DOI:
10.1039/D3CE01097J
(Paper)
CrystEngComm, 2024,
26, 70-79
Fabrication of hierarchically porous ZIF-8 using a competitive ligand via a one-step method in supercritical CO2 and its application for CO2 adsorption†
Received
1st November 2023
, Accepted 14th November 2023
First published on 1st December 2023
1. Introduction
Metal–organic frameworks (MOFs) possess spatially-networked structures formed by the coordination of organic ligands and inorganic metal ions or metal clusters. Owing to their large specific surface area, tunable porosity, and structural diversity, MOFs have shown potential applications in a wide range of fields, such as catalysis,1,2 drug delivery,3 gas separation,4 carbon dioxide capture,5,6 energy storage,7,8 and semiconductor materials.9,10 ZIF-8 is one of the most widely studied MOF structures owing to its high thermal and chemical stability.11,12 As mentioned above, a vast majority of MOFs are microporous. However, hierarchically porous materials can achieve faster molecular diffusion and mass transfer, which is especially important for the capture and separation of gases13,14 and macromolecular catalysis.15,16 Creating hierarchical pores in MOFs has attracted significant research interest. In recent years, some efforts have been made to synthesize hierarchically porous MOFs (HP-MOFs).17–19
Multiple strategies have been explored to obtain HP-MOFs, which can be typically classified into a template method, defect formation method, and supercritical CO2 (scCO2) method. The template method creates hierarchical pores by removing soft or hard templates encapsulated in MOFs. However, the removal of templating agents may lead to the collapse of the structure of MOFs.20 The defect formation method introduces porous defects by incorporating relatively large ligands during the synthesis process.21 For instance, Cai et al.22 successfully achieved a controlled synthesis of hierarchical UiO-66 by a defect-formation strategy using monocarboxylic acid as a modulator in case of an insufficient number of organic ligands. In our study, hierarchically porous UiO-66 (ref. 23) was designed and synthesized using NaOH as the defect density and selective etchant. However, the defect formation strategy might bring some sacrifices in the periodic crystal structure of MOFs. In addition, there are some new strategies to synthesize HP-MOFs. Qi et al.24 developed a strategy to create HP-HKUST-1 by the modulation of cation valence. Without using any solvent or template, Dinker et al.25 synthesized HP-UiO-66 and HP-HKUST-1 utilizing PDAB-IL, which worked as a solitary medium. ScCO2 as a green solvent, which is easily available, inexpensive, non-toxic, and non-flammable, has received extensive attention in the field of material synthesis;26–30 it is highly sought after in the synthesis of HP-MOFs as well.31 The chemical and physical properties of scCO2 can be easily adjusted by changing the working temperature and pressure. Li et al.32 fabricated the hollow and mesoporous Zn-BTC tetrahedroids using a CO2-ionic liquid (IL) interfacial templating route. Zhao et al.33 formed Zn-MOF nanospheres in TMGA/EtFOSA/CO2 microemulsions. Similarly, Yu et al.34 synthesized Co-MOF with various pore structures in an IL/scCO2/surfactant system. Doan et al.35 used a scCO2 solvent expansion technique to synthesize HKUST-1 with large mesopores from a DMSO/MeOH solution. In addition, the scCO2 activation treatment can remove or reduce guest molecules existing in the structure of MOFs, which is an important step after the synthesis.36–40 Liu et al.41 activated the as-synthesized UMCM-9 from DMF using pure scCO2 and obtained a higher SBET of 5357 m2 g−1. Xiang et al.42 indicated that the main structure of HKUST-1 remained and the organic solvent was removed after scCO2 drying. The BET surface area values of the samples were also enhanced compared to those after thermal activation. In recent years, the synthesis of HP-MOF in scCO2 without using any templates has attracted great attention.
In this work, we propose a green and simple synthesis strategy to prepare hierarchically porous ZIF-8 (HP-ZIF-8) without templates and organic solvents in scCO2. ZIF-8 with hierarchical mesopores was successfully prepared by introducing a competitive ligand into scCO2. Meanwhile, the effects of the dosage of the competitive ligands and reaction temperature on the mesoporous structure and morphology were systematically studied. The as-synthesized HP-ZIF-8 was used for CO2 adsorption.
2. Experimental
2.1 Materials
Zinc acetylacetonate (99%, Zn(acac)2), and 2-methylimidazole (98%, 2-MI) were obtained from Aladdin (Shanghai, China). 2-Butyl-1H-imidazole (98%) was purchased from Meryer (Shanghai, China). Carbon dioxide (99.995%, CO2) was purchased from Tianjin Liufang (China). Ethanol was supplied by Tianjin Jiangtian (China). All the chemicals were used without further purification.
2.2 Preparation of HP-ZIF-8
Zinc acetylacetonate, 2-methylimidazole, and 2-butyl-1H-imidazole were mixed in a certain molar ratio, and then transferred to a reaction column. The reactor was heated and pressurized to a certain temperature and pressure. After the reaction for a certain time, CO2 was released by depressurization. The crystals were collected and washed with ethanol three times and then dried for 4 hours at 75 °C under a vacuum. The yield was calculated according to eqn (1)43 |  | (1) |
where Y is the yield of ZIF-8, %; M1 is the mass of Zn in the synthesized ZIF-8, g; M2 is the mass of Zn employed in Zn(acac)2, g. In a typical synthesis, the components were mixed in a molar ratio 1
:
1
:
6 of zinc acetylacetonate, 2-methylimidazole, and 2-butyl-1H-imidazole, and then transferred to the reaction column. The reaction was performed at 50 °C and 30 MPa for three hours. Then, CO2 was released, and the products were collected, washed three times in ethanol, and dried for four hours at 75 °C under vacuum. The synthesized crystals were designated as Z-2.
2.3 CO2 adsorption and regeneration performance.
To remove water and other small molecules, the as-synthesized ZIF-8 was heated at 90 °C for 3 h before the CO2 adsorption experiments. In a typical process, the adsorbent was loaded into the u-shaped tube, and CO2 gas was introduced at a flow rate of 10 mL min−1. CO2 flow rates before and after the adsorption were recorded using flow indicators of the D07-19B mass flow controller and the D07-19 mass flowmeter (Xiaotao, Beijing, China), respectively. The CO2 adsorption capacity was calculated using eqn (2). |  | (2) |
where C is the CO2 adsorption capacity, mg of CO2/g ZIF-8; 1.977 is the density of CO2 under standard conditions, mg mL−1; V1 and V2 are the CO2 cumulative flux values before and after the adsorption, respectively, under standard conditions, mL; m is the mass of adsorbent, g.
The regeneration performance of the as-synthesized HP-ZIF-8 with the highest adsorption capacity was evaluated. Typically, the CO2-saturated sample was heated at 90 °C for 4 h to remove the adsorbed CO2. The regenerated HP-ZIF-8 was used for the next CO2 adsorption–desorption cycle and the cyclic experiment was repeated several times.
2.4 Characterizations
The samples were characterized by powder X-ray diffraction (XRD) in a D8-Focus X-ray diffractometer (Bruker, Germany) using CuKα radiation at a scanning rate of 5° min−1 in the region of 5–50°. The recorded data was compared to previously published simulated patterns derived from X-ray single crystal diffraction studies. The morphology of all the samples was examined using scanning electron microscopy (SEM, S-8100 HITACHI, Japan). Transmission electron microscopy (TEM) was performed using the JEM-2100F (JEOL, Japan) instrument. Thermogravimetric analysis was carried out on a TG209 F3 Tarsus thermogravimetric analyzer (TGA, Netzsch, Germany). The temperature range was from 30 to 800 °C at a heating rate of 10 °C min−1 under an air atmosphere. Nitrogen adsorption and desorption isotherms of the samples were analyzed using the ASAP 2460 physisorption apparatus (Micromeritics, USA) at 77 K. Fourier transform infrared (FT-IR) spectra were analyzed using a VERTEX 70 FT-IR spectrometer (Bruker, Germany) in the region of 3500–500 cm−1.
3. Results and discussion
3.1 Effect of the dosage of competitive ligand on HP-ZIF-8
When the molar ratios of zinc acetylacetonate, 2-methylimidazole, and 2-butyl-1H-imidazole are 1
:
1
:
1, 1
:
1
:
6, and 1
:
1
:
12, respectively, the samples are denoted as Z-1, Z-2, and Z-3, respectively. For comparison, ZIF-8 was synthesized in scCO2 at a molar ratio of zinc acetylacetonate and 2-methylimidazole as 1
:
2, without the addition of 2-butyl-1H-imidazole, and labeled as Z-0. The XRD patterns of the samples are shown in Fig. 1. It is clear that the characteristic diffraction peaks of four samples correspond to those of the simulated ZIF-8, which indicates the formation of ZIF-8.11 The samples synthesized at various molar ratios showed almost identical XRD peaks.44 However, no diffraction peaks of impurities could be observed, which indicated the formation of ZIF-8 crystals.
 |
| Fig. 1 The XRD patterns of ZIF-8 samples synthesized at different molar ratios of Zn(acac)2, 2-methylimidazole, and 2-butyl-1H-imidazole. | |
Fig. 2 presents the SEM and TEM images of ZIF-8 synthesized at different dosages of 2-butyl-1H-imidazole. As shown in Fig. 2a, for Z-1, rhombic dodecahedron (RD) was observed without any obvious mesopores (Fig. 2d). When n(zinc acetylacetonate)
:
n(2-methylimidazole)
:
n(2-butyl-1H-imidazole) = 1
:
1
:
6, Z-2 exhibits regular RD (Fig. 2b) and mesoporous structure (Fig. 2e and S1†). With further increased dosage of 2-butyl-1H-imidazole, extremely small irregular RD particles with low crystallinity with unsharp facets and edges (Fig. 2c) were observed for Z-3, which is consistent with the result of the low intensity in the XRD pattern of Z-3 (Fig. 1). The RD shape comprises twelve equivalent rhomboid (or tetragonal) facets that are all [110]-oriented.45,46 In addition, the mesopores appear in Fig. 2e. The properties of HP-ZIF-8 were further characterized using other techniques. The TGA curves of Z-1, Z-2, and Z-3 are shown in Fig. S2.† It can be found that Z-1 and Z-3 have similar thermostability and both showed minimal weight loss up to 350 °C. In the temperature range of 350–600 °C, the weight loss was 70 wt%, corresponding to the decomposition of the ZIF-8 structure and ZnO was formed. In comparison, Z-2 had a small weight loss at ca. 200 °C and a sharp weight loss of 60% from 350 to 550 °C. In particular, a small weight loss of 10 wt% from 200–350 °C corresponded to the removal of 2-butyl-1H-imidazole, participating in the coordination, which contributed to the hierarchical pore structure of Z-2. Fig. S3† shows the FT-IR spectrum of the as-synthesized ZIF-8, the band at 421 cm−1 is attributed to Zn–N stretching vibration. The bands in the spectral region of 600–800 cm−1 are for the out-of-plane bending modes of the imidazole ring, and those in the region of 900–1350 cm−1 are associated with the out-of-plane bending. The complicated bands at 1350–1500 cm−1 are attributed to the entire ring stretching. The peak at 1597 cm−1 is for the C
N stretch mode. The bands at 2870, 2929, 2960, and 3138 cm−1 are assigned to the aromatic and imidazole aliphatic C–H stretch vibrations. Almost all FT-IR bands are in good agreement with those from the previously reported spectrum of ZIF-8.47 However, the peaks between 1350 cm−1 and 1500 cm−1 for Z-1, Z-2, and Z-3 samples are stronger than those of Z-0, which suggests that the regular coordination of the initial ZIF-8 crystal is disrupted and the imidazole ring plane is stretched to a greater extent due to the coordination of 2-butyl-1H-imidazole and Zn2+. As a result, the peak intensity of the corresponding position was enhanced.48
 |
| Fig. 2 The SEM (a–c) and TEM (d–f) images of HP-ZIF-8 synthesized at different molar ratios of Zn(acac)2, 2-methylimidazole, and 2-butyl-1H-imidazole: (a and d) Z-1, (b and e) Z-2, and (c and f) Z-3. | |
Fig. 3a shows N2 adsorption–desorption isotherms of the ZIF-8 synthesized in scCO2 at different molar ratios of Zn(acac)2, 2-methylimidazole, and 2-butyl-1H-imidazole. The mesopore size distribution curves, calculated from the adsorption branch of the Barrett–Joyner–Halenda (BJH) analysis, are shown in Fig. 3b. Z-1 shows a typical I type isotherm with a high amount of N2 adsorption at low relative pressures and the corresponding BJH results indicate the absence of mesopores. Z-2 exhibits a type IV isotherm and possesses a pronounced hysteresis, which is related to mesoporous materials. Its pore diameter (range of 3.9–30 nm) concentrates in 3.9 nm (Fig. 3b), which denotes a random distribution of mesopores or a system of interconnected pores.49 The t-plot micropore volume is 0.08 cm3 g−1 and the micropore size was centered at 1.7 nm. The BET surface area and total volume are 529 m2 g−1 and 0.72 cm3 g−1, respectively. The t-plot-specific micropore surface area is 338 m2 g−1. The Smeso/Smicro is up to 0.56. When the molar ratio of Zn(acac)2, 2-methylimidazole, and 2-butyl-1H-imidazole was increased to 1
:
1
:
12, the N2 sorption isotherm of Z-3 showed a type of isotherm that was between I and IV and gave a small hysteresis with a pore size located at 3.9 nm. Obviously, the higher dosage of 2-butyl-1H-imidazole contributes to the formation of larger mesoporous, which is consistent with the SEM observations. The detailed porosity properties are listed in Table 1. Z-0 is synthesized by reacting zinc acetylacetonate and 2-methylimidazole in a molar ratio of 1
:
2 at 50 °C and 30 MPa in scCO2. The BET surface area value (834 m2 g−1) of Z-0 synthesized in scCO2 without using the competitive ligand was lower than the typical value (1400–1700 m2 g−1) of samples synthesized using the conventional solvothermal method, which should be attributed to different particle sizes and pore parameters based on different reaction media and conditions. The BET surface area values of the other samples Z-2 and Z-3 synthesized in scCO2 using competitive ligands were lower than those of Z-0 and Z-1. It is clear that the appearance of the mesoporous structure causes the loss of the specific surface area.50,51 The total pore volume (Vt) of the Z-2 was high (0.7 cm3 g−1), which may be beneficial to CO2 adsorption. The results clearly showed that the formation of mesoporous structure in ZIF-8 was caused by the amount of the competitive ligand, which is a decisive factor in guaranteeing the hierarchical pores. However, mesoporous diameter is not simply linearly related to the dosage of 2-butyl-1H-imidazole. The partial coordination of Zn2+ and 2-butyl-1H-imidazole causes a certain degree of malformation in the ZIF-8 unit cell. The overlap of irregular pores will reduce the diameter of the mesoporous.12
 |
| Fig. 3 (a) The nitrogen adsorption–desorption isotherms and (b) the pore size distributions of ZIF-8 samples synthesized with different molar ratios of Zn(acac)2, 2-methylimidazole, and 2-butyl-1H-imidazole. | |
Table 1 Pore parameters of ZIF-8 samples synthesized with different molar ratios of Zn(acac)2, 2-methylimidazole and 2-butyl-1H-imidazolea
Samples |
S
BET
(m2 g−1) |
S
micro
(m2 g−1) |
V
t
(cm3 g−1) |
V
micro
(cm3 g−1) |
D
micro
(nm) |
V
meso
(cm3 g−1) |
D
meso
(nm) |
Yield (%) |
Reaction temperature is 50 °C. The reaction pressure was 30 MPa. The reaction time was 3 hours.
BET-specific surface area.
t-Plot-specific micropore surface area calculated from the N2 adsorption–desorption isotherm from SBET.
Total specific pore volume.
t-Plot micropore volume.
Estimated from the local maximum of NLDFT pore size distribution obtained in the desorption branch of N2 isotherm.
Specific mesopore volume obtained from the BJH cumulative specific adsorption volume.
Estimated from the local maximum of BJH pore size distribution obtained in the desorption branch of N2 isotherm.
|
Z-1 |
983 |
822 |
0.50 |
0.37 |
0.8 |
0.46 |
— |
81.2 |
Z-2 |
529 |
338 |
0.72 |
0.08 |
1.7 |
1.21 |
3.9–30 |
77.0 |
Z-3 |
601 |
497 |
0.30 |
0.22 |
1.1 |
0.20 |
3.9 |
71.6 |
Z-0 |
834 |
782 |
0.40 |
0.36 |
1.6 |
0.10 |
— |
84.8 |
3.2 Effect of reaction temperature on HP-ZIF-8
To investigate how the temperature affects the formation and porosity properties of HP-ZIF-8, the applied temperature was set as 30, 50, and 70 °C while pressure and reaction time were held constant (30 MPa, 3 h). Samples were synthesized at different temperatures and named Z-2-T (x), wherein x represents a specific temperature (°C). The Z-1, Z-2, and Z-3 mentioned above were all synthesized at 50 °C and 3 h. That is, Z-2-T (50) is Z-2.
At a low temperature of 30 °C, large-sized cubic crystals with well-defined and smooth crystal surfaces and bits of small cubes with truncated edges can be seen in the SEM image of Z-2-T (30) (Fig. 4a). The “cubes” with the truncated edges expose the 6{100} and 12{110} faces.35 This is similar to the morphology that appeared in the early stage of the preparation of ZIF-8 by the solvothermal method.52 A clear mesoporous structure was observed in the TEM image of Z-2-T (30), as shown in Fig. 4d. At higher temperatures (50 °C), crystals tended to become RD with uniform sizes (Fig. 2b) and more mesoporous were obtained (Fig. 2e). The RD shape of Z-2-T (50) resulted from the Wulff's rule, in which the slowest growing direction (for ZIF-8, [110]) determines the final shape and the most thermodynamically stable facet orientation.34 When the temperature increases to 70 °C, the crystals are irregular polygonal agglomerates of uneven sizes (Fig. 2c). Which explains that lower temperatures are conducive to the coordination of the ligand and Zn2+, and the growth rate of the crystal is relatively slow. As described in the Ostwald theory, it tends to develop into perfect crystals with a larger size in a long enough time. With the increase in temperature, the coordination rate between the ligand and Zn2+ is accelerated, and the mismatch degree of coordination between the competing ligand and Zn2+ was enhanced, the crystal tended to form small particles, and the proportion of mesoporous structure was increased. It can be seen that temperature influences the crystal morphology and the pore structure by controlling the coordination rate between Zn2+ and ligands.
 |
| Fig. 4 The SEM (a–c) and TEM (d–f) images of ZIF-8 samples synthesized at different temperatures. (a and d) Z-2-T (30), (b and e) Z-2-T (50), and (c and f) Z-2-T (70). | |
To clarify the temperature influence on HP-ZIF-8 synthesis. Fig. 5a and b show the representative N2 adsorption–desorption isotherms and pore size distributions of ZIF-8 synthesized at different temperatures. Z-2-T (30) showed a typical I type isotherm, indicating that there are mainly micropores. The BET and the t-plot-specific micropore surface areas were 849 and 1167 m2 g−1, respectively. The microporous volume was approximately 0.33 cm3 g−1. The N2 adsorption–desorption isotherms of Z-2-T (70) is roughly consistent with type I isotherm. The high nitrogen adsorption at very low relative pressure is due to the existence of micropores, while at high relative pressure (P/P0 > 0.8), a long and narrow hysteresis loop appears, which may be attributed to the formed large pores by ZIF-8 crystal packing.53 The Z-2-T (70) crystal has no mesopores, which is confirmed in Fig. 5b. Table S1† lists the porosity details of ZIF-8 samples synthesized at different temperatures.
 |
| Fig. 5 (a) The nitrogen adsorption–desorption isotherms and (b) the pore size distributions of ZIF-8 samples synthesized at different temperatures. | |
3.3 Formation mechanism of HP-ZIF-8 in scCO2
On the basis of the above results and discussion, we propose a reasonable mechanism for the formation of HP-ZIF-8 in scCO2 (Scheme 1). Since 2-butyl-1H-imidazole and 2-MI have similar structures and both can dissolve well in scCO2, 2-butyl-1H-imidazole, and 2-methylimidazole, they can coordinate with Zn2+ in scCO2. As a competitive ligand, 2-butyl-1H-imidazole successfully coordinates with Zn2+, disrupting the original highly regular and ordered coordination of the ZIF-8 crystal, triggering the coordination vacancy, and forming the defective units. The defect units (framed by the red dashed lines) are self-assembled to form periodic topological structures with defects, namely HP-ZIF-8. Whether or not ZIF-8 can regulate the formation of the hierarchical pore structure depends on the amount of the competing ligand. Meanwhile, the reaction temperature can affect the crystal morphology by controlling the coordination rate between Zn2+ and the ligand. In contrast, Scheme S1† demonstrates how ZIF-8 is synthesized in scCO2. Zn2+ coordinates with 2-methylimidazole to produce regular unit structures, which are precisely constructed to form microporous ZIF-8.
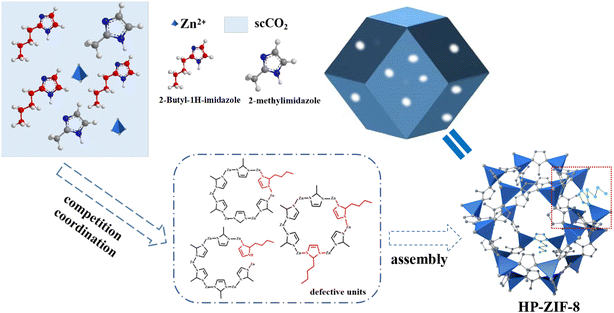 |
| Scheme 1 The formation mechanism of HP-ZIF-8 in scCO2. | |
3.4 CO2 adsorption performance of HP-ZIF-8
The synthesized ZIF-8 was used to adsorb CO2 and the results are shown in Fig. 6 and Table S2.† It is clear that the CO2 equilibrium adsorption capacity of Z-2 synthesized in scCO2 (50 °C and 30 MPa) with the molar ratio of 1
:
1
:
6 among zinc acetylacetonate, 2-methylimidazole and 2-butyl-1H-imidazole reaches 1.49 mmol g−1 MOF at 25 °C and 0.1 MPa (Fig. 6b), which is the highest among the ZIF-8 samples synthesized in scCO2. This may be owing to the mesoporous structure and larger diameter of Z-2. The pore size of ZIF-8 is 3.4 Å, which is comparable to the kinetic diameter of CO2 (3.3 Å), imparting ZIF-8 with a great potential for CO2 adsorption.54 The existence of a mesoporous structure in ZIF-8 can provide more adsorption sites to enhance its physical adsorption performance.49 The CO2 equilibrium adsorption capacity of the as-synthesized HP-ZIF-8 was compared with other HP-MOF materials (Table 2). Z-2 exhibits a higher CO2 equilibrium adsorption capacity than that reported for most of the HP-ZIF-8 materials.55–59 Particularly, the CO2 equilibrium adsorption capacity of Z-2 was lower than that of PEI@ZIF-8 (ref. 55) and ZIF-8-NH2@BC56 which possess both hierarchical pore structures and CO2-phillic functional groups.
 |
| Fig. 6 The plots of CO2 adsorption capacity vs. time (a) and the CO2 equilibrium adsorption capacity (b) of ZIF-8 synthesized in scCO2 with the different dosages of competitive ligand or at different temperatures. | |
Table 2 The CO2 equilibrium adsorption capacity of HP-MOF materials reported in literature
Material |
Experimental conditions |
S
BET (m2 g−1) |
V
t (cm3 g−1) |
CO2 equilibrium adsorption capacity (mmol g−1 sorbent) |
Ref. |
PEI@ZIF-8 |
25 °C, 0.1 MPa |
322 |
0.39 |
≈2.23 |
55
|
ZIF-8-NH2@BC |
25 °C, 0.1 MPa |
455 |
0.17 |
1.63 |
56
|
CS/ZIF-8 |
25 °C, 0.115 MPa |
627 |
— |
0.99 |
57
|
Graphene/ZIF-8 |
25 °C, 0.1 MPa |
1099 |
0.67 |
0.99 |
58
|
ZPMAC (ZIF-8/PMAC) |
25 °C, 0.1 MPa |
322 |
0.39 |
0.80 |
59
|
Z-2 |
25 °C, 0.1 MPa |
529 |
0.72 |
1.49 |
This work |
Fig. 7 displays the CO2 equilibrium adsorption capacity of Z-2 after multiple regenerations. CO2 equilibrium adsorption capacity decreases to 91% after seven cycles, which decreases from 1.49 mmol g−1 Z-2 to 1.36 mmol of CO2/g Z-2. The decrease in the CO2 equilibrium adsorption capacity is due to incomplete CO2 desorption and the destruction of the pore structure during the regeneration process. In order to further explain this phenomenon, the pore parameters and morphology of Z-2 before and after regeneration were characterized. As confirmed by XRD measurements (Fig. S4b†), the reusable Z-2 can maintain well-defined crystal structures. As seen in Fig. S4c,† the mesoporous structure of the regenerative Z-2 sample collapses to some degree compared to that of pristine Z-2. The above results indicate that the destruction of the mesoporous structure is the main reason for the decrease of CO2 equilibrium adsorption capacity of Z-2 after 7 cycles.
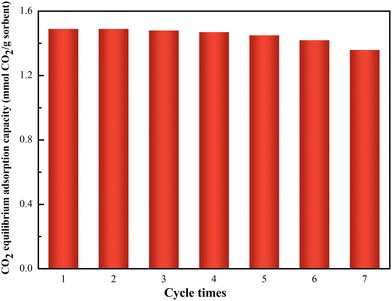 |
| Fig. 7 The effect of cycle times on CO2 equilibrium adsorption capacity of Z-2. | |
4. Conclusions
In summary, HP-ZIF-8 was simply fabricated in scCO2 by a one-step method. Particularly, this process does not involve templating agents or organic solvents, which is an efficient and green method to synthesize HP-MOF. The dosage of competitive ligand is the key to constructing mesopores and the temperature controls the coordination rate to affect the morphology of HP-ZIF-8. The as-synthesized HP-ZIF-8 showed favorable performance in CO2 adsorption. The CO2 equilibrium adsorption capacity of Z-2 could reach up to 1.49 mmol g−1, which is 31.8% higher than that of the pristine ZIF-8. Moreover, Z-2 shows preeminent recycling stability and the CO2 equilibrium adsorption capacity only decreased by 9% after seven adsorption–desorption cycles. Compared with the reported HP-ZIF-8 materials, Z-2 exhibited excellent CO2 adsorption performance. The present synthetic strategy is easily controlled, fairly simple, and green. It can be readily extended to prepare diverse MOFs with similar structures using other metal ions and ligands.
Conflicts of interest
There are no conflicts of interest to declare.
References
- X. Wu, Y. Hua and Y. Zhang,
et al., Packaging and delivering enzymes by amorphous metal-organic frameworks, Nat. Commun., 2019, 10, 5165 CrossRef.
- Z. Jiang, X. Xu and Y. Ma,
et al., Filling metal–organic framework mesopores with TiO2 for CO2 photoreduction, Nature, 2020, 586, 549–554 CrossRef CAS.
- S. Feng, X. Zhang, D. Shi and Z. Wang, Zeolitic imidazolate framework-8 (ZIF-8) for drug delivery: A critical review, Front. Chem. Sci. Eng., 2021, 15, 221–237 CrossRef CAS.
- E. Adatoz, A.-K. Avci and S. Keskin, Opportunities and challenges of MOF-based membranes in gas separations, Sep. Purif. Technol., 2015, 207–237 CrossRef CAS.
- W. Gao, Y. Chen, Y. Niu, K. Williams, L. Cash, P. J. Perez, L. Wojtas, J. Cai, Y. Chen and S. Ma, Crystal engineering of an nbo topology metal-organic framework for chemical fixation of CO2 under ambient conditions, Angew. Chem., Int. Ed., 2014, 53, 2615–2619 CrossRef CAS PubMed.
- M. Ding, R. W. Flaig, H. Jiang and O. M. Yaghi, Carbon capture and conversion using metal-organic frameworks and MOF-based materials, Chem. Soc. Rev., 2019, 48, 2783–2828 RSC.
- Z. Liang, C. Qu, W. Guo, R. Zou and Q. Xu, Pristine metal-organic frameworks and their composites for energy storage and conversion, Adv. Mater., 2018, 30, 1702891 CrossRef PubMed.
- T. Qiu, Z. Liang, W. Guo, H. Tabassum, S. Gao and R. Zou, Metal–organic framework-based materials for energy conversion and storage, ACS Energy Lett., 2020, 5, 520–532 CrossRef CAS.
- W. Koo, S. Choi, S. Kim, J. Jang, H. L. Tuller and I. Kim, Heterogeneous sensitization of metal-organic framework driven metal@metal oxide complex catalysts on an oxide nanofiber scaffold toward superior gas sensors, J. Am. Chem. Soc., 2016, 138, 13431–13437 CrossRef CAS PubMed.
- J. Xiao, L. Han, J. Luo, S. Yu and H. Jiang, Integration of plasmonic effects and schottky junctions into metal-organic framework composites: steering charge flow for enhanced visible-light photocatalysis, Angew. Chem., Int. Ed., 2018, 57, 1103–1107 CrossRef CAS PubMed.
- K. Park, Z. Ni, A. P. Côte, J. Choi, R. Huang, F. Uribe-Romo, H. K. Chae, O. Michael and O. Yaghi, Exceptional chemical and thermal stability of zeolitic imidazolate frameworks, Proc. Natl. Acad. Sci. U. S. A., 2006, 103, 10186–10191 CrossRef CAS.
- X. Huang, Y. Lin, J. Zhang and X. Chen, Ligand-directed strategy for zeolite-type metal-organic frameworks: zinc(II) imidazolates with unusual zeolitic topologies, Angew. Chem., Int. Ed., 2006, 45, 1557–1559 CrossRef CAS.
- W. Hou, J. Cheng, N. Liu, C. Yang, Y. Chen, H. Zhan, B. Ye and J. Zhou, Selection-diffusion-selection mechanisms in ordered hierarchically-porous MOF-on-MOF: ZIF-8 @NH2-MIL-125 for efficient CO2 separation, J. Environ. Chem. Eng., 2022, 10, 108029 CrossRef CAS.
- X. Zhang, C. Chuang, P. Dong, Y. Cha, T. Bae and M. Song, Hierarchically porous Co-MOF-74 hollow nanorods for enhanced dynamic CO2 separation, ACS Appl. Mater. Interfaces, 2018, 10, 43316–43322 CrossRef CAS.
- C. Lin, K. Xu, R. Zheng and Y. Zheng, Immobilization of amidase into a magnetic hierarchically porous metal-organic framework for efficient biocatalysis, Chem. Commun., 2019, 40, 5697–5700 RSC.
- X. Liu, W. Qi, Y. Wang, D. Lin, X. Yang, R. Su and Z. He, Rational design of mimic multienzyme systems in hierarchically porous biomimetic metal-organic frameworks, ACS Appl. Mater. Interfaces, 2018, 10, 33407–33415 CrossRef CAS.
- S. Yuan, L. Zou, J. Qin, J. Li, L. Huang, L. Feng, X. Wang, M. Bosch, A. Alsalme, T. Cagin and H. Zhou, Construction of hierarchically porous metal-organic frameworks through linker labilization, Nat. Commun., 2017, 8, 15356 CrossRef CAS PubMed.
- L. Feng, S. Yuan, L. Zhang, K. Tan, J. Li, A. Kirchon, L. Liu, P. Zhang, Y. Han, Y. Chabal and H. Zhou, Creating hierarchical pores by controlled linker thermolysis in multivariate metal-organic frameworks, J. Am. Chem. Soc., 2018, 140, 2363–2372 CrossRef CAS PubMed.
- S. Furukawa, J. Reboul, S. Diring, K. Sumida and S. Kitagawa, Structuring of metal-organic frameworks at the mesoscopic/macroscopic scale, Chem. Soc. Rev., 2014, 43, 5700–5734 RSC.
- X. Chen, X. Jiang, C. Yin, B. Zhang and Q. Zhang, Facile fabrication of hierarchical porous ZIF-8 for enhanced adsorption of antibiotics, J. Hazard. Mater., 2019, 367, 194–204 CrossRef CAS PubMed.
- J. Koo, I. Hwang, X. Yu, S. Saha, Y. Kim and K. Kim, Hollowing out MOFs hierarchical micro- and mesoporous MOFs with tailorable porosity via selective acid etching, Chem. Soc. Rev., 2017, 8, 6799–6803 CAS.
- G. Cai and H. Jiang, A modulator-induced defect-formation strategy to hierarchically porous metal-organic frameworks with high stability, Angew. Chem., 2017, 129, 578–582 CrossRef.
- X. Yang, Z. Li and S. Tang, Tailored design of hierarchically porous UiO-66 with a controlled pore structure and metal sites, Cryst. Growth Des., 2021, 21, 6092–6100 CrossRef CAS.
- S. Qi, X. Qian, Q. He, K. Miao, Y. Jiang, P. Tan, X. Liu and L. Sun, Generation of hierarchical porosity in metal-organic frameworks by the modulation of cation valence, Angew. Chem., Int. Ed., 2019, 58, 10104–10109 CrossRef CAS.
- M. Dinker, K. Zhao, X. Liu and L. Sun, Solitary medium of a multifunctional ionic liquid for crystallizing hierarchically porous metal-organic frameworks, Inorg. Chem., 2022, 61, 10393–10401 CrossRef CAS.
- P. López-Domínguez, A. López-Periago, F. Fernández-Porras, J. Fraile, G. Tobias and C. Domingo, Supercritical CO2 for the synthesis of nanometric ZIF-8 and loading with hyperbranched aminopolymers. applications in CO2 capture, J. CO2 Util., 2017, 18, 147–155 CrossRef.
- Y. Wang, S. Wang and S. Tang, Synthesis and characterization of imine-based covalent organic framework (COF-LZU1) in supercritical carbon dioxide, Gaodeng Xuexiao Huaxue Xuebao, 2020, 41, 1792–1800 CAS.
- P. Lopez-Aranguren, L. Vega and C. Domingo, A new method using compressed CO2 for the in situ functionalization of mesoporous silica with hyperbranched polymers, Chem. Commun., 2013, 49, 11776–11778 RSC.
- P. Lopez-Aranguren, S. Builes, J. Fraile, A. Lopez-Periago, L. Vega and C. Domingo, Hybrid aminopolymer-silica materials for efficient CO2 adsorption, RSC Adv., 2015, 5, 104943–104953 RSC.
- A. Lopez-Periago, P. Lopez-Domínguez, J. Barrio, G. Tobias and C. Domingo, Binary supercritical CO2 solvent mixtures for the synthesis of 3D metal-organic frameworks, Microporous Mesoporous Mater., 2016, 234, 155–161 CrossRef CAS.
- A. BorrásAlbert, R. Fraile, A. López-Periago, J. Amirali and Y. Domingo, Meso/microporous MOF@graphene oxide composite aerogels prepared by generic supercritical CO2 technology, Microporous Mesoporous Mater., 2022, 335, 111825 CrossRef.
- L. Peng, J. Zhang, J. Li, B. Han, Z. Xue, B. Zhang, J. Shi and G. Yang, Hollow metal-organic framework polyhedra synthesized by a CO2–ionic liquid interfacial templating route, J. Colloid Interface Sci., 2014, 416, 198–204 CrossRef CAS PubMed.
- Y. Zhao, J. Zhang, B. Han, J. Song, J. Li and Q. Wang, Metal-organic framework nanospheres with well-ordered mesopores synthesized in an ionic liquid/CO2/surfactant system, Angew. Chem., Int. Ed., 2011, 50, 636–639 CrossRef CAS.
- H. Yu, D. Xu and Q. Xu, Dual template effect of supercritical CO2 in ionic liquid to fabricate a highly mesoporous cobalt metal-organic framework, Chem. Commun., 2015, 51, 13197–13200 RSC.
- H. V. Doan, Y. Fang, B. Yao, Z. Dong, T. J. White, A. Sartbaeva, U. Hintermair and V. P. Ting, Controlled formation of hierarchical metal-organic frameworks using CO2-expanded solvent systems, ACS Sustainable Chem. Eng., 2017, 5, 7887–7893 CrossRef CAS.
- E. Engel, A. Jouaiti, C. Bezuidenhout, M. Hosseini and L. Barbour, Activation-dependent breathing in a flexible metal-organic framework and the effects of repeated sorption/desorption cycling, Angew. Chem., Int. Ed., 2017, 56, 8874–8878 CrossRef CAS.
- D. Han, F. Jiang, M. Wu, L. Chen, Q. Chen and M. Hong, A non-interpenetrated porous metal-organic framework with high gas-uptake capacity, Chem. Commun., 2011, 47, 9861–9863 RSC.
- J. Liu, J. Culp, S. Natesakhawat, B. Bockrath, B. Zande, S. G. Sankar, G. Garberoglio and J. K. Johnson, Experimental and theoretical studies of gas adsorption in Cu3(BTC)2: an effective activation procedure, J. Phys. Chem. C, 2007, 111, 9305–9313 CrossRef CAS.
- Z. Herm, J. Swisher, B. Smit, R. Krishna and J. Long, Metal-organic frameworks as adsorbents for hydrogen purification and precombustion carbon dioxide capture, J. Am. Chem. Soc., 2007, 133, 5664–5667 CrossRef PubMed.
- H. Li, M. Eddaoudi, M. O'Keefe and O. Yaghi, Design and synthesis of an exceptionally stable and highly porous metal-organic framework, Nature, 1999, 402, 276–279 CrossRef CAS.
- B. Liu, A. Wong-Foy and A. Matzger, Rapid and enhanced activation of microporous coordination polymers by flowing supercritical CO2, Chem. Commun., 2013, 49, 1419–1421 RSC.
- Z. Xiang, D. Cao, X. Shao, W. Wang, J. Zhang and W. Wu, Facile preparation of high-capacity hydrogen storage metal-organic frameworks: A combination of microwave-assisted solvothermal synthesis and supercritical activation, Chem. Eng. Sci., 2010, 65, 3140–3146 CrossRef CAS.
- D. N. Ta, H. K. D. Nguyen, B. X. Trinh, Q. T. N. Le, H. N. Ta and H. T. Nguyen, Preparation of nano-ZIF-8 in methanol with high yield, Can. J. Chem. Eng., 2018, 96, 1518–1531 CrossRef CAS.
- W. Zheng, R. Ding, K. Yang, Y. Dai, X. Yan and G. He, ZIF-8 nanoparticles with tunable size for enhanced CO2 capture of pebax based MMMs, Sep. Purif. Technol., 2019, 214, 111–119 CrossRef CAS.
- J. Troyano, A. Carné-Sánchez, C. Avci, I. Imaza and D. Maspoch, Colloidal metal-organic framework particles the pioneering case of ZIF-8, Chem. Soc. Rev., 2019, 48, 5529–5618 RSC.
- J. Cravillon, R. Nayuk, S. Springer, A. Feldhoff, K. Huber and M. Wiebcke, Controlling zeolitic imidazolate framework nano- and microcrystal formation insight into crystal growth by time-resolved in situ static light scattering, Chem. Mater., 2011, 23, 2130–2141 CrossRef CAS.
- M. He, J. Yao, Q. Liu, K. Wang, F. Chen and H. Wang, Facile synthesis of zeolitic imidazolate framework-8 from a concentrated aqueous solution, Microporous Mesoporous Mater., 2014, 184, 55–60 CrossRef CAS.
- Y. Hu, H. Kazemian, S. Rohani, Y. Huang and Y. Song, In situ high pressure study of ZIF-8 by FTIR spectroscopy, Chem. Commun., 2011, 47, 12694 RSC.
- Y. Wu, M. Zhou, B. Zhou, B. Wu, J. Li, J. Qiao, X. Guan and F. Li, Amino acid assisted templating synthesis of hierarchical zeolitic imidazolate framework-8 for efficient arsenate removal, Nanoscale, 2014, 6, 1105–1112 RSC.
- S. Wang and S. Tang, Rapid and facile synthesis of metal organic framework materials by reaction crystallization in supercritical CO2, Mater. Lett., 2019, 251, 65–68 CrossRef CAS.
- M. Sinnwell, Q. Miller, L. Liu, J. Tao, M. Bowden, L. Kovarik, D. Barpaga, Y. Han, R. Motkuri, M. Sushko, H. Schaef and P. Thallapally, Kinetics and mechanisms of ZnO to ZIF-8 transformations in supercritical CO2 revealed by in situ X-ray diffraction, ChemSusChem, 2020, 13, 2602–2612 CrossRef CAS PubMed.
- J. Cravillon, C. Schröder, H. Bux, A. Rothkirch, J. Carob and M. Wiebcke, Formate modulated solvothermal synthesis of ZIF-8 investigated using time-resolved in situ X-ray diffraction and scanning electron microscopy, CrystEngComm, 2012, 14, 492–498 RSC.
- Y. Pan, Y. Liu, G. Zeng, L. Zhao and Z. Lai, Rapid synthesis of zeolitic imidazolate framework-8 (ZIF-8) nanocrystals in an aqueous system, Chem. Commun., 2011, 47, 2071–2073 RSC.
- Y. Xie, J. Liang, Y. Fu, M. Huang, X. Xu, H. Wang, S. Tu and J. Li, Hypercrosslinked mesoporous poly(ionic liquid)s with high ionic density for efficient CO2 capture and conversion into cyclic carbonates, J. Mater. Chem. A, 2018, 6, 6660–6666 RSC.
- Q. Guo, C. Chen, L. Zhou, X. Lia, Z. Lia, D. Yuana, J. Dinga, H. Wana and G. Guan, Design of ZIF-8/ion copolymer hierarchically porous material coordination effect on the adsorption and diffusion for carbon dioxide, Microporous Mesoporous Mater., 2018, 261, 79–87 CrossRef CAS.
- H. Ma, Z. Wang, X. Zhang, M. Ding and J. Yao, In situ growth of amino-functionalized ZIF-8 on bacterial cellulose foams for enhanced CO2 adsorption, Carbohydr. Polym., 2021, 270, 118376 CrossRef CAS.
- M. Fan, F. Gai, Z. Yu Cao, Y. Zhao, Y. Ao and Q. H. Liu, Structuring ZIF-8-based hybrid material with hierarchical pores by in situ synthesis and thermal treatment for enhancement of CO2 uptake, J. Solid State Chem., 2019, 269, 507–512 CrossRef CAS.
- Z. Zhang, N. Sun, W. Wei and Y. Sun, Facilely controlled synthesis of a core-shell structured MOF composite and its derived N-doped hierarchical porous carbon for CO2 adsorption, RSC Adv., 2018, 8, 21460–21471 RSC.
- M. Jiang, H. Li, L. Zhou, R. Xing and J. Zhang, Hierarchically porous graphene/ZIF-8 hybrid aerogel preparation, CO2 uptake capacity, and mechanical property, ACS Appl. Mater. Interfaces, 2018, 10, 827–834 CrossRef CAS PubMed.
|
This journal is © The Royal Society of Chemistry 2024 |
Click here to see how this site uses Cookies. View our privacy policy here.