem., 2017, 221, 267–277 CrossRef CAS PubMed.
- C. Demitri, V. M. De Benedictis, M. Madaghiele, C. E. Corcione and A. Maffezzoli, Nanostructured active chitosan-based films for food packaging applications: Effect of graphene stacks on mechanical properties, Measurement, 2016, 90, 418–423 CrossRef.
- A. Giannakas, M. Vlacha, C. Salmas, A. Leontiou, P. Katapodis, H. Stamatis, N.-M. Barkoula and A. Ladavos, Preparation, characterization, mechanical, barrier and antimicrobial properties of chitosan/PVOH/clay nanocomposites, Carbohydr. Polym., 2016, 140, 408–415 CrossRef CAS PubMed.
- L. Zhang, H. Wang, C. Jin, R. Zhang, L. Li, X. Li and S. Jiang, Sodium lactate loaded chitosan-polyvinyl alcohol/montmorillonite composite film towards active food packaging, Innovative Food Sci. Emerging Technol., 2017, 42, 101–108 CrossRef CAS.
- M. Abdollahi, M. Rezaei and G. Farzi, A novel active bionanocomposite film incorporating rosemary essential oil and nanoclay into chitosan, J. Food Eng., 2012, 111, 343–350 CrossRef CAS.
- Y. Kasirga, A. Oral and C. Caner, Preparation and characterization of chitosan/montmorillonite-K10 nanocomposites films for food packaging applications, Polym. Compos., 2012, 33, 1874–1882 CrossRef CAS.
- J. F. Rubilar, D. Candia, A. Cobos, O. Díaz and F. Pedreschi, Effect of nanoclay and ethyl-Nα-dodecanoyl-l-arginate hydrochloride (LAE) on physico-mechanical properties of chitosan films, LWT–Food Sci. Technol., 2016, 72, 206–214 CrossRef CAS.
- M. Zhou, Q. Liu, S. Wu, Z. Gou, X. Wu and D. Xu, Starch/chitosan films reinforced with polydopamine modified MMT: Effects of dopamine concentration, Food Hydrocolloids, 2016, 61, 678–684 CrossRef CAS.
- M. Vlacha, A. Giannakas, P. Katapodis, H. Stamatis, A. Ladavos and N.-M. Barkoula, On the efficiency of oleic acid as plasticizer of chitosan/clay nanocomposites and its role on thermo-mechanical, barrier and antimicrobial properties – Comparison with glycerol, Food Hydrocolloids, 2016, 57, 10–19 CrossRef CAS.
- S. Shi, W. Wang, L. Liu, S. Wu, Y. Wei and W. Li, Effect of chitosan/nano-silica coating on the physicochemical characteristics of longan fruit under ambient temperature, J. Food Eng., 2013, 118, 125–131 CrossRef CAS.
- H. Song, W. Yuan, P. Jin, W. Wang, X. Wang, L. Yang and Y. Zhang, Effects of chitosan/nano-silica on postharvest quality and antioxidant capacity of loquat fruit during cold storage, Postharvest Biol. Technol., 2016, 119, 41–48 CrossRef CAS.
- G. C. Pradhan, S. Dash and S. K. Swain, Barrier properties of nano silicon carbide designed chitosan nanocomposites, Carbohydr. Polym., 2015, 134, 60–65 CrossRef CAS PubMed.
- W. Xiao, J. Xu, X. Liu, Q. Hu and J. Huang, Antibacterial hybrid materials fabricated by nanocoating of microfibril bundles of cellulose substance with titania/chitosan/silver-nanoparticle composite films, J. Mater. Chem. B, 2013, 1, 3477 RSC.
- G. Xiao, X. Zhang, Y. Zhao, H. Su and T. Tan, The behavior of active bactericidal and antifungal coating under visible light irradiation, Appl. Surf. Sci., 2014, 292, 756–763 CrossRef CAS.
- R. T. De Silva, M. M. M. G. P. G. Mantilaka, S. P. Ratnayake, G. A. J. Amaratunga and K. M. N. de Silva, Nano-MgO reinforced chitosan nanocomposites for high performance packaging applications with improved mechanical, thermal and barrier properties, Carbohydr. Polym., 2017, 157, 739–747 CrossRef CAS PubMed.
- S. Sanuja, A. Agalya and M. J. Umapathy, Studies on Magnesium Oxide Reinforced Chitosan Bionanocomposite Incorporated with Clove Oil for Active Food Packaging Application, Int. J. Polym. Mater. Polym. Biomater., 2014, 63, 733–740 CrossRef CAS.
- M. Shahbazi, G. Rajabzadeh and S. J. Ahmadi, Characterization of nanocomposite film based on chitosan intercalated in clay platelets by electron beam irradiation, Carbohydr. Polym., 2017, 157, 226–235 CrossRef CAS PubMed.
- T. Pan, S. Xu, Y. Dou, X. Liu, Z. Li, J. Han, H. Yan and M. Wei, Remarkable oxygen barrier films based on a layered double hydroxide/chitosan hierarchical structure, J. Mater. Chem. A, 2015, 3, 12350–12356 RSC.
- S. K. Swain, S. Dash, S. K. Kisku and R. K. Singh, Thermal and Oxygen Barrier Properties of Chitosan Bionanocomposites by Reinforcement of Calcium Carbonate Nanopowder, J. Mater. Sci. Technol., 2014, 30, 791–795 CrossRef CAS.
- H. Wu, D. Wang, J. Shi, S. Xue and M. Gao, Effect of the Complex of Zinc(II) and Cerium(IV) with Chitosan on the Preservation Quality and Degradation of Organophosphorus Pesticides in Chinese Jujube (Zizyphus jujuba Mill. cv. Dongzao), J. Agric. Food Chem., 2010, 58, 5757–5762 CrossRef CAS PubMed.
- M. Curcio, F. Puoci, F. Iemma, O. I. Parisi, G. Cirillo, U. G. Spizzirri and N. Picci, Covalent Insertion of Antioxidant Molecules on Chitosan by a Free Radical Grafting Procedure, J. Agric. Food Chem., 2009, 57, 5933–5938 CrossRef CAS PubMed.
- L. Fernández-de Castro, M. Mengíbar, Á. Sánchez, L. Arroyo, M. C. Villarán, E. Díaz de Apodaca and Á. Heras, Films of chitosan and chitosan-oligosaccharide neutralized and thermally treated: Effects on its antibacterial and other activities, LWT–Food Sci. Technol., 2016, 73, 368–374 CrossRef.
- S. Wu, Effect of chitosan-based edible coating on preservation of white shrimp during partially frozen storage, Int. J. Biol. Macromol., 2014, 65, 325–328 CrossRef CAS PubMed.
- R. Belalia, S. Grelier, M. Benaissa and V. Coma, New Bioactive Biomaterials Based on Quaternized Chitosan, J. Agric. Food Chem., 2008, 56, 1582–1588 CrossRef CAS PubMed.
- D. Mitra, M. Li, R. Wang, Z. Tang, E.-T. Kang and K. G. Neoh, Scalable Aqueous-Based Process for Coating Polymer and Metal Substrates with Stable Quaternized Chitosan Antibacterial Coatings, Ind. Eng. Chem. Res., 2016, 55, 9603–9613 CrossRef CAS.
- D. Hu, H. Wang and L. Wang, Physical properties and antibacterial activity of quaternized chitosan/carboxymethyl cellulose blend films, LWT–Food Sci. Technol., 2016, 65, 398–405 CrossRef CAS.
- M. T. McDonnell, D. A. Greeley, K. M. Kit and D. J. Keffer, Molecular Dynamics Simulations of Hydration Effects on Solvation, Diffusivity, and Permeability in Chitosan/Chitin Films, J. Phys. Chem. B, 2016, 120, 8997–9010 CrossRef CAS PubMed.
- H. Jafari, M. Pirouzifard, M. A. Khaledabad and H. Almasi, Effect of chitin nanofiber on the morphological and physical properties of chitosan/silver nanoparticle bionanocomposite films, Int. J. Biol. Macromol., 2016, 92, 461–466 CrossRef CAS PubMed.
- A. R. V. Ferreira, C. A. V. Torres, F. Freitas, C. Sevrin, C. Grandfils, M. A. M. Reis, V. D. Alves and I. M. Coelhoso, Development and characterization of bilayer films of FucoPol and chitosan, Carbohydr. Polym., 2016, 147, 8–15 CrossRef CAS PubMed.
- Y. Wu, X. Luo, W. Li, R. Song, J. Li, Y. Li, B. Li and S. Liu, Green and biodegradable composite films with novel antimicrobial performance based on cellulose, Food Chem., 2016, 197, 250–256 CrossRef CAS PubMed.
- P. Kumari, K. Barman, V. B. Patel, M. W. Siddiqui and B. Kole, Reducing postharvest pericarp browning and preserving health promoting compounds of litchi fruit by combination treatment of salicylic acid and chitosan, Sci. Hortic., 2015, 197, 555–563 CrossRef CAS.
- G. L. Dotto, M. L. G. Vieira and L. A. A. Pinto, Use of chitosan solutions for the microbiological shelf life extension of papaya fruits during storage at room temperature, LWT–Food Sci. Technol., 2015, 64, 126–130 CrossRef CAS.
- L. Al-Naamani, S. Dobretsov and J. Dutta, Chitosan-zinc oxide nanoparticle composite coating for active food packaging applications, Innovative Food Sci. Emerging Technol., 2016, 38, 231–237 CrossRef CAS.
- A. M. Díez-Pascual and A. L. Díez-Vicente, Antimicrobial and sustainable food packaging based on poly(butylene adipate-co-terephthalate) and electrospun chitosan nanofibers, RSC Adv., 2015, 5, 93095–93107 RSC.
- S. del Hoyo-Gallego, L. Pérez-Álvarez, F. Gómez-Galván, E. Lizundia, I. Kuritka, V. Sedlarik, J. M. Laza and J. L. Vila-Vilela, Construction of antibacterial poly(ethylene terephthalate) films via layer by layer assembly of chitosan and hyaluronic acid, Carbohydr. Polym., 2016, 143, 35–43 CrossRef CAS PubMed.
- E. Latou, S. F. Mexis, A. V. Badeka, S. Kontakos and M. G. Kontominas, Combined effect of chitosan and modified atmosphere packaging for shelf life extension of chicken breast fillets, LWT–Food Sci. Technol., 2014, 55, 263–268 CrossRef CAS.
- A. B. de Aquino, A. F. Blank and L. C. L. de Aquino Santana, Impact of edible chitosan–cassava starch coatings enriched with Lippia gracilis Schauer genotype mixtures on the shelf life of guavas (Psidium guajava L.) during storage at room temperature, Food Chem., 2015, 171, 108–116 CrossRef CAS PubMed.
- A. M. Youssef, S. M. EL-Sayed, H. S. EL-Sayed, H. H. Salama and A. Dufresne, Enhancement of Egyptian soft white cheese shelf life using a novel chitosan/carboxymethyl cellulose/zinc oxide bionanocomposite film, Carbohydr. Polym., 2016, 151, 9–19 CrossRef CAS PubMed.
- X. Shao, B. Cao, F. Xu, S. Xie, D. Yu and H. Wang, Effect of postharvest application of chitosan combined with clove oil against citrus green mold, Postharvest Biol. Technol., 2015, 99, 37–43 CrossRef CAS.
- A. M. Youssef, H. Abou-Yousef, S. M. El-Sayed and S. Kamel, Mechanical and antibacterial properties of novel high performance chitosan/nanocomposite films, Int. J. Biol. Macromol., 2015, 76, 25–32 CrossRef CAS PubMed.
- I. Bano, M. A. Ghauri, T. Yasin, Q. Huang and A. D. Palaparthi, Characterization and potential applications of gamma irradiated chitosan and its blends with poly(vinyl alcohol), Int. J. Biol. Macromol., 2014, 65, 81–88 CrossRef CAS PubMed.
- J. Hafsa, M. ali Smach, M. R. Ben Khedher, B. Charfeddine, K. Limem, H. Majdoub and S. Rouatbi, Physical, antioxidant and antimicrobial properties of chitosan films containing Eucalyptus globulus essential oil, LWT–Food Sci. Technol., 2016, 68, 356–364 CrossRef CAS.
- C. E. Ochoa-Velasco and J. Á. Guerrero-Beltrán, Postharvest quality of peeled prickly pear fruit treated with acetic acid and chitosan, Postharvest Biol. Technol., 2014, 92, 139–145 CrossRef CAS.
- L. Landi, E. Feliziani and G. Romanazzi, Expression of Defense Genes in Strawberry Fruits Treated with Different Resistance Inducers, J. Agric. Food Chem., 2014, 62, 3047–3056 CrossRef CAS PubMed.
- M. V. Bhaskara Reddy, J. Arul, P. Angers and L. Couture, Chitosan Treatment of Wheat Seeds Induces Resistance toFusarium graminearumand Improves Seed Quality, J. Agric. Food Chem., 1999, 47, 1208–1216 CrossRef CAS PubMed.
- X. Zhang, S. Lu and X. Chen, A visual pH sensing film using natural dyes from Bauhinia blakeana Dunn, Sens. Actuators, B, 2014, 198, 268–273 CrossRef CAS.
- L. Dai, R. Li, Y. Liang, Y. Liu, W. Zhang and S. Shi, Development of Pomegranate Peel Extract and Nano ZnO Co-Reinforced Polylactic Acid Film for Active Food Packaging, Membranes, 2022, 12, 1108 CrossRef CAS PubMed.
- X. Ge, X. Huang, L. Zhou and Y. Wang, Essential oil-loaded antimicrobial and antioxidant zein/poly(lactic acid) film as active food packaging, Food Packag. Shelf Life, 2022, 34, 100977 CrossRef CAS.
- Y. Wu, X. Hao, F. Lin, S. Wang, L. Chen, X. Lin, D. Gan, S. Fan, L. Song and Y. Liu, Developing a cerium lactate antibacterial nucleating agent for multifunctional polylactic acid packaging film, Int. J. Biol. Macromol., 2022, 220, 56–66 CrossRef CAS PubMed.
- L. Behera, M. Mohanta and A. Thirugnanam, Intensification of yam-starch based biodegradable bioplastic film with bentonite for food packaging application, Environ. Technol. Innovation, 2022, 25, 102180 CrossRef CAS.
-
I. Clester, A. F. Blackwell, E. Cocker, G. Cox, A. McLean and T. Magnusson, Live Coding: A User's Manual, MIT Press, Cambridge, MA, 2022, DOI:10.7551/mitpress/13770.001.0001, ISBN: 9780262544818;
Organised Sound, 2023, 28, 315–318 Search PubMed.
- R. Poudel, N. Dutta and N. Karak, A mechanically robust biodegradable bioplastic of citric acid modified plasticized yam starch with anthocyanin as a fish spoilage auto-detecting smart film, Int. J. Biol. Macromol., 2023, 242, 125020 CrossRef CAS PubMed.
- S. F. Mirpoor, I. Corrado, R. Di Girolamo, G. Dal Poggetto, L. Panzella, E. Borselleca, C. Pezzella and C. V. L. Giosafatto, Manufacture of active multilayer films made of functionalized pectin coated by polyhydroxyalkanoates: A fully renewable approach to active food packaging, Polymer, 2023, 281, 126136 CrossRef CAS.
- L. Mao, J. Zuo, Y. Liu, B. Zheng, X. Dai, Z. Bai, Y. Liu and J. Yao, Alginate based films integrated with nitrogen-functionalized carbon dots and layered clay for active food packaging applications, Int. J. Biol. Macromol., 2023, 253, 126653 CrossRef CAS PubMed.
- G. I. B. Florentino, D. A. S. Lima, M. M. F. Santos, V. C. D. S. Ferreira, C. V. B. Grisi, M. S. Madruga and F. A. P. Da Silva, Characterization of a new food packaging material based on fish by-product proteins and passion fruit pectin, Food Packag. Shelf Life, 2022, 33, 100920 CrossRef CAS.
- O. Velgosová, S. Dolinská and A. Mražíková, Synergic effect of sodium alginate and Citrus lemon extract on the in-situ synthesis of AgNPs in a polymeric matrix, Nano-Struct. Nano-Objects, 2021, 28, 100795 CrossRef.
- S. Yousuf and S. S. Maktedar, Influence of quince seed mucilage-alginate composite hydrogel coatings on quality of fresh walnut kernels during refrigerated storage, J. Food Sci. Technol., 2022, 59, 4801–4811 CrossRef CAS PubMed.
- N. A. Peppas and A. R. Khare, Preparation, structure and diffusional behavior of hydrogels in controlled release, Adv. Drug Delivery Rev., 1993, 11, 1–35 CrossRef CAS.
- R. A. Batista, P. J. P. Espitia, J. d. S. S. Quintans, M. M. Freitas, M. Â. Cerqueira, J. A. Teixeira and J. C. Cardoso, Hydrogel as an alternative structure for food packaging systems, Carbohydr. Polym., 2019, 205, 106–116 CrossRef CAS PubMed.
- S. Yousuf and S. S. Maktedar, Utilization of quince (Cydonia oblonga) seeds for production of mucilage: functional, thermal and rheological characterization, Sustainable Food Technol., 2023, 1, 107–115 RSC.
- S. Ahmad, M. Ahmad, K. Manzoor, R. Purwar and S. Ikram, A review on latest innovations in natural gums based hydrogels: Preparations & applications, Int. J. Biol. Macromol., 2019, 136, 870–890 CrossRef CAS PubMed.
- H. M. C. Azeredo and K. W. Waldron, Crosslinking in polysaccharide and protein films and coatings for food contact – A review, Trends Food Sci. Technol., 2016, 52, 109–122 CrossRef CAS.
- J. George, C.-C. Hsu, L. T. B. Nguyen, H. Ye and Z. Cui, Neural tissue engineering with structured hydrogels in CNS models and therapies, Biotechnol. Adv., 2020, 42, 107370 CrossRef CAS PubMed.
- A. Ali and S. Ahmed, Recent Advances in Edible Polymer Based Hydrogels as a Sustainable Alternative to Conventional Polymers, J. Agric. Food Chem., 2018, 66, 6940–6967 CrossRef CAS PubMed.
- C. G. Otoni, P. J. P. Espitia, R. J. Avena-Bustillos and T. H. McHugh, Trends in antimicrobial food packaging systems: Emitting sachets and absorbent pads, Food Res. Int., 2016, 83, 60–73 CrossRef CAS.
- M. Shivani, S. Prathibha, B. Kavya Sri, A. D. Chintagunta, N. S. S. Kumar, S. P. J. Kumar, N. S. V. Kumar and V. R. Dirisala, Extraction of Natural Dye from and its Bougainvillea glabraApplications in Food Industries, Indian J. Ecol., 2020, 47, 207–211 Search PubMed.
- D. Alpaslan, Use of colorimetric hydrogel as an indicator for food packaging applications, Bull. Mater. Sci., 2019, 42, 247, DOI:10.1007/s12034-019-1908-z.
- P. Lu, R. Liu, X. Liu and M. Wu, Preparation of Self-supporting Bagasse Cellulose Nanofibrils Hydrogels Induced by Zinc Ions, Nanomaterials, 2018, 8, 800 CrossRef PubMed.
- L.-F. Wang and J.-W. Rhim, Preparation and application of agar/alginate/collagen
ternary blend functional food packaging films, Int. J. Biol. Macromol., 2015, 80, 460–468 CrossRef CAS PubMed.
- J. Yang, M. Shen, Y. Luo, T. Wu, X. Chen, Y. Wang and J. Xie, Advanced applications of chitosan-based hydrogels: From biosensors to intelligent food packaging system, Trends Food Sci. Technol., 2021, 110, 822–832 CrossRef CAS.
- S. Bodbodak and Z. Rafiee, Recent trends in active packaging in fruits and vegetables, Eco-Friendly Technol. Postharvest Prod. Qual., 2016, 77–125 Search PubMed.
- E. Benito-Peña, V. González-Vallejo, A. Rico-Yuste, L. Barbosa-Pereira, J. M. Cruz, A. Bilbao, C. Alvarez-Lorenzo and M. C. Moreno-Bondi, Molecularly imprinted hydrogels as functional active packaging materials, Food Chem., 2016, 190, 487–494 CrossRef PubMed.
- F. Croisier and C. Jérôme, Chitosan-based biomaterials for tissue engineering, Eur. Polym. J., 2013, 49, 780–792 CrossRef CAS.
- T. Kean and M. Thanou, Biodegradation, biodistribution and toxicity of chitosan, Adv. Drug Delivery Rev., 2010, 62, 3–11 CrossRef CAS PubMed.
- S. Baek, D. Kim, S. L. Jeon and J. Seo, Preparation and characterization of pH-responsive poly( N,N -dimethyl acrylamide- co -methacryloyl sulfadimethoxine) hydrogels for application as food freshness indicators, React. Funct. Polym., 2017, 120, 57–65 CrossRef CAS.
- A. Kwan and G. Davidov-Pardo, Controlled release of flavor oil nanoemulsions encapsulated in filled soluble hydrogels, Food Chem., 2018, 250, 46–53 CrossRef CAS PubMed.
- S. Park, S. Mun and Y.-R. Kim, Effect of xanthan gum on lipid digestion and bioaccessibility of β-carotene-loaded rice starch-based filled hydrogels, Food Res. Int., 2018, 105, 440–445 CrossRef CAS PubMed.
- M. Zhao, P. Wang, Y. Guo, L. Wang, F. Luo, B. Qiu, L. Guo, X. Su, Z. Lin and G. Chen, Detection of aflatoxin B1 in food samples based on target-responsive aptamer-cross-linked hydrogel using a handheld pH meter as readout, Talanta, 2018, 176, 34–39 CrossRef CAS PubMed.
- R. A. Batista, P. J. P. Espitia, J. d. S. S. Quintans, M. M. Freitas, M. Â. Cerqueira, J. A. Teixeira and J. C. Cardoso, Hydrogel as an alternative structure for food packaging systems, Carbohydr. Polym., 2019, 205, 106–116 CrossRef CAS PubMed.
-
M. R. Havstad, Biodegradable plastics, Plastic Waste and Recycling, 2020, pp. 97–129 Search PubMed.
- A. Krzan, S. Hemjinda, S. Miertus, A. Corti and E. Chiellini, Standardization and certification in the area of environmentally degradable plastics, Polym. Degrad. Stab., 2006, 91, 2819–2833 CrossRef CAS.
-
R. Müller, Biodegradability of Polymers: Regulations and Methods for Testing, Biopolymers Online, 2005, DOI:10.1002/3527600035.bpola012.
- C. Winotapun, A. Aontee, J. Inyai, B. Pinsuwan and W. Daud, Laser perforation of polyethylene terephthalate/polyethylene laminated film for fresh produce packaging application, Food Packag. Shelf Life, 2021, 28, 100677 CrossRef CAS.
- A. Khumkomgool, T. Saneluksana and N. Harnkarnsujarit, Active meat packaging from thermoplastic cassava starch containing sappan and cinnamon herbal extracts via LLDPE blown-film extrusion, Food Packag. Shelf Life, 2020, 26, 100557 CrossRef.
- A. S. Luyt and S. Gasmi, Influence of TiO2 Nanoparticles on the Crystallization Behaviour and Tensile Properties of Biodegradable PLA and PCL Nanocomposites, J. Polym. Environ., 2017, 26, 2410–2423 CrossRef.
- H. A. H. Ibrahim, S. Wefky, G. M. A. Elela, N. A. E. Sersy, D. E. Badan and A. Hassan, Bio-Surfactants: A Package Of Environmental And Industrial Benefits, Int. J. Recent Adv. Multidiscip. Res., 2018, 5, 4275–4291 Search PubMed.
- W. Zhang, Y. Zhang, J. Cao and W. Jiang, Improving the performance of edible food packaging films by using nanocellulose as an additive, Int. J. Biol. Macromol., 2021, 166, 288–296 CrossRef CAS PubMed.
- O. O. Oluwasina, B. P. Akinyele, S. J. Olusegun, O. O. Oluwasina and N. D. S. Mohallem, Evaluation of the effects of additives on the properties of starch-based bioplastic film, SN Appl. Sci., 2021, 3, 421, DOI:10.1007/s42452-021-04433-7.
- M. Zhang, G. M. Biesold, W. Choi, J. Yu, Y. Deng, C. Silvestre and Z. Lin, Recent advances in polymers and polymer composites for food packaging, Mater. Today, 2022, 53, 134–161 CrossRef CAS.
- F. Wu, M. Misra and A. K. Mohanty, Challenges and new opportunities on barrier performance of biodegradable polymers for sustainable packaging, Prog. Polym. Sci., 2021, 117, 101395 CrossRef CAS.
- D. C. M. Ferreira, G. Molina and F. M. Pelissari, Biodegradable trays based on cassava starch blended with agroindustrial residues, Composites, Part B, 2020, 183, 107682 CrossRef CAS.
- N. Burgos, I. Armentano, E. Fortunati, F. Dominici, F. Luzi, S. Fiori, F. Cristofaro, L. Visai, A. Jiménez and J. M. Kenny, Functional Properties of Plasticized Bio-Based Poly(Lactic Acid)_Poly(Hydroxybutyrate) (PLA_PHB) Films for Active Food Packaging, Food Bioprocess Technol., 2017, 10, 770–780 CrossRef CAS.
- K. Mazur and S. Kuciel, Mechanical and Hydrothermal Aging Behaviour of Polyhydroxybutyrate-Co-Valerate (PHBV) Composites Reinforced by Natural Fibres, Molecules, 2019, 24, 3538 CrossRef CAS PubMed.
- M. G. A. Vieira, M. A. da Silva, L. O. dos Santos and M. M. Beppu, Natural-based plasticizers and biopolymer films: A review, Eur. Polym. J., 2011, 47, 254–263 CrossRef CAS.
- A. E. Zeenat, D. A. Bukhari, S. Shamim and A. Rehman, Plastics degradation by microbes: A sustainable approach, J. King Saud Univ., Sci., 2021, 33, 101538 CrossRef.
-
M. Ramesh, G. Narendra, and S. Sasikanth, A Review on Biodegradable Packaging Materials in Extending the Shelf Life and Quality of Fresh Fruits and Vegetables, Waste Management as Economic Industry Towards Circular Economy, 2020, pp. 59–65, DOI:10.1007/978-981-15-1620-7_6.
- C. Turek and F. C. Stintzing, Stability of Essential Oils: A Review, Compr. Rev. Food Sci. Food Saf., 2013, 12, 40–53 CrossRef CAS.
-
E. A. Decker, R. J. Elias and D. J. McClements, Oxidation in Foods and Beverages and Antioxidant Applications, Elsevier, 2010 Search PubMed.
- R. Ribeiro-Santos, M. Andrade, A. Sanches-Silva and N. R. de Melo, Essential Oils for Food Application: Natural Substances with Established Biological Activities, Food Bioprocess Technol., 2017, 11, 43–71 CrossRef.
- Y. Jiang, D. Wang, F. Li, D. Li and Q. Huang, Cinnamon essential oil Pickering emulsion stabilized by zein-pectin composite nanoparticles: Characterization, antimicrobial effect and advantages in storage application, Int. J. Biol. Macromol., 2020, 148, 1280–1289 CrossRef CAS PubMed.
- H. Sun, S. Li, S. Chen, C. Wang, D. Liu and X. Li, Antibacterial and antioxidant activities of sodium starch octenylsuccinate-based Pickering emulsion films incorporated with cinnamon essential oil, Int. J. Biol. Macromol., 2020, 159, 696–703 CrossRef CAS PubMed.
- H. Almasi, S. Azizi and S. Amjadi, Development and characterization of pectin films activated by nanoemulsion and Pickering emulsion stabilized marjoram (Origanum majorana L.) essential oil, Food Hydrocolloids, 2020, 99, 105338 CrossRef CAS.
- Y. Xu, Y. Chu, X. Feng, C. Gao, D. Wu, W. Cheng, L. Meng, Y. Zhang and X. Tang, Effects of zein stabilized clove essential oil Pickering emulsion on the structure and properties of chitosan-based edible films, Int. J. Biol. Macromol., 2020, 156, 111–119 CrossRef CAS PubMed.
- S. Roy and J.-W. Rhim, Gelatin/agar-based functional film integrated with Pickering emulsion of clove essential oil stabilized with nanocellulose for active packaging applications, Colloids Surf., A, 2021, 627, 127220 CrossRef CAS.
- H. Lai, Y. Liu, G. Huang, Y. Chen, Y. Song, Y. Ma and P. Yue, Fabrication and antibacterial evaluation of peppermint oil-loaded composite microcapsules by chitosan-decorated silica nanoparticles stabilized Pickering emulsion templating, Int. J. Biol. Macromol., 2021, 183, 2314–2325 CrossRef CAS PubMed.
- S. Zhang, Z. He, F. Xu, Y. Cheng, G. I. N. Waterhouse, D. Sun-Waterhouse and P. Wu, Enhancing the performance of konjac glucomannan films through incorporating zein–pectin nanoparticle-stabilized oregano essential oil Pickering emulsions, Food Hydrocolloids, 2022, 124, 107222 CrossRef CAS.
- M. Wu, Z. Zhou, J. Yang, M. Zhang, F. Cai and P. Lu, ZnO nanoparticles stabilized oregano essential oil Pickering emulsion for functional cellulose nanofibrils packaging films with antimicrobial and antioxidant activity, Int. J. Biol. Macromol., 2021, 190, 433–440 CrossRef CAS PubMed.
- R. Krishnan, K. Mohan, K. V. Ragavan and P. Nisha, Insights into headway in essential oil-based Pickering emulsions for food applications, Sustainable Food Technol., 2023, 1, 363–376 RSC.
- S. Roy and J.-W. Rhim, Gelatin/agar-based functional film integrated with Pickering emulsion of clove essential oil stabilized with nanocellulose for active packaging applications, Colloids Surf., A, 2021, 627, 127220 CrossRef CAS.
- H. Sun, S. Li, S. Chen, C. Wang, D. Liu and X. Li, Antibacterial and antioxidant activities of sodium starch octenylsuccinate-based Pickering emulsion films incorporated with cinnamon essential oil, Int. J. Biol. Macromol., 2020, 159, 696–703 CrossRef CAS PubMed.
- Y. Zhou, S. Sun, W. Bei, M. R. Zahi, Q. Yuan and H. Liang, Preparation and antimicrobial activity of oregano essential oil Pickering emulsion stabilized by cellulose nanocrystals, Int. J. Biol. Macromol., 2018, 112, 7–13 CrossRef CAS PubMed.
- S. Hussain and S. S. Maktedar, Structural, functional and mechanical performance of advanced Graphene-based composite hydrogels, Results Chem., 2023, 6, 101029 CrossRef CAS.
- S. Priyanka, S. Karthick Raja Namasivayam, R. S. Arvind Bharani and A. John, Biocompatible green technology principles for the fabrication of food packaging material with noteworthy mechanical and antimicrobial properties – A sustainable developmental goal towards the effective, safe food preservation strategy, Chemosphere, 2023, 336, 139240 CrossRef CAS PubMed.
- L. K. Ncube, A. U. Ude, E. N. Ogunmuyiwa, R. Zulkifli and I. N. Beas, Environmental Impact of Food Packaging Materials: A Review of Contemporary Development from Conventional Plastics to Polylactic Acid Based Materials, Materials, 2020, 13, 4994 CrossRef CAS PubMed.
- K. Marsh and B. Bugusu, Food Packaging?Roles, Materials, and Environmental Issues, J. Food Sci., 2007, 72, R39–R55 CrossRef CAS PubMed.
- B. Chalermthai, P. Charoensuppanimit, K. Nootong, B. D. Olsen and S. Assabumrungrat, Techno-economic assessment of co-production of edible bioplastic and food supplements from Spirulina, Sci. Rep., 2023, 13, 10190, DOI:10.1038/s41598-023-37156-3.
-
L. Piergiovanni and S. Limbo, Chemical Features of Food Packaging Materials, SpringerBriefs in Molecular Science, 2015, pp. 69–75 Search PubMed.
- R. V. Sathianathan, K. Arockia Jayalatha, A. Sundararajan, S. Kannan, N. Paramasivam and S. Jothipandian, Intelligent Food Packaging and Shelf-Life Improvement of Chapattis Using Hybrid Nanoparticle-Based Biopolymer Electrospin Coating, ACS Food Sci. Technol., 2023, 3(4), 753–760, DOI:10.1021/acsfoodscitech.3c00012.
- B. M. Bharti, T. Bhuvana and C. Chandraprakash, Burst and Physicochemical Characteristics of Glycerol-Added Chitosan Films for Food Packaging, ACS Food Sci. Technol., 2023, 3(4), 772–780, DOI:10.1021/acsfoodscitech.3c00020.
- Y. Song, H. Zhang, H. Huang, Y. Zhang, H. Wang, Y. Li and C. Wang, Allicin-Loaded Electrospun PVP/PVB Nanofibrous Films with Superior Water Absorption and Water Stability for Antimicrobial Food Packaging, ACS Food Sci. Technol., 2022, 2(5), 941–950, DOI:10.1021/acsfoodscitech.2c00080.
- B. Hazer, Z. Karahaliloglu and R. D. Ashby, Poly(vinyl chloride) Derived Food Packaging Applications with Antioxidative and Anticancer Properties, ACS Food Sci. Technol., 2023, 3(4), 761–771, DOI:10.1021/acsfoodscitech.3c00021.
- Y. Amaregouda, K. Kamanna and A. Kamath, Multifunctional Bionanocomposite Films Based on Chitosan/Polyvinyl Alcohol with ZnO NPs and Carissa carandas Extract Anthocyanin for Smart Packaging Materials, ACS Food Sci. Technol., 2023, 3(9), 1411–1422, DOI:10.1021/acsfoodscitech.3c00065.
- A. Hadi, A. Nawab, F. Alam and S. Naqvi, Development of sodium alginate–aloe vera hydrogel films enriched with organic fibers: study of the physical, mechanical, and barrier properties for food-packaging applications, Sustainable Food Technol., 2023, 1(6), 863–873, 10.1039/d3fb00122a.
- G. Dalei, S. K. Das, S. S. Mohapatra and S. Das, In situ crosslinked Schiff base biohydrogels containing Carica papaya peel extract: application in the packaging of fresh berries, Sustainable Food Technol., 2023, 1(6), 906–920, 10.1039/d3fb00096f.
- T. Gasti, S. Dixit, R. B. Chougale and S. P. Masti, Fabrication of novel gallic acid functionalized chitosan/pullulan active bio-films for the preservation and shelf-life extension of green chillies, Sustainable Food Technol., 2023, 1(3), 390–403, 10.1039/d3fb00007a.
- P. Shan, K. Wang, F. Sun, Y. Li, L. Sun, H. Li and L. Peng, Humidity-adjustable functional gelatin hydrogel/ethyl cellulose bilayer films for active food packaging application, Food Chem., 2024, 439, 138202, DOI:10.1016/j.foodchem.2023.138202.
- C. Shen, Y. Ma, D. Wu, P. Liu, Y. He and K. Chen, Preparation of covalent organic framework-based nanofibrous films with temperature-responsive release of thymol for active food packaging, Food Chem., 2023, 410, 135460, DOI:10.1016/j.foodchem.2023.135460.
- L. Panzella and A. Napolitano, Condensed Tannins, a Viable Solution To Meet the Need for Sustainable and Effective Multifunctionality in Food Packaging: Structure, Sources, and Properties, J. Agric. Food Chem., 2022, 70, 751–758 CrossRef CAS PubMed.
- X. Wang, S. Xuan, K. Ding, P. Jin, Y. Zheng and Z. Wu, Photothermal controlled antibacterial Ta4C3Tx-AgNPs/nanocellulose bioplastic food packaging, Food Chem., 2024, 448, 139126 CrossRef CAS PubMed.
- M. R. Sharaby, E. A. Soliman, A. B. Abdel-Rahman, A. Osman and R. Khalil, Novel pectin-based nanocomposite film for active food packaging applications, Sci. Rep., 2022, 12, 20673, DOI:10.1038/s41598-022-25192-4.
Footnote |
† Both authors (SH and RA) contributed equally to this work. |
|
This journal is © The Royal Society of Chemistry 2024 |