Electron beam-induced demetallation of Fe, Co, Ni, Cu, Zn, Pd, and Pt metalloporphyrins: insights in e-beam chemistry and metal cluster formations†
Received
30th November 2023
, Accepted 30th January 2024
First published on 31st January 2024
Abstract
Electron beams are versatile tools for nanoscale fabrication processes, however, the underlying e-beam chemistry remains in its infancy. Through operando transmission electron microscopy investigations, we elucidate a redox-driven cargo release of individual metal atoms triggered by electron beams. The chosen organic delivery molecule, tetraphenylporphyrin (TPP), proves highly versatile, forming complexes with nearly all metals from the periodic table and being easily processed in solution. A comprehensive cinematographic analysis of the dynamics of single metal atoms confirms the nearly instantaneous ejection of complexed metal atoms under an 80 kV electron beam, underscoring the system's broad versatility. Providing mechanistic insights, we employ density functional theory to support the proposed reductive demetallation pathway facilitated by secondary electrons, contributing novel perspectives to electron beam-mediated chemical reaction mechanisms. Lastly, our findings demonstrate that all seven metals investigated form nanoclusters once ejected from TPP, highlighting the method's potential for studying and developing sustainable single-atom and nanocluster catalysts.
Introduction
Recently, we published findings on the unexpected electron beam-induced demetallation of custom-made metallobenzoporphyrins deposited on graphene as a substrate.1 While previous studies reported on the use of heavy transition metal contrast markers in molecular imaging by high-angle annular dark-field scanning transmission electron microscopy (HAADF-STEM),2–6 our operando transmission electron microscopy (TEM) observations on metallobenzoporphyrins suggested that the metal atoms exhibited deficient complexation behavior by the macrocycle, once subjected to the electron beam (e-beam). This, in turn, is an opportunity for analyzing single metal atoms.
The investigation of single metal atom dynamics, encompassing their diffusion behavior on different substrates and the propensity to form larger aggregates and clusters, holds significant importance for various nanotechnological applications.7,8 For instance, understanding the deactivation pathways of single-atom catalysts (SACs) is crucial for designing durable and sustainable catalysis solutions.8,9 Likewise, in nanoelectronics, precise control over each atom's state is essential when they are implemented in single-atom transistors (SATs) for the fabrication of electronic quantum circuits.10,11
Apart from computational analysis of atom–substrate interactions, in situ TEM and respective single-molecule atomic-resolution time-resolved electron microscopy (SMART-EM) techniques stand out as powerful experimental tools for investigating ad-atom dynamics.12–14 One way of depositing single metal atoms on substrates is using organic cargo-delivery molecules. As exemplified by Sinha et al., Gd-atoms can be deposited on graphene by drop-casting a solution of Gd3N@C80 endohedral metallofullerenes (EMFs), followed by the release of the metal atoms onto the substrate upon annealing to 900 °C.15 While this cargo methodology is elegant, its limitation to only a few and expensive EMFs poses a challenge.
In this work, we present a generalized approach for the redox-driven cargo release of solution-based single-metal-atom deposition on graphene. Therefore, we employed the widely accessible tetraphenylporphyrin (TPP) as a redox-active macrocyclic ligand for transition metals, which upon e-beam irradiation, releases the complexed metal atoms and subsequently form metal clusters (Scheme 1). By studying seven different metalloporphyrins (Fe, Co, Ni, Cu, Zn, Pd, and Pt), we elucidate herein the e-beam chemistry of metalloporphyrins through operando (scanning) transmission electron microscopy, complemented by energy-dispersive X-ray spectroscopy (EDS) and density functional theory (DFT).
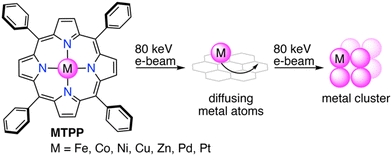 |
| Scheme 1 Redox-driven cargo release of single metal atoms from metalloporphyrins and formation of metal clusters. | |
Experimental
Synthesis of metallotetraphenylporphyrins
Chemicals were purchased from Sigma-Aldrich and used without any further purification. CH2Cl2 was neutralized with K2CO3 and distilled prior to usage. Reactions were carried out in darkness and under a dry argon atmosphere using standard Schlenk techniques. Reaction vessels were heated with polymer-coated heat-on blocks. All synthetic and structural characterizations are described in detail in the ESI.† Briefly, the MTPPs were synthesized from TPP, as shown in Fig. 1a, following the protocols described below. Absorption spectra of the synthesized MTPPs are shown in Fig. 1b.
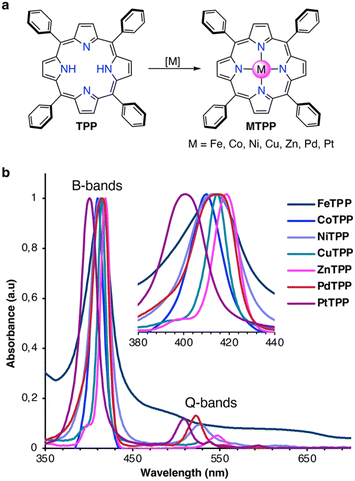 |
| Fig. 1 (a) Synthesis of MTPPs from TPP. (b) Normalized UV/Vis absorption spectra of MTPPs in CH2Cl2 at rt. | |
FeTPP
.
TPP (100 mg, 163 μmol) and FeCl2 (309 mg, 2.45 mmol) were dissolved in DMF (40 mL) and brought to reflux for 5 h. The mixture was plug filtered (SiO2; MeOH/Hex/EtOAc (1
:
1
:
1)) and concentrated. The crude was purified by column chromatography (SiO2; EtOAc/Hex (1
:
1)) and precipitated from MeOH with H2O to give the product as a dark-brown colored solid in 64% (70 mg, 100 μmol) yield.
CoTPP
.
TPP (100 mg, 163 μmol) and Co(OAc)2·2H2O (288 mg, 1.63 mmol) were dissolved in THF (50 mL), and the mixture was brought to reflux for 20 h. The mixture was plug filtered (SiO2; CH2Cl2/Hex (1
:
1)) and precipitated from CH2Cl2 with MeOH to give the product as a burgundy-colored solid in 57% (62 mg, 93.0 μmol) yield.
NiTPP
.
TPP (50 mg, 81.3 μmol) and Ni(acac)2 (209 mg, 813 μmol) were dissolved in toluene (25 mL) and brought to reflux for 24 h. The mixture was plug-filtered (SiO2; toluene), concentrated, and precipitated from toluene with MeOH. The solid was filtered, washed with MeOH, and dried in vacuum to give the product as a purple solid in 46% (25 mg, 37.2 μmol) yield.
CuTPP
.
TPP (50 mg, 81.3 μmol) dissolved in CH2Cl2 (50 mL) was charged with a saturated solution of Cu(OAc)2·H2O in MeOH (5 mL) and stirred for 20 h at rt. The mixture was plug filtered (SiO2; CH2Cl2), concentrated, and purified by column chromatography (SiO2; CH2Cl2/Hex (1
:
1)). The product was precipitated from CH2Cl2 with MeOH to give a red-colored solid in 97% (53 mg, 79 μmol) yield.
ZnTPP
.
TPP (60 mg, 98.0 μmol) dissolved in CH2Cl2 (50 mL) was charged with a saturated solution of Zn(OAc)2 in MeOH (5 mL) and stirred for 20 h at rt. The mixture was plug filtered (SiO2; CH2Cl2), concentrated, and purified by column chromatography (SiO2; CH2Cl2/Hex (1
:
1)). The product was precipitated from CH2Cl2 with MeOH to give a pink-colored solid in 72% (48 mg, 71 μmol) yield.
PdTPP
.
PdCl2 (289 mg, 1.63 mmol) was dissolved in benzonitrile (25 mL) and brought to reflux for 30 min. TPP (100 mg, 163 μmol) was added as a solid, and the mixture was brought to reflux for 6 h. The solvent was distilled off, the mixture was plug-filtered (SiO2; toluene), concentrated, and precipitated from toluene with MeOH. The solid was filtered, washed with MeOH, and dried in vacuum to yield the product as a red solid in 70% (82 mg, 114 μmol) yield.
PtTPP
.
Pt(acac)2 (641 mg, 1.63 mmol) was dissolved in benzonitrile (25 mL) and brought to reflux for 30 min. TPP (100 mg, 163 μmol) was added as a solid, and the mixture was brought to reflux for 6 h. The solvent was distilled off, the mixture was plug-filtered (SiO2; toluene), concentrated, and precipitated from toluene with MeOH. The solid was filtered, washed with MeOH, and dried in vacuum to yield the product as a red solid in 60% (80 mg, 97.8 μmol) yield.
Graphene grid preparation
Graphene was synthesized via chemical vapor deposition (CVD) on 25 μm Cu foils (99.8%, Alfa Aesar).16 An illustration of the graphene transfer steps onto TEM grids is presented in Fig. S1 in the ESI.† Briefly, graphene on the back side of the Cu foil was etched by O2 plasma in the reactive-ion etching (RIE) mode (CUTE, Femto Science Co.) (power = 70 W, flow rate = 20 sccm). Polymethyl methacrylate (PMMA) solution (PMMA-950 K A4, MicroChem Corp.) was spin-coated on graphene/Cu foils at 3000 rpm for 1 min and dried in air. The copper foil was etched with an aqueous 2 mM ammonium persulfate [(NH4)2S2O8] solution for 24 h. The PMMA/graphene stacks were rinsed three times in deionized water. The stacks were scooped onto Quantifoil holey carbon TEM grids (R2/2 400 mesh Gold, SPI Supplies Inc.) and dried in air for 24 h. To enhance the adhesion between graphene and the carbon film of the TEM grids, PMMA/graphene/grids were heated at 150 °C for 10 min. Finally, the PMMA film was dissolved with acetone.17
(S)TEM analysis and data processing
Atomic-resolution operando electron microscopy was carried out on a cold FEG JEOL JEM-ARM200F instrument equipped with a double Cs aberration corrector and double JED-2300T energy-dispersive X-ray spectrometer at an acceleration voltage of 80 kV and a vacuum of 1 × 10−5 Pa in the TEM column. TEM, STEM, and EDS elemental mapping experiments were carried out on a reinforced beryllium double tilt holder (JEOL EM-01360RSTHB) at rt. TEM videos were recorded on a CMOS camera (Gatan OneView-IS, 2048 × 2048 pixels) operated in binning 1 mode (output image size 2048 × 2048 pixels, with a pixel resolution of 0.01 nm at ×2
000
000), an exposure time of 40 ms, and a frame rate of 25 fps. All images were automatically processed using Gatan DigitalMicrograph software. HAADF-STEM camera lengths of 6 and 8 cm (with detection angles ranging from 90 to 370 mrad and 68 to 280 mrad, respectively) were used. The probe size was 6 and 7C (120 and 60 pA, respectively), and the condenser lens (CL) aperture size was 40 μm. The probe convergence angle was between 28 to 33 mrad. Images were collected in the .dm4 format on Gatan DigitalMicrograph software and processed using ImageJ 1.54f software.18 Images were subjected to thermal drift correction, and Gaussian blurred before analysis. Trajectories of single metal atoms and clusters were tracked and analyzed with TrackMate as implemented in Fiji.19,20 Electron diffraction pattern was simulated using the SingleCrystal software (CrystalMaker Software Ltd). TEM simulation images were generated using a multi-slice procedure implemented in Bionet elbis software.21 Simulation parameters were set to agree with the actual experimental parameters: acc. volt. = 80.00 kV, λ = 0.04176 Å, Cs = −0.00490 mm, df = 129.49 Å, Cc = 0.25 mm, de = 0.43 eV, α = 0.20 mrad, OL aperture radius = 20.88 mrad, OL aperture radius = 0.50 Å−1, pixel size = 0.1089 Å, max. intens. = 1.5165.
DFT calculations
Geometry optimization and energy calculations were performed on Q-Chem as implemented in the SPARTAN ‘20 work package.22–24 Structures were first optimized using the semi-empirical method PM6,25 followed by the Head Gordon range-separated global hybrid generalized gradient approximation density functional ωB97X-D with Grimme D2 dispersion correction,26,27 using the Pople-type double-ζ split-valence basis set 6-31G(d).28–30 To reduce calculation costs, the meso-phenyl rings were replaced by hydrogen atoms to represent metalloporphins (MPs). Single-point calculations were obtained at the triple-ζ 6-311+G(2df,2p) level of theory.31,32 Ionization energies were obtained from the frozen geometry of the neutral molecule in the respective ionization state, whereby possible, the electronic closed-shell configurations were considered. Threshold displacement energies Ed were determined at the ωB97X-D/6-311G(2d,p)//B3LYP/6-31G(d) level of theory. Ed was obtained from the Morse potential calculations for the gradual extension over 20 steps of the respective N4–M bonds from equilibrium distance to a length of 7 Å. The intersection of the slopes for the binding potential (equilibrium −2.8 Å) and the non-binding potential (4–7 Å) resulted in the determined threshold energies for the homolytic bond scissions at different redox states.1,17
Results and discussion
Diffusion of single metal atoms
For this generalization study of the redox-driven cargo release of single metal atoms, we decided to investigate the catalytically active group ten elements Ni, Pd, and Pt, as well as the neighboring transition metals from the fourth period, Fe, Co, Cu, and Zn, to have a comprehensive view on the goings-on under the e-beam. We drop-casted 10 μL of a 1.5 mM solution of the MTPPs in tetrahydrofuran (THF) (Fig. 2a) on the freshly prepared graphene grids, and excess of the solution was removed with a blotting paper as illustrated in Fig. 2b. The grids were then dried in vacuum (10−3 mbar) before being subjected to TEM analysis. Compared to previously published metal atom deposition techniques, such as thermal evaporation,33 e-beam deposition,34 soft-landing deposition,35 atomic layer deposition,36 chemical vapor deposition,37 or magnetron sputtering,38 our solution-based deposition is a highly versatile and easily accessible method that does not require any additional equipment.
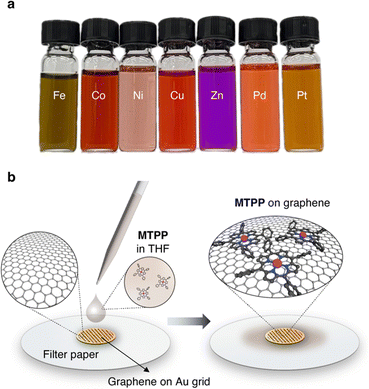 |
| Fig. 2 TEM Sample preparation. (a) Image of the used 1.5 mM solutions of MTPPs in THF. (b) Illustration of the solution-based deposition of MTPPs on the graphene grid. | |
We initiated our investigation with PtTPP, which features the Pt in the +II oxidation state. Upon examining the substrate for pristine graphene regions at high magnifications (×2
000
000) with a moderate electron flux of ∼10−6 e− nm−2 s−1 for SMART-EM studies,39–44 we discovered omnipresent single Pt atoms. As highlighted in the time-dependent image sequence of two Pt atoms in Fig. 3a (Video S1, ESI†), dynamic behavior for both atoms was observed, as evidenced by the diffusion trajectories. Hereby, Pt atom 1, which was situated on the graphene monolayer at the edge of an amorphous carbon layer showed an increased translational mobility compared to Pt atom 2, which was embedded in the amorphous carbon layer and only moving due to dynamic changes of the layer (Fig. 3b). The omnipresent amorphous carbon islands on the graphene surface are residues from the graphene grid fabrication (compare also Fig. S2 in the ESI†).45–47
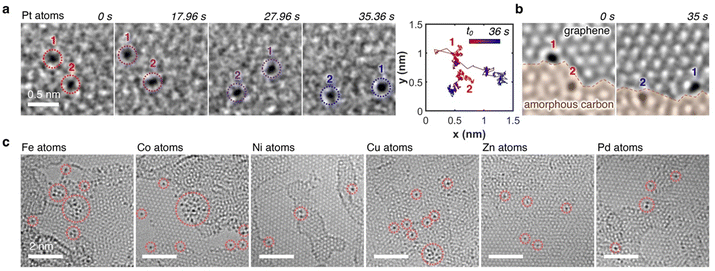 |
| Fig. 3 Released single metal atoms. (a) Time-dependent sequence of two Pt atoms. The coloration of the metal atoms corresponds to the time stamp from the respective trajectories. (b) Images of stacked sequence (25 frames corresponding to 1 s exposure time), highlighting the location of each atom. Atom 1 is located on the graphene monolayer at the edge of an amorphous carbon layer; atom 2 is embedded within the amorphous carbon layer. (c) Overview images of remaining single metal atoms (Fe, Co, Ni, Cu, Zn, Pd) released by the e-beam. | |
This dynamic behavior of single metal atoms was also observed when other MTPPs (M = Fe, Co, Ni, Cu, Zn, and Pd) were used, as summarized in Fig. 3c, unequivocally indicating a successful demetallation of all MTPPs under the electron beam. Notably, all metals within the MTPPs are present in the +II oxidation state; only in the case of FeTPP does the iron carry a fifth ligand (chloro) and is, therefore, in the +III oxidation state. Nonetheless, Fe is equally well demetallated compared to the +II metals. The dynamics of the metal atoms align with previous in situ TEM studies on single metal atoms,7,48–50 confirming that the demetallation pathway utilized herein yields comparable results to previously published deposition methods.
Regarding general chemical demetallation strategies of porphyrins, ZnTPP is routinely demetallated under wet-chemical conditions with mild acids, which makes demetallation induced by diffusing H+ atoms, in principle, plausible. On the other hand, derivatives like NiTPP exhibit a high resistance to acid-mediated demetallation and necessitate alternative strategies, such as reductive demetallation with Grignard reagents.51–53 This observation provides valuable insights into the demetallation mechanisms (vide infra). Further, at the employed electron flux, a metal release due to critical fragmentations of the meso or α-carbons in the TPP ligand is earliest expected to proceed after ∼30 s; however, the metal atoms were immediately detected upon scanning the surface, rendering a fragmentation-induced metal release less likely (vide infra).1
Theoretical evaluation
To rationalize the mechanism of the observed demetallation, we considered beam-molecule interactions based on elastic and inelastic scattering events.54 In the case of elastic scattering, the electrons are deflected from their trajectory through attractive Coulomb interactions between the positively charged nucleus and the beam electrons. During this process, momentum is transferred to the nucleus, which causes a translation of the atom from its equilibrium position. As a consequence, the atoms in the molecules vibrate and are thus lifted into vibronically excited states. If the transferred kinetic energy exceeds the threshold displacement energy Ed of the atom in the molecule, this vibronic excitation can terminate in a homolytic bond cleavage and a fragmentation of the molecule occurs.55 Herein, we will denote the non-destructive e-beam-induced vibronic excitation of molecular bonds, induced by elastic scattering, as ‘knock-on excitation’, whereas the destructive bond cleavage will be termed ‘knock-on displacement’.
The transferred kinetic energy ET to the nucleus can be calculated according to eqn (1), where E0 is the intrinsic kinetic energy of the electron, A is the atomic mass number, and θ is the scattering angle.56
| 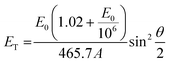 | (1) |
At a scattering angle of 180°, the transferred energy is at its maximum (
Emax). If
ET exceeds
Ed, knock-on displacement can occur. As indicated in
Fig. 4, only light elements, including H, C, and N, can receive enough momentum to result in a bond cleavage. All investigated metals do not receive enough momentum to be displaced from the molecule. While a scattering angle of only 23° is necessary to displace hydrogen from
MTPP, it should be noted that the cross-section for this event is relatively low 5.2 × 10
−6a02 sr
−1 (carbon: 5.1 × 10
−7a02 sr
−1; nitrogen: 2.5 × 10
−7a02 sr
−1; compare Fig. S3 in the ESI
†).
57 Nonetheless, C–H bond cleavages are expected to occur after prolonged irradiation. These fragmented hydrogen atoms can have a high enough kinetic energy to be transferred to the atoms, denoted as
EH-flux.
40 As listed in
Table 1,
EH-flux is high enough to remove most metals from the porphyrins; however, it is not large enough to demetallate
PdTPP and
PtTPP. Additionally, the probability of this cascade reaction occurring is substantially reduced as opposed to electron-driven processes and, therefore, is unlikely to be the main driving force for the rapid demetallation process.
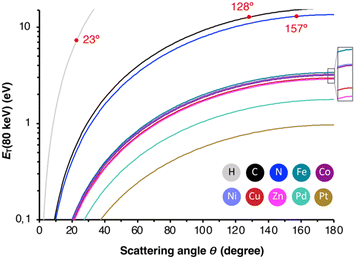 |
| Fig. 4 Transferred energy ET against the elastic scattering angle Θ for all studied elements. In red are the angles indicated, at which ET exceeds Ed. | |
Table 1 Summaries on calculated energies involved during elastic scattering processes
Element |
E
max (80 keV)/eV |
E
H-flux/eV |
E
d/eV |
σ
1KO (80 keV)/barn |
H |
187.5 |
187.5 |
7.73 |
66 |
C |
15.73 |
53.57 |
12.70 |
20 |
N |
13.49 |
46.97 |
12.95 |
4.5 |
Fe |
3.38 |
13.06 |
10.91 |
0 |
Co |
3.21 |
12.40 |
9.50 |
0 |
Ni |
3.22 |
12.45 |
10.23 |
0 |
Cu |
2.97 |
11.53 |
7.24 |
0 |
Zn |
2.89 |
11.21 |
6.80 |
0 |
Pd |
1.78 |
6.97 |
8.08 |
0 |
Pt |
0.97 |
3.84 |
10.38 |
0 |
Alternatively, inelastic scattering events, which stem from electron–electron interactions, where the beam-electron donates a substantial amount of energy to the electrons of the molecules, were considered. The probability for inelastic scattering to occur is several magnitudes larger (∼105) than for elastic scattering processes, which renders it likely the initial driving force behind the demetallation.58–60 Thus, we evaluated the oxidation and reduction of metalloporphyrins employing DFT calculations. As summarized in Table 2, the one and two-electron oxidation of metalloporphyrins is energetically demanding. In contrast, the one-electron and, to a lesser extent, the two-electron reduction are energetically feasible. Hence, we determined Ed for the N4⋯M bond cleavage, as summarized in Table 1 and depicted in the form of the Morse potentials in Fig. 5a. As shown, ZnP exhibits the weakest metal complexation, whereas FeP, PtP, and NiP exhibit the strongest complexations. These calculations agree well with wet-chemical complexation stabilities, as discussed above. Notably, the complexation affinity is strongly altered upon ionization of the molecule. As exemplified for ZnP in Fig. 5b, reduction to ZnP− and ZnP2− leads to a decrease in binding strength, whereas oxidation to ZnP+ and ZnP2+ has the inverse effect (the remaining Morse potentials are displayed in Fig. S9 in the ESI†). This behavior is well reflected in reports about the wet-chemical reductive demetallation of porphyrins.52,53
Table 2 Vertical ionization energies of MTPPs calculated at the wB97X-D/6-311+G(2df,2p) level of theory
Metal |
ΔEionization/eV |
[MTPP]2+ |
[MTPP]+ |
[MTPP]− |
[MTPP]2− |
Fe |
17.49 |
7.97 |
−2.77 |
1.05 |
Co |
17.44 |
9.51 |
−0.92 |
1.54 |
Ni |
18.10 |
6.90 |
−0.78 |
1.46 |
Cu |
18.33 |
7.94 |
−0.67 |
1.65 |
Zn |
17.58 |
6.73 |
−1.28 |
1.32 |
Pd |
17.80 |
6.83 |
−1.26 |
1.33 |
Pt |
17.89 |
6.87 |
−1.20 |
1.38 |
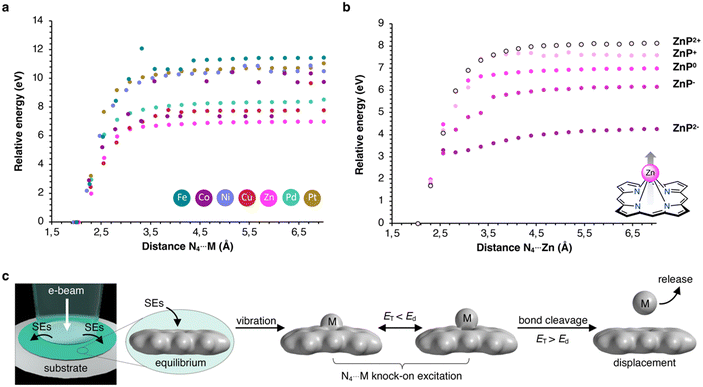 |
| Fig. 5 Evaluation of the homolytic bond cleavage of the N4⋯M bond in metalloporphins MPs. (a) Morse potential curves for all MPs in the neutral state. (b) Exemplified Morse potential of ZnP in different ionization states (−2, −1, 0, +1, +2). (c) Proposed demetallation mechanism induced by secondary electrons (SEs). Electron density maps of ZnP models at increasing bond length are calculated at the ωB97X-D/6-311G(2d,p) level of theory; iso-value of electron density shows a 95% electron probability (0.0164 e− a.u.−3). | |
Therefore, we propose that the demetallation is driven by omnipresent secondary electrons (SEs), which can reduce metalloporphyrins through electron attachment. Subsequent vibronic excitations, enhanced by elastic scattering, lead to the rapid ejection of the complexed metal atoms, as depicted in Fig. 5c (further mechanistic explanations are depicted in Fig. S8 in the ESI†). This pathway explains why metal atoms are already found to be displaced when screening the surface without being exposed to prolonged e-beams, as SEs are ejected into the periphery of the primary electron beam (beam shadow).1 At the same time, this effect is enhanced in thicker samples as more SEs are generated. Consequently, using (S)TEM for molecular structure determination, using a low electron flux, thin samples to reduce the formation of SEs, and a conductive specimen to facilitate charge recombination processes, is critical.5
Formation and dynamics of metal clusters
In regions characterized by a high concentration of metal atoms, a tendency towards cluster formation under the e-beam in TEM mode was evident. Fig. 6a (Video S2, ESI†) illustrates three amorphous Pt clusters, each approximately 1 nm in size, maintaining mobility despite their dimensions. 60 s after t0, two of the three clusters coalesced. 28 s later, this fused cluster approached the remaining one, culminating into a single amorphous cluster. Positioned on an amorphous carbon layer, these clusters traversed a distance of about 3 nm over a 100 s duration, converging into a single amorphous Pt cluster, as depicted in the trajectory plot in Fig. 6a. Even in a crystalline state, metal atoms exhibited mobility under electron beam irradiation, which support the formation of flexible metal clusters rather than rigid metal carbides,61,62 as exemplified by an image sequence of a Ni cluster in Fig. 6b (Video S3, ESI†). Adopting a body-centered cubic (BCC) packing unit, the Ni atoms continually rearranged themselves in response to the electron beam. Notably, the small Ni cluster stabilized in the BCC packing due to its high surface-to-volume ratio, whereas larger crystals usually favor a face-centered cubic (FCC) motif.63,64 The metastable BCC crystal packing persisted throughout the video sequence, with only the crystal's shape and orientation on the substrate evolving over time.
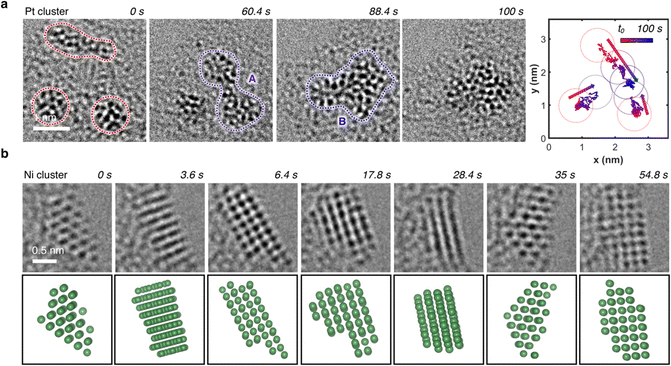 |
| Fig. 6 TEM mode analysis of metal cluster dynamics. (a) Merging of three amorphous Pt clusters over a time period of 100 s. The moment of initial coalescence is indicated by (A) and the final by (B). (b) Atomistic rearrangement in a crystalline Ni cluster in the BCC packing over a time period of 55 s. | |
To discern whether the electron beam in STEM mode also induced dynamic atomistic changes, we investigated various areas with metal clusters, as exemplified by Ni clusters in Fig. 7a. Highlighted in the green area, at t0, the two clusters were distinctly separated by approximately 2 nm. Under continuous scanning e-beam irradiation over 11 minutes, both clusters translated on the surface and merged into a single crystalline Ni cluster. Examination of the cluster highlighted in the green box in Fig. 7b revealed a Ni crystal exhibiting 0.2 nm interatomic distances. Further analysis of the Fast Fourrier Transform (FFT) pattern correlates with the peak (011) along the [100] plane, which agrees well with the simulated diffraction pattern for Ni's BCC structure. Ultimately, as evidenced by HAADF-STEM and EDS mapping images in Fig. 7c, cluster formations were observed for all seven metals investigated herein, underscoring the general applicability of MTPPs as redox-driven cargo delivery systems for the generation of nanoclusters under the electron beam.
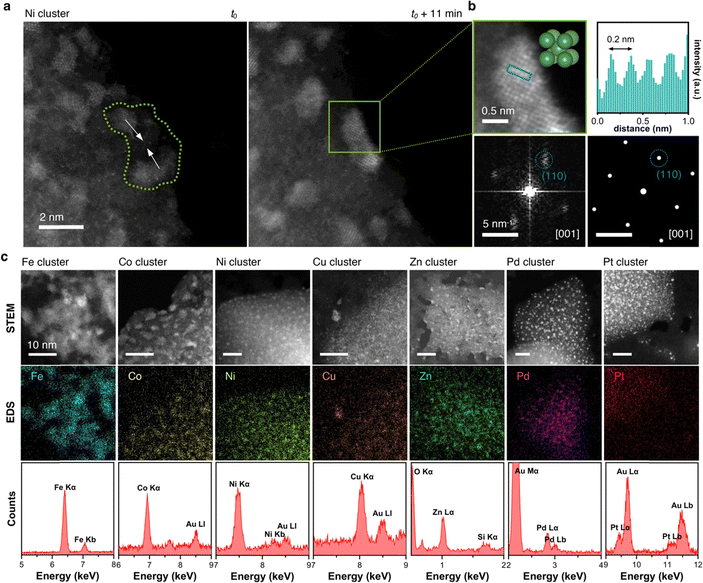 |
| Fig. 7 STEM mode analysis of metal clusters. (a) HAADF-STEM images of the coalescing crystalline Ni clusters upon irradiating the area in STEM mode for 11 min. (b) Magnified HAADF-STEM image of the BCC Ni crystal, the measured plot profile and experimental FFT (left) and simulated (right) diffraction pattern. (c) HAADF-STEM images and EDS mapping of all investigated metals (Fe, Co, Ni, Cu, Zn, Pd, Pt) and clusters. | |
Conclusions
In this work, we present a straightforward and widely applicable approach for the solution-based deposition and electron beam-triggered liberation of individual metal atoms onto carbon substrates. Significantly, we employ readily available organic cargo-delivery systems, specifically tetraphenylporphyrins, which form complexes with a broad spectrum of transition and main group metals, imparting exceptional versatility to these molecules. Through operando transmission electron microscopy, we illustrate the characteristic surface mobility of the metal atoms, indicating their release from the molecular complexes. Density functional theory calculations support our observation that the rapid metal release is attributed to a reductive demetallation pathway induced by secondary electrons rather than physical fragmentation of the macrocycle through knock-on displacement. As a consequence, decreasing the acceleration voltage will increase the secondary electron formation and subsequently increase the demetallation process. This mechanistic insight contributes to the understanding of electron beam-initiated chemical reaction mechanisms, an area that remains largely unexplored.17,59,60,65
Notably, our method exhibits high versatility by operating at room temperature without the necessity for annealing, hydrogenation, or any pre-treatment. Thus, through redox-driven cargo release, we demonstrate a methodology for e-beam-synthesized metallic nanoclusters for diverse applications, such as catalysis. Furthermore, the facile implementation of heteroatoms on the substrate, such as nitrogen, can be envisioned through additional molecular engineering of the porphyrin's periphery.66–70 Incorporating heteroatom-rich substituents allows for precise modulation of material properties, potentially serving as dopants or anchor points within the substrate, thereby offering a means to fine-tune the materials as needed.
Author contributions
D. L. conceived the project and supervised it with J. C. J. P. carried out electron microscopy experiments and data analysis with the help of S. L. S. L. prepared the graphene grids. O. F. J. synthesized and characterized the metalloporphyrins. D. L. wrote the manuscript with the help of J. P., S. L., and O. F. J. All authors discussed the results.
Conflicts of interest
There are no conflicts to declare.
Acknowledgements
This research was supported by the Institute for Basic Science (IBS-R026-Y1, IBS-R026-D1). Professor Eiichi Nakamura and Professor Koji Harano (University of Tokyo) are thanked for their valuable discussions of the involved mechanisms and for supporting initial SMART-EM experiments.
Notes and references
- H. Hoelzel, S. Lee, K. Y. Amsharov, N. Jux, K. Harano, E. Nakamura and D. Lungerich, Time-resolved imaging and analysis of the electron beam-induced formation of an open-cage metallo-azafullerene, Nat. Chem., 2023, 15, 1444–1451 CrossRef CAS PubMed
.
- M. A. Gerkman, S. Sinha, J. H. Warner and G. G. D. Han, Direct Imaging of Photoswitching Molecular Conformations Using Individual Metal Atom Markers, ACS Nano, 2019, 13, 87–96 CrossRef CAS PubMed
.
- E. S. Kengmana, J. K. Lee, X. Li, J. H. Warner and G. G. D. Han, Self-Assembly of Bowlic Supramolecules on Graphene Imaged at the Individual Molecular Level using Heavy Atom Tagging, Small, 2020, 16, e2002860 CrossRef PubMed
.
- E. Kano, M. Takeguchi, J. Fujita and A. Hashimoto, Direct observation of Pt-terminating carbyne on graphene, Carbon, 2014, 80, 382–386 CrossRef CAS
.
- P. Kharel, B. E. Janicek, S. Hyun Bae, A. L. Loutris, P. T. Carmichael and P. Y. Huang, Atomic-Resolution Imaging of Small Organic Molecules on Graphene, Nano Lett., 2022, 22, 3628–3635 CrossRef CAS PubMed
.
- J. K. Lee, I. Bulut, M. Rickhaus, Y. Sheng, X. Li, G. G. D. Han, G. A. D. Briggs, H. L. Anderson and J. H. Warner, Metal Atom Markers for Imaging Epitaxial Molecular Self-Assembly on Graphene by Scanning Transmission Electron Microscopy, ACS Nano, 2019, 13, 7252–7260 CrossRef CAS PubMed
.
- I. Popov, S. Ghaderzadeh, E. C. Kohlrausch, L. T. Norman, T. J. A. Slater, G. N. Aliev, H. Alhabeadi, A. Kaplan, W. Theis, A. N. Khlobystov, J. A. Fernandes and E. Besley, Chemical Kinetics of Metal Single Atom and Nanocluster Formation on Surfaces: An Example of Pt on Hexagonal Boron Nitride, Nano Lett., 2023, 23, 8006–8012 CrossRef CAS PubMed
.
- I. H. Kim, J. Lim and S. O. Kim, Discovery of Single-Atom Catalyst: Customized Heteroelement Dopants on Graphene, Acc. Mater. Res., 2021, 2, 394–406 CrossRef CAS
.
- X. He, Y. Deng, Y. Zhang, Q. He, D. Xiao, M. Peng, Y. Zhao, H. Zhang, R. Luo, T. Gan, H. Ji and D. Ma, Mechanochemical Kilogram-Scale Synthesis of Noble Metal Single-Atom Catalysts, Cell Rep. Phys. Sci., 2020, 1, 100004 CrossRef
.
- C. Schirm, M. Matt, F. Pauly, J. C. Cuevas, P. Nielaba and E. Scheer, A current-driven single-atom memory, Nat. Nanotechnol., 2013, 8, 645–648 CrossRef CAS PubMed
.
- M. Fuechsle, J. A. Miwa, S. Mahapatra, H. Ryu, S. Lee, O. Warschkow, L. C. L. Hollenberg, G. Klimeck and M. Y. Simmons, A single-atom transistor, Nat. Nanotechnol., 2012, 7, 242–246 CrossRef CAS PubMed
.
- K. Cao, J. Biskupek, C. T. Stoppiello, R. L. McSweeney, T. W. Chamberlain, Z. Liu, K. Suenaga, S. T. Skowron, E. Besley, A. N. Khlobystov and U. Kaiser, Atomic mechanism of metal crystal nucleus formation in a single-walled carbon nanotube, Nat. Chem., 2020, 12, 921–928 CrossRef CAS PubMed
.
- K. Cao, T. Zoberbier, J. Biskupek, A. Botos, R. L. McSweeney, A. Kurtoglu, C. T. Stoppiello, A. V. Markevich, E. Besley, T. W. Chamberlain, U. Kaiser and A. N. Khlobystov, Comparison of atomic scale dynamics for the middle and late transition metal nanocatalysts, Nat. Commun., 2018, 9, 3382 CrossRef PubMed
.
- K. Cao, S. T. Skowron, J. Biskupek, C. T. Stoppiello, C. Leist, E. Besley, A. N. Khlobystov and U. Kaiser, Imaging an unsupported metal–metal bond in dirhenium molecules at the atomic scale, Sci. Adv., 2020, 6, eaay5849 CrossRef CAS PubMed
.
- S. Sinha, Y. Sheng, I. Griffiths, N. P. Young, S. Zhou, A. I. Kirkland, K. Porfyrakis and J. H. Warner, In Situ Atomic-Level Studies of Gd Atom Release and Migration on Graphene from a Metallofullerene Precursor, ACS Nano, 2018, 12, 10439–10451 CrossRef CAS PubMed
.
- X. Li, W. Cai, J. An, S. Kim, J. Nah, D. Yang, R. Piner, A. Velamakanni, I. Jung, E. Tutuc, S. K. Banerjee, L. Colombo and R. S. Ruoff, Large-Area Synthesis of High-Quality and Uniform Graphene Films on Copper Foils, Science, 2009, 324, 1312–1314 CrossRef CAS PubMed
.
- D. Lungerich, H. Hoelzel, K. Harano and N. Jux, K. Yu. Amsharov and E. Nakamura, A Singular Molecule-to-Molecule Transformation on Video: The Bottom-Up Synthesis of Fullerene C60 from Truxene Derivative C60H30, ACS Nano, 2021, 15, 12804–12814 CrossRef CAS PubMed
.
- C. A. Schneider, W. S. Rasband and K. W. Eliceiri, NIH Image to ImageJ: 25 years of image analysis, Nat. Methods, 2012, 9, 671–675 CrossRef CAS PubMed
.
- J.-Y. Tinevez, N. Perry, J. Schindelin, G. M. Hoopes, G. D. Reynolds, E. Laplantine, S. Y. Bednarek, S. L. Shorte and K. W. Eliceiri, TrackMate: an open and extensible platform for single-particle tracking, Methods, 2017, 115, 80–90 CrossRef CAS PubMed
.
- D. Ershov, M.-S. Phan, J. W. Pylvänäinen, S. U. Rigaud, L. L. Blanc, A. Charles-Orszag, J. R. W. Conway, R. F. Laine, N. H. Roy, D. Bonazzi, G. Duménil, G. Jacquemet and J.-Y. Tinevez, TrackMate 7: integrating state-of-the-art segmentation algorithms into tracking pipelines, Nat. Methods, 2022, 19, 829–832 CrossRef CAS PubMed
.
- F. Hosokawa, T. Shinkawa, Y. Arai and T. Sannomiya, Benchmark test of accelerated multi-slice simulation by GPGPU, Ultramicroscopy, 2015, 158, 56–64 CrossRef CAS PubMed
.
- E. Epifanovsky, A. T. B. Gilbert, X. Feng, J. Lee, Y. Mao, N. Mardirossian, P. Pokhilko, A. F. White, M. P. Coons, A. L. Dempwolff, Z. Gan, D. Hait, P. R. Horn, L. D. Jacobson, I. Kaliman, J. Kussmann, A. W. Lange, K. U. Lao, D. S. Levine, J. Liu, S. C. McKenzie, A. F. Morrison, K. D. Nanda, F. Plasser, D. R. Rehn, M. L. Vidal, Z.-Q. You, Y. Zhu, B. Alam, B. J. Albrecht, A. Aldossary, E. Alguire, J. H. Andersen, V. Athavale, D. Barton, K. Begam, A. Behn, N. Bellonzi, Y. A. Bernard, E. J. Berquist, H. G. A. Burton, A. Carreras, K. Carter-Fenk, R. Chakraborty, A. D. Chien, K. D. Closser, V. Cofer-Shabica, S. Dasgupta, M. de Wergifosse, J. Deng, M. Diedenhofen, H. Do, S. Ehlert, P.-T. Fang, S. Fatehi, Q. Feng, T. Friedhoff, J. Gayvert, Q. Ge, G. Gidofalvi, M. Goldey, J. Gomes, C. E. González-Espinoza, S. Gulania, A. O. Gunina, M. W. D. Hanson-Heine, P. H. P. Harbach, A. Hauser, M. F. Herbst, M. H. Vera, M. Hodecker, Z. C. Holden, S. Houck, X. Huang, K. Hui, B. C. Huynh, M. Ivanov, Á. Jász, H. Ji, H. Jiang, B. Kaduk, S. Kähler, K. Khistyaev, J. Kim, G. Kis, P. Klunzinger, Z. Koczor-Benda, J. H. Koh, D. Kosenkov, L. Koulias, T. Kowalczyk, C. M. Krauter, K. Kue, A. Kunitsa, T. Kus, I. Ladjánszki, A. Landau, K. V. Lawler, D. Lefrancois, S. Lehtola, R. R. Li, Y.-P. Li, J. Liang, M. Liebenthal, H.-H. Lin, Y.-S. Lin, F. Liu, K.-Y. Liu, M. Loipersberger, A. Luenser, A. Manjanath, P. Manohar, E. Mansoor, S. F. Manzer, S.-P. Mao, A. V. Marenich, T. Markovich, S. Mason, S. A. Maurer, P. F. McLaughlin, M. F. S. J. Menger, J.-M. Mewes, S. A. Mewes, P. Morgante, J. W. Mullinax, K. J. Oosterbaan, G. Paran, A. C. Paul, S. K. Paul, F. Pavošević, Z. Pei, S. Prager, E. I. Proynov, Á. Rák, E. Ramos-Cordoba, B. Rana, A. E. Rask, A. Rettig, R. M. Richard, F. Rob, E. Rossomme, T. Scheele, M. Scheurer, M. Schneider, N. Sergueev, S. M. Sharada, W. Skomorowski, D. W. Small, C. J. Stein, Y.-C. Su, E. J. Sundstrom, Z. Tao, J. Thirman, G. J. Tornai, T. Tsuchimochi, N. M. Tubman, S. P. Veccham, O. Vydrov, J. Wenzel, J. Witte, A. Yamada, K. Yao, S. Yeganeh, S. R. Yost, A. Zech, I. Y. Zhang, X. Zhang, Y. Zhang, D. Zuev, A. Aspuru-Guzik, A. T. Bell, N. A. Besley, K. B. Bravaya, B. R. Brooks, D. Casanova, J.-D. Chai, S. Coriani, C. J. Cramer, G. Cserey, A. E. DePrince, R. A. DiStasio, A. Dreuw, B. D. Dunietz, T. R. Furlani, W. A. Goddard, S. Hammes-Schiffer, T. Head-Gordon, W. J. Hehre, C.-P. Hsu, T.-C. Jagau, Y. Jung, A. Klamt, J. Kong, D. S. Lambrecht, W. Liang, N. J. Mayhall, C. W. McCurdy, J. B. Neaton, C. Ochsenfeld, J. A. Parkhill, R. Peverati, V. A. Rassolov, Y. Shao, L. V. Slipchenko, T. Stauch, R. P. Steele, J. E. Subotnik, A. J. W. Thom, A. Tkatchenko, D. G. Truhlar, T. V. Voorhis, T. A. Wesolowski, K. B. Whaley, H. L. Woodcock, P. M. Zimmerman, S. Faraji, P. M. W. Gill, M. Head-Gordon, J. M. Herbert and A. I. Krylov, Software for the frontiers of quantum chemistry: an overview
of developments in the Q-Chem 5 package, J. Chem. Phys., 2021, 155, 084801 CrossRef CAS PubMed
.
- Y. Shao, Z. Gan, E. Epifanovsky, A. T. B. Gilbert, M. Wormit, J. Kussmann, A. W. Lange, A. Behn, J. Deng, X. Feng, D. Ghosh, M. Goldey, P. R. Horn, L. D. Jacobson, I. Kaliman, R. Z. Khaliullin, T. Kuś, A. Landau, J. Liu, E. I. Proynov, Y. M. Rhee, R. M. Richard, M. A. Rohrdanz, R. P. Steele, E. J. Sundstrom, H. L. Woodcock, P. M. Zimmerman, D. Zuev, B. Albrecht, E. Alguire, B. Austin, G. J. O. Beran, Y. A. Bernard, E. Berquist, K. Brandhorst, K. B. Bravaya, S. T. Brown, D. Casanova, C.-M. Chang, Y. Chen, S. H. Chien, K. D. Closser, D. L. Crittenden, M. Diedenhofen, R. A. DiStasio, H. Do, A. D. Dutoi, R. G. Edgar, S. Fatehi, L. Fusti-Molnar, A. Ghysels, A. Golubeva-Zadorozhnaya, J. Gomes, M. W. D. Hanson-Heine, P. H. P. Harbach, A. W. Hauser, E. G. Hohenstein, Z. C. Holden, T.-C. Jagau, H. Ji, B. Kaduk, K. Khistyaev, J. Kim, J. Kim, R. A. King, P. Klunzinger, D. Kosenkov, T. Kowalczyk, C. M. Krauter, K. U. Lao, A. D. Laurent, K. V. Lawler, S. V. Levchenko, C. Y. Lin, F. Liu, E. Livshits, R. C. Lochan, A. Luenser, P. Manohar, S. F. Manzer, S.-P. Mao, N. Mardirossian, A. V. Marenich, S. A. Maurer, N. J. Mayhall, E. Neuscamman, C. M. Oana, R. Olivares-Amaya, D. P. O’Neill, J. A. Parkhill, T. M. Perrine, R. Peverati, A. Prociuk, D. R. Rehn, E. Rosta, N. J. Russ, S. M. Sharada, S. Sharma, D. W. Small, A. Sodt, T. Stein, D. Stück, Y.-C. Su, A. J. W. Thom, T. Tsuchimochi, V. Vanovschi, L. Vogt, O. Vydrov, T. Wang, M. A. Watson, J. Wenzel, A. White, C. F. Williams, J. Yang, S. Yeganeh, S. R. Yost, Z.-Q. You, I. Y. Zhang, X. Zhang, Y. Zhao, B. R. Brooks, G. K. L. Chan, D. M. Chipman, C. J. Cramer, W. A. Goddard, M. S. Gordon, W. J. Hehre, A. Klamt, H. F. Schaefer, M. W. Schmidt, C. D. Sherrill, D. G. Truhlar, A. Warshel, X. Xu, A. Aspuru-Guzik, R. Baer, A. T. Bell, N. A. Besley, J.-D. Chai, A. Dreuw, B. D. Dunietz, T. R. Furlani, S. R. Gwaltney, C.-P. Hsu, Y. Jung, J. Kong, D. S. Lambrecht, W. Liang, C. Ochsenfeld, V. A. Rassolov, L. V. Slipchenko, J. E. Subotnik, T. V. Voorhis, J. M. Herbert, A. I. Krylov, P. M. W. Gill and M. Head-Gordon, Advances in molecular quantum chemistry contained in the Q-Chem 4 program package, Mol. Phys., 2015, 113, 184–215 CrossRef CAS
.
- Y. Shao, L. F. Molnar, Y. Jung, J. Kussmann, C. Ochsenfeld, S. T. Brown, A. T. B. Gilbert, L. V. Slipchenko, S. V. Levchenko, D. P. O’Neill, R. A. D. Jr, R. C. Lochan, T. Wang, G. J. O. Beran, N. A. Besley, J. M. Herbert, C. Y. Lin, T. V. Voorhis, S. H. Chien, A. Sodt, R. P. Steele, V. A. Rassolov, P. E. Maslen, P. P. Korambath, R. D. Adamson, B. Austin, J. Baker, E. F. C. Byrd, H. Dachsel, R. J. Doerksen, A. Dreuw, B. D. Dunietz, A. D. Dutoi, T. R. Furlani, S. R. Gwaltney, A. Heyden, S. Hirata, C.-P. Hsu, G. Kedziora, R. Z. Khalliulin, P. Klunzinger, A. M. Lee, M. S. Lee, W. Liang, I. Lotan, N. Nair, B. Peters, E. I. Proynov, P. A. Pieniazek, Y. M. Rhee, J. Ritchie, E. Rosta, C. D. Sherrill, A. C. Simmonett, J. E. Subotnik, H. L. W. III, W. Zhang, A. T. Bell, A. K. Chakraborty, D. M. Chipman, F. J. Keil, A. Warshel, W. J. Hehre, H. F. S. III, J. Kong, A. I. Krylov, P. M. W. Gill and M. Head-Gordon, Advances in methods and algorithms in a modern quantum chemistry program package, Phys. Chem. Chem. Phys., 2006, 8, 3172–3191 RSC
.
- J. J. P. Stewart, Optimization of parameters for semiempirical methods V: modification of NDDO approximations and application to 70 elements, J. Mol. Model., 2007, 13, 1173–1213 CrossRef CAS PubMed
.
- J.-D. Chai and M. Head-Gordon, Long-range corrected hybrid density functionals with damped atom–atom dispersion corrections, Phys. Chem. Chem. Phys., 2008, 10, 6615–6620 RSC
.
- Y. Minenkov, Å. Singstad, G. Occhipinti and V. R. Jensen, The accuracy of DFT-optimized geometries of functional transition metal compounds: a validation study of catalysts for olefin metathesis and other reactions in the homogeneous phase, Dalton Trans., 2012, 41, 5526–5541 RSC
.
- V. A. Rassolov, M. A. Ratner, J. A. Pople, P. C. Redfern and L. A. Curtiss, 6-31G* basis set for third-row atoms, J. Comput. Chem., 2001, 22, 976–984 CrossRef CAS
.
- S. G. Wang and W. H. E. Schwarz, Density functional study of first row transition metal dihalides, J. Chem. Phys., 1998, 109, 7252–7262 CrossRef CAS
.
- R. Ditchfield, W. J. Hehre and J. A. Pople, Self-Consistent Molecular-Orbital Methods. IX. An Extended Gaussian-Type Basis for Molecular-Orbital Studies of Organic Molecules, J. Chem. Phys., 1971, 54, 724–728 CrossRef CAS
.
- T. Clark, J. Chandrasekhar, G. W. Spitznagel and P. V. R. Schleyer, Efficient diffuse function-augmented basis sets for anion calculations. III. The 3-21+G basis set for first-row elements, Li–F, J. Comput. Chem., 1983, 4, 294–301 CrossRef CAS
.
- R. Krishnan, J. S. Binkley, R. Seeger and J. A. Pople, Self-consistent molecular orbital methods. XX. A basis set for correlated wave functions, J. Chem. Phys., 1980, 72, 650–654 CrossRef CAS
.
- A. Liu, H. Zhu, T. Zou, Y. Reo, G.-S. Ryu and Y.-Y. Noh, Evaporated nanometer chalcogenide films for scalable high-performance complementary electronics, Nat. Commun., 2022, 13, 6372 CrossRef CAS PubMed
.
- X. Wang, Y. Hu, S. Y. Kim, R. Addou, K. Cho and R. M. Wallace, Origins of Fermi Level Pinning for Ni and Ag Metal Contacts on Tungsten Dichalcogenides, ACS Nano, 2023, 17, 20353–20365 CrossRef CAS PubMed
.
- R. K. Biroju, P. Harrison, W. Theis, N. V. Rees, R. Sharma, T. N. Narayanan and M. G. Hahm, Pt147 Nanoclusters Soft-Landed on WS2 Nanosheets for Catalysis and Energy Harvesting, ACS Appl. Nano Mater., 2021, 4, 13140–13148 CrossRef CAS
.
- M. Kim, S. Nabeya, S.-M. Han, M.-S. Kim, S. Lee, H.-M. Kim, S.-Y. Cho, D.-J. Lee, S.-H. Kim and K.-B. Kim, Selective Atomic Layer Deposition of Metals on Graphene for Transparent Conducting Electrode Application, ACS Appl. Mater. Interfaces, 2020, 12, 14331–14340 CrossRef CAS PubMed
.
- J. Feng, X. Gong, X. Lou and R. G. Gordon, Direct-Liquid-Evaporation Chemical Vapor Deposition of Nanocrystalline Cobalt Metal for Nanoscale Copper Interconnect Encapsulation, ACS Appl. Mater. Interfaces, 2017, 9, 10914–10920 CrossRef CAS PubMed
.
- S. Lee, W. Lee, H.-T. Jung and C. A. Ross, Selective Deposition of Copper on Self-Assembled Block Copolymer Surfaces via Physical Vapor Deposition, ACS Appl. Mater. Interfaces, 2021, 13, 52931–52937 CrossRef CAS PubMed
.
- T. Shimizu, D. Lungerich, J. Stuckner, M. Murayama, K. Harano and E. Nakamura, Real-Time Video Imaging of Mechanical Motions of a Single Molecular Shuttle with Sub-Millisecond Sub-Angstrom Precision, Bull. Chem. Soc. Jpn., 2020, 93, 1079–1085 CrossRef CAS
.
- J. Biskupek, S. T. Skowron, C. T. Stoppiello, G. A. Rance, S. Alom, K. L. Y. Fung, R. J. Whitby, M. H. Levitt, Q. M. Ramasse, U. Kaiser, E. Besley and A. N. Khlobystov, Bond Dissociation and Reactivity of HF and H2O in a Nano Test Tube, ACS Nano, 2020, 14, 11178–11189 CrossRef CAS PubMed
.
- S. Okada, S. Kowashi, L. Schweighauser, K. Yamanouchi, K. Harano and E. Nakamura, Direct Microscopic Analysis of Individual C60 Dimerization Events: Kinetics and Mechanisms, J. Am. Chem. Soc., 2017, 139, 18281–18287 CrossRef CAS PubMed
.
- T. Nakamuro, K. Kamei, K. Sun, J. W. Bode, K. Harano and E. Nakamura, Time-Resolved Atomistic Imaging and Statistical Analysis of Daptomycin Oligomers with and without Calcium Ions, J. Am. Chem. Soc., 2022, 144, 13612–13622 CrossRef CAS PubMed
.
- H. Hanayama, J. Yamada, K. Harano and E. Nakamura, Cyclodextrins as Surfactants for Solubilization and Purification of Carbon Nanohorn Aggregates, Chem. – Asian J., 2020, 15, 1549–1552 CrossRef CAS PubMed
.
- T. Nakamuro, M. Sakakibara, H. Nada, K. Harano and E. Nakamura, Capturing the Moment of Emergence of Crystal Nucleus from Disorder, J. Am. Chem. Soc., 2021, 143, 1763–1767 CrossRef CAS PubMed
.
- K. Jia, J. Zhang, L. Lin, Z. Li, J. Gao, L. Sun, R. Xue, J. Li, N. Kang, Z. Luo, M. H. Rummeli, H. Peng and Z. Liu, Copper-Containing Carbon Feedstock for Growing Superclean Graphene, J. Am. Chem. Soc., 2019, 141, 7670–7674 CrossRef CAS PubMed
.
- X. Li, W. Cai, L. Colombo and R. S. Ruoff, Evolution of Graphene Growth on Ni and Cu by Carbon Isotope Labeling, Nano Lett., 2009, 9, 4268–4272 CrossRef CAS PubMed
.
- J. Robertson, Amorphous carbon, Adv. Phys., 1986, 35, 317–374 CrossRef CAS
.
- J. Zhao, Q. Deng, S. M. Avdoshenko, L. Fu, J. Eckert and M. H. Rümmeli, Direct in situ observations of single Fe atom catalytic processes and anomalous diffusion at graphene edges, Proc. Natl. Acad. Sci. U. S. A., 2014, 111, 15641–15646 CrossRef CAS PubMed
.
- T. Furnival, R. K. Leary, E. C. Tyo, S. Vajda, Q. M. Ramasse, J. M. Thomas, P. D. Bristowe and P. A. Midgley, Anomalous diffusion of single metal atoms on a graphene oxide support, Chem. Phys. Lett., 2017, 683, 370–374 CrossRef CAS
.
- C. Gong, A. W. Robertson, K. He, C. Ford, A. A. R. Watt and J. H. Warner, Interactions of Pb and Te atoms with graphene, Dalton Trans., 2014, 43, 7442–7448 RSC
.
- K. Murakami, Y. Yamamoto, H. Yorimitsu and A. Osuka, Demetalation of Metal Porphyrins via Magnesium Porphyrins by Reaction with Grignard Reagents, Chem. – Eur. J., 2013, 19, 9123–9126 CrossRef CAS PubMed
.
- J. A. Cowan and J. K. M. Sanders, Reductive demetallation of porphyrins: evidence for peripheral and axial modes of reduction, Tetrahedron Lett., 1986, 27, 1201–1204 CrossRef CAS
.
- M. Kumar, P. Neta, T. P. G. Sutter and P. Hambright, One-electron reduction and demetallation of copper porphyrins, J. Phys. Chem., 1992, 96, 9571–9575 CrossRef CAS
.
- T. Susi, J. C. Meyer and J. Kotakoski, Quantifying transmission electron microscopy irradiation effects using two-dimensional materials, Nat. Rev. Phys., 2019, 1, 397–405 CrossRef
.
- S. T. Skowron, T. W. Chamberlain, J. Biskupek, U. Kaiser, E. Besley and A. N. Khlobystov, Chemical Reactions of Molecules Promoted and Simultaneously Imaged by the Electron Beam in Transmission Electron Microscopy, Acc. Chem. Res., 2017, 50, 1797–1807 CrossRef CAS PubMed
.
- R. F. Egerton, P. Li and M. Malac, Radiation damage in the TEM and SEM, Micron, 2004, 35, 399–409 CrossRef CAS PubMed
.
-
A. Jablonski, F. Salvat, C. J. Powell and A. Y. Lee, NIST Electron Elastic-Scattering Cross-Section Database Version 4.0, NIST Standard Reference Database Number 64, National Institute of Standards and Technology, 2016 Search PubMed
.
- R. F. Egerton, Mechanisms of radiation damage in beam-sensitive specimens, for TEM accelerating voltages between 10 and 300 kV, Microsc. Res. Tech., 2012, 75, 1550–1556 CrossRef CAS PubMed
.
- D. Liu, S. Kowashi, T. Nakamuro, D. Lungerich, K. Yamanouchi, K. Harano and E. Nakamura, Ionization and electron excitation of C60 in a carbon nanotube: a variable temperature/voltage transmission electron microscopic study, Proc. Natl. Acad. Sci. U. S. A., 2022, 119, e2200290119 CrossRef CAS PubMed
.
- D. Liu, D. Lungerich, T. Nakamuro, K. Harano and E. Nakamura, Excited state modulation of C70 dimerization in a carbon nanotube under a variable electron acceleration voltage, Micron, 2022, 160, 103316 CrossRef CAS PubMed
.
- A. S. Sinitsa, T. W. Chamberlain, T. Zoberbier, I. V. Lebedeva, A. M. Popov, A. A. Knizhnik, R. L. McSweeney, J. Biskupek, U. Kaiser and A. N. Khlobystov, Formation of Nickel Clusters Wrapped in Carbon Cages: Toward New Endohedral Metallofullerene Synthesis, Nano Lett., 2017, 17, 1082–1089 CrossRef CAS PubMed
.
- I. V. Lebedeva, T. W. Chamberlain, A. M. Popov, A. A. Knizhnik, T. Zoberbier, J. Biskupek, U. Kaiser and A. N. Khlobystov, The atomistic mechanism of carbon nanotube cutting catalyzed by nickel under an electron beam, Nanoscale, 2014, 6, 14877–14890 RSC
.
- C. S. Tian, D. Qian, D. Wu, R. H. He, Y. Z. Wu, W. X. Tang, L. F. Yin, Y. S. Shi, G. S. Dong, X. F. Jin, X. M. Jiang, F. Q. Liu, H. J. Qian, K. Sun, L. M. Wang, G. Rossi, Z. Q. Qiu and J. Shi, Body-Centered-Cubic Ni and Its Magnetic Properties, Phys. Rev. Lett., 2005, 94, 137210 CrossRef CAS PubMed
.
- C. Han, S. Yang, K. G. Chang, P. P. Wang, R. Murakami and X. P. Song, Structure transition and magnetism of bcc-Ni nanowires, J. Mater. Chem. C, 2014, 3, 1004–1010 RSC
.
- T. Shimizu, D. Lungerich, K. Harano and E. Nakamura, Time-Resolved Imaging of Stochastic Cascade Reactions over a Submillisecond to Second Time Range at the Angstrom Level, J. Am. Chem. Soc., 2022, 144, 9797–9805 CrossRef CAS PubMed
.
- H. Hölzel, M. Muth, D. Lungerich and N. Jux, Addressing Environmental Challenges of Porphyrin Mixtures Obtained from Statistical Syntheses, Chem. Methods, 2021, 1, 142–147 CrossRef
.
- M. Lepper, J. Köbl, L. Zhang, M. Meusel, H. Hölzel, D. Lungerich, N. Jux, A. de Siervo, B. Meyer, H. Steinrück and H. Marbach, Controlling the Self-Metalation Rate of Tetraphenylporphyrins on Cu(111) via Cyano Functionalization, Angew. Chem., Int. Ed., 2018, 57, 10074–10079 CrossRef CAS PubMed
.
- D. Lungerich, J. F. Hitzenberger, W. Donaubauer, T. Drewello and N. Jux, Three Short Stories about Hexaarylbenzene–Porphyrin Scaffolds, Chem. – Eur. J., 2016, 22, 16755–16759 CrossRef CAS PubMed
.
- M. Ruppel, D. Lungerich, S. Sturm, R. Lippert, F. Hampel and N. Jux, A Comprehensive Study on Tetraaryltetrabenzoporphyrins, Chem. – Eur. J., 2020, 26, 3287–3296 CrossRef CAS PubMed
.
- M. Ruppel, L.-P. Gazetas, D. Lungerich, F. Hampel and N. Jux, Investigations of Low-Symmetrical Tetraaryltetrabenzoporphyrins Produced by Mixed Condensation Reactions, J. Org. Chem., 2020, 85, 7781–7792 CrossRef CAS PubMed
.
|
This journal is © the Owner Societies 2024 |
Click here to see how this site uses Cookies. View our privacy policy here.