Synthesis of a sustainable marine oleogel and its application as a fat substitute in a sponge cake system
Received
15th December 2023
, Accepted 4th April 2024
First published on 5th April 2024
Abstract
Oleogel technology offers a promising solution for reducing trans fatty acids in food by solidifying liquid oils. However, this technology encounters challenges due to limited vegetable oil resources. This study explores the use of sustainable fish oil, a by-product of sardine canning, especially the saturated fatty acid from the biowaste of PUFA purification, as a liquid phase in oleogels. Beeswax and rice bran wax were tested as oleogelators at concentrations of 5, 10, 15, and 20%. The 20% rice bran wax oleogel exhibited superior gelation properties, reached 90% oil binding capacity, and improved pore distribution without affecting volume (p > 0.05). A sponge cake prepared with commercial margarine (0% oleogel) displayed similar characteristics to a sponge cake with oleogel substitution in terms of hardness (∼6 N), springiness (∼25 mm), cohesiveness (0.4), gumminess (2–3 N), and chewiness (74 J). This indicated that the oleogel substitution mimicked and yielded margarine-like textures. The increasing concentration of oleogel substitution slightly decreased hedonic scores from ∼8.3 to ∼6.6 (from ‘like very much’ to ‘like slightly’). Importantly, the sponge cake with 25% oleogel substitution exhibited high acceptability and overall liking similar to the 0% oleogel (commercial margarine), both scoring at approximately 8. Moreover, the specific texture attributes of the generated sponge cake, such as roughness, moistness, and smoothness, were demonstrated to be similar and comparable to those of commercial margarine, particularly at 25% and 50% oleogel substitution levels. Lipolysis during the in vitro digestion of the sponge cake revealed a reduction in the release of free fatty acids, decreasing from 93.01% to 62.79% with oleogel substitutions ranging from 0% to 75%, respectively. Consequently, the total energy of the product decreased by 14.26% with a 75% oleogel substitution. These findings offer an opportunity to utilize by-products from fish canning for the development of higher-value products. Specifically, this involves constructing an oleogel with a new liquid-phase system while simultaneously reducing margarine consumption. In comparison to other available reports on the utilisation of fish by-products, our study places emphasis on the sequential valorisation of fish oil biowaste after its initial use as the PUFA source. This approach represents a novel way to address challenges in achieving a zero-waste concept in industry related to Sustainable Development Goals (SDGs).
Sustainability spotlight
Our sequential valorization strategy addresses waste in the fishing industry, aligning with Sustainable Development Goals (SDGs) 12 (responsible consumption) and 3 (good health). Recovering fish oil during pre-cooking in the fish canning industry supports both industry resilience and SDGs. Globally, conventional solid fats in cake consumption pose health and environmental challenges. Our research pioneers marine oleogels from fish oil by-products, notably unexplored saturated fatty acids. We attempt to better understand its physicochemical properties to mimic commercial margarine use, eventually offering a financially feasible and environmentally sustainable solution. Repurposing fish oil from canned sardine production not only reduces waste pollution but also aligns with broader SDGs for responsible resource use and public health improvement, promoting sustainable and healthy food production.
|
Introduction
Cakes are widely consumed bakery products globally, produced through industrial or traditional methods using flour, eggs, sugar, and solid fats as the primary ingredients. Fats play a role in incorporating and stabilizing air bubbles during dough preparation, preventing the amalgamation of gas bubbles. This phenomenon contributes to a higher volume and a uniform porous structure within final baked products, facilitating efficient heat transfer during the baking process.1 However, the use of solid fats raises health concerns due to their high-calorie content, trans fats, and saturated fatty acids.2
Solid or semi-solid vegetable oils are extensively used in the food industry for their desirable sensory attributes and high oxidative stability.3 The rheological properties of vegetable oils are improved by partial hydrogenation, a process that generates elevated levels of trans fatty acids.4 Trans fatty acids have been associated with adverse health consequences, such as high cholesterol levels, atherosclerosis, cardiovascular disease, diabetes, and metabolic syndrome.5,6 Consequently, the U.S. Food and Drug Administration (FDA) removed partially hydrogenated oils from its list of generally recognized as safe (GRAS) substances in 2015.7
In recent years, the use of oleogels instead of structured oils such as shortening has shown promising potential to reduce the fat and saturated fatty acid content of products. Oleogels are generated by the gelation of oils using oleogelators by turning liquid oils into structures resembling those of gels, with similar rheological, viscoelastic, spreadability, and firmness properties to solid fats.8,9 Furthermore, oleogelators can be lipid-based, including plant waxes, fatty alcohols, phospholipids, mono- and diacylglycerols, phytosterols, or polymeric. Edible waxes such as rice bran wax (RBW) and beeswax (BW) are common oleogelelators that have been extensively studied for their applications.10,11 Recently, various types of oleogels have been developed to further design and optimize their specific properties in food application.12–15 Oleogels are typically fabricated using various techniques, including direct dispersion,16 biphasic templates,17 and solvent exchange strategies.18 Technically, direct dispersion strategies involve crystallite particle conformations (such as vegetable wax and other edible waxes), aids from polymeric agents (such as hydroxyethyl cellulose), hydrophobic polymers, inorganic particles (such as fumed silica), and self-assembled networks (such as lecithin).14
Despite the excellence of the indirect dispersion oleogelation technique, especially as the scaffold for oleogel systems serving as biofunctional carriers,11,15 direct dispersion, especially wax–oil complex crystal systems, has been deemed the most effective, widely available, and economically feasible options in the food industry.19 It offers a wide range of functionalities across different fields and types of food. The physicochemical properties of wax-based oleogels are influenced by many factors, with particular focus on the interaction between waxes and other components,20 especially the liquid phase. Oleogels are often prepared using liquid phases as a colloidal structure including canola, olive,21 sunflower,22 and sunflower seed oils.23 Although vegetable oils are primarily used, their usage presents challenges such as pesticide residues, harmful chemical contamination, and high production costs.24
The exploration of alternative liquid phases from novel sources such as marine oil is crucial due to the issues often encountered with vegetable oils. The challenge of identifying cost-effective and economically viable sources has hindered the use of a new reservoir of fish oil. Currently, there is a significant demand for the optimization of valuable materials obtained from industrial waste.25–28 In Indonesia, the annual canned sardine production reaches 235
000 tons, generating approximately 5% fish oil as a by-product during pre-cooking.29 This fish oil presents a financially feasible solution, simultaneously promoting environmental sustainability through the repurposing of liquid waste. Industrially, managing these liquid by-products as biowaste is challenging because the oil easily mixes with a large amount of water as liquid waste, leading to its discharge into waste installations and rendering it poorly biodegradable. In fact, fish oil is widely recognized for its high concentration of omega-3 fatty acids and plays a crucial role in the production of fortified food, nutraceuticals, and pharmaceutical products.28,30 Currently, byproducts contribute approximately 26% of the overall world fish oil production.26,27,31
The process of extracting omega-3 fatty acids from fish oil encompasses a variety of processing operations. The technique utilised is determined by the oil's origin and the intended composition of the final product. Subsequent to the extraction of omega-3 fatty acids from primary materials via solvent and/or wet extraction processes, fatty acids are refined by distilling fish oil to remove impurities.32,33 Nowadays, the extraction methods that are more environmentally sustainable, specifically winterisation and supercritical fluid extraction, are regarded as being of greater significance.34,35 However, despite the industry's primary focus on omega-3 fatty acids and PUFAs, over 30% of saturated fatty acids (SFAs) remain unexplored and underutilised. Our previous report revealed that monounsaturated fatty acids (MUFAs) and saturated fatty acids (SFAs) had percentages of 25.05% and 37.11% respectively.36
While the extraction of omega-3 fatty acids from marine-derived oil is widely established, the effective utilisation of other fish oil components, particularly saturated fatty acids (SFAs), has not been extensively studied. Residual SFAs obtained from the purification process of omega-3 fatty acids have significant potential to be employed as a crucial ingredient in the production of shortening or fat replacers. Utilising by-products and waste from the fishing industry downstream is anticipated to offer efficient resolutions for addressing global health challenges and concerns related to Sustainable Development Goals (SDGs) worldwide. Hence, this sequential valorisation strategy represents a new approach to fully optimizing biowaste to apply the zero waste concept in the fishing industry.
Previously, using the direct dispersion oleogelation technique, our group successfully reported the proof of concept for synthesizing ‘marine oleogels’ based on by-products from sardine fish canning. We evaluated the rheology, thermal properties, functional groups during oleogel synthesis, and its overall physical properties.36 However, there is still a limit to the application of this marine oleogel in food products. Furthermore, we are focusing our efforts on investigating the possibilities for using this system in various food model systems.
In addition, ongoing discussions focus on how to more effectively utilize polyunsaturated fatty acids (PUFAs) retained in the byproduct before incorporating them into the oleogel system, as they possess higher economic value. Therefore, after extracting the PUFA-rich oil from the sardine canning byproduct, we sequentially valorised the separated SFA oil from PUFA extraction process as the new liquid phase for generating a ‘sustainable marine oleogel’. This approach is considered a more sustainable option in terms of sourcing and adheres to the zero-waste concept in production of oleogels. Although the utilization of fish oil in preparing cakes was previously studied,37 no research has been conducted on the application of fish oil gels or marine oleogels in cake products. The ‘sustainable marine oleogel’ offers promising potential for integration into food products due to its favourable impact on food quality. Therefore, this study aimed to determine the most suitable types and concentrations of edible waxes as oleogelators, as well as to assess the effects of various levels of generated oleogel substitution on the sensory, physical, and chemical attributes of a sponge cake as a food model system.
Experimental
Materials
The materials used in this study were by-products of canned sardine fish oil (PT Maya Muncar, Banyuwangi, East Java, Indonesia), commercial margarine (Blueband, Indonesia), edible wax beeswax (BW) (Cera Alba, Shanghai, China), rice bran wax (RBW) (Aogubio, Shanghai, China), wheat flour (Bogasari Segitiga Biru, Jakarta, Indonesia), eggs, sugar (Gulaku, Lampung, Indonesia), baking powder (Koepoe Koepoe, Jakarta, Indonesia), and sorbitan monostearate (span 60, emulsifier) (Koepoe Koepoe, Jakarta, Indonesia). All chemicals used were of analytical grade and were acquired from PT. Merck Chemicals and Life Sciences (Darmstadt, Germany).
Preparation of the oleogel
The oleogel was prepared according to the procedures established in our earlier studies36 which were commenced by combining sardine fish oil with BW and RBW at concentrations of 0%, 5%, 10%, 15%, and 20% (w/w). The mixture was heated with a stirrer at 1000 rpm and a temperature of 100 °C for 15 minutes. The oleogel was left at room temperature until complete gelation occurred, stored in a refrigerator (4–5 °C) for 24 hours, and transferred to room temperature for 1 hour before analysis.
Characterisation of oleogels
Colour measurement.
The colour parameters of oleogels and sponge cakes (CIE L*, a*, and b*) were evaluated through image processing methods using a camera (SM-A525F/DS) for photography and ImageJ software for processing.7
Oil binding capacity (OBC).
OBC analysis was carried out by centrifuging the oleogel at a speed of 10
000 rpm for 15 minutes,38 using the equation below:
% OBC = 100 − % oil released |
Preparation of sponge cakes
Sponge cake samples were prepared by mixing sugar (20 g), baking powder (0.6 g), eggs (50 g), and emulsifier (span 60) (5 g) for 3 minutes at medium speed using a mixer based on the method of Wettlaufer20 with modifications. The mixture was expanded with wheat flour (30 g), margarine, chocolate powder (5 g) and vanilla powder (1 g). Before the application in the food model, we conducted the masking process of the oleogel with another compound to hide undesirable aroma or unpleasant taste from the fish oil. We added garlic oil (2%),39 ginger essence (2%), and butter flavour paste (1%) in the oleogel. The margarine in the batter was replaced with the oleogel at 0% (100% commercial margarine), 25%, 50%, and 75%. Subsequently, the sponge cake batter was placed into a baking pan and baked for 20 minutes at 170 °C.
Characterisation of sponge cakes
Sensory evaluation of sponge cakes.
The sensory evaluation included 70 naïve panellists (untrained or consumer panellists), consisting of 21 males/49 females, aged between 19 and 25 years. Panellists used a nine-point hedonic scale, ranging from 1-Dislike extremely, 2-Dislike very much, 3-Dislike moderately, 4-Dislike slightly, 5-Neither like nor dislike, 6-Like slightly, 7-Like moderately, 8-Like very much, and 9-Like extremely. The parameters assessed were appearance, colour, odour, taste, texture, roughness, moistness, smoothness, and acceptance.
Volume expansion of sponge cakes.
The expansion test procedure for sponge cakes was conducted according to Hajrah40 with modification. In this process, the cake was measured using a stick inserted into the centre of the batter before and after baking. The expansion percentage was calculated using the following equation:
Pore distribution analysis.
The internal porosity of the sponge cake product was characterized by allowing the sample to sit for 24 hours and cutting it vertically in half using a knife.23 The porous surface of the sample cross-section was photographed, followed by pore image analysis for pore count and size using ImageJ 1.52 software.
Texture profile analysis.
Texture profile analysis was carried out following the method by Demirkesen23 with the aid of a texture analyzer TA.XT Plus. The cake sample was continuously compressed to 50% of its original height using a cylindrical probe with a diameter of 50 mm, as well as pre- and post-test speeds of 1 mm s−1 and 10 mm s−1, respectively.
Proximate analysis.
The proximate chemical analysis of sponge cakes was carried out using the established standard analytical procedure.41 The moisture content was measured by the gravimetric process. Crude lipid and total protein were extracted using Soxhlet extraction and the Kjeldahl method, respectively. Protein content was calculated using a conversion factor of 6.25 for nitrogen, while the total carbohydrate content was determined using the difference, expressed as 100 – % protein – % fat – % moisture – % ash. All the final parameters were calculated on a dry basis.
In vitro lipid digestibility.
The sponge cake samples were cut into approximately 2 mm long pieces using a knife and then mixed until a texture resembling that of mashed potatoes was obtained. Subsequently, the samples were subjected to digestion using the INFOGEST standardized static in vitro – gastrointestinal digestion simulation42,43 with a few modifications. The simulated salivary fluid (SSF), simulated gastric fluid (SGF), and simulated intestinal fluid (SIF) were heated to 37 °C just before in vitro digestion. The oral phase was initiated by adding 4 mL of SSF, 25 μL of 0.3 M CaCl2, and 975 μL of water to the sample. The sample was then agitated continuously at a temperature of 37 °C for a duration of 2 minutes. Subsequently, 8 mL of SGF, 5 μL of a 0.3 M CaCl2 solution, and 667 μL of an aqueous pepsin solution with an activity of 2000 U mL−1 were added to the sample to create the final chyme. The gastric phase was initiated by acidifying the solution to a pH of 3.0 using 1 M HCl and then increasing the volume to 20 mL with water. The mixture was agitated consistently at a temperature of 37 °C for a duration of 2 hours. Following that, 8 mL of SIF, 4 μL of a 0.3 M CaCl2 solution, 5 mL of a lipase pancreatin solution (made in SIF) with activity levels of 2000 and 100 U mL−1, respectively, in the final mixture, and 3 mL of a 160 mM bile extract (produced in SIF) were added to the sample. The intestinal phase was initiated by adjusting the pH to 8.00 ± 0.10 using 1 M NaOH and changing the volume to 40 mL with water. The mixture was agitated consistently at a temperature of 37 °C for a maximum duration of 2 hours. After in vitro digestion, a 30 mL portion of the mixed micellar phase was obtained by centrifugation at 30
000× g for 70 minutes.
The lipid digestibility or lipolysis of oleogels in sponge cakes was assessed by quantifying the amount of free fatty acids (FFAs) released during the intestinal phase of in vitro digestion. The maximum percentage of free fatty acids (FFAs) released during lipolysis was determined using the method previously described by Ciuffarin.44
Total energy analysis.
The calculation of food energy used Atwater factors for carbohydrates, fats, and proteins. The energy derived from fats was calculated based on the digestion of fats by the body. In this analysis, the percentage of free fatty acids (FFAs) released during in vitro lipolysis was calculated relative to the total fat content measured in the proximate analysis, denoted as ‘digested fat.’ Energy intake and reduction were estimated on a wet basis for a 50 g serving size of sponge cake. The final total energy intake (TEI) was determined using the formula:
Total energy (kcal) = (carbohydrate mass (g) × 4.0 kcal g−1) + (protein mass (g) × 4.0 kcal g−1) + (digested fat mass (g) × 9.0 kcal g−1) |
Statistical analysis.
Data distributions were analysed using analysis of variance (ANOVA) with IBM SPSS Statistics for Windows, Ver. 26 software (IBM Corp., Armonk, NY, USA). The mean comparison was accomplished using Duncan's multiple range tests (p < 0.05), with three replications for each evaluation or treatment.
Results and discussion
Characterisation of fish oil
The byproduct of fish oil was determined to have a fatty acid composition totalling around 93.34%. The profiling results indicate that the percentages of monounsaturated fatty acids (MUFAs), polyunsaturated fatty acids (PUFAs), and saturated fatty acids (SFAs) were 18.01%, 5.94%, and 69.39% respectively. Saturated fatty acids (SFAs) were the most abundant major elements of sardine fish oil after the purification of polyunsaturated fatty acid (PUFA) fish oil. The components, especially SFAs, played a crucial role in establishing the basic structure of the oleogel. SFA, with its extended aliphatic chains, has exceptional gelling capabilities, allowing for the creation of stable gels even at lower concentrations.45 In addition, free fatty acid content, PV, p-anisidine value, and total oxidation, all other parameters complied with the safety standards set by CODEX.46 Furthermore, the samples did not contain any heavy metals.
Characterisation of oleogels
Visualization and colour profiles of oleogels.
As shown in Fig. 1A, the leftmost sample represented the control group, which received no treatment with BW/RBW (0% concentration). Fig. 1B shows the evaluation of oleogel gelation using the inverse tube methodology for 1 hour at ambient temperature. This method was used as a qualitative measure to distinguish gelation states by observing the flow dynamics when samples were subjected to tube inversion. Since inversion, BW and RBW oleogels at 0%, 5%, 10%, and BW 15% showed rapid flow tendencies. After one hour of gelation at room temperature, the tube containing the 20% BW oleogel system exhibited a reversal of the gel to a liquid-like material. The gel system exhibited a gradual drop to the bottom, along with visible residues at the border of the tube. Surprisingly, the non-uniform oleogel structure showed that the 15% RBW oleogel concentration had not reached complete gelation conditions but was able to adhere to the upside of the tube, while the 20% RBW presented a strong ability to generate a stable and compact gel matrix. This indicated the capability of a 20% RBW concentration to generate solid structures at room temperature. Furthermore, each wax type had a varied minimum quantity required for the production of cohesive solid structures.47 The colour variation of oleogels is presented in Table 1.
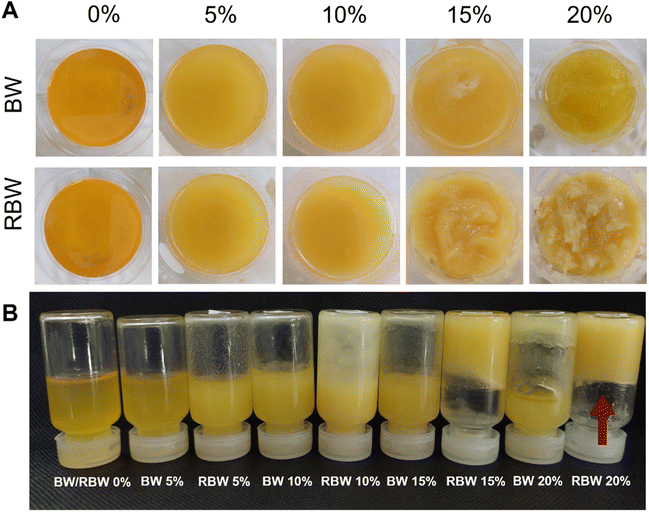 |
| Fig. 1 (A) Oleogel formation of fish oil using BW and RBW at various concentrations (5%, 10%, 15%, and 20% (w/w)), and (B) assessment of oleogel gelation through the inverse tube method (t = 1 hour, 24 °C). | |
Table 1 Colour profile and OBC of oleogelsa
Oleogelator |
L* |
a* |
b* |
OBC (%) |
Different letters in the same column denote statistically significant differences (p < 0.05).
|
RBW |
5% |
68.20 ± 2.72a |
4.68 ± 1.99a |
61.95 ± 0.39d |
38.67 ± 2.91b |
10% |
66.86 ± 4.24a |
7.21 ± 2.17a |
58.36 ± 1.82c d |
68.00 ± 1.33d e |
15% |
64.83 ± 3.84a |
9.10 ± 4.34a |
50.59 ± 6.98b |
78.67 ± 0.67f |
20% |
58.82 ± 2.46a |
8.46 ± 2.97a |
44.40 ± 2.98a |
90.44 ± 2.04g |
BW |
5% |
66.86 ± 3.27a |
4.97 ± 2.19a |
60.13 ± 1.92c d |
28.89 ± 2.69a |
10% |
65.43 ± 2.61a |
6.39 ± 0.74a |
60.48 ± 1.14c d |
61.33 ± 1.33c |
15% |
62.03 ± 2.18a |
9.97 ± 1.55a |
56.34 ± 0.81c |
64.67 ± 4.67c d |
20% |
63.38 ± 1.20a |
1.89 ± 0.65a |
61.03 ± 0.86c d |
71.33 ± 3.71e |
Control |
0% |
59.87 ± 1.47 |
16.91 ± 2.54 |
61.70 ± 2.71 |
— |
As shown in Table 1, there were no significant differences in colour qualities across the samples for L* and a* values. A significant trend was observed in the b* values, which decreased as oleogelator concentrations increased and turned more yellow. In this study, the BW oleogel was white, but the RBW oleogel was yellow due to the natural yellowish pigment of wax-based substitution influencing the augmentation of the coloration.48 The previous study found that increasing the concentration of RBW elevated the lightness of the oleogel, despite the oil type but had no effect on a* and b*. This suggested that the oil type influenced coloration49 due to fish oil pigments such as carotenoid and tocopherol.50
Oil binding capacity (OBC).
OBC of oleogels is an important characteristic for assessing their value in food applications. Based on the results, RBW consistently showed the highest OBC values for all concentrations. As shown in Table 1, the highest OBC value (90.44 ± 2.04%), was obtained in the 20% RBW, with increasing concentrations causing a significant increase in the OBC value (p < 0.05). Previous reports38,51 showed a strong relationship between the oleogel network and mechanical strength in oil binding. Furthermore, it was discovered that increasing the concentration of the oleogel improved viscosity, structural compactness, and OBC.52 The ability of the oleogel to synthesize a more stable three-dimensional network was also influenced by the fatty acid composition found in oil. Saturated fatty acids (SFAs) were found to be more effective at forming an established oleogel network.53 Oil leaked in food products with low oil binding capacity could have a negative impact on the texture and sensory characteristics of the product.54 RBW 20% was selected for the next oleogelator in the food model system due to its effective and proper gelation properties and strong ability to bind oil.
Characterisation of sponge cakes as a food model
Sensory evaluation of cakes.
Table 2 shows an overview of the sensory evaluations defined for various cake samples. The 25% oleogel substitution sample had the highest acceptance scores, followed by sponge cakes with 0%, 50%, and 75% oleogel substitutes. The appearance, colour, texture, roughness, moistness, and smoothness of sponge cakes with 25%, 50%, and 75% oleogel replacements received ‘like moderately’ to ‘like very much’ ratings. However, the sensory evaluation showed that the presence of slight unpleasant odours at higher oleogel substitution levels reduced panellists' acceptance of the product's odour (neither like nor dislike). This slight rancid odour was caused by volatile compounds present in fish oil oleogels, particularly polyunsaturated fatty acids (PUFAs).55
Table 2 Hedonic analysis of sponge cakesa
Attribute |
Oleogel substitution (%) |
0 |
25 |
50 |
75 |
Different letters in the same row denote statistically significant differences (p < 0.05).
|
Appearance |
8.16 ± 0.79a |
8.76 ± 0.74a |
7.70 ± 0.79b |
7.50 ± 0.89b |
Colour |
8.02 ± 0.82a |
8.52 ± 0.89a |
7.60 ± 0.81b |
7.26 ± 0.85b |
Aroma |
8.56 ± 0.58a |
7.58 ± 1.16b |
6.88 ± 1.19c |
5.48 ± 1.03c |
Taste |
8.46 ± 0.58a |
7.92 ± 1.07b |
6.46 ± 0.99c |
6.24 ± 0.87c |
Texture |
8.12 ± 0.87a |
8.50 ± 0.95b |
7.44 ± 0.81b |
6.20 ± 0.99b |
Roughness |
7.96 ± 0.81a |
7.24 ± 0.89b |
7.12 ± 0.92b |
7.00 ± 0.90b |
Moistness |
8.12 ± 0.75a |
7.54 ± 0.95b |
7.34 ± 0.94b |
7.30 ± 0.84b |
Smoothness |
8.16 ± 0.65a |
8.60 ± 0.76a |
7.52 ± 0.89b |
7.22 ± 0.97c |
Overall |
8.38 ± 0.53a |
8.34 ± 0.98a |
7.10 ± 0.99b |
6.62 ± 0.92c |
Overall, the panellists liked the texture of sponge cakes with oleogel substitutes. Among the substitutions, the sponge cake with a 25% oleogel obtained the most acceptance, while 0% and 50% generated comparable results, compared to the 75% substitution. The appearance of larger and uniform pores was associated with increased oleogel substitution levels. The unequal dispersion of oleogels caused small aggregates to agglomerate within the sponge cake batter. The uneven distribution of air in the batter, which was not adequately stabilized due to the lack of solid fat crystals in the batter network, reduced consistency in size or porosity in the cake, as reported by Patel.53 Despite the non-uniformity of these air pockets, the cake remained less cohesive but was acceptable to panellists. Although this evaluation indicated that the RBW oleogel functioned effectively in sponge cakes, improvements were recommended, particularly in flavour preferences.
Importantly, overall liking demonstrated that a 25% oleogel substitution achieved comparable acceptance among panellists. To maximize the oleogel concentration (>75% substitution) for commercial margarine, it is necessary to enhance the masking process to mitigate the unpleasant fish oil odour. This involves increasing the concentration of specific essences sufficiently to neutralize the oil taste and odour. In fact, all the oleogel-based systems that were reported had a maximum integration of 50% in the food system.9,49,56–58 There is currently no available report on the utilization of oleogels in food items with a substitution rate over 50%. Interestingly, this oleogelation technique with edible wax significantly reduces the fish odour from fish oil. This realization not only utilizes the by-product effectively but also initiates possibilities for fortifying fish oil in a milder way. This initial screening demonstrates the feasibility of incorporating this by-product into value-added product development.
Colour parameters.
The colour profile of sponge cakes with varied oleogel substitution ratios is shown in Table 3. Specifically, the L* values (brightness, scale 0–100) of the samples with 25% and 50% oleogel substitutions were not significantly different, while 75% substitution showed substantial variation. The L* value of the 0% sample varied significantly from those of the 25%, 50%, and 75% substitutions due to the yellowish colour of RBW used, which increased the brightness value.
Table 3 Colour profile of sponge cakesa
Oleogel substitution (%) |
L* |
a* |
b* |
Different letters in the same row denote statistically significant differences (p < 0.05).
|
0 |
19.64 ± 3.78a b |
6.48 ± 1.62a |
6.34 ± 2.41a |
25 |
18.33 ± 3.77a |
9.57 ± 0.71b |
8.02 ± 0.08a |
50 |
18.06 ± 1.42a |
7.98 ± 2.23 ab |
8.93 ± 3.26a |
75 |
22.93 ± 3.45b |
9.49 ± 1.58b |
10.02 ± 3.37a |
Previous studies regarding shortening substitution with wax-based oleogels in cake samples increased L* values, while no significant differences were observed in a* and b* values.23 Generally, colour is an attribute that improves consumer acceptability of a product. In this study, the similarity in colour properties between oleogel-containing cakes and the margarine sample suggested that marine oil-based oleogels could be used in the bakery industry.2
Volume and textural characteristics of sponge cakes.
According to Table 4, there were no significant differences in the volume parameters between oleogel-containing cakes and the margarine sample. Sponge cake batter was a more complex water-in-oil (O/W) emulsion with distributed bubbles as the discontinuous phase and an egg–sugar–water–fat mixture, consisting of dispersed flour particles as the continuous phase. When the temperature expands, fat particles attached to the air–water interface melt, providing air bubbles to expand. The presence of numerous fat particles allowed air bubbles to develop without bursting.59 Sponge cakes containing oleogels had larger volumes compared to those with conventional margarine. This result showed that a higher oleogel concentration enhanced gas retention capability within the batter.
Table 4 Texture profile and specific volume of cakes added with different levels of oleogela
Parameter |
Oleogel substitution (%) |
0 |
25 |
50 |
75 |
Different letters in the same row denote statistically significant differences (p < 0.05).
|
Hardness (N) |
6.33 ± 0.48a |
6.30 ± 0.21a |
6.15 ± 0.47a |
7.39 ± 0.31b |
Springiness (mm) |
25.00 ± 0.27a b |
25.55 ± 0.24b |
25.44 ± 0.34b |
24.74 ± 0.33a |
Cohesiveness |
0.47 ± 0.00b |
0.46 ± 0.00b |
0.47 ± 0.00b |
0.43 ± 0.01a |
Gumminess (N) |
2.96 ± 0.20a |
2.93 ± 0.11a |
2.87 ± 0.20a |
3.19 ± 0.14a |
Chewiness (J) |
73.84 ± 4.21a |
74.76 ± 2.52a |
73.04 ± 5.25a |
78.83 ± 4.27a |
Increasing volume of cake (%) |
29.36 ± 1.44a |
34.07 ± 5.04a |
30.64 ± 3.51a |
29.68 ± 4.29a |
Table 4 summarizes the textural parameters obtained from texture profile analysis, including hardness, springiness, cohesiveness, gumminess, and chewiness. The hardness of sponge cakes with 25% and 50% RBW oleogel substitutions was not statistically different from that of the 0% substitution, while that of 75% substitution increased significantly (7.39 ± 0.31 N). Patel53 found that oleogel-based cakes had a harder texture compared to shortening-based cakes. This characteristic is a physical indicator of cake products, which has a negative effect on consumer acceptance.60 Furthermore, it was related to the density of the cake, where a higher air bubble volume during batter mixing led to reduced density and hardness.61 In oleogel-substituted cakes, increased hardness can be attributed to a drop in batter viscosity, followed by a decrease in density and air bubble count.23
The springiness values of sponge cakes with 25% and 50% substitutions were not significantly different but showed a decreasing value compared to 75% substitution. Cohesiveness values of sponge cake samples with 0%, 25%, and 50% substitutions were not statistically different, while 75% had a significant decrease. According to Kim and co-workers,61 substituting 50% canola oil with a carnauba wax-based oleogel resulted in springiness and cohesiveness values, which were not significantly different from those of commercial shortening. Springiness values represent elasticity or the ability of a product to recover to its initial structure after exposure to pressure.2 According to Adili,7 a cake substituted with a 50% oleogel containing 2% ethyl cellulose and 4% adipic acid had cohesive values that were not significantly different from those of commercial shortening. Cohesiveness ratings represent the ability of a product to adapt to relative deformation during the second cycle of deformation in comparison with resistance in the first cycle.62
The chewiness values of the cake samples range from 2.87 ± 0.20 to 3.19 ± 0.14 J. In a previous study, it was discovered that 6% ethyl cellulose increased cake chewiness but did not show any significant differences with 2% ethyl cellulose or 4% adipic acid substitutions.7 Chewiness refers to the amount of energy required to chew food materials and is considered in food manufacturing, depending on the target consumer class for the end product.2
Pore evaluation of cakes.
The distribution of pore size diameters in sponge cakes as shown in Fig. 2 ranged from 0.01 to 0.4 cm. This result showed an increasing trend in the distribution of pore diameters in sponge cakes toward larger-sized fractions with high oleogel substitution ratios. Generally, pore size and distribution can affect the crumb structure, softness, and moisture retention of the cake. Uniform pore distribution leads to a softer and more delicate crumb, while uneven distribution results in a drier and less resilient texture. Demirkesen and Mert23 discovered higher pore diameters in cakes containing candelilla wax and RBW oleogel. The gas retention characteristics of RBW within the cake batter allowed the air bubbles created during the baking process to resist cracks. The uneven dispersion of the oleogel within the batter contributed to the formation of larger pores in the sponge cake. Meanwhile, a high substitution led to the formation of small clusters of oleogels that solidified within the batter.
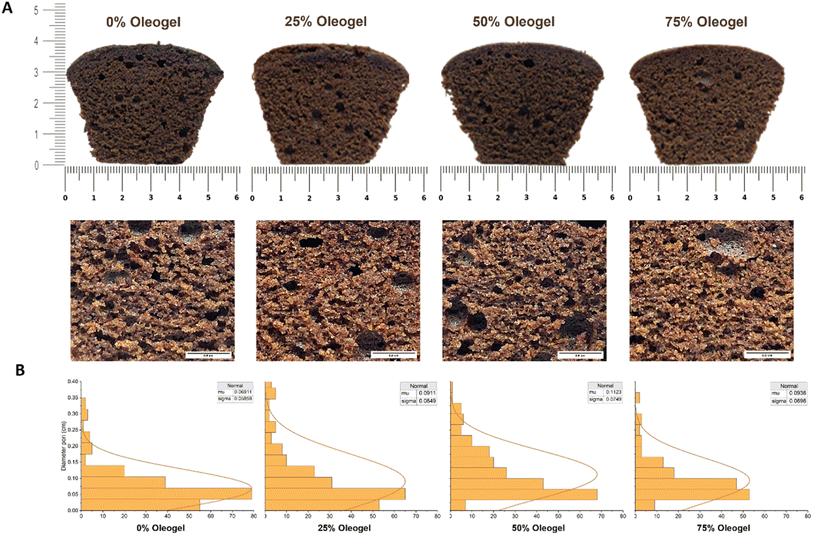 |
| Fig. 2 (A) Scans of truncated sponge cakes with varying oleogels and (B) pore size distribution (PSD, as Feret diameter) of sponge cakes with different levels of oleogel. | |
In vitro lipid digestion of sponge cakes.
The lipolysis or lipid digestibility of sponge cakes substituted by oleogels was monitored by measuring the free fatty acid (FFA) released during the intestinal phase of in vitro digestion (Fig. 3). The total free fatty acid (FFA) release during intestinal digestion was determined by measuring the maximal release after 2 hours of digestion, consistent with a prior study.63
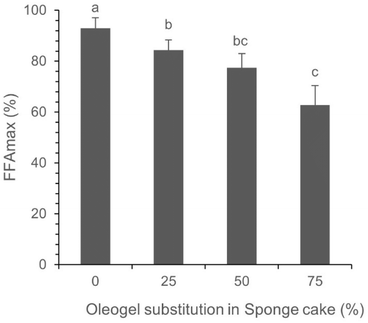 |
| Fig. 3 Total free fatty acids (FFAmax) released during in vitro intestinal digestion of sponge cakes with various concentrations of oleogel substitution. Different letters denote statistically significant differences (p < 0.05). | |
The FFA release did not reach a 100% rate, possibly due to the selective hydrolysis carried out by lipase on specific types of free fatty acids or triglycerides. Fig. 3 illustrates a significant difference in the release of FFAs (free fatty acids) between the sponge cake made with a 0% oleogel (using commercial margarine), which achieved a 93% release, and the cakes made with 25%, 50%, and 75% substitution, showing 87%, 72%, and 54% release of FFAs, respectively.
Wax esters, such as RBW, are esterified long-chain fatty alcohols with long-chain fatty acids, which are poorly digested by many mammals, including humans, and resistant to lipase hydrolysis.64 The addition of an RBW oleogelator resulted in a reduction in the release of maximum free fatty acid (FFAmax) content. The presence of structured edible wax allowed lipase to retain accessibility to its active sites, explaining this behaviour when compared to a food model using commercial margarine.
Based on our findings, two potential processes could hinder or slow down the lipid digestion process in sponge cakes substituted with the oleogel. First, the crystal network may restrict the accessibility of lipase to the lipid. Additionally, the crystallization process of the oleogel may have an impact. The oleogel is composed of needle-shaped crystals formed through van der Waals interactions between long hydrocarbon chains and polar ester groups, as previously described.65 These factors could potentially impede the lipolysis process and/or reduce the efficiency of fat digestion through lipase hydrolysis.66 Principally, additional research is required to examine the effects of RBW oleogel-incorporating food products on lipid digestion behaviours through in vivo clinical trials.
Proximate compound and energy intake of sponge cakes.
The proximate compound presented in Table 5 indicated that the fat content of oleogel-substituted sponge cakes ranged from 31% to 36% and increased with higher substitution. The elevation in oleogel substitution was due to the increase in RBW content in the sponge cake. Wax-based oleogels comprised various non-polar components, detected as fat in analyses. Based on the results, RBW consists of several non-polar fractions, such as wax esters (93.5%), free fatty acids (6%), hydrocarbons (0.29%), and fatty alcohols (0.22%).67,68 The previous in vitro test successfully revealed that approximately 62% of free fatty acids were released and detected. This indicated that there might be around 40% of undigested fat present in the sponge cake system. Consequently, the increase in oleogel substitution in the sponge cake resulted in undigested fat, ultimately leading to a reduction in energy intake from the food. The previous study also stated that RBW oleogels could reduce fat digestion, decrease accumulation by reducing adipose tissue formation, and enhance excretion through feces.10 This suggested the potential of oleogels to reduce the ability of the body to digest fats from the diet. The total calorie content of sponge cakes decreased significantly as oleogel substitution increased, where a 14.26% reduction was achieved with 75% oleogel substitution. This decrease in total energy was influenced by the lower digestibility of the oleogel system.
Table 5 Proximate compoundb and energy intakeca
Parameter |
Oleogel substitution (%) |
0 |
25 |
50 |
75 |
Different letters in the same row denote statistically significant differences (p < 0.05).
Dry basis.
Estimated on a wet basis per 50 g serving size of sponge cake.
Calculated from the proximate fat and FFAmaxin vitro digestion result.
|
Ash (%) |
1.89 ± 0.01c |
1.57 ± 0.03b |
1.59 ± 0.04b |
1.32 ± 0.01a |
Fat (%) |
31.10 ± 0.13a |
31.70 ± 0.57a |
34.10 ± 0.31b |
36.23 ± 0.23c |
Protein (%) |
12.36 ± 0.10c |
7.57 ± 0.37a |
9.76 ± 0.18b |
10.14 ± 0.29b |
Carbohydrate (%) |
54.66 ± 0.36b |
59.17 ± 0.11c |
54.55 ± 0.28b |
52.33 ± 0.54a |
Protein energy (kcal 50 g−1) |
17.95 ± 0.07d |
11.20 ± 0.42a |
14.30 ± 0.28b |
15.60 ± 0.42b |
Carbohydrate energy (kcal 50 g−1) |
79.35 ± 0.78a |
87.50 ± 0.56a |
79.95 ± 0.35a |
80.60 ± 0.71a |
Fat energyd (kcal 50 g−1) |
170.05 ± 4.45d |
160.34 ± 4.96c |
156.65 ± 5.66b |
132.96 ± 3.76a |
Energy intake (kcal 50 g−1) |
267.32 ± 0.29d |
259.02 ± 3.93c |
250.90 ± 1.31b |
229.19 ± 0.88a |
Energy reduces (%) |
0.00 ± 0.00 |
3.11 ± 0.49 |
6,14 ± 1.17 |
14.26 ± 1.60 |
In addition, a considerable amount of FFAs continued to be released and counted, contributing not only from the structured fat but also from the fat content in eggs, chocolates, and other cake ingredients. As a result, more than 50% of FFAs remained, available for digestion in the gastrointestinal tract. In fact, there is still a limitation on the clinically proven health benefits, safety, and toxicity of oleogels.69 Moreover the high substantial quantity of undigested fat poses notable health risks. Therefore, the potential consequences of oleogel's reduced digestibility vary depending on its specific application within the food industry or specific consumer categories.
From a health perspective, diminished lipid digestibility may trigger a feedback mechanism in the human body, inhibiting food intake, expediting gastric emptying, and enhancing satiety. These effects could indirectly impact eating behavior.70 Diaz-Ruiz and co-workers71 investigated the health benefits of calorie restriction in vivo, including weight loss, enhanced insulin sensitivity, decreased chronic inflammation, prevention of cardiovascular and neurological disorders, as well as anti-cancer protection. Consequently, reducing fat digestion capability in diets might be beneficial for preventing negative health impacts.
Conclusions
In conclusion, this study demonstrated that the type and concentration of oleogelator played a significant role in the formation process of marine oleogels using fish oil derived from the byproduct of the sardine canning industry, especially in utilizing the SFA portion of the fish oil. Based on the results, the oleogel with a 20% RBW concentration exhibited the most effective gelling performance. Hedonic sensory evaluation of the sponge cake indicated that a 25% substitution had high acceptance and overall liking among the panellists. Importantly, substituting margarine with a 25% and 50% oleogel concentration produced a sponge cake with physical properties similar to those of commercial margarine. After 75% oleogel substitution, the nutritional evaluation of the sponge cake suggested a 14% reduction in total energy. Overall, it can be concluded that the marine oleogel has the potential to partially substitute fat in the product matrix, hence enhancing the nutritional profile. This is also achieved by mimicking the rheological and textural properties of margarine. These findings offer an opportunity to utilize fish canning by-products to produce more value-added products, particularly addressing SDG issues and promoting a more sustainable source of oil, while also reducing margarine consumption. An optimization study considering independent variables, such as the temperature of the gelling point, mixing conditions, or any additive materials, including antioxidants, can be crucial for obtaining the most convenient results. Additionally, considering various options such as utilizing different oleogelation methods such as indirect gelation or emulsion templates can be beneficial for generating and incorporating high-temperature-sensitive nutrient components. Future investigations may explore incorporating other nutritional compounds or bioactive substances into the marine oleogel scaffold to fortify value-added products and assess their impact through in vivo studies.
Author contributions
Wahyu Ramadhan: conceptualization (lead); funding acquisition (lead); project administration (lead); writing – original draft (lead). Anita Nurul Firdaos: data curation (lead); writing – original draft (supporting); project administration (supporting); visualization (supporting). William Vito Krisnawan: data curation (supporting); formal analysis (equal); methodology (supporting). Sugeng Heri Suseno: investigation (supporting); supervision (equal). Bambang Riyanto: investigation (equal); supervision (equal). Wini Trilaksani: investigation (supporting); supervision (equal). Joko Santoso: investigation (supporting); supervision (supporting); validation (supporting).
Conflicts of interest
There are no conflicts to declare.
Acknowledgements
The authors are grateful to the Directorate Research and Innovation (DRI), IPB University, Indonesia, for the grant (Grant number: 11459/IT3/PT.01.03/P/B/2023), as part of the Young Lecturer Research Program 2023.
Notes and references
- R. V. Rios, M. D. F. Pessanha, P. F. de Almeida, C. L. Viana and S. C. da S. Lannes, Food Sci. Technol., 2014, 34, 3–15 CrossRef.
- H. Pehlivanoglu, G. Ozulku, R. M. Yildirim, M. Demirci, O. S. Toker and O. Sagdic, J. Food Process. Preserv., 2018, 42, 1–11 CrossRef.
- I. K. Oh, C. Amoah, J. Lim, S. Jeong and S. Lee, Lwt, 2017, 86, 430–437 CrossRef CAS.
- Z. Saghafi, M. H. Naeli, M. Bahmaei, M. Tabibiazar and A. Zargaraan, J. Food Meas. Char., 2019, 13, 3040–3048 CrossRef.
- J. Alvarez-Ramirez, E. J. Vernon-Carter, Y. Carrera-Tarela, A. Garcia and C. Roldan-Cruz, LWT, 2020, 130, 109701 CrossRef CAS.
- P. Nestel, Clin. Ther., 2014, 36, 315–321 CrossRef CAS PubMed.
- L. Adili, L. Roufegarinejad, M. Tabibiazar, H. Hamishehkar and A. Alizadeh, Lwt, 2020, 126, 109277 CrossRef CAS.
-
D. C. Z. Botega, Application of Rice Bran Wax Organogel to Substitute Solid fat and Enhance Unsaturated Fat Content in Ice Cream, The University of Guelph, 2012 Search PubMed.
- E. D. Co and A. G. Marangoni, JAOCS, J. Am. Oil Chem. Soc., 2012, 89, 749–780 CrossRef.
- W. Limpimwong, T. Kumrungsee, N. Kato, N. Yanaka and M. Thongngam, J. Funct. Foods, 2017, 39, 250–256 CrossRef CAS.
- A. J. Martins, A. A. Vicente, L. M. Pastrana and M. A. Cerqueira, Food Sci. Hum. Wellness, 2020, 9, 31–39 CrossRef.
- S. Perţa-Crişan, C. -Ştefan Ursachi, B.-D. Chereji and F.-D. Munteanu, Foods, 2022, 12, 131 CrossRef PubMed.
- Y. Gao and S. Wu, Food Funct., 2020, 11, 7727–7735 RSC.
- R. C. da Silva, M. J. Ferdaus, A. Foguel and T. L. T. da Silva, Gels, 2023, 9, 180 CrossRef PubMed.
- T. C. Pinto, A. J. Martins, L. Pastrana, M. C. Pereira and M. A. Cerqueira, Gels, 2021, 7, 1–24 CrossRef PubMed.
- F. Valoppi, J. Schavikin, P. Lassila, I. Laidmäe, J. Heinämäki, S. Hietala, E. Haeggström and A. Salmi, Food Struct., 2023, 37, 100338 CrossRef CAS.
- K. Rehman, M. C. I. M. Amin and M. H. Zulfakar, J. Oleo Sci., 2014, 63, 961–970 CrossRef CAS PubMed.
- S. Li, Q. Song, K. Liu, Y. Zhang, G. Zhao and Y. Zhou, LWT, 2023, 176, 114545 CrossRef CAS.
- S. Manzoor, F. A. Masoodi, F. Naqash and R. Rashid, Food Hydrocolloids Health, 2022, 2, 100058 CrossRef.
- T. Wettlaufer and E. Flöter, Food Funct., 2022, 13, 9419–9433 RSC.
- E. Yilmaz and M. Öğütcü, RSC Adv., 2015, 5, 50259–50267 RSC.
- E. Panagiotopoulou, T. Moschakis and E. Katsanidis, Food Sci. Technol., 2016, 73, 351–356 CAS.
- I. Demirkesen and B. Mert, JAOCS, J. Am. Oil Chem. Soc., 2019, 96, 545–554 CrossRef CAS.
- Y. Zhou, W. Zhao, Y. Lai, B. Zhang and D. Zhang, Front. Plant Sci., 2020, 11, 1–16 CrossRef PubMed.
- P. Ideia, J. Pinto, R. Ferreira, L. Figueiredo, V. Spínola and P. C. Castilho, Waste Biomass Valorization, 2020, 11, 3223–3246 CrossRef.
- A. P. Carvalho, M. Amorim, L. Rodríguez-Alcalá, J. Fontecha, P. M. L. Castro and M. E. Pintado, ACS Sustain. Chem. Eng., 2018, 6, 15447–15454 CrossRef CAS.
- D. Coppola, C. Lauritano, F. Palma Esposito, G. Riccio, C. Rizzo and D. de Pascale, Mar. Drugs, 2021, 19, 116 CrossRef CAS PubMed.
- G. Caruso, R. Floris, C. Serangeli and L. Di Paola, Mar. Drugs, 2020, 18, 622 CrossRef CAS PubMed.
- A. Wujdi, S. Suwarso and W. Wudianto, BAWAL Widya Riset Perikanan Tangkap, 2013, 5, 49–57 Search PubMed.
- D. S. Siscovick, T. A. Barringer, A. M. Fretts, J. H. Y. Wu, A. H. Lichtenstein, R. B. Costello, P. M. Kris-Etherton, T. A. Jacobson, M. B. Engler, H. M. Alger, L. J. Appel and D. Mozaffarian, Circ. J., 2017, 135, e867–e884 CrossRef CAS PubMed.
- R. Ciriminna, A. Scurria, G. Avellone and M. Pagliaro, ChemistrySelect, 2019, 4, 5106–5109 CrossRef CAS.
- S. Heri Suseno, E. Dyah Puri Sintoko, A. M. Jacoeb and N. Fitriana, Orient. J. Chem., 2017, 33, 3150–3159 CrossRef.
- S. Bija, S. H. Suseno and U. Uju, Jurnal Pengolahan Hasil Perikanan Indonesia, 2017, 20, 143 CrossRef.
- K. Ivanovs and D. Blumberga, Energy Procedia, 2017, 128, 477–483 CrossRef CAS.
- F. Al Khawli, M. Pateiro, R. Domínguez, J. M. Lorenzo, P. Gullón, K. Kousoulaki, E. Ferrer, H. Berrada and F. J. Barba, Mar. Drugs, 2019, 17, 689 CrossRef CAS PubMed.
- W. Ramadhan, W. V. Krisnawan, A. N. Firdaos and B. Riyanto, Int. J. Food Sci. Technol., 2024, 59, 754–764 CrossRef CAS.
- T. H. Pi, C. Y. Shiau, C. J. Chang and W. C. Sung, J. Aquat. Food Prod. Technol., 2017, 26, 969–978 CrossRef CAS.
- Z. Meng, K. Qi, Y. Guo, Y. Wang and Y. Liu, Food Chem., 2018, 246, 137–149 CrossRef CAS PubMed.
- K. Sridhar, M. Sharma, A. Choudhary, P. K. Dikkala and K. Narsaiah, J. Food Process. Preserv., 2021, 45, e15346 CAS.
- N. A. Hajrah, A. Hintono, D. Valentinus and P. Bintoro, Jurnal Teknologi Pangan, 2019, 3, 7–12 Search PubMed.
-
[AOAC] Association of Official Analytical Chemists, Official Methods of Analysis of AOAC International, AOAC International Press, Maryland, 17th edn, 2000, vol. 1 Search PubMed.
- A. Brodkorb, L. Egger, M. Alminger, P. Alvito, R. Assunção, S. Ballance, T. Bohn, C. Bourlieu-Lacanal, R. Boutrou, F. Carrière, A. Clemente, M. Corredig, D. Dupont, C. Dufour, C. Edwards, M. Golding, S. Karakaya, B. Kirkhus, S. Le Feunteun, U. Lesmes, A. Macierzanka, A. R. Mackie, C. Martins, S. Marze, D. J. McClements, O. Ménard, M. Minekus, R. Portmann, C. N. Santos, I. Souchon, R. P. Singh, G. E. Vegarud, M. S. J. Wickham, W. Weitschies and I. Recio, Nat. Protoc., 2019, 14, 991–1014 CrossRef CAS PubMed.
- S. Sabet, S. J. Kirjoranta, A.-M. Lampi, M. Lehtonen, E. Pulkkinen and F. Valoppi, Food Res. Int., 2022, 160, 111633 CrossRef CAS PubMed.
- F. Ciuffarin, M. Alongi, S. Plazzotta, P. Lucci, F. P. Schena, L. Manzocco and S. Calligaris, Food Res. Int., 2023, 173, 113239 CrossRef CAS PubMed.
- P. E. Thomas, M. Saravanan and P. Prabhasankar, Int. J. Food Sci. Technol., 2023, 58, 1434–1443 CrossRef CAS.
-
CODEX, Standard for Fish Oil CXS 329-2017 Amended in 2021, 2021, vol. i Search PubMed.
- H. S. Hwang, M. Singh and S. Lee, J. Food Sci., 2016, 81, C1045–C1054 CrossRef CAS PubMed.
- A. Aliasl khiabani, M. Tabibiazar, L. Roufegarinejad, H. Hamishehkar and A. Alizadeh, Food Chem., 2020, 333, 127446 CrossRef CAS PubMed.
- M. Zhao, Y. Lan, L. Cui, E. Monono, J. Rao and B. Chen, Food Chem., 2020, 309, 1–9 Search PubMed.
- S. H. Suseno, A. Y. Tajul, W. A. Nadiah and A. F. Noor, Int. Food Res. J., 2012, 19, 1383–1386 Search PubMed.
- G. Fayaz, S. A. H. Goli, M. Kadivar, F. Valoppi, L. Barba, S. Calligaris and M. C. Nicoli, LWT, 2017, 86, 523–529 CrossRef CAS.
- K. Zhang, W. Wang, X. Wang, S. Cheng, J. Zhou, Z. Wu and Y. Li, J. Sci. Food Agric., 2019, 99, 4063–4071 CrossRef CAS PubMed.
- A. R. Patel, P. S. Rajarethinem, A. Grędowska, O. Turhan, A. Lesaffer, W. H. De Vos, D. Van De Walle and K. Dewettinck, Food Funct., 2014, 5, 645–652 RSC.
- C. Park and F. Maleky, Front. Sustain. Food Syst., 2020, 4, 1–8 CrossRef.
- Y. Q. Wen, H. W. Zhang, C. H. Xue, X. H. Wang, S. J. Bi, L. L. Xu, Q. Q. Xue, Y. Xue, Z. J. Li, J. Velasco and X. M. Jiang, Food Chem., 2023, 399, 1–8 CrossRef PubMed.
- A. J. Martins, A. A. Vicente, R. L. Cunha and M. A. Cerqueira, Food Funct., 2018, 9, 758–773 RSC.
- Z. Huang, B. Guo, D. Gong and G. Zhang, Food Chem., 2022, 404, 134553 CrossRef PubMed.
- L. Li, G. Liu, O. Bogojevic, J. N. Pedersen and Z. Guo, Compr. Rev. Food Sci. Food Saf., 2022, 21, 2077–2104 CrossRef PubMed.
-
S. G. Sumnu and S. Sahin, Food Engineering Aspects of Baking Sweet Goods, CRC Press, Boca Raton, 2008 Search PubMed.
- H. Pehlivanoğlu, M. Demirci, O. S. Toker, N. Konar, S. Karasu and O. Sagdic, Crit. Rev. Food Sci. Nutr., 2018, 58, 1330–1341 CrossRef PubMed.
- J. Y. Kim, J. Lim, J. Lee, H.-S. Hwang and S. Lee, J. Food Sci., 2017, 82, 445–452 CrossRef CAS PubMed.
- X. Ye, P. Li, Y. M. Lo, H. Fu and Y. Cao, J. Food Sci., 2019, 84, 1456–1464 CrossRef CAS PubMed.
- D. M. Pascoviche, N. Goldstein, A. Fishman and U. Lesmes, Colloids Surf., A, 2019, 561, 70–78 CrossRef CAS.
- S. R. Vali, Y. Ju, T. N. B. Kaimal and Y. Chern, J. Am. Oil Chem. Soc., 2005, 82, 57–64 CrossRef CAS.
- L. S. K. Dassanayake, D. R. Kodali, S. Ueno and K. Sato, J. Oleo Sci., 2012, 61, 1–9 CrossRef PubMed.
- P. J. Wilde and B. S. Chu, Adv. Colloid Interface Sci., 2011, 165, 14–22 CrossRef CAS PubMed.
- C. D. Doan, C. M. To, M. De Vrieze, F. Lynen, S. Danthine, A. Brown, K. Dewettinck and A. R. Patel, Food Chem., 2017, 214, 717–725 CrossRef CAS PubMed.
- A. Singh, F. I. Auzanneau and M. A. Rogers, Food Res. Int., 2017, 97, 307–317 CrossRef CAS PubMed.
- T. H. Tan, E. Chan, M. Manja, T. Tang, E. Phuah and Y. Lee, J. Am. Oil Chem. Soc., 2023, 100, 681–697 CrossRef CAS.
- C. M. O'Sullivan, S. Barbut and A. G. Marangoni, Trends Food Sci. Technol., 2016, 57, 59–73 CrossRef.
- A. Diaz-Ruiz, T. Rhinesmith, L. C. D. Pomatto-Watson, N. L. Price, F. Eshaghi, M. R. Ehrlich, J. M. Moats, M. Carpenter, A. Rudderow, S. Brandhorst, J. A. Mattison, M. A. Aon, M. Bernier, V. D. Longo and R. de Cabo, Nat. Commun., 2021, 12, 1–13 CrossRef PubMed.
Footnote |
† Both WR and ANF equally contributed to this work and share common first authorship. |
|
This journal is © The Royal Society of Chemistry 2024 |