DOI:
10.1039/D4FB00035H
(Review Article)
Sustainable Food Technol., 2024,
2, 1183-1205
Hemicelluloses from bioresidues and their applications in the food industry – towards an advanced bioeconomy and a sustainable global value chain of chemicals and materials
Received
31st January 2024
, Accepted 1st July 2024
First published on 2nd July 2024
Abstract
A major concern in the food industry is the use of non-renewable, petroleum-based materials and its detrimental impact on the environment. Consequently, there has been a growing interest in the use of biopolymers in food packaging and other applications due to their renewable origin and biodegradable properties, which have a positive environmental benefit. Hemicelluloses are biodegradable heteropolymers, which are associated with lignocellulose cell walls of vegetative and storage tissues of annual and perennial plants. They represent an immense renewable resource of biopolymers. Hemicelluloses are the second most abundant component of lignocellulosic biomass, and they are comparatively underutilized in industrial applications, even though it is a main by-product or residue in the lignocellulosic biomass processing. Therefore, it is important to include hemicellulose valorisation through the biorefinery concept to promote a Sustainable Bioeconomy (SBE), Circular Bioeconomy (CBE), and Circular Economy (CE). Extraction procedures on different plants have enabled the isolation of a diversity of hemicellulose structures with different yields and purities. However, compared to other biopolymers, their commercial uses have been underscored by their low yields, hydrophilicity, and low mechanical strength. While the applications of pure hemicelluloses are limited in the food industry, the use of hemicellulose composites as edible films, coatings, preservatives, fillers, and emulsifiers, is more promising. This review summarizes the current applications of plant hemicellulose biopolymers in the food industry and future perspectives in the advanced bioeconomy and value chain of chemicals and materials as well as ways of mitigating the challenges associated with their use.
Sustainability spotlight
The food industry generates up to a third of annual greenhouse gas (GHG) emissions worldwide, with food loss and waste accounting for half of these emissions. The valorization of hemicellulose in biorefineries from lignocellulosic biomass can help promote a circular bioeconomy and reduce GHG emissions. Hemicelluloses can be used in the food industry to create biodegradable packaging alternatives that can also help preserve the shelf life of food and reduce wastage. Hemicelluloses are also being explored in the manufacture of plant-based alternatives to meat, which can help reduce GHG emissions from the meat industry. These topics, which are aligned with the United Nations Sustainable Development Goal 12, are discussed in this review.
|
1. Introduction
The global food industry generates up to a third of global anthropogenic greenhouse gas (GHG) emissions annually, with half of these emissions resulting from food loss and waste (FLW).1 Consequently, the United Nations Sustainable Development Goal (UN SDG) 12 (Responsible Consumption and Production) seeks to mitigate this problem, with the aims of halving the global food waste per capita and use of more sustainable raw materials and promoting resource efficiency. Consequently, there has been several strategies that the food industry has been applying to combat this problem, which includes recycling food waste as raw materials, and manufacturing plant alternatives to reduce meat consumption. There has also been a paradigm shift towards vegan additives with low allergenicity compared to animal-based counterparts, that can be used as coatings, preservatives, and texture enhancers.
Additionally, in the food industry, most packaging and food additives are synthetic largely due to cost, convenience, and availability. The most popular food packaging materials, paper and plastic, are produced using largely unsustainable practices, namely logging and petroleum mining respectively. Packaging is the greatest contributor to waste worldwide with about 97% being unrecycled.2 While recycling attempts are increasing, they cannot counteract the growing demand for plastic.3 For single-use plastics (SUP), the working life, from use to disposal, can be as short as a few minutes. Some forms of plastic packaging release compounds that are hazardous to health when stored over time.4 About 95% of plastic packaging is single use but only about 5% is recycled.5
Within recent times, there has been a paradigm shift to safer, plant derived, biodegradable alternatives and research is exponentially growing. Plant based packaging has been gaining popularity in many first-world countries. For some bioplastics such as polylactic acid (PLA), while the source of raw materials (plants) is renewable, the material is not immediately biodegradable as this polymer is not naturally occurring within the plant.6 Therefore, utilizing the naturally occurring biopolymers found in plants can circumvent this, since they are naturally biodegradable. The lignocellulosic materials found in plants are the most abundant component of their biomass.7
Lignocellulosic biomass (LCB), derived not only from the food industry but from various plant-based sources such as agricultural residues, forestry wastes, and dedicated energy crops, has emerged as a promising renewable resource for the development of a sustainable bioeconomy. The integration of lignocellulosic biomass into the circular economy aligns with several other SDGs and paves the way for a resilient bioeconomy: (a) lignocellulosic biomass can be converted into biofuels, such as bioethanol, biodiesel, and biogas, contributing to the transition towards renewable energy sources and reducing dependence on fossil fuels (SDG 7 – Affordable and Clean Energy); (b) the development of lignocellulosic biorefineries promotes innovation in biomass processing technologies, resource efficiency, and the establishment of sustainable industrial practices (SDG 9 – Industry, Innovation, and Infrastructure); (c) the substitution of fossil-based products with bio-based alternatives derived from lignocellulosic biomass can contribute to the reduction of greenhouse gas emissions and mitigate the impacts of climate change (SDG 13 – Climate Action).
The integration of LCB into the circular economy through the development of lignocellulosic biorefineries supports the transition towards a sustainable bioeconomy. Lignocellulosic biorefineries aim to maximize the utilization of biomass by converting its components (cellulose, hemicellulose, and lignin) into a wide range of value-added products, including biofuels, biochemicals, and biomaterials. By valorizing renewable resources, reducing waste, and promoting resource efficiency, these biorefineries contribute to the achievement of several SDGs and pave the way for a more resilient and sustainable future.
Cellulose is the most abundant component of lignocellulose, followed by hemicellulose and lignin.7 The applications of cellulose in the food industry has been widely studied, while the potential of hemicelluloses is yet to be fully exploited. There is a diverse range of hemicelluloses available, with several applications in the food industry based on their properties. Despite the research that has been conducted, the mainstream commercialization of hemicelluloses in the food industry is forthcoming.8 This is due to several drawbacks associated with hemicelluloses that make them less competitive that conventional plastics. Therefore, this review summarizes the research on plant hemicellulose biopolymers and their applications in the food industry that has been conducted within the last decade (2014 to 2023), highlighting key gaps for future research.
2. Market for hemicellulose materials
The commercialization of products based on hemicellulose faces several hurdles. One major obstacle is the costly production process, arising from the complex extraction and purification procedures required to obtain pure hemicellulose. Another challenge is the lack of standardization in production, making it difficult to ensure consistent quality and quantity of hemicellulose. Additionally, the restricted availability of raw materials and competition with other industries utilizing lignocellulosic biomass further impede the commercialization of hemicellulose-based products (Qaseem et al., 2021; Rao et al., 2023; Sutay Kocabaş et al., 2020).9–11 There is a lack of a critical ecosystem of value-added processors of fruits and vegetables looking beyond the core product value and in some cases, the production of these raw waste materials is far away from processing facilities. Furthermore, the lack of awareness and understanding of these products among consumers and industries presents a challenge.12 There is a need for national policy to guide plant waste disposal to sustain hemicellulose demand and increased awareness of the use of these waste materials in industries other than biofuels. Finally, the development of efficient and cost-effective methods for extracting and fractionating hemicellulose from complex lignocellulosic biomass is imperative for the successful commercialization of hemicellulose-based products.13 There is a lack of availability of technical infrastructure, competent human pool, and indigenization of machinery for extraction of various phenolic and phytochemical based by-products.
There are several studies discussing the market for hemicellulose materials worldwide.14 Abejón (2018) analysed the research trends related to hemicellulose valorization from 2000 to 2016.14 The global annual production of hemicellulose is analysed in previous studies.10 Sutay Kocabaş et al. (2020) discussed global efforts to replace petroleum-based products with renewable biomass-based products through sustainable processes, with an emphasis on hemicellulose extraction and its potential applications.11
There are also some market reports available, such as the “Hemicellulose Market” research study for 2023, which delves deeply into market segmentation by types and applications, including the global sales and revenue forecast, regional forecast, and competitive landscape.15 This report provides both qualitative and quantitative insights into the market. It includes details on revenue distribution by application and type, along with a focus on key hemicellulose manufacturers. The report covers global market scope and segments, offering comprehensive data on market introduction, segmentations, status, and trends. There is a dearth of reliable information on the hemicellulose market trends for the food industry, and this should be the focus of future research. However, examining the patent literature can uncover various trends in the commercially viable food applications of hemicellulose.
In this literature review a bibliometric investigation was performed using Google Patents and Web of Science. Research and review articles published about hemicelluloses in the food industry from 2014-01-01 to 2023-12-31 were searched in the Web of Science database using the search terms ‘(“hemicellulose” or “xylan” or “glucan” or “mannan”) in the title and (“food” and “plant”) in any field’. The same search query was used in the Google Patents search. The search yielded 468 patents and 392 publication results. The trend in the number of patent applications and publications are shown in Fig. 1. While there has been an upward trend in the number of publications in hemicelluloses and food, there has been a general decline in the number of patents filed from 2016 onwards (Fig. 1a). In 2020 and 2023, the number of academic publications surpassed that of the number of patents filed, indicating that while hemicelluloses are an increasingly popular area of research, these research findings do not always translate into commercial applications. Therefore, this section describes the different applications in which hemicelluloses were used in recent patent applications, to better guide the future trajectories for research in this field.
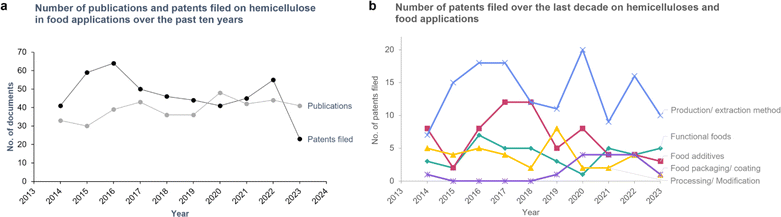 |
| Fig. 1 (a) Trends in the numbers of scholarly publications and patents filed with search terms ‘(“hemicellulose” or “xylan” or “glucan” or “mannan”) in the title and (“food” and “plant”) in any field’ during the period 2013-01-01 to 2023-12-31 (b) number of patents filed between 2014–2023 on the applications of plant hemicelluloses in the food industry. | |
Most patents filed in this area describe methods to extract hemicelluloses from lignocellulosic biomass or from plant storage polysaccharides (Fig. 1b). The second most popular category was food additives, which showed a decline over the years in the number of patents filed (Fig. 1b). Hemicelluloses have been used in various food products as emulsifiers, fibre additives, and texture enhancers. For example, one patent used Kojac Gluco Mannan (KGM) to enhance the texture of a plant-based ham alternative.16 Several of the patents filed incorporated hemicelluloses into functional food or food supplement formulations (capsules, tablets etc.) that are beneficial to human health. There has been a declining trend in patents filed for hemicellulose modifications (Fig. 1b). Interestingly, there were very few patents on hemicellulose food packaging/coatings, despite the growing body of research in this area (see Section 4.1). This suggests that there needs to be a stronger link between academic research and commercial endeavours. Some examples of patented food packaging are a xylan-based composite edible film17 and a hemicellulose-based plastic.18
3. Synergy of bioeconomy, biorefinery, and life cycle analysis (LCA) for the sustainable production of biodegradable chemicals and materials from hemicellulose
The synergy of bioeconomy, biorefinery, and Life Cycle Analysis (LCA) plays a crucial role in the sustainable production of biodegradable chemicals and materials from hemicellulose. Lignocellulosic biorefineries employ various processes to maximize the utilization of biomass, including pretreatment to enhance component accessibility, enzymatic hydrolysis to break down polysaccharides like hemicellulose into fermentable sugars, and fermentation of these sugars to yield valuable biochemicals and biodegradable materials.
Ongoing research efforts in lignocellulosic biorefineries focus on optimizing and integrating the various unit operations to improve process efficiency, reduce waste streams, and enhance overall economic viability. Key areas of technological advancement and challenges include developing cost-effective and environmentally friendly pretreatment methods, improving enzymatic hydrolysis through tailored enzyme cocktails and novel enzyme discovery, engineering microorganisms for efficient fermentation of various sugars, integrating advanced separation and purification technologies, and exploring novel valorization pathways for side streams and by-products. Furthermore, comprehensive techno-economic and Life Cycle Assessments (LCA) are essential for evaluating the economic feasibility, environmental impacts, and sustainability of lignocellulosic biorefineries at different scales, ensuring the sustainable production of biodegradable chemicals and materials from hemicellulose while contributing to the bioeconomy.
Despite the potential of hemicellulose for applications in the food industry, much of it is not utilized for value-added applications and is primarily incinerated to produce energy. However, recent research has focused on the development and optimization of hemicellulose extraction methods to increase its utilization in the bioeconomy and biorefinery concept.19 Enhancing specific critical parameters is crucial for the progress of commercializing these processes.20 A comprehensive assessment of the environmental sustainability of lignocellulose biorefineries is needed to create policies and regulatory frameworks that support the commercialization of these biorefineries.21 Life Cycle Assessment (LCA) provides a structured method for compiling energy and material input inventories, assessing environmental releases, and evaluating the overall environmental impact associated with products or processes. This assessment covers economic, social, and ecological considerations in different domains, such as from-cradle-to-grave (i.e. from raw materials to end-of-life), from-cradle-to-gate (from raw materials to marketable product), and from-gate-to-gate.22
Although, limited information about LCA studies, specifically regarding hemicellulose biorefineries, exist up to this date, there are some studies that provide a wide overview of the importance of biowastes valorization routes for the production, application and end-of-life scenarios of various chemicals and materials within a major biorefinery scope. The study by Ali et al. (2022) contributes to a comprehensive understanding of the life cycle of biodegradable plastics.23 They also review end-of-life scenarios (up-cycle and down-cycle) to attain the maximum environmental, social, and economic benefit from biodegradable bioplastic products under the biorefinery concept. The authors also discuss the challenges and prospects of bioplastics in transitioning towards a circular economy, emphasizing the significance of evaluating the environmental benefit over the entire life cycle. Similarly, Nessi et al. (2021) focused on the comparative LCA of alternative feedstock for plastic production, including recycled plastics and CO2, providing valuable insights into the environmental impact of different feedstock options.24 Gioia et al. (2021) evaluated three different end-of-life scenarios (composting, recycling, and upcycling) for biodegradable plastics in terms of literature review, contributing to a better understanding of the sustainability of these materials.25 Ali et al. (2023) discussed the state of the art in life cycle assessment (LCA) for bioplastic production, with a focus on bioplastics manufacturing, providing a comprehensive overview of the environmental impact of biodegradable chemicals and materials.26
A recent cradle-to-gate LCA study compared the sustainability of a first-generation biorefinery model that produces lignin, glucose, and hemicellulose, with that of a second-generation biorefinery model, which additionally produces ethanol and furfural derived from biopolymer degradation.27 Limited data availability was a constraint to the development of the model, and thus future studies are required to fully understand the environmental impact of biorefineries. They found that a broader spectrum of materials and chemicals being produced at a biorefinery can cause a balance between environmental sustainability and maximizing revenue, as long as the volume and the product type was reasonable.27
Several materials and chemicals derived from hemicellulose breakdown can be produced for commercialization purposes, which are briefly discussed here. These include xylooligosaccharides (XOs) and xylitol, which are valuable products obtained from hemicellulose. Additionally, hemicellulose can be utilized in the production of bioethanol, furan derivatives, and hydrophobic and processable derivatives, which can be used to prepare films and composites.28 Furthermore, hemicellulose can be employed in the production of various value-added biomaterials.29 Hemicellulose monomers can undergo various chemical transformations, such as dehydration or oxidation, to yield the desired chemical compounds.30,31 Pentoses and hexoses from hemicellulose play important roles as feedstocks for the production of various chemicals, including furfural,19 gamma-valerolactone (GVL), hydroxymethylfurfural (HMF), levulinic acid,32,33 and others.23,34
Furfural is a chemical manufactured in a lignocellulosic biorefinery from the acid-catalyzed dehydration of xylose, derived from hydrolyzing C5 polysaccharides found in biomass hemicellulose. Another platform chemical, HMF, can be used for the synthesis of a variety of value-added chemicals and materials. HMF is a precursor for the production of bio-based polymers, such as polyesters and polyurethanes and can be converted into 2,5-furandicarboxylic acid (FDCA), which is a key building block for bio-based plastics like polyethylene furanoate (PEF).35,36
Furfural serves as a chemical precursor for furfuryl alcohol and can be converted into various 5-membered oxygen-heterocycles, succinic acid, and levulinic acid.37 Furfural has widespread applications in the plastics, pharmaceutical, agrochemical, adhesive, and flavor enhancement industries. Succinic acid serves as a platform chemical and can be converted into succinate esters and precursors for various compounds. Dehydrogenative cyclization produces succinic anhydride, a key material for fumaric and maleic acid production.19 Levulinic acid is used in the production of plasticizers, pharmaceuticals, agrochemicals, and is a platform for the food industry primarily as a flavouring agent and food additive.32 It has also been found that levulinic acid has certain antimicrobial properties helping to extend the shelf life of certain food products.38 Levulinic acid is also a precursor for the synthesis of gamma-valerolactone (GVL), which is employed as a solvent and has applications in the production of biofuels.33
As a final example, Götz et al. (2023) conducted a comparative life cycle assessment (LCA) of simulated biorefineries producing 5-Hydroxymethylfurfural (HMF) from maize and miscanthus, representing first- and second-generation biomass.39 The objective was to evaluate the environmental impacts and process development suitability of these sources. The study aimed to understand the environmental consequences of HMF production from both biomass types. The findings revealed that, in general, the miscanthus-based biorefinery exhibited lower emissions across various impact categories compared to its maize-based counterpart, except for land occupation. The authors underscored HMF's potential as a versatile platform chemical for a bio-based chemical industry and emphasized the importance of LCA studies in guiding sustainable process development. This research provides valuable insights into the environmental performance of HMF biorefineries using different biomass sources, offering guidance for the advancement of sustainable biorefinery practices and the shift toward a bio-based chemical industry.39
The conclusions from these works highlight the importance of lignocellulosic residues, hemicellulose and its potential applications, as well as the role of sustainable processes, biowastes valorization, and life cycle assessment in the production and management of biodegradable chemicals and materials.
4. Current strategies and challenges in lignocellulosic biomass valorisation
4.1 From an oil/fossil-based society to a bio-based society
Due to the growing world population, there is intensive use of land and natural resources for food, commodities and energy production, that has delivered a standard of living never seen before in industrialized countries, but at the same time has affected environment and human health. The earth's natural balance has been altered and consequently, we can witness climate change, biodiversity loss, water shortage, famine and diseases. Modern society relies on petroleum and fossil resources that are finite and non-renewable, for energy, chemicals and materials; their use represents an environmental threat. Despite the efforts to decrease its consumption and use renewable resources, oil demand especially for energy for transportation continues to grow in 2024, according to the Deloitte report.40 Regarding global warming, the World Meteorological Organization (WMO) indicates that there is a 66% probability that the annual average near-surface global temperature between 2023 and 2027 will be more than 1.5 °C above pre-industrial levels.41 Biomass – including biodiversity, lignocellulosic biomass, and residual biomass is a sustainable alternative with economic and environmental advantages. Biomass wastes, including forest, agriculture, agro-industry and food wastes are increasing as the world population grows and the need of food, energy and commodities escalates. Biomass wastes have started to be seen as the resource for the next generation of biofuels, biochemicals and biomaterials. Bio-resources would reduce the dependency on oil and at the same time would have the benefit of good waste disposal, saving economic resources.42
4.2 Food supply chain waste (FSCW) valorisation
Food, water, sanitation and shelter are basic needs for human survival, however, low-income communities, especially in developing countries face difficulties when it comes to finding these minimum requirements.43 In contrast, there is a growth in nutrition-related diseases such as diabetes and obesity in middle and high-income countries.44 Studies highlight that these metabolic diseases are due to food processing that uses a great quantity of refined and energy-dense additives without nutritional value.45 Food processing industries are energy and water intensive and still inefficient, thus food supply chain waste (FSCW) is becoming a challenge for food security as well as for the development of a sustainable bioeconomy.45 One-third of global food produced is wasted in the food value chain, therefore there is an urgent need of changing behaviour towards the FSCW toward its valorisation and utilization, so that the carbon footprint throughout the FSC be a eco-friendly, more effective, and sustainable process.46 Efficient and advanced waste management disposal should focus on redesign, reuse, recovery, and recycling of food residues applying novel greener technologies.
5. Biomass cell wall structure and the role of hemicellulose in plant cell wall growth
Lignocellulosic biomass (LCB) is the most abundant material on earth. It is believed that 200 billion tons per year of vegetable biomass grow in our planet, but only 4–5% is utilized while the rest is recycled via natural pathways.47 The cell wall biomass cell is constituted by layers and sublayers: middle lamella, primary wall, and secondary wall, which is constituted by three sublayers such as secondary wall S1, S2 and S3 (Fig. 2). Chemically, lignocellulosic biomass consists of four biopolymers: cellulose (mostly in the primary wall and in the S2 sublayer); hemicellulose (mostly in the primary wall and secondary wall S3); lignin (mostly in the middle lamella and in the secondary wall S1); and pectin (mostly in the primary wall) (Fig. 2). These biopolymers are present in different proportions, associated each other in a matrix called lignocellulose; whose relative composition depends on type, species, and variety of the plant and even the age of the plant and the ecosystem where is grown and developed influence the biomass composition and structure. A fourth biopolymer, pectin, is also present in the primary cell wall.48 The relative abundance of these three biopolymers is a key factor in the quality of LCB and its utilization. Since the dawn of humankind, human beings have used LCB as material for housing, clothing, household goods, and as fuel to cook their foods and heat their homes. LCB utilization is based on the chemical composition, and the structure and ultrastructure of the LCB cell wall. Cellulose is a linear homopolymer formed by glucose monomer units, linked through β-1,4 glucan chains which make cellulose microfibrils and form a semicrystalline structure, hence, chemically cellulose is a polysaccharide. Hemicellulose is a non-cellulosic polysaccharide that consists of a β-1,4-linked chain having side chains that prevent the formation of crystalline structures. This makes hemicellulose an amorphous biopolymer that forms long chains which can associate with cellulose through hydrogen bonding, so that hemicellulose forms a strengthening network that holds microfibrils together at specific sites. It is also speculated that hemicelluloses act as plasticizers keeping microfibrils apart from each other as well as sites of expansion enzyme activity. The type of hemicellulose, branching and abundance is species and biomass maturity dependent. There is still intense research related to the role of hemicelluloses in plants, some of them having side/chain substitutions (methylation). Lignin, the third component of the cell wall, is an aromatic network that confers strength and hydrophobicity to the cell wall and poses a significant barrier to wall-degrading enzymes. Lignin biopolymer is formed by monolignols and are oxidatively polymerized by free radicals in the cell wall.49 However, recent studies have revised its biosynthetic pathway, lignin monomers and the flux regulation throughout the pathway, concluding that there is a multilevel regulation of the phenylpropanoid pathway. When pathway perturbations occur, several intermediates are formed and can be converted into multiple different derivatives; both intermediates and derivatives can be integrated into the lignin polymer or remain soluble and can affect physiological processes.50 LCB mainly consists of secondary cell walls, and therefore the amount of pectin present in the LCB is minor, however, their industrial importance has focused the attention of several researchers. Pectins are complex cross-linked polysaccharides that contain D-galacturonic acid. There are three types of pectin in plant cell walls: homogalacturonan (HG), rhamnogalacturonan-I (RG-I) and RG-II. In general, pectin in plant cell walls consists of c. 65% HG, 20–30% RG-I and 10% RG-II.49
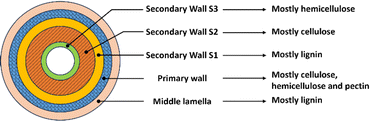 |
| Fig. 2 Scheme of the plant cell wall, its structure and general composition. | |
6. Applications and benefits of biorefineries in circular economy
6.1 Sustainable bioeconomy (SBE), circular bioeconomy (CBE) and circular engineering (CEng) – towards new product development (NPD) and an advanced bioeconomy (ABE)
Our modern society faces three big challenges: overpopulation, resource depletion and climate change; this causes pollution and greenhouse effect gases (GHG), therefore more food, more resources, and sustainable new sources to replace fossil fuels are needed. One of the most promising sources of carbon are renewable biological resources, namely biodiversity, biomass and residual biomass. The LCB and their residues are abundant and a suitable raw material for further processing and valorisation due to their richness in important chemical compounds such as low molecular weight compounds, most of them extractable bioactive phytochemicals; as well as high molecular weight compounds, which are structural and reserve biopolymers in the cell wall, described in the previous section. The LCB has been used for centuries; however, this renewable material has been underutilized. Hence, most LCB used as feedstock generates a great volume of wastes that until now are abandoned in the field or are buried in the landfills, not being part of the value chain of these industries currently. The challenge is to develop new clean technologies than can convert substantial amounts of biomass and residual biomass (RB) in a low cost and sustainable way, into commercially viable advanced biofuels, high value-added bioproducts and smart biomaterials suitable for the needs of our technological society favouring and advanced bioeconomy (ABE).51 This can be accomplished by applying the biorefinery concept and zero waste technology.52 Therefore, to develop a sustainable bioeconomy (SBE), one needs to consider the balance between a sustainable use of biological resources and the industrial use of biomass resources for multiple uses including bioenergy, food, feed, chemicals, fibres, polymers, plastics, and composites.51 Additionally, the waste generated in a LCB and RB processing can be integrated to the existing value chains through the development of new products, or novel sustainable processes can create new value chains stimulating a new product development (NPD). The NPD is beneficial since novel approaches and research are developed, boosting the Research + Development + Innovation (R + D + I) departments in academia and industrial settings. This promotes the circular bioeconomy (CBE) and enables the reduction of wastes in the environment as well as the efficient use of resources such as energy and water, laying the foundations for Circular Engineering (CEng). CEng would allow to not only take care of industry resources and improve their productivity and competitiveness, but also to reduce the waste and minimize greenhouse gases from the environment and ensure a clean environment, a growing industry and sustainable development.
6.2 Biorefineries, zero waste technology (ZWT)
The sustainable use of LCB and RB can be optimized applying the biorefinery concept and zero-waste technology, in which most of their valuable chemical components are extracted, isolated, purified, and/or functionalized (to enhance their physico-chemical properties), in a cascade way attempting to minimize residues in all stages of the process. These lignocellulose chemical compounds can be included in high value-added bio-products or biomaterials, as it is visualized in Fig. 3. This scheme depicts the integrated cascade biorefinery of LCB and RB in which the first step (1) is to extract the phytochemicals using green solvents such as deep eutectic solvents and then purified and characterized for suitable applications in pharmaceuticals, nutraceuticals, cosmeceuticals, foods, textiles, and so on. In a second step (2), the spent solid fraction can undergo a clean pretreatment, for instance a hydrothermal treatment combined with deep eutectic solvents. In the pretreatment, the LCB cell wall deconstruction leads to three different streams: a solid cellulose-rich fraction, and a liquid fraction rich in xylooligosaccharides and sugars coming from the hemicellulose biopolymer fractionation as well as lignin and phenolic compounds coming from the lignin biopolymer fractionation of the cell wall. Further processing of these fractions may lead to steps (3) lignins, nanolignins and their nanocomposites and advanced biomaterials, (4) hemicelluloses, xylooligosaccharides, advanced biomaterials, and sugars (monomers), and (5) cellulose, micro/nano fibrillated cellulose (MFC/NFC), advanced biomaterials, glucose (monomer) and biofuels.
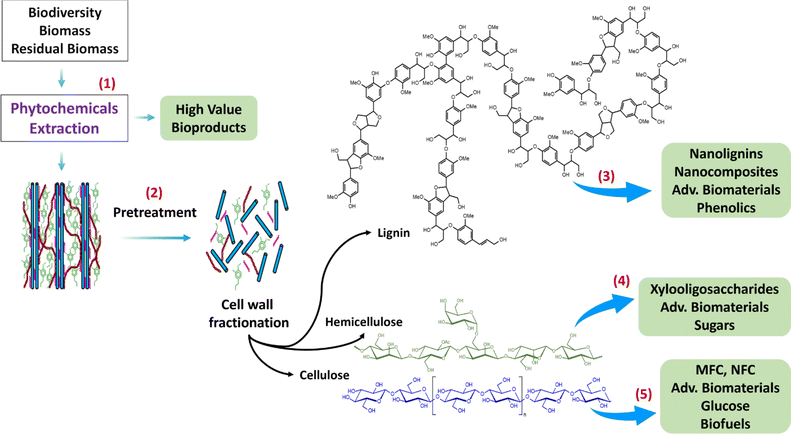 |
| Fig. 3 Integrated cascade biorefinery approach and zero waste biotechnology for LCB and RB. | |
6.3 Integrated cascade biorefinery of hemicellulose-rich fraction of LCB and RB
The valorisation of the hemicellulose-rich fraction from LCB and RB applying the biorefinery approach leads to the production of high value-added bioproducts and bioenergy, developing the bioeconomy, the circular bioeconomy and advanced bioeconomy. For this reason, the development of processes at pilot and industrial level for the fractionation of biomass, and the products applied to the food, materials, and energy sectors are a viable and sustainable alternative for the development of our society. This review emphasizes the valorisation of hemicellulose fraction, processing technology as hydrothermal processes in batch for extraction of hemicellulose, and high value-added products as xylooligosaccharides, xyloglucan, mannan, xylitol, mannitol, biofilms, coatings, and other applications described in the following sections.
6.4 Incorporation of bioproducts from the valorization of LCB and RB in biorefineries with the circular economy (CE) – sustainable global value chain of chemicals and materials
The incorporation of circular economy with the bioresidues to the supply chain of chemical products is a necessary step to ensure the successful development of biorefineries and the commercial viability of the biochemicals and biomaterials produced applying the advanced bioeconomy approach. A recent study reveals that there are more than 350
000 chemicals distributed in 22 inventories worldwide; most of them come from fossil sources, especially from oil, and these chemicals are the major sources of pollution. In addition, most of these compounds are difficult to recycle.53 On the other hand, there are 70
000 of these chemicals classified as “complex biological materials” of unknown or variable composition, which need to be classified to facilitate simplification of their sustainable circular use for their rational management in the medium and long terms.54 Hence, the production of biofuels, bioproducts and biomaterials is an alternative for an urgently needed solution for an efficient management of existing fuels, chemicals and waste in the global market. The acceptance of new biofuels, bioproducts and biomaterials from cascade biorefineries (advanced bioeconomy) is necessary for their integration into the global chemical products supply chain. This could reduce and possibly eliminate environmental pollution due to chemicals, biochemicals and bioresidues.
The sustainable and effective use of bioresidues is an important factor to close the circle of the circular economy in the chemical industry, related industries and those that consume final or intermediate chemical products, which we find practically in all sectors. Advances in science and technology, especially those related to biomass and bioresidue pretreatments and the downstream processing in cascade biorefineries, allow bioresidues to be considered feedstocks for a wide range of chemical products, and biochemicals that can be distributed to the final consumer or to specialized companies that generate high value-added biomaterials.
7. Chemistry, types, and properties of plant hemicelluloses
7.1 Chemistry
Biopolymers are macromolecules which serve physiological roles either for support or storage.55 They range from liquid to semi-solid to solid which dictate their application. Hemicellulose is a heteropolymer of glucose and other sugars that comprise 20–35% of the plant cell wall (20–30% in dicots and softwoods; 20–35% in hardwoods; 40–50% in grasses) and is built from carbon, hydrogen and oxygen.56 Like cellulose, it contains β-glucose but has a diversity of other sugars as monomers including xylose, mannose, galactose, arabinose and glucuronic acid (Fig. 4).12,49
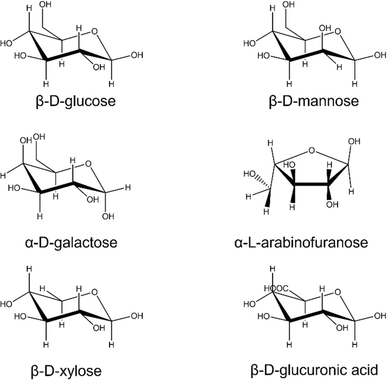 |
| Fig. 4 Chemical structures of the monomers that are used to build hemicelluloses. | |
Hemicellulose biopolymers (500–3000 monomer units) are much shorter than cellulose (7000–15,000 monomer units).57 These polymers have a diversity of chemical structures based on different permutations of the pyranose and furanose sugars (Fig. 3). However, there are repetitive blocks of monomers in the backbones of the four main types of hemicelluloses in plants: xylans, mannans, xyloglucans, and mixed linkage β-glucans (MLGs).9 The diversity of the side chains makes the structural elucidation challenging since hydrolysis can yield multiple polymeric products.57 Hemicellulose sugar ratios vary based on extraction method but also varies between different varieties, environmental conditions, stage of maturity.58
They are chemically bonded via a glycosidic bond to lignin and hydrogen bonded to cellulose.56 Hemicellulose can form hydrogen bonds with cellulose if the hydroxyl groups are in the axial position. Depending on the sugars constituting the backbone, there may be interruptions in the hydrogen bonding, making cellulose–cellulose bonds stronger than cellulose–hemicellulose bonds. While cellulose microfibrils impart strength to the cell walls, hemicellulose imparts flexibility. Apart from the variation in hydrogen bonding, there exists variation in branching, degree of acetylation, presence of carboxyl groups and presence of aldehyde groups. More polar groups can associate more strongly with cellulose while less polar groups like acetyl groups associate more strongly with lignin. More extensive 1 → 6 branching disrupts the hydrogen bonding and the branches are more susceptible to enzymatic cleavage and alkali and acid hydrolysis.59 Hemicelluloses are highly branched in nature and were initially regarded as amorphous but developments in extraction procedures have enabled isolation of nanocrystalline hemicellulose.10
7.2 Types of hemicelluloses
The structural characteristics of hemicelluloses have been recently reviewed.60 The four major classes of hemicelluloses can be subdivided into various subclasses, with some examples being listed in Fig. 5. A detailed overview of the chemical structures of different types of hemicelluloses has been reviewed previously, so only a brief overview is provided here.61 The chemical structures of some industrially important examples illustrated in Fig. 6 and 7.
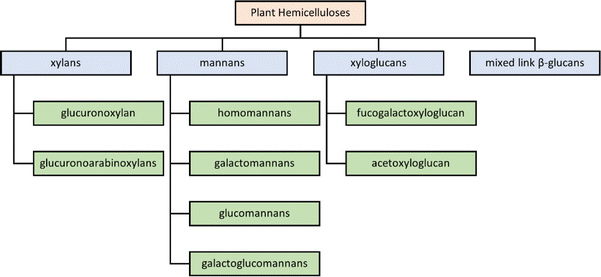 |
| Fig. 5 Classes of plant hemicelluloses, as described by Qaseem et al.9 | |
 |
| Fig. 6 (a) Chemical structure of xylan in hardwood (b) chemical structure of konjac glucomannan. | |
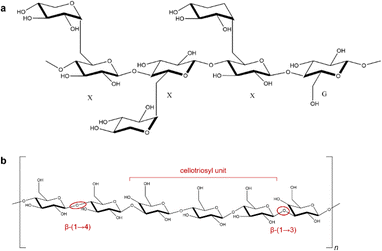 |
| Fig. 7 (a) Chemical structure of xyloglucan in tamarind seed (XXXG type) (b) chemical structure of mixed linkage β-(1 → 3) β-(1 → 4) glucans in oats. | |
7.2.1 Xylans.
Xylans usually consist of a backbone of β-(1 → 4)-linked xylose residues and can be classified into homoxylans and heteroxylans. Homoxylans have a backbone of D-xylopyranose residues linked by β(1 → 4) glycosidic linkages. Homoxylans mainly have structural functions. Heteroxylans such as glucuronoxylans, glucuronoarabinoxylans, and complex heteroxylans, have a backbone of D-xylopyranose and short carbohydrate branches comprising other sugars.
7.2.2 Mannans.
Mannans can be classified into galactomannans and glucomannans based on the presence of the hexoses in their chain. Galactomannans have only β-(1 → 4) linked D-mannopyranose residues in the backbone. Glucomannans have both β-(1 → 4) linked D-mannopyranose residues and β-(1 → 4) linked D-glucopyranose residues in the main backbone. The branches have β-(1 → 6) linked D-galactopyranose residues for both types.
7.2.3 Xyloglucans.
Xyloglucans have a backbone similar to cellulose comprising β(1 → 4)-linked D-glucose with three out of four glucose residues substituted with α-D-xylose at position 6. There are different types of side chains: G – unbranched Glc residue; X – α-D-Xyl-(1 → 6)-Glc; L – β-Gal; S – α-L-Araf; F – α-L-Fuc. The two most common types of xyloglucans in plant cell walls are identified as XXXG and XXGG (X = xylopyranose; G = glucopyranose).
7.2.4 Mixed linkage β-glucans.
Mixed linkage β-glucans has strings of consecutive β-(1 → 4) D-glucopyranose residues separated by a single β-(1 → 3) D-glucopyranose. The relative abundances of β-(1 → 4) and β-(1 → 3) glucopyranoses are about 70% and 30%. These glucans primarily consist of cellotriosyl and cellotetrasyl segments in random order.61
7.3 Properties of hemicelluloses for food applications
The food industry actively seeks new ingredients and natural additives to use in food and enhance food properties such as texture, stability, solubility and emulsifying properties. The properties of hemicelluloses change depending on the tissue, (e.g. fruits, stems, roots and seeds), class of plant (monocotyledon, dicotyledon, softwood, hardwood), environmental conditions (e.g. temperature, season), and chemical structure. The type of monomer, substitution, and branching (e.g. arabinose, gluconic acids and galacturonic acids in xylans, or galactose in mannans) also affect the physicochemical properties.56 The physicochemical properties of arabinoxylans from various sources62 and O-acetyl-glucomannans from various Amorphophallus species have previously been reviewed.63
Solubility is an important property of hemicelluloses that can be tailored for different food applications. The longer the polymer chains are, the lower the water solubility.64 High molecular weight hemicelluloses can form gels, with rheological properties of high viscosity and elasticity. The degree of branching also impacts solubility, with the solubility increasing when the polysaccharide is more highly branched.62 The types of substituents also affect solubility, since hemicelluloses with the same degree of branching, but with different substituents, will have different properties.62 Thirdly, the orientation of the substituents will impact solubility, since removing arabinose moieties from a disubstituted arabinoxylan chain does not greatly impact solubility.62
The rheology and viscosity of hemicellulose biopolymer solutions also influences their applications. Chain flexibility is a major factor affecting the biopolymer rheology, since the less flexible the chain, the more viscous the solution. The degree of acetylation, temperature, and the average molecular weight affect the gelation process. For example, glucomannan is hydrophilic and has the capacity to form gels due to the self-association of glucomannan's acidic residues.65 Temperature can induce conformational transitions in some polysaccharides, which affects the flexibility, charge density, and size of the chain, which in turn will affect viscosity.66 Glucomannan can react with other polysaccharides to form thermo-irreversible stable gels, which could be use as gelling and thickening agents, which has industrial potential as emulsion stabilizers in food products.64,67 Hemicelluloses with less galactose residues can absorb more water in a stable viscosity range, forming colloidal suspensions better than sodium alginate, carboxymethyl cellulose (CM) or pectin.56,68 The ability of the hemicellulose to form hydrogen bonds with water results in a high water activity, in addition to preventing ice crystals forming in frozen products, making it a good stabilizing agent. Hydration and viscosity range depends on the operation conditions such as pH, temperature, and concentration of solutes.69 Galactoglucomannan and glucoxylans do not display high viscosity and has the capacity to reduce the surface tension of water.69 These properties of hemicelluloses that determine their assembly at liquid–liquid interfaces gives them emulsifying properties that can be exploited in oil–water emulsions in various foods such as yogurts, dressings and deserts.56,69,70
The thermal and optical properties of various plant hemicelluloses were recently investigated, and they were found to have a glass transition temperature from 250–300 °C which indicates that they are thermally stable. The hemicelluloses were also transparent, indicating that they are suitable for making packaging material.71 However, the high number of free hydroxyl groups in hemicelluloses make them hygroscopic and some hemicelluloses, such as xylans, are brittle.58 Despite this, hemicelluloses have excellent properties, such as biodegradability, biocompatibility, and bioactivity, which enable it to be applied in various industries, including the food industry.9
8. Extraction and processing methods of hemicelluloses for industrial uses
There are several ways to obtain hemicelluloses, namely, the extraction of hemicelluloses from lignocellulosic waste or biowaste, the polymerization of bio-based monomers, and microbial fermentation.
8.1 Extraction of hemicellulose polymers from bioresidues
The extraction of hemicellulose from lignocellulosic biomass has been reviewed recently, and is only briefly described here, emphasizing recent developments in this area.30,72 Additionally, the extraction of glucomannans from various plant sources have been extensively reviewed.73 The extraction of xyloglucan from tamarind seed (which is an industrial waste) for its use in the food industry has also been previously reviewed.74 Depending on the extraction conditions, they can be isolated as polymers, oligomers, or monomers of differing molecular masses.
Table 1 shows some examples extraction procedures for high molecular weight and therefore high authenticity hemicelluloses that would be useful for industrial applications. There are three main categories of hemicellulose extraction methods. The first method is physical pretreatment, where the hemicellulose is obtained via hydrothermal extraction (or autohydrolysis) or steam explosion.30 The second method is chemical pretreatment, which includes alkaline extraction, acid extraction, or solvent pretreatment (e.g. organosolv extraction, high pressure CO2/O2 technology, ionic liquid extraction, deep-eutectic solvent extraction).30 The last major category is combined pretreatment, which includes physical–chemical pretreatment (e.g. ultrasonic-assisted extraction, microwave-assisted extraction), a combination of chemical and hydrothermal processes (e.g. alkali-assisted hydrothermal process, acid-assisted hydrothermal process), and other combined processes.30
Table 1 Examples of plant and bioresidues sources of hemicelluloses and extraction methods
Plant source |
Hemicellulose type |
Extraction conditions [extraction category] |
MW (kDa) |
Uses |
Reference |
Aloe vera
|
Acemannan |
Aqueous extraction/ethanol leaf : water ratio (1 : 3) at varying times (1–6 h) and temperatures (80–100 °C) |
1000–1600 |
Functional foods and pharmaceutical products |
Liu et al., 201975 |
Sugarcane (Saccharum officinarum) bagasse |
Xylan |
Ethanol, followed by 25% NaOH 120 °C [alkaline treatment] |
380 |
Food packaging bioplastic |
Jia et al., 202376 |
Konjac (Amorphophallus konjac) |
Glucomannan |
Aqueous extraction/ethanol at varying temperatures (35–95 °C) |
200–2000 |
Food additives |
Yang et al., 201777 |
Oat bran (Avena sativa) |
Mixed linked β-glucan |
1 N NaOH (1 : 50) 1 h, pH 6.5 HCl and CaCl2 (1 h), pH 4.5 HCl (15 min), 50% ethanol [alkali extraction] |
>2000 |
Food and pharmaceutical industries |
Mashewari et al., 201778 |
Spruce softwood (Picea spp.) |
Arabinoglucoronoxylan |
Soaked in 1 M NaOH (1 : 7) or water, followed by microwave-assisted extraction [microwave-assisted extraction] |
66 |
Various e.g. packaging |
Chadni et al., 201979 |
Tamarind seed (Tamarindus indica) |
Xyloglucan |
Aqueous extraction/95% ethanol at 50 °C (4 h) [hydrothermal extraction] |
770 |
Food additive – gelling agent |
Limsangouan et al., 201980 |
8.2 Polymerization of bio-based monomers
Hemicelluloses can be synthesized from its constituent monomers by chemical polymerization, enzymatic polymerization, and/or chemical synthesis.81 The synthesis of α-glucans, β-glucans, xyloglucans, xylans, arabinoxylans, α-mannans, and β-mannans have recently been reviewed.81 This method is less popular than the extraction methods described previously, since it is time-consuming, and in the case of enzymatic synthesis, the specificity of enzymes for a particular reaction limits the substrate scope.81 These disadvantages lead to poor scalability.81 This process has the advantage that there is batch-to-batch reproducibility, unlike with hemicellulose extraction, where the extraction method and hemicellulose source introduce variability into the final product.81 Due to the inherent disadvantages of this method, the rest of this review will focus on hemicelluloses obtained via extraction.81
8.3 Microbial fermentation
Glucans can be produced by microbial fermentation, where the microbes secrete exopolysaccharides under fermentation conditions. This production method has been reviewed previously.82,83 For example, scleroglucan is a β-1,3-β-1,6-glucan that is secreted by Sclerotium fungi under fermentation conditions.84 Various microbial exopolysaccharides, such as xanthan gum, curdlan, and gellan gum, are already used commercially in the food industry.85 However, this review focuses on plant-based hemicelluloses and thus will not be discussed further.
9. Overcoming the challenges associated with hemicellulose in the food industry
The physical and mechanical properties of hemicelluloses can be improved by enzymatic or chemical reactions with substituents to confer unique or advantageous properties. Additionally, various additives can be used to create hemicellulose composites that have superior qualities. These modifications have been summarized previously, so only recent examples from food industry applications are described here.86
9.1 Chemical modifications
The various chemical modifications to hemicelluloses that can improve their properties for applications in the food industry has been recently reviewed.10,88–90
9.1.1 Etherification.
Hemicellulose etherification reactions include: carboxymethylation, alkylation, benzylation, hydroxypropylation, and quaternization. These reactions modify the solubility and biodegradability of hemicelluloses.30
Carboxymethylation is an industrially attractive hemicellulose modification because it does not require extreme solvents or modification conditions.91 Carboxymethylated hemicellulose is more thermally stable than hemicellulose, with better film-forming and oxygen barrier properties, but the material is still hygroscopic, which limits its function as a packaging film.91 However, various carboxymethylated hemicelluloses have enhanced antibacterial and prebiotic properties, so that they may be better suited as food additives.92
Alkylation reactions use alkyl groups as the substituent. For example, methylation can improve the water solubility and thermal stability of hemicellulose.30 Benzylation improves the water resistance and hydrophobicity of hemicellulose, which can make benzylated hemicellulose suitable for making bioplastics.30 Hydroxypropylation can be used modify the amphiphilicity of hemicelluloses, which can improve their barrier properties and thermal stability.30 Quaternization has been used to create cationic or amphoteric hemicellulose derivatives.30 Cationic xylan derivatives that have enhanced antimicrobial and antioxidant activities.93
9.1.2 Esterification.
Hemicelluloses can be esterified by acetylation, sulfation, succinylation, oleolation, lauroylation, and fluorination. In these reactions, the hydroxyl groups react with a carboxylic acid or its derivatives to form an ester. The replacement of hydroxyl groups decreases the hydrogen bonding capacity of the polymer, thus making it more hydrophobic and increasing the flexibility of hemicellulose films.7
Acetylation of polysaccharides, such as mannans, can improve their physicochemical properties and bioactivity.94 Acetylation can improve the hydrophobicity, water solubility, and water absorbance of mannans and may also improve their antioxidant activity.94 A greater degree of acetylation in tamarind seed xyloglucan corresponded to better water solubility, thermal stability, solution transmittance, and zeta potential (a characteristic that affects emulsifying and film-forming properties) but decreased gelling potential.94 Acetylation of xylan improved its barrier and antimicrobial properties, making it more suitable for use in food packaging.95 Acetylated xylans can also reduce the biodegradation of bioplastic, which can be useful for creating bioplastic films for long-term storage.90 Sulfation has also been used to create anionic xylan derivatives with improved antioxidant and antibacterial activities.93
Succinylation is the process where acid anhydrides, such as long-chain acid anhydrides (dodecenyl succinic anhydride), are esterified with the hemicellulose. For example, succinylation of glucuronoxylans, arabinoxylan, or xylan improved their emulsifying capabilities and gave them surfactant-like properties.96–98 Oleoylation and lauroylation are the esterification of fatty acid chlorides to the hemicellulose hydroxyl groups.88 The long fatty acid chains impart hydrophobic properties to the hemicellulose polymer.88 Fluorination is used to improve the hydrophobicity, chemical resistance, and thermal stability of hemicellulose films.7
9.1.3 Oxidation.
The hemicellulose rings can be opened by periodate oxidation, which can improve oxygen permeability or be used as an intermediate step before other chemical modifications, such as amination.86 However, oxidation can lead to depolymerization, which could affect the mechanical properties.86
9.1.4 Reductive amination and amidation.
Alkyl aminated glucuronoxylan showed higher emulsifying properties with higher degrees of amination up to a certain point.99 Aminated hemicelluloses also have better amphiphilicity, which makes it a suitable reinforcement for films or coatings.87 Amidation reactions can improve the swelling stability of hemicellulose.87
9.1.5 Cross-linking/graft copolymerization.
Xylan/polyvinyl alcohol films that could be used in food packaging were cross-linked with 1,2,3,4-butane tetracarboxylic acid to improve their hydrophobicity, oxygen barrier and mechanical properties.100 Cross-linking brings adjacent hemicellulose chains closer, which improves its barrier function so that cross-linked hemicellulose films extended the shelf life of apples by reducing oxidation and dehydration.101 Graft copolymerization introduces functional groups into the hemicellulose network which decreases the extent of hydrogen bonding, and increases its hydrophobicity.7
9.2 Enzymatic modification
Various enzymes can be used to modify hemicellulose polysaccharide chains to customize or improve their properties, and these modifications have been described previously.10,86,102,103 Enzymatic modifications have some advantages over chemical modifications, such as the use of milder reaction conditions that are less prone to degrading the polysaccharide and the specificity or regioselectivity of the reactions. Exo-α-L-arabinofuranosidases, exo-α-glucuronidase, and endo-β-xylanase can cleave side chains or the polysaccharide backbone to shorten xylan chain length, which affects its solubility and barrier properties.102 The gelling or film formation properties of mannans can be modified exo-α-galactosidase, endo-β-mannanase, and galactose oxidase enzymes which modify the backbone, side chains, or adds carbonyl groups.102 Xyloglucans can have their solubility and gelling properties altered by exo-β-galactosidase, xyloglucanase, and xyloglucan endo-transglycosylase which cleaves the backbone or side chains or can add xyloglucan oligosaccharides to the polymer.102 A disadvantage of enzymatic modifications is that different enzymes are needed for different types of hemicellulose, and these enzymes are expensive.86
9.3 Additives/composites
9.3.1 Additives.
Various plasticizers, such as chitosan, carboxymethylcellulose, xylitol, sorbitol, or lignin, are added to hemicelluloses to improve their film-forming properties.104,105 For example, plasticizing with glycerol or other polyols at high temperatures have better tensile strength properties compared to the pure polymers.106 These reactions take place with low concentrations of the polymers (1–4%) at low temperatures (60 °C) and the films are also dried at low temperatures (25–35 °C) so it is not energy intensive.
9.3.2 Composites.
Composites are materials made with two or more components which together result in a material with beneficial properties. Reinforcing agents are added to composites to enhance the mechanical properties of hemicellulose films, examples of which are described in Section 7.1.7 In addition to creating uniform hemicellulose composites, some research has been conducted into creating hemicellulose composite films as bilayers or multilayers, where the various components of the film are casted layer by layer.107 The creation of chitosan–konjac glucomannan (KGM) bilayers led to lower water vapor permeability and swelling degree, but there was reduced mechanical strength and thermal stability.107 The creation of bilayers facilitates the combination of materials that have complementary characteristics (e.g. mechanical strength and antibacterial activity) but incompatible conditions for mechanical properties.108
9.4 Bio-nanocomposites
9.4.1 Films.
Films can be made of biopolymer composites interacting via secondary forces of attraction to form three-dimensional (3D) structures. The use of hemicellulose-based nanocomposite films in the food industry has recently been reviewed.109 The addition of nanofillers to the hemicellulose biopolymer can improve the functional properties of hemicellulose packaging films, such as mechanical strength, thermal stability, and water permeability.110 For example, the addition of gliadin/casein nanoparticles to KGM/carboxymethyl chitosan edible films significantly improved its mechanical strength, water barrier properties, thermal stability, and UV blocking ability and the resulting edible film extended grape shelf life.111
9.4.2 Emulsions.
Hemicelluloses can also be used to create nanoemulsions, typically where an emulsifier is used to stabilize an emulsion of two immiscible liquids – a hydrophobic phase consisting of droplets (20–200 nm diameter) suspended in a hydrophilic phase.112 Nanoemulsions can also facilitate the slow release of active compounds, which make them suitable for active packaging. Hydroxypropyl-β-cyclodextrin (HPCD)/lecithin emulsifier was used to stabilize cinnamon essential oil (which has antioxidant and antibacterial properties) in a KGM/pullulan film that extended the shelf life of cherries and Agaricus bisporus mushrooms.112 KGM has also been used in edible films formed from Pickering emulsions, which is where an emulsion is stabilized by solid colloidal particles.113–115
10. Advantages and disadvantages of using hemicelluloses in the food industry
Hemicelluloses are the second most abundant component of lignocellulosic biomass. Therefore, they are readily available and renewable raw materials that do not compete with the raw materials used in food production. They also are amenable to easy chemical modification due to the presence of several free hydroxy groups (Fig. 1) that can facilitate carboxymethylation, acylation, or cationic generation.104 The chemical modifications can alter its properties to make it more suitable for different applications.104 Hemicelluloses have film-forming properties, are biodegradable, and are biocompatible.104 Hemicelluloses also have low water solubility and oxygen barrier properties.116 Some hemicelluloses, such as glucomannan, have slight antioxidant properties and can facilitate the controlled release of active substances.117 Glucomannan is widely studied for food industry applications as it is a neutral, non-ionic biopolymer, and this imparts chemical inertness and stability to the polysaccharide which makes it suitable for food packaging applications, as it will not interfere with the food's stability.117 A demand from the food industry for hemicellulose from lignocellulosic waste could create jobs for farming communities and minimizes postharvest wastage by creating a downstream value chain. However, extensive research and development will be required to innovate and refine the new products and processes.
The relatively low molecular weight of hemicellulose compared to that of cellulose indicates that hemicellulose has lower mechanical strength and thermal stability.7 Additionally, hemicellulose composition is not uniform, but varies depending on the source and there is high heterogeneity of the mixture.116 The presence of several hydroxyl groups allows them to make several intramolecular and intermolecular hydrogen bonds, which make hemicelluloses semi-crystalline in nature, hygroscopic, and hydrophilic.104 Some hemicelluloses, such as glucomannan, have high molar masses, which can increases the difficulty in preparing food packaging films.117 Additionally, the extraction of hemicellulose from lignocellulosic biomass is more challenging than extracting other components, such as cellulose. These challenges have recently been reviewed.13
11. Applications of hemicelluloses in the food industry
11.1 Food packaging
Hemicelluloses and their composites have been studied for their applications in creating different types of food packaging. KGM has been extensively studied for its use in food packaging and has been reviewed previously, so only more recent KGM studies will be mentioned here.117–120
A composite of carboxymethyl xylan, chitosan, and graphene oxide was used to create food packaging films that exhibit antibacterial activity, excellent oxygen barrier properties and mechanical properties.121 Montmorillonite clay has been used as an additive in quaternized hemicellulose–chitosan composites to increase thermal stability, water vapor permeability, and oxygen permeability.104 The addition of aminopropyl polyhedral oligomeric silsesquioxane to carboxymethyl hemicellulose enhanced the water vapor barrier characteristics, tensile strength, and thermal performance of the resulting film which extended the shelf life of blueberries for a week longer than a commercial film.122 Hemicelluloses can also be used as an additive to films based on other biopolymers to improve their mechanical properties. For examples, KGM added up to 30% (w/w) to carrageenan derivatives improved the mechanical properties of the film, which made the film strong enough to store sesame oil.123 A trilayer film of KGM and tragacanth gum (inner layer), and ε-polylysine, chitosan, and tannic acid (outer layers) showed excellent mechanical and antibacterial properties and was moisture-proof, so that it could be used to store dry food products such as crackers.124 A cost-effective bioplastic was formulated from the simultaneous extraction of β-glucan and protein from barley.125 In addition to these examples of food packaging, there are three further subcategories in which hemicelluloses have been used.
11.1.1 Active packaging.
Active packaging is a term used to describe packaging which contains or is made of components which have been intentionally incorporated into or on either the packaging material itself or the packaging headspace (the space inside the packaging void of product) with the purpose of enhancing the package system. KGM composites have been used to create antibacterial, antifungal, and antioxidant active packaging.117,126 Antibacterial hemicellulose composites are fabricated with the addition of natural antimicrobial compounds (e.g. curcumin, gallic acid, essential oils, epigallocatechin-3-catechate [EGCG]) or synthetic compounds (silver nanoparticles, poly (diallydimethylammonium chloride)).117 For example, phlorotannin is a phenolic compound extracted from Sargassum seaweed that was incorporated into KGM/cellulose nanocrystal films to impart antioxidant and antibacterial properties to the film.127 Lycopene is natural antioxidant that when incorporated into KGM:sodium alginate active films, was able to prolong the shelf life of sweet cherries.128 Hemicellulose/pectin/nanocellulose biocomposites were fabricated to slowly release polyphenol antioxidants that can be used as an active packaging for fatty foods.129
11.1.2 Intelligent packaging.
Intelligent packaging is a system capable of sensing, detecting, recording, and communicating in order to facilitate the extension of shelf life, improve quality and enhance food quality. Most indicators make use of indicator chemicals which will change colour based on the pH of the gas detected. These are placed on frozen and chilled products to monitor changes in temperature as they may relate to shelf life, food quality and food safety. Anthocyanins and tea polyphenols were added to KGM–sodium alginate composite films to create pH-responsive intelligent packaging that show excellent performance in the preservation and freshness monitoring of milk.130 Elderberry anthocyanins were incorporated into KGM/chitosan bilayer films to create a pH-responsive edible film for use in cheese preservation.108 Galactomannan has also been investigated to create pH-responsive composite food packaging films.131 Various studies have also investigated different formulations of KGM-based pH-responsive films to monitor the freshness of stored seafood.132–134
11.1.3 Edible films and coatings.
Hemicelluloses are good candidates for edible films and coatings because most are non-toxic and contain non-allergic substances, easily manufactured, and provide semi-permeability to maintain the internal equilibrium of gases involved during anaerobic and aerobic respiration. They are neutral in taste so do not affect the nutritional and organoleptic properties. Edible films provide an alternative solution to prevent postharvest losses of produce by enabling their storage and preservation at room temperature. KGM has been widely studied for its incorporation into edible film composites.135–139 For example, KGM has been used to improve the compactness and brittleness of red pitaya peel films.136 Composites of KGM, carrageenan, and nano-SiO2 were used to create edible films that increased the shelf like of white mushrooms stored at 4 ± 1 °C.137 Another study evaluated the addition of Litsea cubeba essential oil to KGM edible films and found that this increased citrus fruit shelf life via the antibacterial properties of the essential oil.138 KGM and synbiotics (a mixture of prebiotics and probiotic bacteria) were used to create an edible film for bread buns that extended their shelf life.139 Galactomannans have also been used to create composite edible films.140,141
11.2 Food additives
Hemicelluloses are non-toxic, with some having Generally Regarded As Safe (GRAS) status.142 For example, hemicellulose extracts containing galactoglucomannan and glucuronoxylan from industrial wood byproducts were found to be non-toxic in an in vitro study, which supports the valorization of wood by-products in the food industry.143 Therefore, various hemicelluloses are currently in commercial use or being explored for their potential as various food additives. The uses of hemicelluloses as food additives in animal feed and as emulsifiers have been reviewed previously, so that our review expands on the additional uses of hemicelluloses in the food industry.56 Additionally, the use of mannans64 and arabinoxylan as an additive in the food industry has been reviewed.62,144,145
11.2.1 Preservatives.
The valorization of hemicelluloses as a potential preservative in cosmetics and food have previously been investigated, due to concerns about the negative effects of some of the preservatives that are currently available. Eucalyptus alkaline peroxide mechanical pulp (APMP) waste liquor contains hemicelluloses that were extracted and etherified to form carboxymethyl hemicellulose (CMHC).146 The CMHC was then esterified with p-hydroxybenzoic acid to form hemicellulose p-hydroxybenzoate (p-CMHC), a novel preservative.146 The p-CMHC has almost no cytotoxicity, and has excellent antibacterial, antioxidant, moisturizing, and hygroscopic properties which make it a suitable candidate for future research on its role as a preservative.146 The addition of Caesalpinia pulcherrima galactomannans and Tamarindus indica xyloglucans to frozen French bread dough caused improved stability and reduced freezing damage, which preserved the dough.147 Therefore, hemicelluloses have the potential to improve the shelf life of bakery items.
11.2.2 Nutrient enhancers.
Hemicelluloses have several nutraceutical properties that make them applicable as functional food additives. For example, there are several health benefits of glucomannan consumption which have been reviewed.148 Xylan was carboxymethylated to make it more resistant to breakdown in the digestive tract and was then tested for prebiotic activity.92 Carboxymethylated xylan showed prebiotic activity on Lactobacillus and Streptococcus probiotic strains, which shows potential for its use as a dietary supplement.92 An O-acetylated glucomannan isolated from the Chinese medicinal herb, Dendrobium catenatum, showed immunostimulatory effects in vitro and can possibly be used as an immunostimulating food additive.149 Acetyl-glucomannan from Dendrobium officinale exhibited immunomodulatory effects that warrant investigations into their incorporation into functional foods. De-acetylation of the acetyl-glucomannan compromised its immunomodulatory effects.150 An α-(1 → 4)-D-glucan isolated from stem lettuce peel waste showed immunostimulatory effects in vitro.151 Galactomannan obtained from spent coffee grounds showed prebiotic and antioxidant activities and increased the viscosity of a milkshake product.152
KGM has been consumed in Asian and European countries for its numerous health benefits, such as prebiotic activity, laxative effects, anti-obesity effects, anti-inflammatory properties, and anti-diabetic effects.153,154 KGM has high viscosity and hydration capacity which make it an excellent nutritional additive as a source of dietary fiber for satiety regulation and glucose control.155 While previous studies found that the incorporation of nutritional additives to KGM reduced its viscosity, Chen et al. were able to incorporate the polyphenol, dihydromyricetin (DMY), into KGM, leading to improved physicochemical activity.155
Hemicelluloses have also been investigated for their ability to help control post-prandial blood glucose levels, which would make them potential ingredients for dietary supplements targeted towards those looking for these health benefits or diabetics. A galactoglucan from Lanzhou lily (Lilium davidii var. unicolor Cotton) bulbs showed a hypoglycemic effect.156 A double-blind, randomized, controlled trial on healthy humans found that a Plant Fiber Extract (PFE) containing xylan and cellulose polysaccharides and oligosaccharides elicited comparatively low insulinemic and glycemic responses.157 Additionally, an arabinomannan from Anemarrhena asphodeloides Bge. showed anti-diabetic osteoporosis activity.158 High molecular weight hemicelluloses from black spruce sawdust mill waste were recently explored to assess its potential as high-fibre a functional food ingredient.159
11.2.3 Emulsifiers.
Hemicelluloses can be used to stabilize emulsifications and Pickering emulsions in the manufacturing of various food products, and has recently been reviewed.160–162 Microencapsulation is an emerging technology in the food industry that can be used to protect lipids, such as plant oils, from atmospheric oxidation that would otherwise decrease the oil's nutritional value and organoleptic properties.163 Emulsifiers are required for microencapsulation. Spruce galactoglucomannans (GGM) and birch glucuronoxylans (GX) show comparable efficiency to commercially used guar gum during the microencapsulation process.164 However, GGM and GX had a woody taste in sensory evaluations of yoghurt that prepared with these emulsifiers. In another study, GGM and GX were used to microencapsulate bilberry powder for use in functional foods to protect its bioactive compounds from atmospheric oxidation.165 The microencapsulation efficiency of 71–73% was comparable to that of gum Arabic (76%).165 Glucomannan obtained from the aqueous extract of the Acrocomia aculeata fruit pulp showed significant emulsifying properties.166 β-glucan can also be used in the encapsulation of elderberry extract as an alternative to gum Arabic.167
11.2.4 Thickening agents/texture enhancers.
Recent reviews have summarized how some galactomannans, such as guar gum,168 locust bean gum,169,170 and tara gum171 are already commercially used in the food industry as thickeners, binding agents, and stabilizers. Therefore, other hemicelluloses as thickeners/texture enhancers are described here.
Hemicelluloses also have the potential to replace fat as a binding agent in foods to reduce their calorie content or to improve the physicochemical properties of plant fats to create vegan analogs to animal fats.172–174 For example, glucomannan was found to be a suitable fat replacer in cookies made from dough contain cassava flour and soy protein.173 Glucomannan–coconut oil emulsions that were created using 3D printing may have potential to be a plant-based replacement to animal fats.175 Additionally, β-glucan improves the spreadability and texture of reduced-fat cream cheese.176
Hemicelluloses can be used as substitutes for ingredients in foods that certain persons choose to eliminate from their diet. There has been a recent trend in plant-based, vegan diets and a corresponding increase in plant-based alternatives to animal-based products. For example, Highland barley β-glucan (HBG) was used as a fat replacer in a plant-based cheese to investigate whether it improves the viscoelastic properties, textural properties, and melting behaviour, when compared to the current commercially available alternative, Violife.177 HBG caused improved stretchability of the plant-based cheese compared to the Violife product, but it was outperformed by Cheddar cheese.177 HBG (10%) did not cause any significant improvement in the melting properties compared to the Violife cheese, but it improved the texture (gumminess, hardness) to be comparable with Cheddar cheese.177 Glucomannan (1.5%) added to bread formulations improved the bread texture and delayed bread staling.178 Additionally, Porang (Amorphophallus muelleri) tuber glucomannan may be a promising plant-based alternative to gelatine.179 Plant protein
:
KGM (80
:
20) gels that were created using soy, pea, or peanut protein showed promising results that support their use in plant-based foods.180 One study found that soybean protein isolate:coconut oil:KGM composites could be an acceptable alternative to pork fat.181 β-glucan can fortify plant-based highland barley milk, with the added benefits of improving the physicochemical properties and stability of the milk.182,183 Tamarind seed galactoxyloglucan and KGM have been used to create oleogels (edible oil gels) that could be used as a fat replacer.184,185 Furthermore, honey locust (Gleditsia triacanthos) gum (which is composed of galactomannans) showed comparable results to guar gum when used as a natural texture enhancer in gluten-free bread.186 Additional studies on honey locust gum, which have recently been summarized, highlight its future potential in the food industry.187
The gelling properties of hemicelluloses make them ideal for stabilizing protein-based products. For example, hemicelluloses can stabilize the myofibrillar protein network in fish and animal meat. This allows them to be processed in a healthier manner with lower salt content or to improve the texture for consumption by elderly persons with dysphagia.188,189 This property also can be exploited in creating plant-based meat analogs, where hemicellulose-protein composites are used to mimic the texture of meat.190 For example, KGM was added to plant-based fishball (PFB) seafood alternatives and it was found that, by cross-linking with the soy protein in the PFB, there was enhanced texture and rheological properties of the product.191 Additionally, hemicelluloses can be used to formulate composite hydrogels that can be used as a delivery vehicle for nutrients, flavours, or bioactive compounds in functional foods. Sorghum arabinoxylan and soy protein isolate were used to fabricate functional edible hydrogels.192 Oat and cereal-based β-glucans are used as gelling agents to improve the texture of protein-based foods such as pea protein yogurt,193 walnut meal flour,194 quinoa milk,195 and plant-based egg yolks made from potato protein.196 The uses of cereal β-glucans as a gelling agent in protein-based foods have been summarized recently.197
12. Future vision in industry
Hemicellulose is an underutilized biopolymer of LCB in industry. However, with the increased push for zero waste and biorefineries, hemicellulose can be used in all aspects of the food production value chain from energy for plant operation to food additives to packaging. While other biopolymers have been used industrially at unprecedented rates for packaging, the literature suggests that the commercialization of hemicellulose-based products will continue to grow exponentially as novel food additives.
It is expected in the future that there will be advances in the hemicellulose extraction methods that can improve the commercial viability of hemicellulose products. Current methods of hemicellulose extraction require expensive purification steps for use in packaging applications.198 Therefore, hemicellulose packaging is less competitive that cellulose and starch packaging.198 An alternative to improving purification methods can be to consider using impure hemicellulose fractions (which may also contain cellulose and lignin) which may also have more nutritional value. For example, impure hemicellulose fractions from oat bran processing can form stable emulsions or suspensions with time, and these fractions are also rich in phytochemical compounds that confer additional health benefits.198 A disadvantage of using impure hemicellulose fractions as food additives is that it may not have the quantities of hemicellulose compounds that can confer health benefits, as stipulated by the U. S. Food and Drug Administration (FDA).
The current trend is that there will be a greater demand for LCB as feedstocks for various industrial processes, and a consequent increase in hemicellulose side stream fractions that can be valorized to contribute to the circular economy. Researchers have recently designed an improved process to obtain valuable, food-grade sugars such as D-mannose, D-galactose and D-glucose syrups from galactoglucomannan fractions.199 Their innnovative industrial process has wide applicability to a variety of hemicellulose side streams from biorefineries and has the potential to be quite profitable. This process can be coupled to already-existing industrial processes.199 Future developments like these are expected to boost the marketability and profitability of hemicellulose products in the future.
13. Conclusions
Biopolymers from underutilized, valorized, lignocellulosic biomass are attractive, biodegradable substitutes to synthetic polymers. The diversity of hemicellulose structures confers a wide range of functional properties, which in turn influence their practical applications. Extraction of high molecular weight hemicelluloses, from a variety of ubiquitous sources, were reviewed as stable, high-quality options for future research into new product development using circular engineering and zero waste technology. While de novo methods such as microbial fermentation and polymerization offer high-yield alternatives, extraction from bioresidues using the biorefinery concept is more sustainable and environmentally friendly. The low molecular weight, mechanical strength and hygroscopicity of hemicelluloses are the main impediments to their mainstream applications. These challenges have been addressed by chemical modifications, enzymatic modifications, addition of reinforcing agents and biocomposites. While the extra step is an additional cost to production, studies have demonstrated augmentation of their biochemical properties, such as increasing antimicrobial and antioxidant properties and stability. This has extended their applications in the food industry to preservatives and vegan food additives which can be stable over the shelf life of the product. The packaging applications of optimized hemicellulose formulations have extended from bioplastics to secondary packaging such as smart packaging and edible films and coatings. The trend in hemicellulose research in the food sector has moved toward food additives and packaging.
Despite the plethora of benefits and applications of hemicelluloses, there is a gap that needs to be filled to realize the full potential. Involvement of stakeholders at all levels, from farmers to decision makers, is essential to mobilize research and development to advance the circular economy and increase the market for value-added hemicellulose-based products in the food industry. An elastic assessment of the new infrastructure, method standardization and sustainable processes, balanced against potential green jobs and growing market share is needed to increase industry and consumer reception. Future commercialization efforts need to be supported with financial analyses to examine the feasibility and profitability of scaling up industrially. While there has been a plethora of studies that have investigated the applications of hemicelluloses in the food industry, there is a considerable lag in the commercialization of such technologies. Most studies have investigated the effectiveness of a single hemicellulose material or composite, as opposed to comparing several different types of hemicelluloses on different types of food products to determine a cost-effective, optimal formulation that is compatible with a wide range of applications. There are several published studies with different formulations available, but future studies should seek to develop commercially viable solutions that have a wider range of applications in the food industry.
Author contributions
Conceptualization: Lourdes M. Orejuela-Escobar. All authors contributed to the writing and reviewing of this manuscript.
Conflicts of interest
There are no conflicts to declare.
Acknowledgements
The authors would like to acknowledge the Hemispheric University Consortium (HUC), Universidad San Francisco de Quito (USFQ), Ecuador (Poligrant Hubi 17034) and The University of the West Indies (UWI), St. Augustine, Trinidad and Tobago for making this collaboration possible.
References
- J. Zhu, Z. Luo, T. Sun, W. Li, W. Zhou, X. Wang, X. Fei, H. Tong and K. Yin, Cradle-to-grave emissions from food loss and waste represent half of total greenhouse gas emissions from food systems, Nat. Food, 2023, 4, 247–256 CrossRef CAS PubMed.
- J.-G. Rosenboom, R. Langer and G. Traverso, Bioplastics for a circular economy, Nat. Rev. Mater., 2022, 7, 117–137 CrossRef PubMed.
-
New Greenpeace Report, https://www.greenpeace.org/usa/news/new-greenpeace-report-plastic-recycling-is-a-dead-end-street-year-after-year-plastic-recycling-declines-even-as-plastic-waste-increases/, accessed 4 January 2024.
- J. Muncke, Tackling the toxics in plastics packaging, PLoS Biol., 2021, 19, e3000961 CrossRef CAS PubMed.
-
W. Phillips, E. Thorne and C. Roopnarine, Economic implications of the ban on single-use plastics in the Caribbean, Digital Repository BETA Economic Comission for Latin America and The Caribbean (ECLAC), 2020, vol. 95, https://repositorio.cepal.org/entities/publication/8fccf1bc-87de-4615-8da2-f239917587ee Search PubMed.
- B. Xu, Y. Chen, J. He, S. Cao, J. Liu, R. Xue, F. Xin, X. Qian, J. Zhou, W. Dong and M. Jiang, New insights into the biodegradation of polylactic acid: from degradation to upcycling, Environ. Rev., 2022, 30, 30–38 CrossRef CAS.
- Y. Zhao, H. Sun, B. Yang and Y. Weng, Hemicellulose-Based Film: Potential Green Films for Food Packaging, Polymers, 2020, 12, 1775 CrossRef CAS PubMed.
- P. Nechita, R. Mirela and F. Ciolacu, Xylan Hemicellulose: A Renewable Material with Potential Properties for Food Packaging Applications, Sustainability, 2021, 13, 13504 CrossRef CAS.
- M. F. Qaseem, H. Shaheen and A.-M. Wu, Cell wall hemicellulose for sustainable industrial utilization, Renewable Sustainable Energy Rev., 2021, 144, 110996 CrossRef CAS.
- J. Rao, Z. Lv, G. Chen and F. Peng, Hemicellulose: Structure, chemical modification, and application, Prog. Polym. Sci., 2023, 140, 101675 CrossRef CAS.
- D. Sutay Kocabaş, M. Köle and S. Yağcı, Development and optimization of hemicellulose extraction bioprocess from poppy (Papaver somniferum L.) stalks assisted by instant controlled pressure drop (DIC) pretreatment, Biocatal. Agric. Biotechnol., 2020, 29, 101793 CrossRef.
- L.-Z. Huang, M.-G. Ma, X.-X. Ji, S.-E. Choi and C. Si, Recent Developments and Applications of Hemicellulose From Wheat Straw: A Review, Front. Bioeng. Biotechnol., 2021, 9, 1–14 Search PubMed.
- N. I. Wan Azelee, H. I. Mahdi, Y.-S. Cheng, N. Nordin, R. M. Illias, R. A. Rahman, S. M. Shaarani, P. Bhatt, S. Yadav, S. W. Chang, B. Ravindran and V. Ashokkumar, Biomass degradation: Challenges and strategies in extraction and fractionation of hemicellulose, Fuel, 2023, 339, 126982 CrossRef CAS.
- R. Abejón, A Bibliometric Study of Scientific Publications regarding Hemicellulose Valorization during the 2000–2016 Period: Identification of Alternatives and Hot Topics, ChemEngineering, 2018, 2, 7 CrossRef.
-
M. Phagare, Hemicellulose Market size was USD 1.5 billion in 2023!, https://www.cognitivemarketresearch.com/hemicellulose-market-report, accessed 27 January 2024.
-
HB, Lee, Method for manufacturing vegetable ham using mannan, Kr. Pat., KR20230174772A, 2023 Search PubMed.
-
Q. Miao, G. Liu, C. Zhang, M. Chen, L. Chen, and Y. Ni, Xylan-based composite preservative film and preparation method thereof, Cn. Pat., CN113896956A, 2022 Search PubMed.
-
F. Peng, S. Jia, and J. Rao, Preparation method of hemicellulose-based plastic, Cn. Pat., CN115466414A, 2022 Search PubMed.
- S. Takkellapati, T. Li and M. A. Gonzalez, An overview of biorefinery-derived platform chemicals from a cellulose and hemicellulose biorefinery, Clean Technol. Environ. Policy, 2018, 20, 1615–1630 CrossRef CAS PubMed.
- Z. Lin, M. Ierapetritou and V. Nikolakis, Phthalic anhydride production from hemicellulose solutions: Technoeconomic analysis and life cycle assessment, AIChE J., 2015, 61, 3708–3718 CrossRef CAS.
- N. Ryan and P. Yaseneva, A critical review of life cycle assessment studies of woody biomass conversion to sugars, Philos. Trans. R. Soc., A, 2021, 379, 20200335 CrossRef CAS PubMed.
- J.-G. Rosenboom, R. Langer and G. Traverso, Bioplastics for a circular economy, Nat. Rev. Mater., 2022, 7, 117–137 CrossRef PubMed.
- S. S. Ali, T. Elsamahy, E. A. Abdelkarim, R. Al-Tohamy, M. Kornaros, H. A. Ruiz, T. Zhao, F. Li and J. Sun, Biowastes for biodegradable bioplastics production and end-of-life scenarios in circular bioeconomy and biorefinery concept, Bioresour. Technol., 2022, 363, 127869 CrossRef CAS PubMed.
-
S. Nessi, T. Sinkko, C. Bulgheroni, P. Garcia-Gutierrez, J. Giuntoli, A. Konti, M. E. Sanye, D. Tonini, R. Pant, L. Marelli and F. Ardente, Life Cycle Assessment (LCA) of alternative feedstocks for plastics production, https://publications.jrc.ec.europa.eu/repository/handle/JRC125046, accessed 29 January 2024.
- C. Gioia, G. Giacobazzi, M. Vannini, G. Totaro, L. Sisti, M. Colonna, P. Marchese and A. Celli, End of Life of Biodegradable Plastics: Composting versus Re/Upcycling, ChemSusChem, 2021, 14, 4167–4175 CrossRef CAS PubMed.
- S. S. Ali, E. A. Abdelkarim, T. Elsamahy, R. Al-Tohamy, F. Li, M. Kornaros, A. Zuorro, D. Zhu and J. Sun, Bioplastic production in terms of life cycle assessment: A state-of-the-art review, Environ. Sci. Ecotechnology, 2023, 15, 100254 CrossRef CAS PubMed.
- S. Bello, C. Ríos, G. Feijoo and M. T. Moreira, Comparative evaluation of lignocellulosic biorefinery scenarios under a life-cycle assessment approach, Biofuels, Bioprod. Biorefin., 2018, 12, 1047–1064 CrossRef CAS.
-
P. de Oliveira Rodrigues, A. G. Corrêa, M. A. Baffi and D. Pasquini, in Handbook of Biomass, ed. S. Thomas, M. Hosur, D. Pasquini and C. Jose Chirayil, Springer Nature, Singapore, 2023, pp. 1–31 Search PubMed.
-
W. Farhat, Investigation of hemicellulose biomaterial approaches: the extraction and modification of hemicellulose and its use in value-added applications, Polymers, Université de Lyon; North Carolina State University, 2018 Search PubMed.
- Y. Lu, Q. He, G. Fan, Q. Cheng and G. Song, Extraction and modification of hemicellulose from lignocellulosic biomass: A review, Green Process. Synth., 2021, 10, 779–804 CrossRef CAS.
- N. Sobuś and I. Czekaj, Catalytic Transformation of Biomass-Derived Hemicellulose Sugars by the One-Pot Method into Oxalic, Lactic, and Levulinic Acids Using a Homogeneous H2SO4 Catalyst, Catalysts, 2023, 13, 349 CrossRef.
- A. Kumar, D. Z. Shende and K. L. Wasewar, Production of levulinic acid: A promising building block material for pharmaceutical and food industry, Mater. Today: Proc., 2020, 29, 790–793 CAS.
- A. Kumar, D. Z. Shende and K. L. Wasewar, Extractive separation of levulinic acid using natural and chemical solvents, Chem. Data Collect., 2020, 28, 100417 CrossRef CAS.
- M. M. Abe, M. C. Branciforti and M. Brienzo, Biodegradation of Hemicellulose-Cellulose-Starch-Based Bioplastics and Microbial Polyesters, Recycling, 2021, 6, 22 CrossRef.
- A. A. Rosatella, S. P. Simeonov, R. F. M. Frade and C. A. M. Afonso, 5-Hydroxymethylfurfural (HMF) as a building block platform: Biological properties, synthesis and synthetic applications, Green Chem., 2011, 13, 754–793 RSC.
- S. Maitra and V. Singh, A consolidated bioprocess
design to produce multiple high-value platform chemicals from lignocellulosic biomass and its technoeconomic feasibility, J. Cleaner Prod., 2022, 377, 134383 CrossRef CAS.
- G. Machado, S. Leon, F. Santos, R. Lourega, J. Dullius, M. Mollmann and P. Eichler, Literature Review on Furfural Production from Lignocellulosic Biomass, Nat. Resour., 2016, 7, 115–129 CAS.
- L. Zhao, M. Y. Zhao, C. P. Phey and H. Yang, Efficacy of low concentration acidic electrolysed water and levulinic acid combination on fresh organic lettuce (Lactuca sativa Var. Crispa L.) and its antimicrobial mechanism, Food Control, 2019, 101, 241–250 CrossRef CAS.
- M. Götz, J. Lask, I. Lewandowski and A. Kruse, Comparative LCA studies of simulated HMF biorefineries from maize and miscanthus as an example of first- and second-generation biomass as a tool for process development, GCB Bioenergy, 2023, 15, 1011–1029 CrossRef.
-
2024 oil and gas industry outlook, https://www2.deloitte.com/us/en/insights/industry/oil-and-gas/oil-and-gas-industry-outlook.html, accessed 23 January 2024.
-
G. Polya, WMO Warning: 1.5 Degree C Warming Breach Very Soon & With Increasing Frequency. Act Now!| Countercurrents, https://countercurrents.org/2023/05/wmo-warning-1-5-degree-c-warming-breach-very-soon-with-increasing-frequency-act-now/, accessed 23 January 2024.
- M. K. Awasthi, S. Sarsaiya, A. Patel, A. Juneja, R. P. Singh, B. Yan, S. K. Awasthi, A. Jain, T. Liu, Y. Duan, A. Pandey, Z. Zhang and M. J. Taherzadeh, Refining biomass residues for sustainable energy and bio-products: An assessment of technology, its importance, and strategic applications in circular bio-economy, Renewable Sustainable Energy Rev., 2020, 127, 109876 CrossRef.
- M. Balasubramanian, Climate change, famine, and low-income communities challenge Sustainable Development Goals, Lancet Planet. Health, 2018, 2, e421–e422 CrossRef PubMed.
- D. J. Magliano, L. Chen, R. M. Islam, B. Carstensen, E. W. Gregg, M. E. Pavkov, L. J. Andes, R. Balicer, M. Baviera, E. B. Dam, G. L. Booth, J. C. N. Chan, Y. X. Chua, S. Fosse-Edorh, S. Fuentes, H. L. Gulseth, R. Gurevicius, K. H. Ha, T. R. Hird, G. Jermendy, M. D. Khalangot, D. J. Kim, Z. Kiss, V. I. Kravchenko, M. Leventer-Roberts, C.-Y. Lin, A. O. Y. Luk, M. Mata-Cases, D. Mauricio, G. A. Nichols, M. M. Nielen, D. Pang, S. K. Paul, C. Pelletier, S. Pildava, A. Porath, S. H. Read, M. C. Roncaglioni, P. L.-D. Ruiz, M. Shestakova, O. Vikulova, K.-L. Wang, S. H. Wild, N. Yekutiel and J. E. Shaw, Trends in the incidence of diagnosed diabetes: a multicountry analysis of aggregate data from 22 million diagnoses in high-income and middle-income settings, Lancet Diabetes Endocrinol, 2021, 9, 203–211 CrossRef PubMed.
- A. J. van der Goot, P. J. M. Pelgrom, J. A. M. Berghout, M. E. J. Geerts, L. Jankowiak, N. A. Hardt, J. Keijer, M. A. I. Schutyser, C. V. Nikiforidis and R. M. Boom, Concepts for further sustainable production of foods, J. Food Eng., 2016, 168, 42–51 CrossRef.
-
A. S. Matharu, E. M. de Melo and J. A. Houghton, in Waste Biorefinery, ed. T. Bhaskar, A. Pandey, S. V. Mohan, D.-J. Lee and S. K. Khanal, Elsevier, 2018, pp. 219–236 Search PubMed.
- M. Mujtaba, L. Fernandes Fraceto, M. Fazeli, S. Mukherjee, S. M. Savassa, G. Araujo de Medeiros, A. do Espírito Santo Pereira, S. D. Mancini, J. Lipponen and F. Vilaplana, Lignocellulosic biomass from agricultural waste to the circular economy: a review with focus on biofuels, biocomposites and bioplastics, J. Cleaner Prod., 2023, 402, 136815 CrossRef CAS.
-
P. Bajpai, in Pretreatment of Lignocellulosic Biomass for Biofuel Production, ed. P. Bajpai, Springer, Singapore, 2016, pp. 7–12 Search PubMed.
- P. E. Marriott, L. D. Gómez and S. J. McQueen-Mason, Unlocking the potential of lignocellulosic biomass through plant science, New Phytol., 2016, 209, 1366–1381 CrossRef CAS PubMed.
- R. Vanholme, B. De Meester, J. Ralph and W. Boerjan, Lignin biosynthesis and its integration into metabolism, Curr. Opin. Biotechnol., 2019, 56, 230–239 CrossRef CAS PubMed.
-
S. Narasimhan, B. S. Srikanth and P. Poltronieri, in Biotransformation of Agricultural Waste and By-Products, ed. P. Poltronieri and O. F. D'Urso, Elsevier, 2016, pp. 49–67 Search PubMed.
- L. M. Orejuela-Escobar, A. C. Landázuri and B. Goodell, Second generation biorefining in Ecuador: Circular bioeconomy, zero waste technology, environment and sustainable development: The nexus, J. Bioresour. Bioprod., 2021, 6, 83–107 CrossRef.
- Z. Wang, G. W. Walker, D. C. G. Muir and K. Nagatani-Yoshida, Toward a Global Understanding of Chemical Pollution: A First Comprehensive Analysis of National and Regional Chemical Inventories, Environ. Sci. Technol., 2020, 54, 2575–2584 CrossRef CAS PubMed.
- A. Lai, A. M. Clark, B. I. Escher, M. Fernandez, L. R. McEwen, Z. Tian, Z. Wang and E. L. Schymanski, The Next Frontier of Environmental Unknowns: Substances of Unknown or Variable Composition, Complex Reaction Products, or Biological Materials (UVCBs), Environ. Sci. Technol., 2022, 56, 7448–7466 CrossRef CAS PubMed.
-
R. A. Pradhan, S. S. Rahman, A. Qureshi and A. Ullah, in Biopolymers and Their Industrial Applications, ed. S. Thomas, S. Gopi and A. Amalraj, Elsevier, 2021, pp. 281–303 Search PubMed.
- F. Abik, C. Palasingh, M. Bhattarai, S. Leivers, A. Ström, B. Westereng, K. S. Mikkonen and T. Nypelö, Potential of Wood Hemicelluloses and Their Derivates as Food Ingredients, J. Agric. Food Chem., 2023, 71, 2667–2683 CrossRef CAS PubMed.
- L. J. Gibson, The hierarchical structure and mechanics of plant materials, J. R. Soc., Interface, 2012, 9, 2749–2766 CrossRef CAS PubMed.
- N. M. L. Hansen and D. Plackett, Sustainable Films and Coatings from Hemicelluloses: A Review, Biomacromolecules, 2008, 9, 1493–1505 CrossRef CAS PubMed.
-
Historical Wood: Structure, Properties and Conservation, ed. M. Broda and C. Hill, MDPI – Multidisciplinary Digital Publishing Institute, 2022, pp. 1–305 Search PubMed.
- Y. Gao, M. Guo, D. Wang, D. Zhao and M. Wang, Advances in extraction, purification, structural characteristics and biological activities of hemicelluloses: A review, Int. J. Biol. Macromol., 2023, 225, 467–483 CrossRef CAS PubMed.
- H. V. Scheller and P. Ulvskov, Hemicelluloses, Annu. Rev. Plant Biol., 2010, 61, 263–289 CrossRef CAS PubMed.
- E. Zannini, Á. Bravo Núñez, A. W. Sahin and E. K. Arendt, Arabinoxylans as Functional Food Ingredients: A Review, Foods, 2022, 11, 1026 CrossRef CAS PubMed.
- X.-D. Shi, J.-Y. Yin, L.-J. Zhang, X.-J. Huang and S.-P. Nie, Studies on O-acetyl-glucomannans from Amorphophallus species: Comparison of physicochemical properties and primary structures, Food Hydrocolloids, 2019, 89, 503–511 CrossRef CAS.
- S. Singh, G. Singh and S. K. Arya, Mannans: An overview of properties and application in food products, Int. J. Biol. Macromol., 2018, 119, 79–95 CrossRef CAS PubMed.
- M. S. Izydorczyk and J. E. Dexter, Barley β-glucans and arabinoxylans: Molecular structure, physicochemical properties, and uses in food products–a Review, Food Res. Int., 2008, 41, 850–868 CrossRef CAS.
-
K. Nishinari, M. Takemasa, H. Zhang and R. Takahashi, in Comprehensive Glycoscience, Elsevier, 2007, pp. 613–652 Search PubMed.
- L. R. S. Moreira and E. X. F. Filho, An overview of mannan structure and mannan-degrading enzyme systems, Appl. Microbiol. Biotechnol., 2008, 79, 165–178 CrossRef CAS PubMed.
-
J. Milani and G. Maleki, in Food Industrial Processes - Methods and Equipment, ed. B. Valdez, InTech, 2012 Search PubMed.
- S. Kirjoranta, A. Knaapila, P. Kilpeläinen and K. S. Mikkonen, Sensory profile of hemicellulose-rich wood extracts in yogurt models, Cellulose, 2020, 27, 7607–7620 CrossRef CAS.
- W. Farhat, R. A. Venditti, M. Hubbe, M. Taha, F. Becquart and A. Ayoub, A Review of Water-Resistant Hemicellulose-Based Materials: Processing and Applications, ChemSusChem, 2017, 10, 305–323 CrossRef CAS PubMed.
- H. Abid, S. Maqsood Khan and S. Iqbal, A study on optical and thermal properties of natural polymer-based hemicellulose compounds, J. Biomater. Sci., Polym. Ed., 2021, 32, 1472–1488 CrossRef CAS PubMed.
-
E. M. Jincy and K. S. Femina, in Handbook of Biomass, ed. S. Thomas, M. Hosur, D. Pasquini and C. Jose Chirayil, Springer Nature, Singapore, 2023, pp. 1–32 Search PubMed.
- X.-D. Shi, J.-Y. Yin, S. W. Cui, Q. Wang, S.-Y. Wang and S.-P. Nie, Plant-derived glucomannans: Sources, preparation methods, structural features, and biological properties, Trends Food Sci. Technol., 2020, 99, 101–116 CrossRef CAS.
- P. Thivya, N. Bhanu Prakash Reddy and S. Vadakkepulppara Ramachandran Nair, Extraction of xyloglucan from tamarind industrial waste by different methods and their potential application in the food sector, Int. J. Food Sci. Technol., 2023, 58, 2014–2020 CrossRef CAS.
- C. Liu, Y. Cui, F. Pi, Y. Cheng, Y. Guo and H. Qian, Extraction, Purification, Structural Characteristics, Biological Activities and Pharmacological Applications of Acemannan, a Polysaccharide from Aloe vera: A Review, Molecules, 2019, 24, 1554 CrossRef CAS PubMed.
- S. Jia, Z. Lv, J. Rao, B. Lü, G. Chen, J. Bian, M. Li and F. Peng, Xylan Plastic, ACS Nano, 2023, 17, 13627–13637 CrossRef CAS PubMed.
- D. Yang, Y. Yuan, L. Wang, X. Wang, R. Mu, J. Pang, J. Xiao and Y. Zheng, A Review on Konjac Glucomannan Gels: Microstructure and Application, Int. J. Mol. Sci., 2017, 18, 2250 CrossRef PubMed.
- G. Maheshwari, S. Sowrirajan and B. Joseph, Extraction and Isolation of β-Glucan from Grain Sources—A Review, J. Food Sci., 2017, 82, 1535–1545 CrossRef CAS PubMed.
- M. Chadni, O. Bals, I. Ziegler-Devin, N. Brosse and N. Grimi, Microwave-assisted extraction of high-molecular-weight hemicelluloses from spruce wood, C. R. Chim., 2019, 22, 574–584 CrossRef CAS.
- N. Limsangouan, N. Milasing, M. Thongngam, P. Khuwijitjaru and W. Jittanit, Physical and chemical properties, antioxidant capacity, and total phenolic content of xyloglucan component in tamarind (Tamarindus indica) seed extracted using subcritical water, J. Food Process. Preserv., 2019, 43, e14146 CAS.
- G. Fittolani, T. Tyrikos-Ergas, D. Vargová, M. A. Chaube and M. Delbianco, Progress and challenges in the synthesis of sequence controlled polysaccharides, Beilstein J. Org. Chem., 2021, 17, 1981–2025 CrossRef CAS PubMed.
-
R. R. Philippini, S. E. Martiniano, J. C. dos Santos, S. S. da Silva and A. K. Chandel, in Bioprocessing for Biomolecules Production, John Wiley & Sons, Ltd, 2019, pp. 303–320 Search PubMed.
- Z. Chen, D. Ni, W. Zhang, T. Stressler and W. Mu, Lactic acid bacteria-derived α-glucans: From enzymatic synthesis to miscellaneous applications, Biotechnol. Adv., 2021, 47, 107708 CrossRef CAS PubMed.
- N. A. Castillo, A. L. Valdez and J. I. Fariña, Microbial production of scleroglucan and downstream processing, Front. Microbiol., 2015, 6 DOI:10.3389/fmicb.2015.01106.
- G. L. Utama, C. Dio, E. Lembong, Y. Cahyana and R. L. Balia, Microorganism-Based β-Glucan Production and their Potential as Antioxidant, Syst. Rev. Pharm., 2020, 10, 868–873 Search PubMed.
- Z. Li and X. Pan, Strategies to modify physicochemical properties of hemicelluloses from biorefinery and paper industry for packaging material, Rev. Environ. Sci. Biotechnol., 2018, 17, 47–69 CrossRef CAS.
-
X. Peng, F. Du and L. Zhong, in Sustainable Polymer Composites and Nanocomposites, ed. Inamuddin, S. Thomas, R. Kumar Mishra and A. M. Asiri, Springer International Publishing, Cham, 2019, pp. 1267–1322 Search PubMed.
- L. Hu, X. Fang, M. Du, F. Luo and S. Guo, Hemicellulose-Based Polymers Processing and Application, Am. J. Plant Sci., 2020, 11, 2066–2079 CrossRef CAS.
- P. Nechita, M. Roman (Iana Roman) and S. M. Năstac, Green Approaches on Modification of Xylan Hemicellulose to Enhance the Functional Properties for Food Packaging Materials—A Review, Polymers, 2023, 15, 2088 CrossRef CAS PubMed.
-
J. R. Martins, M. M. Abe and M. Brienzo, in Hemicellulose Biorefinery: A Sustainable Solution for Value Addition to Bio-Based Products and Bioenergy, ed. M. Brienzo, Springer Nature, Singapore, 2022, pp. 171–205 Search PubMed.
- W. Geng, R. A. Venditti, J. J. Pawlak, H. Chang, L. Pal and E. Ford, Carboxymethylation of hemicellulose isolated from poplar (Populus grandidentata) and its potential in water-soluble oxygen barrier films, Cellulose, 2020, 27, 3359–3377 CrossRef CAS.
- Y. Sun, Y. Guan, H. E. Khoo and X. Li, In vitro Assessment of Chemical and Pre-biotic Properties of Carboxymethylated Polysaccharides From Passiflora edulis Peel, Xylan, and Citrus Pectin, Front. Nutr., 2021, 8, 1–12 CAS.
- A. C. Fröhlich, G. C. Bazzo, H. K. Stulzer and A. L. Parize, Synthesis and physico-chemical characterization of quaternized and sulfated xylan-derivates with enhanced microbiological and antioxidant properties, Biocatal. Agric. Biotechnol., 2022, 43, 102416 CrossRef.
- H. Zhang, T. Zhao, Y. Wu, F. Xie, Z. Xiong, Z. Song, L. Ai and G. Wang, Acetylation modification improved the physicochemical properties of xyloglucan from tamarind seeds, Int. J. Biol. Macromol., 2022, 223, 193–201 CrossRef CAS PubMed.
- M. Roman (Iana-Roman), P. Nechita, M.-A. Vasile and A.-M. Cantaragiu Ceoromila, Barrier and Antimicrobial Properties of Coatings Based on Xylan Derivatives and Chitosan for Food Packaging Papers, Coatings, 2023, 13, 1761 CrossRef.
- Z. Hu, Z. Xiang, L. Wang, Y. Liu and P. Wang, Homogeneous esterification of glucuronoxylans and investigation of their emulsifying properties, Cellulose, 2023, 30, 6855–6867 CrossRef CAS.
- Z. Xiang and T. Runge, Emulsifying properties of succinylated arabinoxylan-protein gum produced from corn ethanol residuals, Food Hydrocolloids, 2016, 52, 423–430 CrossRef CAS.
- H. N. Cheng, A. Biswas, S. Kim, C. R. Alves and R. F. Furtado, Synthesis and Characterization of Hydrophobically Modified Xylans, Polymers, 2021, 13, 291 CrossRef CAS PubMed.
- Z. Hu, C. Wang, Z. Xiang and F. Lu, Amino-functionalized glucuronoxylan as an efficient bio-based emulsifier, Cellulose, 2021, 28, 3677–3689 CrossRef CAS.
- G. Liu, K. Shi, H. Sun, B. Yang and Y. Weng, Enhancing Hydrophobicity and Oxygen Barrier of Xylan/PVOH Composite Film by 1,2,3,4-Butane Tetracarboxylic Acid Crosslinking, Polymers, 2023, 15, 2811 CrossRef CAS PubMed.
- Y. Zhao, H. Sun, B. Yang, B. Fan, H. Zhang and Y. Weng, Enhancement of Mechanical and Barrier Property of Hemicellulose Film via Crosslinking with Sodium Trimetaphosphate, Polymers, 2021, 13, 927 CrossRef CAS PubMed.
- A. Martínez-Abad, A. C. Ruthes and F. Vilaplana, Enzymatic-assisted extraction and modification of lignocellulosic plant polysaccharides for packaging applications, J. Appl. Polym. Sci., 2016, 133(2) DOI:10.1002/app.42523 , Special issue: Bio-based Packaging.
-
D. Bueno, C. de Freitas and M. Brienzo, in Hemicellulose Biorefinery, A Sustainable Solution for Value Addition to Bio-Based Products and Bioenergy, ed. M. Brienzo, Springer Nature, Singapore, 2022, pp. 207–230 Search PubMed.
- G.-G. Chen, X.-M. Qi, Y. Guan, F. Peng, C.-L. Yao and R.-C. Sun, High Strength Hemicellulose-Based Nanocomposite Film for Food Packaging Applications, ACS Sustainable Chem. Eng., 2016, 4, 1985–1993 CrossRef CAS.
- M. Fajri, E. Julianti and J. Silalahi, Porang glucomannan based edible film with the addition of mangosteen peel extract, IOP Conf. Ser. Earth Environ. Sci., 2021, 782, 032103 CrossRef.
- F. M. Fakhouri, D. Costa, F. Yamashita, S. M. Martelli, R. C. Jesus, K. Alganer, F. P. Collares-Queiroz and L. H. Innocentini-Mei, Comparative study of processing methods for starch/gelatin films, Carbohydr. Polym., 2013, 95, 681–689 CrossRef CAS PubMed.
- Y. Chen, S. Wang, C. Yang, L. Zhang, Z. Li, S. Jiang, R. Bai, X. Ye and W. Ding, Chitosan/konjac glucomannan bilayer films: Physical, structural, and thermal properties, Int. J. Biol. Macromol., 2024, 257, 128660 CrossRef CAS PubMed.
- D. Zhang, G. Cao, N. Bu, L. Huang, H. Lin, R. Mu, J. Pang and L. Wang, Multi-functional konjac glucomannan/chitosan bilayer films reinforced with oregano essential oil loaded β-cyclodextrin and anthocyanins for cheese preservation, Int. J. Biol. Macromol., 2023, 244, 125365 CrossRef CAS PubMed.
- G. Liu, K. Shi and H. Sun, Research Progress in Hemicellulose-Based Nanocomposite Film as Food Packaging, Polymers, 2023, 15, 979 CrossRef CAS PubMed.
- C. Wu, Y. Li, Y. Du, L. Wang, C. Tong, Y. Hu, J. Pang and Z. Yan, Preparation and characterization of konjac glucomannan-based bionanocomposite film for active food packaging, Food Hydrocolloids, 2019, 89, 682–690 CrossRef CAS.
- N. Bu, N. Zhou, G. Cao, R. Mu, J. Pang, C. Ma and L. Wang, Konjac glucomannan/carboxymethyl chitosan film embedding gliadin/casein nanoparticles for grape preservation, Int. J. Biol. Macromol., 2023, 249, 126131 CrossRef CAS PubMed.
- F. Zhou, L. Yu, Y. Liu, Z. Zeng, C. Li, Z. Fang, B. Hu, H. Chen, C. Wang, S. Chen, H. Wu, W. Wu and Y. Liu, Effect of hydroxypropyl-β-cyclodextrin and lecithin co-stabilized nanoemulsions on the konjac glucomannan/pullulan film, Int. J. Biol. Macromol., 2023, 235, 123802 CrossRef CAS PubMed.
- S. Zhang, Z. He, F. Xu, Y. Cheng, G. I. N. Waterhouse, D. Sun-Waterhouse and P. Wu, Enhancing the performance of konjac glucomannan films through incorporating zein–pectin nanoparticle-stabilized oregano essential oil Pickering emulsions, Food Hydrocolloids, 2022, 124, 107222 CrossRef CAS.
- Y. Du, S. Zhang, L. Sheng, H. Ma, F. Xu, G. I. N. Waterhouse, D. Sun-Waterhouse and P. Wu, Food packaging films based on ionically crosslinked konjac glucomannan incorporating zein-pectin nanoparticle-stabilized corn germ oil-oregano oil Pickering emulsion, Food Chem., 2023, 429, 136874 CrossRef CAS PubMed.
- Y. Wang, X. Ni, M. Wen, S. Lou, W. Xiao and Z. Gao, Preparation of antioxidant konjac glucomannan-based films enriched with Ocimum gratissimum L. essential oil Pickering emulsion and its effect on walnuts preservation, Colloids Surf., A, 2023, 665, 131220 CrossRef CAS.
- E. I. Goksu, M. Karamanlioglu, U. Bakir, L. Yilmaz and U. Yilmazer, Production and Characterization of Films from Cotton Stalk Xylan, J. Agric. Food Chem., 2007, 55, 10685–10691 CrossRef CAS PubMed.
- Y. Ni, Y. Liu, W. Zhang, S. Shi, W. Zhu, R. Wang, L. Zhang, L. Chen, J. Sun, J. Pang and J. Wang, Advanced konjac glucomannan-based films in food packaging: Classification, preparation, formation mechanism and function, LWT-Food Sci. Technol, 2021, 152, 112338 CrossRef CAS.
- W. Zhang and J.-W. Rhim, Recent progress in konjac glucomannan-based active food packaging films and property enhancement strategies, Food Hydrocolloids, 2022, 128, 107572 CrossRef CAS.
- Y. Sun, X. Xu, Z. Wu, H. Zhou, X. Xie, Q. Zhang, R. Liu and J. Pang, Structure, Merits, Gel Formation, Gel Preparation and Functions of Konjac Glucomannan and Its Application in Aquatic Food Preservation, Foods, 2023, 12, 1215 CrossRef CAS PubMed.
-
K. Danalakoti, H. A. Avinashe and N. Dubey, in Natural Gums, ed. S. Ahmed and A. Ali, Elsevier, 2023, pp. 339–346 Search PubMed.
- J. Rao, Z. Lv, G. Chen, X. Hao, Y. Guan and F. Peng, Fabrication of flexible composite film based on xylan from pulping process for packaging application, Int. J. Biol. Macromol., 2021, 173, 285–292 CrossRef CAS PubMed.
- J. Li, W. Wang, H. Wu, F. Peng, H. Gao and Y. Guan, Preparation and characterization of hemicellulose films reinforced with amino polyhedral oligomeric silsesquioxane for biodegradable packaging, Int. J. Biol. Macromol., 2024, 254, 127795 CrossRef CAS PubMed.
- A. R. Ganesan, M. Shanmugam, P. Ilansuriyan, R. Anandhakumar and B. Balasubramanian, Composite film for edible oil packaging from carrageenan derivative and konjac glucomannan: Application and quality evaluation, Polym. Test., 2019, 78, 105936 CrossRef.
- R. Mu, N. Bu, Y. Yuan, J. Pang, C. Ma and L. Wang, Development of chitosan/konjac glucomannan/tragacanth gum tri-layer food packaging films incorporated with tannic acid and ε-polylysine based on mussel-inspired strategy, Int. J. Biol. Macromol., 2023, 242, 125100 CrossRef CAS PubMed.
- H. A. A. Razzaq, M. Pezzuto, G. Santagata, C. Silvestre, S. Cimmino, N. Larsen and D. Duraccio, Barley β-glucan-protein based bioplastic film with enhanced physicochemical properties for packaging, Food Hydrocolloids, 2016, 58, 276–283 CrossRef CAS.
- N. Duan, Q. Li, X. Meng, Z. Wang and S. Wu, Preparation and characterization of k-carrageenan/konjac glucomannan/TiO2 nanocomposite film with efficient anti-fungal activity and its application in strawberry preservation, Food Chem., 2021, 364, 130441 CrossRef CAS PubMed.
- Z. Wu, C. Tong, J. Zhang, J. Sun, H. Jiang, M. Duan, C. Wen, C. Wu and J. Pang, Investigation of the structural and physical properties, antioxidant and antimicrobial activity of konjac glucomannan/cellulose nanocrystal bionanocomposite films incorporated with phlorotannin from Sargassum, Int. J. Biol. Macromol., 2021, 192, 323–330 CrossRef CAS PubMed.
- Y. Zeng, Y. Wang, J. Tang, H. Zhang, J. Dai, S. Li, J. Yan, W. Qin and Y. Liu, Preparation of sodium alginate/konjac glucomannan active films containing lycopene microcapsules and the effects of these films on sweet cherry preservation, Int. J. Biol. Macromol., 2022, 215, 67–78 CrossRef CAS PubMed.
- L. R. Mugwagwa and F. A. Annie, Chimphango, Physicochemical properties and potential application of hemicellulose/pectin/nanocellulose biocomposites as active packaging for fatty foods, Food Packag. Shelf Life, 2022, 31, 100795 CrossRef CAS.
- S. Wang, R. Li, M. Han, D. Zhuang and J. Zhu, Intelligent active films of sodium
alginate and konjac glucomannan mixed by Lycium ruthenicum anthocyanins and tea polyphenols for milk preservation and freshness monitoring, Int. J. Biol. Macromol., 2023, 253, 126674 CrossRef CAS PubMed.
- E. Yuyu, Q. Qi, Z. Chang, J. Jiang, X. Yao, P. Li, F. Lei and K. Wang, Fabrication of pH-sensitive galactomannan/glycerol bio-composite films for food packaging applications, React. Funct. Polym., 2022, 181, 105465 CrossRef.
- Z. Bian, W. Xu, H. Zhang, M. Shi, X. Ji, S. Dong, C. Chen, G. Zhao, X. Zhuo, S. Komarneni, K. Zhang, Z. Ni and G. Hu, Simultaneously realizing enhancement of sensitivity for freshness monitoring and multinomial properties of carrageenan/konjac glucomannan/blueberry anthocyanin-based intelligent film by diatomite, Int. J. Biol. Macromol., 2023, 251, 126192 CrossRef CAS PubMed.
- G. Cao, N. Bu, T. Zeng, R. Sun, R. Mu, J. Pang and L. Wang, Development of pH-responsive konjac glucomannan/pullulan films incorporated with acai berry extract to monitor fish freshness, Int. J. Biol. Macromol., 2022, 219, 897–906 CrossRef CAS PubMed.
- P. You, L. Wang, N. Zhou, Y. Yang and J. Pang, A pH-intelligent response fish packaging film: Konjac glucomannan/carboxymethyl cellulose/blackcurrant anthocyanin antibacterial composite film, Int. J. Biol. Macromol., 2022, 204, 386–396 CrossRef CAS PubMed.
- W. Xu, Y. Jia, J. Wei, Y. Ning, H. Sun, L. Jiang, L. Chai, D. Luo, S. Cao and B. R. Shah, Characterization and antibacterial behavior of an edible konjac glucomannan/soluble black tea powder hybrid film with ultraviolet absorption, RSC Adv., 2022, 12, 32061–32069 RSC.
- W. D. R. Putri, R. A. Rahma, A. A. Wardana, Z. H. Wijayanti, M. Nur and A. Z. Mubarok, Optimizing Edible Film Production from Red Pitaya Peel Powder, Konjac Glucomannan and Kappa Carrageenan, J. Polym. Environ., 2023, 1–20 Search PubMed.
- R. Zhang, X. Wang, L. Li, M. Cheng and L. Zhang, Optimization of konjac glucomannan/carrageenan/nano-SiO2 coatings for extending the shelf-life of Agaricus bisporus, Int. J. Biol. Macromol., 2019, 122, 857–865 CrossRef CAS PubMed.
- S. Lou, X. Ni, W. Xiao, Y. Li and Z. Gao, Physical stability, microstructure and antimicrobial properties of konjac glucomannan coatings enriched with Litsea cubeba essential oil nanoemulsion and its effect on citruses preservation, Int. J. Biol. Macromol., 2024, 256, 128306 CrossRef CAS PubMed.
- P. Pruksarojanakul, C. Prakitchaiwattana, S. Settachaimongkon and C. Borompichaichartkul, Synbiotic edible film from konjac glucomannan composed of Lactobacillus casei-01® and Orafti®GR, and its application as coating on bread buns, J. Sci. Food Agric., 2020, 100, 2610–2617 CrossRef CAS PubMed.
- W. Liu, Z. Ling, C. Huang, C. Lai and Q. Yong, Investigation of galactomannan/deacetylated chitosan nanocomposite films and their anti-bacterial capabilities, Mater. Today Commun., 2022, 30, 103002 CrossRef CAS.
- A. González, G. N. Barrera, P. I. Galimberti, P. D. Ribotta and C. I. Alvarez Igarzabal, Development of edible films prepared by soy protein and the galactomannan fraction extracted from Gleditsia triacanthos (Fabaceae) seed, Food Hydrocolloids, 2019, 97, 105227 CrossRef.
- L. Pitkänen, M. Heinonen and K. S. Mikkonen, Safety considerations of plant polysaccharides for food use: a case study on phenolic-rich softwood galactoglucomannan extract, Food Funct., 2018, 9, 1931–1943 RSC.
- D. Granato, D. Reshamwala, R. Korpinen, L. Azevedo, M. A. Vieira do Carmo, T. M. Cruz, M. B. Marques, M. Wen, L. Zhang, V. Marjomäki and P. Kilpeläinen, From the forest to the plate – Hemicelluloses, galactoglucomannan, glucuronoxylan, and phenolic-rich extracts from unconventional sources as functional food ingredients, Food Chem., 2022, 381, 132284 CrossRef CAS PubMed.
- J. Pang, Y. Zhang, X. Tong, Y. Zhong, F. Kong, D. Li, X. Liu and Y. Qiao, Recent Developments in Molecular Characterization, Bioactivity, and Application of Arabinoxylans from Different Sources, Polymers, 2023, 15, 225 CrossRef CAS PubMed.
- F. J. Hernández-Pinto, J. D. Miranda-Medina, A. Natera-Maldonado, Ó. Vara-Aldama, M. P. Ortueta-Cabranes, J. A. Vázquez del Mercado-Pardiño, S. A. M. El-Aidie, S. A. Siddiqui and R. Castro-Muñoz, Arabinoxylans: A review on protocols for their recovery, functionalities and roles in food formulations, Int. J. Biol. Macromol., 2024, 259, 129309 CrossRef PubMed.
- T. Chen, H. Liu, S. Song, S. Qiang, Y. An, J. Li, J. Liu, B. Chen, L. Chen, F. Liu, R. Liu, X. Jiang and X. Liao, Synthesis and its biological activity of carboxymethyl hemicellulose p-hydroxybenzoate (P-CMHC), Carbohydr. Res., 2023, 534, 108972 CrossRef CAS PubMed.
- L. H. E. Mourão, F. R. da Silva Mendes, F. D. de Sousa, A. C. de Oliveira Monteiro Moreira, S. R. A. Medeiros, M. do Socorro Rocha Bastos, G. D. Saraiva, A. M. R. Teixeira and R. de Azevedo Moreira, Effect of Added Plant Hemicelluloses on the Stability of Frozen Bread Dough, J. Food Process. Preserv., 2023, 2023, e8893925 Search PubMed.
- R. Tester and F. Al-Ghazzewi, Glucomannans and nutrition, Food Hydrocolloids, 2017, 68, 246–254 CrossRef CAS.
- J. Liu, L. Yu, C. Wang, Y. Zhang, H. Xi, J. Si, L. Zhang and J. Yan, Preparation, Structural Features and in vitro Immunostimulatory Activity of a Glucomannan From Fresh Dendrobium catenatum Stems, Front. Nutr., 2022, 8, 823803 CrossRef PubMed.
- X. Guo, M. Yang, C. Wang, S. Nie, S. W. Cui and Q. Guo, Acetyl-glucomannan from Dendrobium officinale: Structural modification and immunomodulatory activities, Front. Nutr., 2022, 9, 1016961 CrossRef PubMed.
- X. Zou, M. Shen, J. Li, P. Sun, X. Zhong and K. Yang, Isolation, structure characterization and in vitro immune-enhancing activity of a glucan from the peels of stem lettuce (Lactuca sativa), J. Sci. Food Agric., 2023, 2097–2109 Search PubMed.
- A. Ray and R. S. Singhal, Hydrogel formulation based on galactomannan from residual spent coffee ground confers bioactivities and viscosifying properties in milkshake, Food Biosci., 2023, 55, 102958 CrossRef CAS.
- R. D. Devaraj, C. K. Reddy and B. Xu, Health-promoting effects of konjac glucomannan and its practical applications: A critical review, Int. J. Biol. Macromol., 2019, 126, 273–281 CrossRef CAS PubMed.
- Q. Du, J. Liu and Y. Ding, Recent progress in biological activities and health benefits of konjac glucomannan and its derivatives, Bioact. Carbohydr. Diet. Fibre, 2021, 26, 100270 CrossRef CAS.
- Y. Chen, X. Gao, B. Li and J. Tian, Konjac glucomannan-dihydromyricetin complex improves viscosity and hydration capacity of konjac glucomannan as well as the thermal stability of dihydromyricetin, Int. J. Biol. Macromol., 2023, 242, 124666 CrossRef CAS PubMed.
- H. Hui, H. Jin, X. Yang, X. Wang and B. Qin, Fine structure and hypoglycemic effect of a galactoglucan from the bulbs of Lanzhou lily, Int. J. Biol. Macromol., 2024, 254, 127774 CrossRef CAS PubMed.
- D. Moura de Oliveira Beltrame, T. J. Simmons, A. L. Jenkins, T. Dinan and T. J. Nicholson, Gastrointestinal Tolerability and Acute Glycemic Response of Oligosaccharides and Polysaccharides from Cellulose and Xylan in Healthy Adults: Two Double-Blinded, Randomized, Controlled, Cross-over Trials, J. Am. Nutr. Assoc., 2023, 1–10 Search PubMed.
- B. Lin, X. Deng, P. Xu, Q. Ye, G. Zhao, M. Ye and N. Wang, Structural characterization and anti-osteoporosis effect of an arabinomannan from Anemarrhena asphodeloides Bge, Int. J. Biol. Macromol., 2023, 231, 123324 CrossRef CAS PubMed.
- C. P. Fortin and T. Stevanovic, Study of High Molecular Weight Hemicelluloses Recovered from Black Spruce Mill Waste for Potential Value-Added Applications in Foods, Waste Biomass Valorization, 2024, 15, 219–231 CrossRef CAS.
-
E. O. Olorunsola, E. I. Akpabio, M. O. Adedokun and D. O. Ajibola, Emulsifying Properties of Hemicelluloses, in Science and Technology behind Nanoemulsions, IntechOpen, 2018 Search PubMed.
- K. S. Mikkonen, Strategies for structuring diverse emulsion systems by using wood lignocellulose-derived stabilizers, Green Chem., 2020, 22, 1019–1037 RSC.
- M. T. Safian, S. H. Sekeri, A. A. Yaqoob, A. Serrà, M. D. Jamudin and M. N. Mohamad Ibrahim, Utilization of lignocellulosic biomass: A practical journey towards the development of emulsifying agent, Talanta, 2022, 239, 123109 CrossRef CAS PubMed.
- A. Yakdhane, S. Labidi, D. Chaabane, A. Tolnay, A. Nath, A. Koris and G. Vatai, Microencapsulation of Flaxseed Oil—State of Art, Processes, 2021, 9, 295 CrossRef CAS.
- T. M. Ho, M. Lehtonen, H. Räikkönen, P. O. Kilpeläinen and K. S. Mikkonen, Wood hemicelluloses as effective wall materials for spray-dried microcapsulation of polyunsaturated fatty acid-rich oils, Food Res. Int., 2023, 164, 112333 CrossRef CAS PubMed.
- A. Halahlah, H. Räikkönen, V. Piironen, F. Valoppi, K. S. Mikkonen and T. M. Ho, Wood hemicelluloses as sustainable wall materials to protect bioactive compounds during spray drying of bilberries, Powder Technol., 2023, 415, 118148 CrossRef CAS.
- W. Denagbe, R. Covis, J.-P. Guegan, J.-C. Robinson, D. Bereau and T. Benvegnu, Structure and emulsifying properties of unprecedent glucomannan oligo- and polysaccharides from Amazonia Acrocomia aculeata palm fruit, Carbohydr. Polym., 2024, 324, 121510 CrossRef CAS PubMed.
- M. Sobieralska and M. A. Kurek, Beta-Glucan as Wall Material in Encapsulation of Elderberry (Sambucus nigra) Extract, Plant Foods Hum. Nutr., 2019, 74, 334–341 CrossRef CAS PubMed.
-
P. Saraf and M. Montazer, in Natural Gums, ed. S. Ahmed and A. Ali, Elsevier, 2023, pp. 185–260 Search PubMed.
-
M. Upadhyay, A. Kumar and B. Mishra, in Natural Gums, ed. S. Ahmed and A. Ali, Elsevier, 2023, pp. 261–275 Search PubMed.
- K. Nasrallah, S. Khaled, S. El Khatib and M. Krayem, Nutritional, biochemical and health properties of Locust beans and its applications in the food industry: a review, J. Food Sci. Technol., 2024, 61, 621–630 CrossRef PubMed.
-
M. Göksel Saraç, in Natural Gums, ed. S. Ahmed and A. Ali, Elsevier, 2023, pp. 453–473 Search PubMed.
- Z.-H. Huang, Y. Zhao, Z.-X. Hu, L. Ma, S.-Z. Geng, K.-Y. Chen and H.-M. Zhou, Preparation of fat replacer utilizing gluten and barley β-glucan and the interaction between them, J. Sci. Food Agric., 2023, 103, 6288–6296 CrossRef CAS PubMed.
- A. A. Anggraeni, P. Triwitono, L. A. Lestari and E. Harmayani, Evaluation of glucomannan as a fat replacer in the dough and cookies made from fermented cassava flour and soy protein concentrate, Food Chem., 2024, 434, 137452 CrossRef CAS PubMed.
- H. Jeong, J. Lee, Y.-J. Jo and M.-J. Choi, Thermo-irreversible emulsion gels based on deacetylated konjac glucomannan and methylcellulose as animal fat analogs, Food Hydrocolloids, 2023, 137, 108407 CrossRef CAS.
- Y. Wen, B. Cho, H. J. Park and H. W. Kim, Development of 3D-printable plant-based fat analogs utilizing coconut oil and glucomannan emulsion gels, Food Biosci., 2023, 56, 103255 CrossRef CAS.
- D. W. Ningtyas, B. Bhandari, N. Bansal and S. Prakash, Texture and lubrication properties of functional cream cheese: Effect of β-glucan and phytosterol, J. Texture Stud., 2018, 49, 11–22 CrossRef PubMed.
- L. Liu, G. Huang, S. Li, Q. Meng, F. Ye, J. Chen, J. Ming, G. Zhao and L. Lei, Replacement of fat with highland barley β-glucan in zein-based cheese: Structural, rheological, and textual properties, Food Chem.: X, 2023, 20, 100907 CAS.
- T. Kumala, A. Sutrisno and Yunianta, Glucomannan as an anti-staling agent to improve the texture value of whole wheat bread, IOP Conf. Ser. Earth Environ. Sci., 2020, 475, 012030 CrossRef.
- B. Azhar, S. Gunawan, E. R. Febriana Setyadi, L. Majidah, F. Taufany, L. Atmaja and H. W. Aparamarta, Purification and separation of glucomannan from porang tuber flour (Amorphophallus muelleri) using microwave assisted extraction as an innovative gelatine substituent, Heliyon, 2023, 9, e21972 CrossRef CAS PubMed.
- Y. Yao, W. He and B. Xu, Physiochemical characteristics and sensory properties of plant protein isolates–konjac glucomannan compound gels, Food Sci. Nutr., 2023, 11, 5063–5077 CrossRef CAS PubMed.
- L. Huang, Y. Ren, H. Li, Q. Zhang, Y. Wang, J. Cao and X. Liu, Create Fat Substitute From Soybean Protein Isolate/Konjac Glucomannan: The Impact of the Protein and Polysaccharide Concentrations Formulations, Front. Nutr., 2022, 9, 1–13 Search PubMed.
- R. Li, H. Cao, Y. Wang, H. Song, K. Huang, Y. Zhang, Q. Sun, Z. Sun and X. Guan, Improving physicochemical stability of highland barley-based milk by the addition of endogenous β-glucan, Food Hydrocolloids, 2023, 143, 108875 CrossRef CAS.
- H. Cao, R. Li, S. Li, H. Song, K. Huang, Y. Zhang, J. Lu and X. Guan, Unravelling the synergistic effect of incorporation of β-glucan and high intensity ultrasound treatment on the stability of highland barley milk, Food Hydrocolloids, 2024, 147, 109396 CrossRef CAS.
- F. Xie, X. Ren, Z. Zhu, J. Luo, H. Zhang, Z. Xiong, Y. Wu, Z. Song and L. Ai, Tamarind seed polysaccharide-assisted fabrication of stable emulsion-based oleogel structured with gelatin: Preparation, interaction, characterization, and application, Food Hydrocolloids, 2023, 142, 108761 CrossRef CAS.
- N. Bu, L. Huang, G. Cao, J. Pang and R. Mu, Stable O/W emulsions and oleogels with amphiphilic konjac glucomannan network: preparation, characterization, and application, J. Sci. Food Agric., 2022, 102, 6555–6565 CrossRef CAS PubMed.
- L. S. Sciarini, P. M. Palavecino, P. D. Ribotta and G. N. Barrera, Gleditsia triacanthos Galactomannans in Gluten-Free Formulation: Batter Rheology and Bread Quality, Foods, 2023, 12, 756 CrossRef CAS PubMed.
-
T. Dedebas, in Natural Gums, ed. S. Ahmed and A. Ali, Elsevier, 2023, pp. 305–317 Search PubMed.
- Y. Gao, S. Wang, H. Liu, Y. Gu and J. Zhu, Design and characterization of low salt myofibrillar protein-sugar beet pectin double-crosslinked gels pretreated by ultrasound and konjac glucomannan: Conformational and gelling properties, Food Hydrocolloids, 2023, 141, 108717 CrossRef CAS.
- S. Fei, Y. Li, K. Liu, H. Wang, A. M. Abd El-Aty and M. Tan, Salmon protein gel enhancement for dysphagia diets: Konjac glucomannan and composite emulsions as texture modifiers, Int. J. Biol. Macromol., 2024, 258, 128805 CrossRef CAS PubMed.
- Y. Wang, H. Zhang, Q. Liang, X. Guo, Z. Niu, S. Qiu, W. Xu and R. Li, Effect of konjac glucomannan with different degrees of deacetylation on the gel behavior of transglutaminase induced soybean protein isolate emulsion gels, Food Hydrocolloids, 2024, 148, 109493 CrossRef CAS.
- X. Ran, X. Lou, H. Zheng, Q. Gu and H. Yang, Improving the texture and rheological qualities of a plant-based fishball analogue by using konjac glucomannan to enhance crosslinks with soy protein, Innovative Food Sci. Emerging Technol., 2022, 75, 102910 CrossRef CAS.
- J. Yan, L. Yin, Y. Qu, W. Yan, M. Zhang, J. Su and X. Jia, Effect of calcium ions concentration on the properties and microstructures of doubly induced sorghum arabinoxylan/soy protein isolate mixed gels, Food Hydrocolloids, 2022, 133, 107997 CrossRef CAS.
- P. Zhao, N. Li, L. Chen, Y. Guo, Y. Huang, L. Tong, L. Wang, B. Fan, F. Wang and L. Liu, Effects of Oat β-Glucan on the Textural and Sensory Properties of Low-Fat Set Type Pea Protein Yogurt, Molecules, 2023, 28, 3067 CrossRef CAS PubMed.
- X. Zhang, Z. Cheng, X. Zhao, H. Liu, H. Hu, M. Wang and J. Guo, Effects of the oat β-glucan on the functional and structural properties of defatted walnut meal flour, Food Chem. Adv., 2022, 1, 100071 CrossRef.
- K. Huang, S. Zhang, X. Guan, C. Li, S. Li, Y. Liu and J. Shi, Effect of the oat β-glucan on the development of functional quinoa (Chenopodium quinoa wild) milk, Food Chem., 2021, 349, 129201 CrossRef CAS PubMed.
- S. Li, M. Luo, D. Wannasin, X. Hu, J. Ryu, Q. Ju and D. J. McClements, Exploring the potential of plant-based emulsion gels enriched with β-glucan and potato protein as egg yolk alternatives, Food Hydrocolloids, 2024, 148, 109511 CrossRef CAS.
- H. Cao, R. Li, M. Shi, H. Song, S. Li and X. Guan, Promising effects of β-glucans on gelation in protein-based products: A review, Int. J. Biol. Macromol., 2024, 256, 127574 CrossRef CAS PubMed.
- A. N. Arzami, T. M. Ho and K. S. Mikkonen, Valorization of cereal by-product hemicelluloses: Fractionation and purity considerations, Food Res. Int., 2022, 151, 110818 CrossRef CAS PubMed.
- J. Fernández Méndez, F. Farfan Orozco, M. Ladero Galán and H. Grénman, Techno-economic evaluation of obtaining valuable rare sugars from thermo-mechanical pulping side streams utilizing the latest technology, Chem. Eng. J., 2023, 455, 140852 CrossRef.
|
This journal is © The Royal Society of Chemistry 2024 |
Click here to see how this site uses Cookies. View our privacy policy here.