Synergistic electrical and light management enables efficient monolithic inorganic perovskite/organic tandem solar cells with over 24% efficiency†
Received
3rd September 2023
, Accepted 26th October 2023
First published on 15th November 2023
Abstract
Monolithic perovskite/organic tandem solar cells (TSCs) have attracted increasing attention on account of their high efficiency and beneficially compatible solution processibility. However, the severe recombination loss restricts the efficiency improvement of perovskite/organic TSCs. Herein, a synergistic electrical and light management strategy via optimizing the interface contact in the front wide band gap (WBG) inorganic perovskite subcell and regulating the donor/acceptor ratio in the rear narrow band gap (NBG) organic subcell was developed to maximize the voltage output in TSCs. Compared with the commonly used SnO2 electron transport layer (ETL), Cl@MZO ETL exhibits a better energy level, reduced leakage current and strengthened carrier extraction in the front WBG subcell. Through elaborate regulation of the donor/acceptor ratio, the rear organic subcell delivers improved morphology and absorption configuration, thus resulting in a favorable current balance for TSCs. The fabricated inorganic perovskite/organic TSCs exhibit a remarkable power conversion efficiency (PCE) of 24.07% with an ultrahigh open-circuit voltage of 2.15 V. Moreover, the unencapsulated TSCs present excellent stability under air conditions.
Broader context
Monolithic perovskite/organic tandem solar cells (TSCs) exhibit promising advantages for efficient next-generation photovoltaics on account of their significant capability of breaking the Shockley–Queisser limit and flexible device integration. Despite the rapid progress, the large voltage deficit still limits efficiency and requires further improvement. To address this issue, we developed a synergistic electrical and light management strategy to enhance the carrier transfer in the front wide-band gap (WBG) inorganic perovskite subcell and optimize the current balance in the rear narrow-band gap (NBG) organic subcell. The electrical loss was significantly suppressed by the introduction of an energy-level-optimized Cl@MZO electron transport layer, thereby inducing efficient charge extraction and reduced voltage loss in the front WBG inorganic perovskite subcell. Regulating the donor and acceptor ratio in the rear NBG organic subcell not only optimizes the morphology to guarantee efficient exciton generation and separation under filtered illumination but also strengthens the absorption configuration for giving a better current balance between two subcells. Benefiting from the contribution of synergistic electrical and light regulation, an over 24% efficiency with a high voltage of 2.15 V was achieved for monolithic inorganic perovskite/organic TSCs, which is one of the best results reported on perovskite/organic TSCs.
|
Introduction
In the past decade, single junction lead halide perovskite solar cells (PSCs) have progressed enormously with a high certified power conversion efficiency (PCE) of 26.1%, approaching the theoretical Shockley–Queisser (S–Q) limit of 33.7%.1–4 The existence of thermal loss and transmission loss will greatly limit the final efficiency of single junction PSCs.5 Tandem solar cells (TSCs) composed of wide band gap (WBG) and narrow band gap (NBG) subcells can maximally utilize sunlight and break the S–Q limit by minimizing thermal losses.6,7 Perovskite-based TSC PCEs have improved from below 14% to as high as 33.7% within just six years, surpassing the record PCEs of single junction perovskite and Si solar cells.8,9 Based on the dual considerations of efficiency and cost, perovskite-based TSCs will undoubtedly become an important pillar of the future photovoltaic industry.10
Various types of perovskite-based TSCs have been developed, such as perovskite/silicon, perovskite/perovskite, and perovskite/organic.11 Among them, monolithic perovskite/organic TSCs have attracted increasing attention due to their potential for manufacture on flexible substrates using simple solution-based manufacturing processes.12,13 However, these perovskite/organic TSCs usually exhibit a severe energy loss (Eloss), and as a result, their PCEs are far behind those of their thin-film tandem counterparts.14 The notable Eloss primarily originates from the following factors: the defect-induced non-radiative recombination and because electrical loss caused inefficient carrier transfer and unbalanced current match between the two subcells.15,16 To address these issues, several strategies including crystallization optimization, composition regulation, additive engineering and interface modification have been developed to regulate the perovskite properties thereby increasing the PCE of PSCs.17–21 Hou and co-workers recently demonstrated that the sputtered thin indium zinc oxide (IZO) provides better electrical properties with a high-surface coverage (∼96%) of BCP than the conventionally used 1 nm Ag, which provides island-like clusters with much lower coverage (∼54%), contributing to suppressing the recombination, facilitating the carrier transfer and realizing lower voltage loss.22 Riedl et al. developed a ternary system (PM6:Y6:PCBM) of the photoactive layer to enhance the absorption in the range of λ > 650 nm of an NBG organic subcell, resulting in a record PCE of 24.0% (with a certified value of 23.1%) for perovskite/organic TSCs.23 However, these high-efficiency perovskite/organic TSCs are mainly based on perovskites consisting of organic cations, which undergo rapid phase degradation under high temperatures and severe phase separation under long-term illumination.24,25 Replacing the organic cations with stable inorganic ions (e.g., Cs and Rb) to form inorganic perovskites could strengthen the phase stability on account of their strong ionic bond energy and stable chemical properties.26 Therefore, selecting suitable compositions of two subcells and reducing the electrical and light recombination is still challenging for achieving high-efficiency inorganic perovskite/organic TSCs with suppressive voltage loss.27,28
In this work, we developed a synergistic electrical and light management strategy to increase efficiency and maximize the open-circuit voltage (Voc) in inorganic perovskite/organic TSCs. Compared with the commonly used SnO2 electron transport layer (ETL), the Cl@MZO ETL improves the electron transfer efficiency and reduces energy loss. The perovskite film deposited on Cl@MZO ETL shows fewer defects and a more orientated crystal structure. Given the current balance between the two subcells, the D/A ratio and thickness of the PM6:BTP-eC9 blended film were optimized to regulate the absorption configuration of rear NBG organic solar cells (OSCs). Under the best optimization, the CsPbI2Br/PM6:BTP-eC9-based all inorganic perovskite/organic TSCs exhibited the best efficiency of 24.07% and Voc of 2.15 V. After 500 hours of storage in an air environment (<15% RH), the unencapsulated device maintains 93% of initial efficiency.
Results and discussion
For the front subcell, the wide-band gap inorganic CsPbI2Br perovskite (∼1.91 eV) was selected as the absorber layer and the device (Fig. 1a) was constructed with an architecture of ITO/ETL/CsPbI2Br/PM6/MoO3/Ag. In order to suppress the Voc deficit, a new electron transport layer (ETL) of Cl@MZO was synthesized to replace the commonly used SnO2 ETL. From X-ray photoelectron spectroscopy (XPS, Fig. S1, ESI†), the elements of Mg and Cl were effectively doped in the ZnO crystal lattice, showing the successful synthesis of Cl@MZO. A series of physico-chemical characterization techniques were then performed to deeply investigate the Cl@MZO properties and their influence on the perovskite. From the ultraviolet photoelectron spectroscopy (UPS) measurements (Fig. 1b), the calculated Fermi energy levels of SnO2 and Cl@MZO are 4.31 eV and 4.02 eV, respectively. By combining the Tauc plot results (Fig. S2, ESI†), the parameters of the energy levels were calculated and are schematically depicted in Fig. 1c. Cl@MZO ETL exhibits a smaller Fermi energy level difference to CsPbI2Br in contrast to the SnO2 ETL, providing a beneficial potential for strengthening carrier extraction ability and reducing large energy loss. The influences of different ETLs on CsPbI2Br crystalline properties were explored by X-ray diffraction (XRD) characterization (Fig. 1d). Two CsPbI2Br films exhibit a similar diffraction configuration with strong (100), (110) and (200) peaks located at 14.73°, 20.9° and 29.66°, demonstrating a favorable accordance with the standard α-CsPbI2Br phase. The diffraction intensity of the CsPbI2Br/Cl@MZO sample is significantly higher than that of the CsPbI2Br/SnO2 sample, indicating the formation of a highly crystalline film with fewer defects. The CsPbI2Br/Cl@MZO sample shows larger peak intensity ratios of (100)/(110) and (200)/(110) (Fig. 1e), indicating that Cl@MZO ETL can induce a preferred growth along the (100) and (200) planes. The formed crystal orientation perpendicular to the ETL substrate is beneficial for facilitating charge transport.29,30 The surface morphology of ETLs and CsPbI2Br films is characterized by scanning electron microscopy (SEM) in Fig. 1f–g. Obviously, some pores (in the yellow area) and agminated particles (in the red area) were observed for the SnO2 ETL, whereas the Cl@MZO film showed uniform and pin-hole-free properties. The grain size of CsPbI2Br/SnO2 is relatively small and obvious defects can be observed at the grain boundaries, which would interfere with the carrier transfer and induce excrescent non-radiative recombination, ultimately leading to a decrease of Voc output and final device performance. In contrast, the CsPbI2Br film on Cl@MZO presents compact, large and uniform grains. Such a dense and flat surface ensures reduced defects. Based on previous reports, substrate wettability has a strong influence on the perovskite nucleation rate and grain growth kinetics.31,32 To investigate the effects of different ETL surface wettability on the perovskite layer, we measured the water contact angles (Fig. S3, ESI†). Cl@MZO ETL shows a large contact angle of 54.1° vs. 33.2° for the SnO2 ETL. Increasing the hydrophobicity usually reduces wetting of the precursor solutions (i.e., less drag force) and suppresses the nucleation rate resulting in continuous pinhole-free coverage of larger crystal grains (as shown in SEM in Fig. 1f and g).33–35 As shown in the cross-sectional SEM images, the CsPbI2Br/Cl@MZO sample shows large grains penetrating the ETL and HTL, which is beneficial for suppressing the non-radiative recombination due to reduced boundaries. Atomic force microscopy (AFM) (Fig. S4, ESI†) was further carried out to reveal the topographical morphology difference between two perovskite films. CsPbI2Br on Cl@MZO presents a significantly reduced roughness of 11.2 nm vs. 19.1 nm of the SnO2 sample. These results demonstrate that the newly prepared Cl@MZO ETL has significantly promising potential for suppressing the non-radiative recombination and reducing the Voc deficit for CsPbI2Br PSCs.
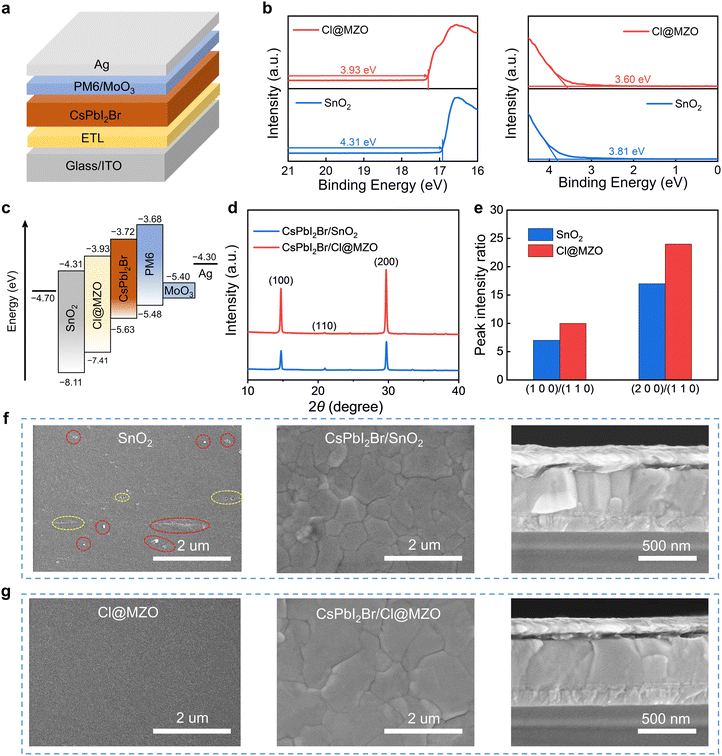 |
| Fig. 1 Characterization of Cl@MZO effects on CsPbI2Br films. (a) Schematic of the device architecture, (b) UPS spectra of SnO2 and Cl@MZO, (c) schematic energy level diagram, (d) XRD patterns, and (e) a diffraction peak intensity ratio; top-views and cross-sectional SEM images of (f) SnO2/CsPbI2Br and (g) Cl@MZO/CsPbI2Br films. | |
The steady-state photoluminescence (PL) spectra of pure CsPbI2Br films and two different ETL-based perovskite films are shown in Fig. 2a. There is no obvious variation in emission peak position (located at about 650 nm) for different samples. The peak intensity of the perovskite films deposited on the ETL is significantly lower than that of pure CsPbI2Br film. The Cl@MZO-based CsPbI2Br sample presents the lowest emission peak, indicating a more effective carrier transport process. In time-resolved PL (TRPL, Fig. 2b) spectra, the curves were fitted according to the double exponential function. The CsPbI2Br/Cl@MZO sample shows a reduced carrier lifetime of 10.5 ns vs. 17.9 ns of CsPbI2Br/SnO2. The shorter lifetime suggests a more efficient carrier extraction and transport process, which is beneficial for suppressing energy loss and boosting device performance. The space charge limited current (SCLC) measurements were conducted by constructing an electron-only structured device of ITO/ETL/perovskite/PCBM/Au (Fig. 2c).
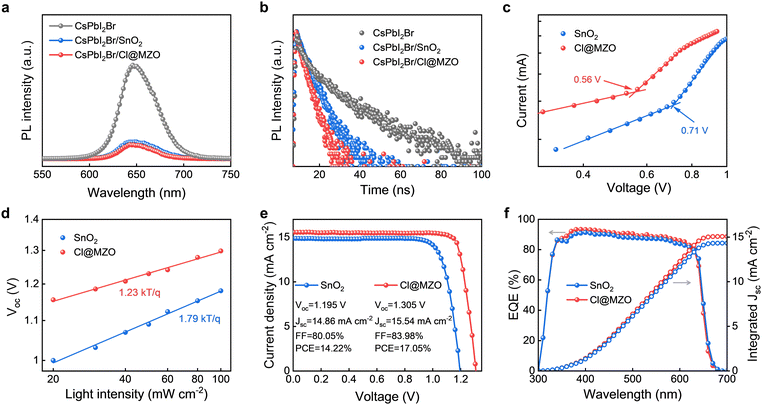 |
| Fig. 2 Optical property characterization and device performance. (a) Steady-state and (b) time-resolved PL spectra, (c) SCLC measurements, (d) relationship between Voc and Plight, (e) J–V curves and (f) EQE spectra of CsPbI2Br PSCs. | |
The trap density was calculated according to the following equation:36
where
ε0,
ε,
VTFL,
e, and
L are the permittivity of vacuum, relative dielectric constant, trap-filled limit voltage, elementary charge, and thickness of the perovskite films, respectively.
37 The
VTFL of the Cl@MZO sample exhibited a lower value (0.56 V) than the control sample (0.71 V). Based on the
VTFL results, the device with Cl@MZO ETL has a lower electron defect density of 0.91 × 10
16 cm
−3. An ideal solar cell has a shunt resistance (
Rsh) much greater than its series resistance (
Rs), and the photocurrent (
Jph) is equal to the dark current (
Jdark).
38 In Fig. S5 (ESI
†), the Cl@MZO-based device shows lower
Jdark for achieving higher
Voc. The light-intensity-dependent
Voc measurements were performed to analyze the recombination mechanism, in which the
n value is usually used to reflect the trap-assisted recombination loss. The
n value of 1 indicates the absence of trap-assisted recombination, while
n > 1 indicates the presence of trap-assisted recombination. As shown in
Fig. 2d, the
n values of the PSCs based on SnO
2 and Cl@MZO ETL are 1.79 and 1.23, indicating that the Cl@MZO-based device exhibits a lower trap-assisted recombination loss. In light-intensity-dependent
Jsc (Fig. S6, ESI
†), the exponent
α reflects the degree of bimolecular recombination. The
α value of 1 indicates no bimolecular recombination loss, while deviation from 1 (<1) indicates the presence of bimolecular recombination loss.
39 The
α values of the devices based on SnO
2 and Cl@MZO ETLs are 0.91 and 0.95, respectively, suggesting that Cl@MZO is beneficial for suppressing the bimolecular recombination loss. PSCs with an architecture of ITO/ETL/CsPbI
2Br/
PM6/MoO
3/Ag were further fabricated to detect the ETL effect on device performance. The
J–V curves of CsPbI
2Br PSCs based on different ETLs are shown in
Fig. 2e (Fig. S7, ESI
†). The PCE of the SnO
2-based PSC is 14.22% with a
Voc of 1.195 V. As expected, the Cl@MZO-based PSC obtained a significantly high PCE of 17.05% with a
Voc of 1.305 V. The integrated
Jsc values of the two PSCs from the EQE spectra match well with the
Jsc measured from
J–V and curves (
Fig. 2f), which proves the accuracy of the photovoltaic test results. As shown in Fig. S8 (ESI
†), Cl@MZO-based PSCs present an improved average PCE of 16.98% from 12.65% for SnO
2-based PSCs. The average
Voc increases from 1.19 V to 1.30 V, indicating a significant decrease of
Voc deficit. Moreover, the PSC with Cl@MZO ETL delivers better stability in the air due to suppressive defects and strengthened interface (Fig. S9, ESI
†).
We found that BTP-eC9 has more extended absorption and better efficiency in the long wavelength range by comparing the absorption spectra and photovoltaic performance of the receptor materials, making it more advantageous as a post-junction active layer material for all inorganic perovskite/organic stacked solar cells. (Fig. S10 and S11, ESI†). Therefore, for the rear NBG organic subcell, the donor of PM6 and the receptor of BTP-eC9 (Fig. 3a) were selected as active light-absorbing materials. As shown in Fig. 3b, there is a strong relationship between the absorption configurations (e.g., intensity and range) and D/A mass ratio of the blended film. With the increase of BTP-eC9 content in the blended film (Fig. 3b), the absorption intensity gradually weakens in the visible light range (∼below 680 nm), whereas the absorption intensity gradually increases in the near-infrared region (over ∼ 810 nm). Moreover, a slight red shift of the overall absorption spectrum was observed with BTP-eC9 content increase, indicating that an increase in receptor content is beneficial for broadening the absorption spectrum to obtain more light absorption. The morphology of the light absorption layer in OSCs plays an important role in determining the exciton dissociation state and influencing the continuous interpenetrating network of two blended active materials, which is the key for efficient solar cells.40 The topographical images of PM6:BTP-eC9 blend films with different D/A ratios are shown in Fig. 3c. It can be seen that the films with 1
:
1.2 and 1
:
1.8 D/A ratios show a smooth surface, which is favorable for exciton dissociation and charge transfer. When the D/A ratio increases to 1
:
2.4, the obviously bright and dark regions indicate severe phase separation in the film, thus affecting the exciton dissociation of the film. In the TSCs, the visible light is firstly absorbed by the front subcell, which leads to the illumination condition of the rear subcell being different from the standard AM 1.5G light. Then, we tested the photovoltaic properties of the PM6:BTP-eC9-based OSCs under light filtered by the front WBG subcell. Different from the photovoltaic performance under standard illumination (Fig. S12 and Table S1, ESI†), with the enhancement of acceptor content from 1.2 to 1.8, both Voc and Jsc slightly increase (Fig. 3d), resulting in an improved PCE of 19.17% (D
:
A = 1
:
1.8). The EQE spectra measured under filtered illumination (Fig. 3e) testify to the current enhancement.
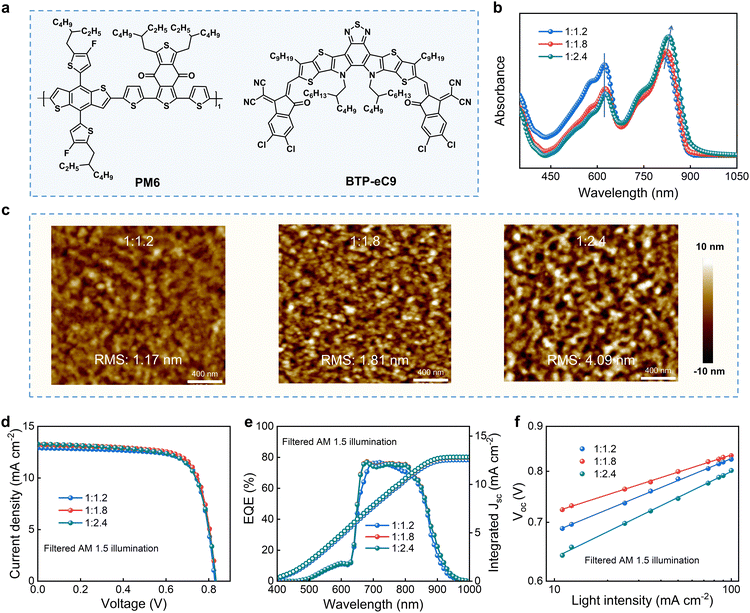 |
| Fig. 3 Characterization of D/A ratio effect. Chemical structures of (a) PM6 and BTP-eC9, (b) UV-vis absorption spectra, (c) AFM images of blended films with different D/A ratios, (d) J–V curves and (e) EQE spectra and (f) relationships of Voc and light intensities of OSCs with different ratios measured under filtered illumination. | |
In order to further investigate the effect of BTP-eC9 content on photon capture and exciton utilization in single junction devices, the Jph–Veff curves of different devices were calculated. As shown in Fig. S13 (ESI†), the probabilities of exciton dissociation (Pdiss) of single junction organic devices based on PM6:BTP-eC9 thin films with D/A ratios of 1
:
1.2, 1
:
1.8, and 1
:
2.4 are 94.2%, 92.0%, and 89.5%, respectively. This indicates that the exciton dissociation efficiency in single junction devices gradually decreases with the increase of BTP-eC9 content in the mixed film. However, the light intensity dependence tested under filtered light has different results (Fig. 3f). The n values of TSCs with 1
:
1.2, 1
:
1.8, and 1
:
2.4 D/A ratios are 1.38, 1.09, and 1.65, respectively, confirming that the photoactive layer with 1
:
1.8 D/A ratio is the best composition for obtaining an efficient rear NBG subcell. These results suggest that regulating the D/A ratio is an effective strategy to optimize the OSC performance under filtered illumination.
Two-terminal TSCs were constructed with an architecture of ITO/Cl@MZO/CsPbI2Br/PM6/MoO3/Ag/PFN-Br/PM6:BTP-eC9/MoO3/Ag (Fig. S14, ESI†). From the cross-sectional SEM image (Fig. S15, ESI†), the front and rear subcells were perfectly stacked by each layer. Optical transfer matrix formalism simulation models were established to analyze the light field distribution (Fig. 4a–c). The front CsPbI2Br subcell mainly absorbs the visible photons from 300 to 680 nm, while the rear organic subcell absorbs the near-infrared photons from 680 to 950 nm. This is consistent with the results obtained from the EQE curve. Compared with the other D/A ratios, the active layer with a D/A ratio of 1
:
1.8 exhibits the strongest photon absorption in the range from 680 to 950 nm, leading to an improvement of Jsc. Fig. 4d shows the J–V curves of TSCs with different D/A ratios. The device using the PM6
:
BTP-eC9 (1
:
1.8) thin film achieves the best PCE of 22.35% with a Jsc of 13.02 mA cm−2, a Voc of 2.11 V and an FF of 81.36%. Compared with other D/A ratios, the TSCs with PM6
:
BTP-eC9 (1
:
1.8) exhibit higher Jsc owing to optimized light absorption regulation. Under the optimization (Fig. S16 and Table S2 and S3, ESI†), the thicknesses of the active layers of the front and rear subcells are 400 nm and 150 nm, respectively. As shown in Fig. 4e, PM6
:
BTP-eC9 (1
:
1.8)-based TSCs present a higher average PCE of 23.02%. In relationships of Voc and light intensities (Fig. 4f), the n values of D/A ratios of 1
:
1.2-, 1
:
1.8-, and 1
:
2.4-based devices are 1.91, 1.48, and 1.82, respectively. The smallest n value of TSCs with a D/A ratio of 1
:
1.8 suggests that the trap-induced recombination of the complete tandem device is effectively suppressed.
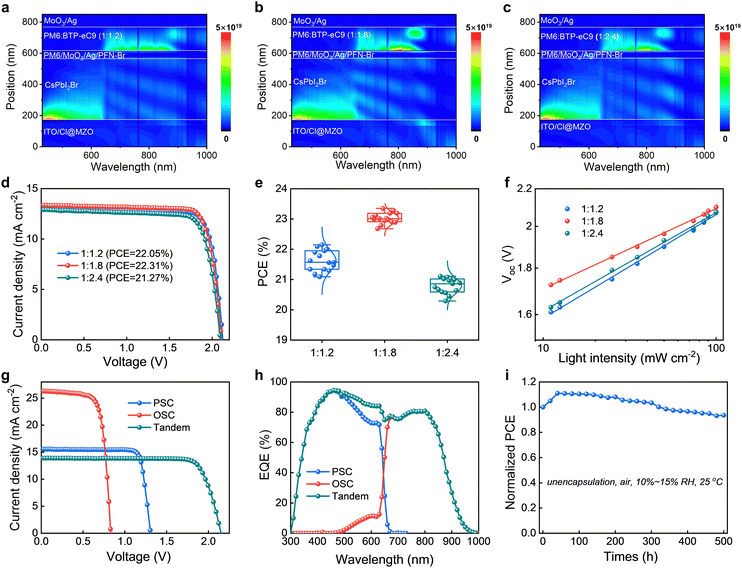 |
| Fig. 4 Photovoltaic performance of TSCs. The charge generation rate distribution spectra in tandem devices based on PM6:BTP-eC9 films with D/A ratios of (a) 1 : 1.2, (b) 1 : 1.8 and (c) 1 : 2.4, (d) J–V curves, (e) the box-line statistics of PCEs and (f) relationships of Voc and light intensities of TSCs based on different ratios of donor and acceptor, (g) J–V curves of the champion TSC, (h) EQE spectra, and (i) long-term stability test of unencapsulated TSCs. | |
Fig. 4g shows the best J–V curves of the TSC and the corresponding front WBG inorganic perovskite subcell and organic subcell (Fig. S17, Table S4, ESI†). The champion TSC achieved a high PCE of 24.07% and a steady-state output efficiency of 23.09% at the maximum power point (Fig. S18–S21, ESI†), which is one of the best results for such inorganic perovskite/organic TSCs (Table S5, ESI†). From the EQE spectrum (Fig. 4h), the integrated Jsc values of the front and rear subcells are 13.49 mA cm−2 and 13.47 mA cm−2, respectively, indicating a favorable Jsc match between the two subcells. In addition, the unencapsulated TSCs also exhibit excellent stability by maintaining 93% of initial efficiency after 500 hours of storage in air below 15% RH (Fig. 4i and Fig. S22, ESI†).
Conclusions
In summary, we have developed a synergistic strategy by reducing the electrical loss and optimizing the current balance to fabricate high-efficiency monolithic inorganic perovskite/organic TSCs. The newly-synthesized Cl@MZO ETL presents better carrier extraction and transfer ability to minimize the Voc deficit in the front CsPbI2Br subcell, resulting in a high PCE of 17.05% for the CsPbI2Br/PM6 device. By fine regulation of the ratio and thickness of the light absorption layer in NBG OSCs, the TSCs obtain a beneficial current balance between front and rear subcells. Under the holistic optimization, the monolithic inorganic perovskite/organic TSCs achieved a champion PCE of 24.07%, which is one of the best results for these types of solar cells. Moreover, the unencapsulated device delivers excellent humidity stability after 500 hours of storage in low-humidity air. This work provides an effective strategy to prepare efficient and stable monolithic inorganic perovskite/organic TSCs.
Author contributions
S. J. and Z. T. conceived the idea. S. J. and R. W. conducted the experiments and carried out characterization. R. Y. and F. W. analysed the data. S. J., R. W. and M. L. wrote the manuscript. Z. T. supervised this project. All the authors discussed the results and commented on the manuscript.
Conflicts of interest
There are no conflicts to declare.
Acknowledgements
Shan Jiang and Ruyue Wang contributed equally to this work. The authors would like to thank financial support from the National Natural Science Foundation of China (52373169, 22379010, 22109166, and 21835006), and the Fundamental Research Funds for the Central Universities (QNTD20).
References
- X. Wu, Y. Z. Liu, F. Qi, F. Lin, H. T. Fu, K. Jiang, S. F. Wu, L. Y. Bi, D. Wang, F. Xu, A. K. Y. Jen and Z. L. Zhu, J. Mater. Chem. A, 2021, 9, 19778–19787 RSC.
- J. Park, J. Kim, H. S. Yun, M. J. Paik, E. Noh, H. J. Mun, M. G. Kim, T. J. Shin and S. I. Seok, Nature, 2023, 616, 724–730 CrossRef CAS.
- K. Yoshikawa, H. Kawasaki, W. Yoshida, T. Irie, K. Konishi, K. Nakano, T. Uto, D. Adachi, M. Kanematsu, H. Uzu and K. Yamamoto, Nat. Energy, 2017, 2, 17032 CrossRef CAS.
- Best research-cell efficiency chart, https://www.nrel.gov/pv/cell-efficiency.html.
- Y. Ding, Q. Guo, Y. Geng, Z. Dai, Z. Wang, Z. Chen, Q. Guo, Z. Zheng, Y. Li and E. Zhou, Nano Today, 2022, 46, 101586 CrossRef CAS.
- S. K. Xie, R. X. Xia, Z. Chen, J. J. Tian, L. Yan, M. R. Ren, Z. C. Li, G. C. Zhang, Q. F. Xue, H. L. Yip and Y. Cao, Nano Energy, 2020, 78, 105238 CrossRef CAS.
- R. Lin, K. Xiao, Z. Qin, Q. Han, C. Zhang, M. Wei, M. I. Saidaminov, Y. Gao, J. Xu, M. Xiao, A. Li, J. Zhu, E. H. Sargent and H. Tan, Nat. Energy, 2019, 4, 864–873 CrossRef CAS.
- L. P. Duan, D. Walter, N. T. Chang, J. Bullock, D. Kang, S. P. Phang, K. Weber, T. White, D. Macdonald, K. Catchpole and H. P. Shen, Nat. Rev. Mater., 2023, 8, 261–281 CrossRef CAS.
- Best research-cell efficiency chart, https://www.nrel.gov/pv/cell-efficiency.html.
- H. Li and W. Zhang, Chem. Rev., 2020, 120, 9835–9950 CrossRef CAS.
- Y.-M. Xie, Q. Yao, Z. Zeng, Q. Xue, T. Niu, R. Xia, Y. Cheng, F. Lin, S.-W. Tsang, A. K. Y. Jen, H.-L. Yip and Y. Cao, Adv. Funct. Mater., 2022, 32, 2112126 CrossRef CAS.
- X. Chen, Z. Y. Jia, Z. Chen, T. M. Jiang, L. Z. Bai, F. Tao, J. W. Chen, X. Y. Chen, T. Y. Liu, X. H. Xu, C. Y. Yang, W. D. Shen, W. E. I. Sha, H. M. Zhu and Y. Yang, Joule, 2020, 4, 1594–1606 CrossRef CAS.
- Q. Zeng, L. Liu, Z. Xiao, F. Liu, Y. Hua, Y. Yuan and L. Ding, Sci. Bull., 2019, 64, 885–887 CrossRef.
- H. Aqoma, I. F. Imran, F. T. A. Wibowo, N. V. Krishna, W. Lee, A. K. Sarker, D. Ryu and S. Y. Jang, Adv. Energy Mater., 2020, 10, 2001188 CrossRef CAS.
- R. He, S. Q. Ren, C. Chen, Z. J. Yi, Y. Luo, H. G. Lai, W. W. Wang, G. G. Zeng, X. Hao, Y. Wang, J. Q. Zhang, C. L. Wang, L. L. Wu, F. Fu and D. W. Zhao, Energy Environ. Sci., 2021, 14, 5723–5759 RSC.
- S. Qin, C. Lu, Z. Jia, Y. Wang, S. Li, W. Lai, P. Shi, R. Wang, C. Zhu, J. Du, J. Zhang, L. Meng and Y. Li, Adv. Mater., 2022, 34, 2108829 CrossRef CAS.
- S. S. Chen, X. Z. Dai, S. Xu, H. Y. Jiao, L. Zhao and J. S. Huang, Science, 2021, 373, 902–907 CrossRef CAS PubMed.
- S. G. Ji, I. J. Park, H. Chang, J. H. Park, G. P. Hong, B. K. Choi, J. H. Jang, Y. J. Choi, H. W. Lim, Y. J. Ahn, S. J. Park, K. T. Nam, T. Hyeon, J. Park, D. H. Kim and J. Y. Kim, Joule, 2022, 6, 2390–2405 CrossRef CAS.
- C. L. Wang, Y. Zhao, T. S. Ma, Y. D. An, R. He, J. W. Zhu, C. Chen, S. Q. Ren, F. Fu, D. W. Zhao and X. F. Li, Nat. Energy, 2022, 7, 744–753 CrossRef CAS.
- W. Hou, G. Han, T. Ou, Y. Xiao and Q. Chen, Angew. Chem., Int. Ed., 2020, 59, 21409–21413 CrossRef CAS.
- L. Zhu, X. Zhang, M. Li, X. Shang, K. Lei, B. Zhang, C. Chen, S. Zheng, H. Song and J. Chen, Adv. Energy. Mater., 2021, 11, 2100529 CrossRef CAS.
- W. Chen, Y. D. Zhu, J. W. Xiu, G. C. Chen, H. M. Liang, S. C. Liu, H. S. Xue, E. Birgersson, J. W. Ho, X. S. Qin, J. Y. Lin, R. J. Ma, T. Liu, Y. L. He, A. M. C. Ng, X. G. Guo, Z. B. He, H. Yan, A. B. Djurisic and Y. Hou, Nat. Energy, 2022, 7, 229–237 CrossRef CAS.
- K. O. Brinkmann, T. Becker, F. Zimmermann, C. Kreusel, T. Gahlmann, M. Theisen, T. Haeger, S. Olthof, C. Tuckmantel, M. Gunster, T. Maschwitz, F. Gobelsmann, C. Koch, D. Hertel, P. Caprioglio, F. Pena-Camargo, L. Perdigon-Toro, A. Al-Ashouri, L. Merten, A. Hinderhofer, L. Gomell, S. Zhang, F. Schreiber, S. Albrecht, K. Meerholz, D. Neher, M. Stolterfoht and T. Riedl, Nature, 2022, 604, 280 CrossRef CAS.
- R. J. Sutton, G. E. Eperon, L. Miranda, E. S. Parrott, B. A. Kamino, J. B. Patel, M. T. Hörantner, M. B. Johnston, A. A. Haghighirad, D. T. Moore and H. J. Snaith, Adv. Energy Mater., 2016, 6, 1502458 CrossRef.
- J. J. Tian, K. C. Zhang, Z. Q. Xie, Z. J. Peng, J. Y. Zhang, A. Osvet, L. Luer, T. Kirchartz, U. Rau, N. Li and C. J. Brabec, ACS Energy Lett., 2022, 7, 4071–4080 CrossRef CAS.
- E. L. Lim, J. X. Yang and Z. H. Wei, Energy Environ. Sci., 2023, 16, 862–888 RSC.
- Y. M. Xie, Q. Yao, H. L. Yip and Y. Cao, Small Methods, 2023, 7, 2201225 CrossRef.
- L. Liu, H. Xiao, K. Jin, Z. Xiao, X. Du, K. Yan, F. Hao, Q. Bao, C. Yi, F. Liu, W. Wang, C. Zuo and L. Ding, Nano-Micro Lett., 2022, 15 DOI:10.1007/s40820-022-00995-2.
- Z. Xu, X. Liu, S. Fu, J. Wang, J. Zhang, L. Huang, Z. Hu and Y. Zhu, Appl. Phys. Lett., 2021, 119, 212101 CrossRef CAS.
- X. Qiu, Y. Xu, R. Li, Y. Jing, Z. Yan, F. Liu, L. Wu, Y. Tu, J. Shi, Z. Du, J. Wu and Z. Lan, Small, 2023, 19, 2206245 CrossRef CAS PubMed.
- C. Gong, C. Zhang, Q. Zhuang, H. Li, H. Yang, J. Chen and Z. Zang, Nano-Micro Lett., 2022, 15, 1 Search PubMed.
- C. Bi, Q. Wang, Y. Shao, Y. Yuan, Z. Xiao and J. Huang, Nat. Commun., 2015, 6, 7747 CrossRef CAS PubMed.
- S. Wang, H. Chen, J. Zhang, G. Xu, W. Chen, R. Xue, M. Zhang, Y. Li and Y. Li, Adv. Mater., 2019, 31, 1903691 CrossRef CAS.
- R. Azmi, C. L. Lee, I. H. Jung and S. Y. Jang, Adv. Energy Mater., 2018, 8, 1701683 CrossRef.
- R. Azmi, W. T. Hadmojo, S. Sinaga, C. L. Lee, S. C. Yoon, I. H. Jung and S. Y. Jang, Adv. Energy Mater., 2017, 8, 1702934 CrossRef.
- D. Yang, R. Yang, K. Wang, C. Wu, X. Zhu, J. Feng, X. Ren, G. Fang, S. Priya and S. F. Liu, Nat. Commun., 2018, 9, 3239 CrossRef.
- Y. Wu, Q. Zhang, L. Fan, C. Liu, M. Wu, D. Wang and T. Zhang, ACS Appl. Energy Mater., 2021, 4, 5583–5589 CrossRef CAS.
- J. C. Yu, S. Badgujar, E. D. Jung, V. K. Singh, D. W. Kim, J. Gierschner, E. Lee, Y. S. Kim, S. Cho, M. S. Kwon and M. H. Song, Adv. Mater., 2019, 31, 1805554 CrossRef.
- T. Lee, Y. Eom, C. E. Song, I. H. Jung, D. Kim, S. K. Lee, W. S. Shin and E. Lim, Adv. Energy Mater., 2019, 9, 1804021 CrossRef.
- D. Xia, Z. Zhang, C. Zhao, J. Wang, J. Xia, G. Chen, S. Li, Z. Tang, S. You and W. Li, J. Colloid Interface Sci., 2021, 601, 70–77 CrossRef CAS PubMed.
|
This journal is © The Royal Society of Chemistry 2024 |