DOI:
10.1039/D3BM01448G
(Paper)
Biomater. Sci., 2024,
12, 176-186
Design of biodegradable polyurethanes and post-modification with long alkyl chains via inhibiting biofilm formation and killing drug-resistant bacteria for the treatment of wound bacterial infection†
Received
6th September 2023
, Accepted 24th October 2023
First published on 2nd November 2023
Abstract
The development of cationic polymers that simulate antimicrobial peptides to treat bacterial infections has received much research interest. In order to obtain polymers that can not only eradicate bacteria but also inhibit biofilm formation, without inducing bacterial drug resistance, a series of cationic polymers have been developed. Despite recent progress, the chemical structures of these polymers are stable, making them recalcitrant to biodegradation and metabolism within organisms, potentially inducing long-term toxicity. To overcome this limitation, herein, a novel strategy of designing biodegradable polyurethanes with tertiary amines and quaternary ammonium salts via condensation polymerization and post-functionalizing them is reported. These polymers were found to exhibit potent antibacterial activity against Staphylococcus aureus and Escherichia coli, effectively prevent the formation of Staphylococcus aureus biofilms, act quickly and effectively against bacteria and display no resistance after repeated use. In addition, the potent in vivo antibacterial effects of these antimicrobial polyurethanes in a mouse model with methicillin-resistant Staphylococcus aureus skin infection are demonstrated.
1 Introduction
The intrusion of deleterious microorganisms remains a principal cause of biosafety predicaments.1 Antibiotics, currently the primary weapon against bacterial infections, have been extensively exploited in the biomedical field, culminating in the emergence of multidrug-resistant (MDR) “super bacteria”, including methicillin-resistant Staphylococcus aureus (MRSA) and intractable Gram-negative bacteria.2,3 These resistant bacterial strains considerably augment the risk of infections contracted in both community and healthcare settings, with roughly 700
000 patients succumbing annually to diverse drug-resistant bacterial infections, largely due to drug unavailability.4,5 The situation is further compounded by the escalating resistance of bacteria to conventional antibiotics.6 Recent findings indicate that the formation of bacterial biofilms intensifies the difficulty of treating drug-resistant bacterial infections, as bacteria within biofilms exhibit formidable resistance to both antibiotics and the human immune system. Moreover, administering a higher dose of antibiotics proves futile against bacteria residing within biofilms.7 Consequently, developing innovative antibacterial technologies and materials that can eradicate biofilms, or even revolutionizing antibacterial strategies through alternative technologies and materials that do not rely on antibiotics, presents a highly promising avenue.
Recently, a novel concept of biosafety materials has been proposed,8,9 which advocates the development of innovative materials for the antibacterial field.10,11 Several antibacterial materials have been successfully synthesized and even applied in clinical settings,12 including but not limited to natural, inorganic, and organic antibacterial materials.13–15 These materials are easily obtainable and possess commendable bactericidal capabilities, owing to continuous improvements.16 However, they remain insufficient in combating bacterial biofilms, with restricted effectiveness. Studies have disclosed that a biologically active polypeptide, the antimicrobial peptide (AMP),17,18 can be induced within organisms which can effectively hinder the formation of biofilms. The primary mechanism of AMP's biofilm-inhibitory activity entails its ability to bind with the bacterial plasma membrane via electrostatic interaction, compromising membrane integrity, causing cellular content leakage, and rapidly killing bacteria.19,20 This bactericidal mechanism is less prone to inducing bacterial resistance, thereby providing a viable solution to the biofilm challenge.21 However, the high cost of antimicrobial peptides imposes constraints on their practical application.
In recent years, the design of economical polymeric antibacterial materials to simulate AMPs has emerged as a prominent research area.22 To this end, a series of cationic polymers,23 guanidine polymers,24N-halamine polymers,25 and polypeptides26 have been developed to imitate the functionality of antimicrobial peptides. These antibacterial polymers possess relatively large molecular weights and high charge densities, which facilitate their interaction with bacteria with negatively charged surfaces.27 Due to their dense antibacterial functional sites, they exhibit potent antibacterial strength. Nonetheless, their stable chemical structures make them recalcitrant to biodegradation and metabolism within organisms, potentially inducing long-term toxicity.28 Thus, developing biodegradable materials that can not only eradicate bacteria but also inhibit biofilm formation, without inducing bacterial drug resistance, would provide a genuine solution to the issue of drug-resistant bacteria, with reduced toxicity and enhanced biological safety.29–31
Herein, degradable polymers with tertiary amines (PTA) and with quaternary ammonium salts (PQA) were synthesized by polycondensation of lysine diisocyanate, diols with disulfide bonds, and diols with tertiary amine, followed by functionalization modification. Among them, diols with disulfide bonds can react with glutathione (GSH) in the bacterial microenvironment, which is key to the degradation of the polymers we synthesized. Diols with tertiary amine can react with haloalkanes with different lengths of carbon chains to facilitate the adjustment of the hydrophilic and hydrophobic balance of polymers. The impact of PQA and PTA structures on their antibacterial activity was systematically investigated. A polymer with a quaternary ammonium salt PQA-12 and a polymer with a tertiary amine PTA-6 that exhibit high antibacterial activity against both Gram-negative E. coli and Gram-positive S. aureus were synthesized by fine-tuning the degree of polymerization, the ratio of degradable monomers to amine monomers, and the length of carbon chains on quaternary amine groups to optimize the hydrophilicity/hydrophobicity balance. E. coli and S. aureus can be effectively killed by PQA-12 and PTA-6, and the repeated use of these polymers is therefore unlikely to lead to bacterial drug resistance (Scheme 1). Furthermore, low toxicity to biological cells was exhibited by PQA-12 and PTA-6. The obtained PQA-12 polymer can also effectively inhibit the formation of biofilms of S. aureus and MRSA. Finally, the efficacy of PQA-12 and PTA-6 in treating wound infections in a rat skin wound infection model was evaluated.
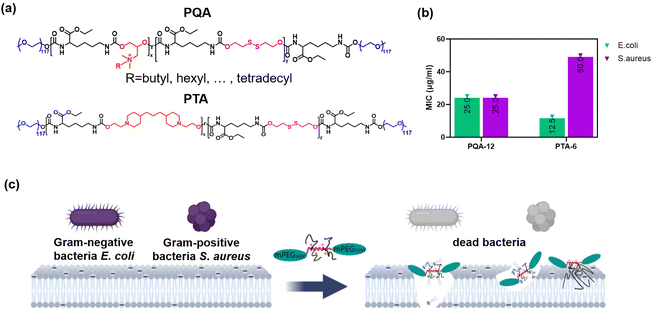 |
| Scheme 1 The antibacterial biodegradable polymers with quaternary ammonium salts and with tertiary amine groups. (a) The polymer structure with quaternary ammonium salts and with tertiary amines. (b) The MIC values against Gram-negative E. coli and Gram-positive S. aureus of PQA-12 and PTA-6. (c) Possible antibacterial mechanism. | |
2 Results and discussion
2.1 Preparation and characterization of antibacterial polymers
Random copolymers were first prepared by polymerizing commercialized lysine diisocyanate with 3-dimethylamino-1,2-propanediol and 2-hydroxyethyl disulfide at different feeding ratios. In this way, three polymer precursors with tertiary amine groups, PTA-1–3, were further end-capped with methoxy polyethylene glycol (with an average molecular weight of 5000). The subscripts x and y represent the average degree of 3-dimethylamino-1,2-propanediol and 2-hydroxyethyl disulfide, respectively. Moreover, the polymers with quaternary ammonium salts, PQA-1–15, were subsequently obtained by alkylating PTA-1–3 with five halogenated alkylating agents of different carbon chain lengths (Fig. 1a and Table S1†). Similarly, biodegradable polymers with tertiary amines, PTA-4–6, were designed by polymerizing lysine diisocyanate with varying proportions of 1,3-bis-[1-(2-hydroxyethyl)-4-piperidinyl]propane and 2-hydroxyethyl disulfide to obtain similar random copolymers, which were further end-capped with methoxy polyethylene glycol (with an average molecular weight of 5000) (Fig. 1b). The molecular parameters of PTA-1–6 are shown in Table 1 and their gel permeation chromatography is shown in Fig. S1.† Subsequently, the degree of quaternization for PQA-1–15 was determined to be within the range of 57.1% to 100% (Table 2), possibly due to the steric hindrance of the polymer chain. The chemical structure and degree of functionalization of polymers were confirmed by 1H NMR (Fig. S2–S22, ESI†). Among them, PQA-6, 11, and 12 exhibited good water solubility and could be directly dissolved in water to prepare aqueous solutions. However, the other polymers prepared could be first dissolved in DMSO before being added dropwise to water to prepare polymer aqueous solutions. In aqueous solutions, PTA-4, 5 and 6 are polymeric nanoparticles, the size of which are 128.4 nm, 131.2 nm and 138.2 nm, respectively (Fig. 1c). The as-prepared polymeric nanoparticles PTA-4–6 and PQA-1–15 exhibited positive zeta potentials in aqueous solutions, ranging from +5.5 mV to +48.3 mV (Table S2†). Taking PTA-3 and PTA-6 as examples, the biodegradation of the synthesized polymers is determined through DL-dithiothreitol (DTT) experiments. Using DTT to simulate GSH in the bacterial microenvironment, PTA-3 and PTA-6 showed significant degradation (Fig. 1d) and produced molecular fragments with a molecular weight less than 5000, due to the presence of disulfide bonds in their main chains. This indicates that the polymers with tertiary amines and those with quaternary ammonium salts may be degraded in the bacterial microenvironment.
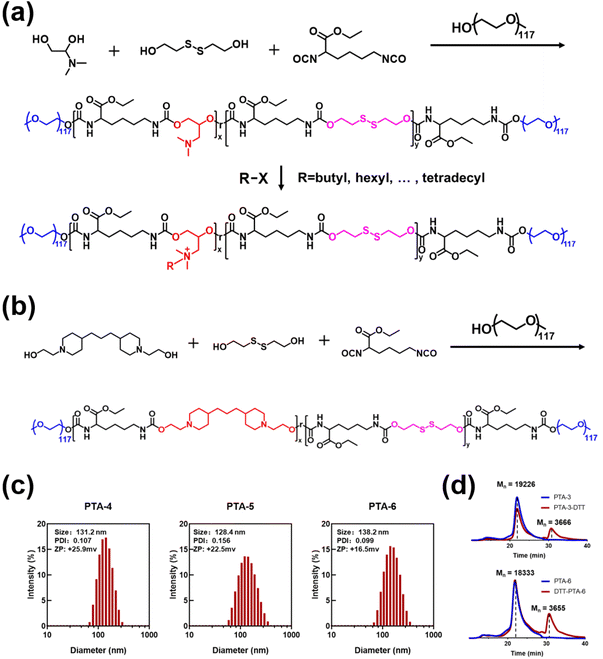 |
| Fig. 1 Chemical structures of PQA and PTA. (a and b) Synthetic route to antibacterial biodegradable polymers with quaternary ammonium salts PQA-1–15 (a) and PTA-4–6 (b). (c) The polymeric nanoparticle size and zeta potential of PTA-4–6. (d) DTT degradation of PTA-3 (above) and PTA-6 (below). | |
Table 1 Molecular parameters of the synthesized PTA-1–6
Sample |
x
|
y
|
M
n
(g mol−1) |
M
n
(g mol−1) |
PDI |
Calculated from 1H NMR spectra.
Determined from gel permeation chromatography.
|
PTA-1 |
3.5 |
4.5 |
13 165 |
18 067 |
1.74 |
PTA-2 |
7.5 |
4.5 |
14 547 |
19 672 |
1.98 |
PTA-3 |
5 |
1.5 |
12 542 |
19 226 |
2.10 |
PTA-4 |
8 |
6 |
16 436 |
17 886 |
1.98 |
PTA-5 |
14 |
7 |
20 253 |
18 685 |
2.26 |
PTA-6 |
21 |
6.5 |
23 746 |
18 333 |
2.18 |
Table 2 Molecular weight and the degree of quaternization of PQA-1–15
Sample |
M
n (g mol−1) |
Degree of quaternization (%) |
Sample |
M
n (g mol−1) |
Degree of quaternization (%) |
PQA-1 |
13 365 |
74.3 |
PQA-9 |
15 605 |
89.3 |
PQA-2 |
13 462 |
85.7 |
PQA-10 |
15 815 |
93.3 |
PQA-3 |
13 561 |
77.1 |
PQA-11 |
12 827 |
92.4 |
PQA-4 |
13 659 |
57.1 |
PQA-12 |
12 967 |
94.0 |
PQA-5 |
13 757 |
71.4 |
PQA-13 |
13 107 |
95.0 |
PQA-6 |
14 975 |
86.7 |
PQA-14 |
13 247 |
85.0 |
PQA-7 |
15 185 |
90.0 |
PQA-15 |
13 387 |
100 |
PQA-8 |
15 395 |
81.3 |
|
|
|
The antimicrobial activity of PQA-1–15 and PTA-4–6 was evaluated by determining the minimum inhibitory concentration (MIC) against two representative bacteria, Gram-positive Staphylococcus aureus and Gram-negative Escherichia coli (Table 3). In all cases, the 18 compounds exhibited antimicrobial activity against both bacteria, with generally higher activity observed against E. coli, which may be attributed to the thicker cell wall of S. aureus hindering the binding of cationic polymers to the bacterial cell membrane. Typically, the outer membrane of E. coli reduces the activity of cationic antimicrobial agents, but the biodegradable polymers PQA-14, 15 and PTA-4–6 showed the opposite effect. All three PTA compounds displayed excellent antimicrobial performance against both bacteria, with PTA-6 containing 23.6% disulfide monomers showing the best activity, with a MIC of 12.5 μg mL−1 against E. coli. However, for PQA, there was a significant difference in the antimicrobial performance depending on the length of the substituent carbon chains in the quaternary ammonium salts and the proportion of the two monomers. Briefly, PQA-12, with a MIC of 25 μg mL−1, showed the best antimicrobial activity against E. coli. Subsequently, the effect of the monomer ratios and the length of the substituent carbon chains in the quaternary ammonium salts on the antimicrobial activity of PQA was studied.
Table 3 Degree of functionalization and antimicrobial activity of the biodegradable polymers
Sample |
Degree of S–S [%] |
Carbon chain length |
MIC [μg mL−1] |
MBC [μg mL−1] |
S. aureus
|
E. coli
|
S. aureus
|
E. coli
|
PQA-1 |
56.3 |
4 |
1000 |
1000 |
1750 |
1750 |
PQA-2 |
6 |
1700 |
1700 |
1700 |
1700 |
PQA-3 |
8 |
975 |
975 |
975 |
975 |
PQA-4 |
10 |
500 |
400 |
1000 |
500 |
PQA-5 |
12 |
500 |
500 |
1000 |
1000 |
PQA-6 |
37.5 |
4 |
1000 |
500 |
>1000 |
1000 |
PQA-7 |
6 |
200 |
200 |
300 |
300 |
PQA-8 |
8 |
100 |
100 |
200 |
200 |
PQA-9 |
10 |
100 |
50 |
200 |
100 |
PQA-10 |
12 |
300 |
200 |
400 |
300 |
PQA-11 |
23.1 |
4 |
1000 |
400 |
1000 |
500 |
PQA-12 |
6 |
25 |
25 |
50 |
50 |
PQA-13 |
8 |
50 |
50 |
100 |
100 |
PQA-14 |
10 |
500 |
100 |
1000 |
200 |
PQA-15 |
12 |
500 |
100 |
1000 |
200 |
PTA-4 |
54.5 |
N/A |
100 |
50 |
200 |
100 |
PTA-5 |
33.3 |
N/A |
100 |
50 |
200 |
100 |
PTA-6 |
23.6 |
N/A |
50 |
12.5 |
100 |
25 |
For PQA with the same monomer ratios, the MIC first decreased and then increased with the increase in the length of the alkyl chains in the quaternary ammonium salts. This may be due to the increased hydrophobicity of the polymer with longer chains, which can penetrate the bacterial membrane, resulting in greater damage to membranes. However, increased hydrophobicity could also make it more difficult for the polymer to damage the bacterial membrane. This may be because the longer hydrophobic chains lead to an increase in the free energy of the molecule leaving the bacterial cell membrane, making it more inclined to remain stuck in the phospholipid bilayer,32 which can weaken the antibacterial effect of polymers. Therefore, there is an optimal length for the alkyl chains in the quaternary ammonium salts of PQA.33 With a MIC of 25 μg mL−1, PQA-12, containing 23.1% disulfide monomers, had the best antibacterial effect with a carbon chain length of 6 against both S. aureus and E. coli. For PQA-6–10, containing 37.5% disulfide monomers, PQA-9 with a carbon chain length of 10 had the best antibacterial effect with a MIC of 50 μg mL−1 against E. coli. For PQA-1–5, containing 56.3% disulfide monomers, the antibacterial effects of all were poor, with a MIC above 400 μg mL−1 against both bacteria. Overall, the antibacterial activity of PQA increased with decreasing disulfide monomer ratios.
Subsequently, the Minimum Bactericidal Concentration (MBC) test was further performed to further investigate the antibacterial activity of polymers. Unlike the MIC, which is to determine the lowest concentration of polymers that could inhibit bacterial growth, the MBC is to determine the lowest polymer density that could completely kill the bacteria. The effect of the monomer ratios and the length of the substituent carbon chains on the MBC could be possibly the same as that on the MIC. The results showed that as for PQA-1–15, with an MBC of 50 μg mL−1, PQA-12 had the best bactericidal activity against both S. aureus and E. coli. As for PTA-4–6, with an MBC of 100 μg mL−1 against S. aureus and an MBC of 25 μg mL−1 against E. coli, PTA-6 had the best bactericidal activity. Considering the MIC and MBC comprehensively, PQA-12 and PTA-6 were determined to both have the best antibacterial effect and bactericidal activity.
2.2 Antibacterial kinetics
The rapid bactericidal performance of PQA-12 and PTA-6 was assessed by studying their bactericidal kinetics against S. aureus and E. coli. The results showed that PQA-12 showed a clear concentration-dependent bactericidal rate against S. aureus, with 85% of the bacteria killed within 2 minutes at the MBC and 99% killed at 4× MBC in 10 minutes. PTA-6, on the other hand, was able to kill 99% of S. aureus within 2 minutes at 4× MBC, which is faster than PQA-12, possibly due to its higher surface positive charge, allowing it to penetrate the dense cell wall of S. aureus more rapidly. Both PQA-12 and PTA-6 demonstrated excellent bactericidal rates against E. coli, with 99% of the bacteria killed within 2 minutes at the MBC and a bactericidal rate greater than 99.99% at 4× MBC in 5 minutes. It is noteworthy that even at half the MBC, PTA-6 can still kill more than 99% of E. coli within two minutes. The above experimental results demonstrated that PQA-12 and PTA-6 could rapidly kill bacteria, making them superior to many commonly used antibiotics that typically require several hours for bactericidal effects. The fast bactericidal kinetics of the polymers could be attributed to the differences in their antibacterial mechanisms compared to antibiotics.
To further evaluate the toxicity of PQA-12 and PTA-6 to mammalian cells, a methyl thiazolyl tetrazolium (MTT) assay was conducted on Vero cells (Fig. 2e). The experimental results showed that when the concentration of PQA-12 was lower than 400 μg mL−1 (8× MBC), the cell survival rate was ≥80%, and the cell survival rate gradually decreased when the concentration exceeded 400 μg mL−1. Furthermore, when the concentration of PTA-6 was lower than 200 μg mL−1 (2× MBC for S. aureus and 8× MBC for E. coli), its cell toxicity was low, but the cell survival rate began to decrease significantly when the concentration exceeded 300 μg mL−1. Compared with PQA-12, the toxicity of PTA-6 to Vero cells was relatively high, but the concentration of PTA-6 that could cause significant damage to Vero cells was much higher than its MBC. Therefore, the cell toxicity of PQA-12 and PTA-6 at concentrations of 400 μg mL−1 and 200 μg mL−1, respectively, that could inhibit S. aureus and E. coli can be considered negligible. These results confirmed the nontoxic nature of PQA-12 and PTA-6.
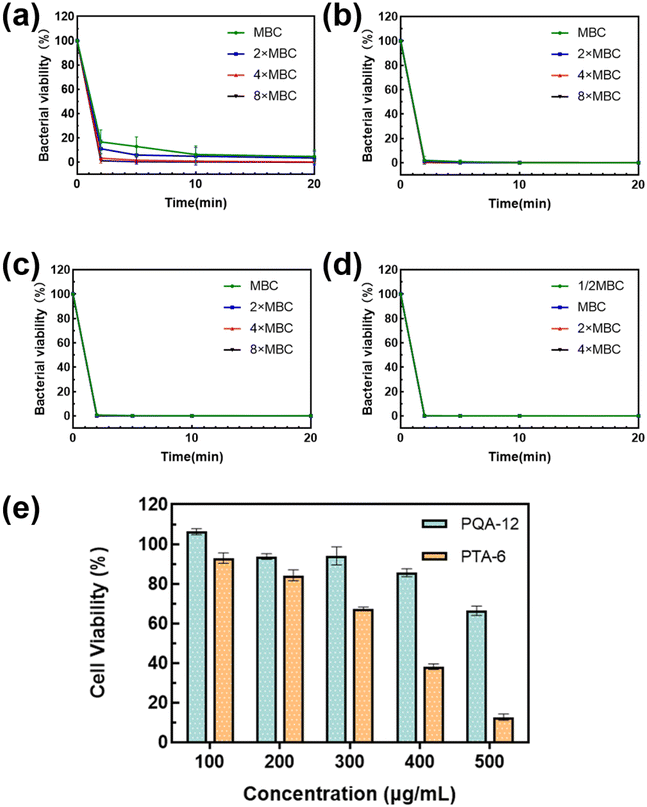 |
| Fig. 2 Antibacterial activity and kinetics. (a and b) Bacterial killing kinetics of PQA-12 (a) and PTA-6 (b) against S. aureus at MBC, 2× MBC, and 4× MBC. (c and d) Bacterial killing kinetics of PQA-12 (c) and PTA-6 (d) against E. coli at MBC, 2× MBC, and 4× MBC. (e) Cell viability of Vero cells against PQA-12 and PTA-6 at different concentrations. | |
2.3 Antibacterial mechanism
In order to explore the antibacterial mechanism of PQA-12 and PTA-6, the cytoplasmic membrane permeabilization was first evaluated. Propidium iodide (PI) was utilized to evaluate the permeability of the cytoplasmic membrane following drug administration. The results showed that a significant and dose-dependent augmentation in cytoplasmic membrane permeability was noted in S. aureus and E. coli upon treatment with PQA-12 and PTA-6, as evidenced by Fig. 3a–d. Conversely, ampicillin exhibited negligible permeability across the cytoplasmic membrane.
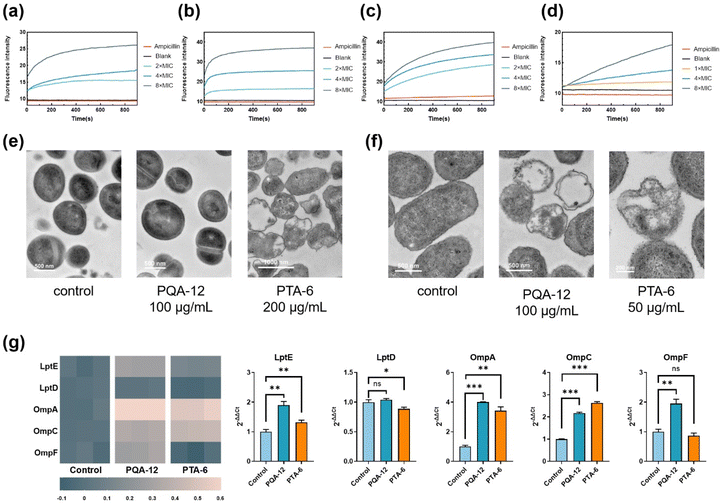 |
| Fig. 3 Antibacterial mechanism. (a and b) Cytoplasmic membrane permeabilization of S. aureus (a) and E. coli (b) after treatment with PQA-12 at different concentrations and ampicillin at 4× MIC. (c and d) Cytoplasmic membrane permeabilization of S. aureus (c) and E. coli (d) after treatment with PTA-6 at different concentrations and ampicillin at 4× MIC. (e) TEM images of S. aureus after PQA-12 and PTA-6 treatment. (f) TEM images of E. coli after PQA-12 and PTA-6 treatment. (g) Gene expression of LptE, LptD, OmpA, OmpC, and OmpF. | |
Then, the morphological changes of bacteria before and after the treatment of PQA-12 and PTA-6 were observed using a transmission electron microscope (TEM). The results showed that at 4× MIC of PQA-12 and PTA-6, significant leakage of the cytoplasm occurred in both S. aureus and E. coli, wherein the contents of bacteria were completely released from the field of view, leaving only the folded bilayer membranes (Fig. 3e and f). Furthermore, in terms of bacterial morphological changes, the structures of Gram-negative E. coli and Gram-positive S. aureus were completely destroyed after treatment with PTA-6, and their rod-shaped or spherical structures no longer existed. Moreover, the results indicated that E. coli after treatment with PQA-12 showed similar morphological changes, while the morphological changes of S. aureus after treatment with PQA-12 were less apparent, which was consistent with the fact that the antibacterial activity of the polymers against S. aureus was inferior to that against E. coli. These results confirmed that at low concentrations, PQA and PTA lead to an increase in bacterial membrane permeability. More importantly, it can lead to the dissolution of the bacterial membranes.
To gain further insight into the mechanism behind the enhanced bactericidal efficacy of polymers against Gram-negative bacteria, transcriptomic analysis of E. coli outer membrane-related genes was performed. Specifically, the change in the expressions of genes of the outer membrane in E. coli was examined before and after treatment with PQA-12 and PTA-6. It is known that lipopolysaccharides (LPSs) are lipids present on the bacterial outer membranes to maintain membrane stability. LptD is responsible for regulating LPS synthesis, whereas LptE facilitates LPS transportation. Our findings indicated that treatment with both PQA-12 and PTA-6 resulted in an upregulation of LptE expression in E. coli, while LptD expression remained unchanged or slightly decreased. Bacteria can increase LPS transportation by producing an excess of LptE to sustain membrane stability. Therefore, it is postulated that PQA-12 and PTA-6 did not affect LPS synthesis pathways, but rather had an impact on LPS transportation.
OmpA is a transmembrane protein that maintains the stability of the outer membranes and is a conserved component of the membranes. OmpC and OmpF are responsible for regulating the permeability of outer membrane porins. What's more, OmpF transports ions. The results indicated that these three types of proteins play a crucial role in maintaining the stability of the cell membranes in Gram-negative bacteria. In E. coli treated with PQA-12, the expressions of OmpA, OmpC, and OmpF were upregulated by 3.0, 1.2, and 1.0 times, respectively. In E. coli treated with PTA-6, the expressions of OmpA and OmpC were upregulated by 2.4 and 1.6 times, while the expression of OmpF was unchanged. Notably, the expression of OmpA was significantly increased in E. coli treated with PTA-6, suggesting that the ability to induce bacterial outer membrane disruption could be the reason that PTA-6 had better antibacterial activity against Gram-negative bacteria.
In summary, similar to AMPs, PQA-12 and PTA-6 could attach to the bacterial surface via electrostatic interactions, disrupting the cell membranes. The delicate balance of hydrophilicity/hydrophobicity of PQA-12 and PTA-6 facilitated the targeting of bacteria via electrostatic and cellular interactions. These findings collectively suggested that the antibacterial effects of PQA-12 and PTA-6 could stem from their interactions with the bacterial cell membranes, akin to AMPs. This could be attributable to the amphiphilic nature of PQA-12 and PTA-6. It is widely believed that Gram-negative bacteria are more challenging to eliminate than Gram-positive bacteria because of their highly impervious outer membrane barriers, which could serve as an additional defensive mechanism. However, PTA-6 was shown to demonstrate superior antibacterial activity against Gram-negative bacteria.
2.4 Antibiofilm activity
In the previous section, we have already discussed that PQA-12 and PTA-6 have a similar antibacterial mechanism to AMPs. Next, we investigated whether they are similar to AMPs in inhibiting biofilm formation. Chronic infections are frequently associated with bacterial biofilms, which are characterized by long treatment times and low cure rates. Biofilms are microbial structures that commonly harbor a range of microorganisms encased in polymeric substances that impede the penetration of antimicrobial agents. In this study, the capacity of PQA-12 and PTA-6 to inhibit the formation of S. aureus biofilms was first assessed by using the MTT assay (Fig. 4a). Furthermore, the ability of PQA-12 and PTA-6 to inhibit MRSA biofilm formation was assessed in the same protocol. PQA-12 exhibited a dose-dependent effect on inhibiting the development of S. aureus biofilms (Fig. 4b). Treatment with PQA-12 at 4× MIC resulted in a 95.91% reduction in bacterial viability within the biofilms. Moreover, increasing the concentration of PQA-12 to 8× MIC resulted in a substantial reduction in bacterial viability within the biofilms to 0.56%. Similarly, at 8× MIC, PTA-6 resulted in a bacterial survival rate of 0.83% (Fig. 4c). These results together indicated that the synthesized polymers PQA-12 and PTA-6 are highly effective at inhibiting the formation of S. aureus biofilms.
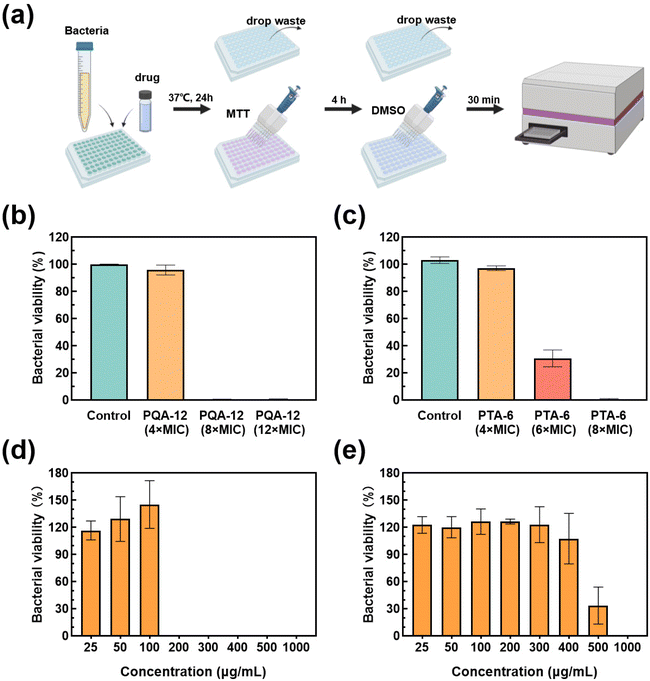 |
| Fig. 4 Antibiofilm activity of PQA-12 and PTA-6. (a) Schematic diagram of the experimental processes. (b and c) The ability of PQA-12 (b) and PTA-6 (c) to inhibit S. aureus biofilm formation. Data are shown as mean ± SD (n = 4 in each group). (d and e) The ability of PQA-12 (d) and PTA-6 (e) to inhibit MRSA biofilm formation. Data are shown as mean ± SD (n = 3 in each group). | |
The ability of the two polymers was further evaluated to combat methicillin-resistant S. aureus (MRSA) biofilm formation. The results showed that when the concentration of PQA-12 reached 200 μg mL−1, it could completely inhibit the formation of biofilms (Fig. 4d). In contrast, PTA-6 was poor in inhibiting MRSA biofilm formation, and the bacterial viability of biofilms only decreased to 33.74% when the concentration of PTA-6 reached 500 μg mL−1. Therefore, the results strongly suggested that PQA-12 could disrupt the biofilms of clinically relevant MRSA and kill the pathogens, proving its efficacy as an excellent antibacterial agent.
2.5 Development of bacterial resistance to antibiotics and polymers
Antimicrobial drug resistance is closely associated with the overuse of antibiotics, becoming a great biosafety concern. It is known that AMPs hardly induce any bacterial resistance. Considering the antimicrobial mechanisms of the synthesized polymers, their potential to induce bacterial resistance was also evaluated. The antimicrobial resistance induced by repeated use of PQA-12 and PTA-6 at 0.5× MIC was investigated (Fig. 5a). For comparison, the conventional antibiotic ampicillin was also used. The results showed that after 30 consecutive passages, the MIC values of PQA-12 and PTA-6 remained constant (Fig. 5b). In contrast, when S. aureus was grown to the 20th generation, the MIC values of ampicillin increased significantly to eight times the original MIC value, indicating that the bacteria had developed resistance to ampicillin. Furthermore, the experiments on E. coli showed similar results, where the MIC value of ampicillin increased to eight times the original MIC value when the bacteria were grown to the 20th generation, and the MIC value of PQA-12 also increased by a factor of two. The MIC value of PTA-6 increased by a factor of two after 10 passages, but did not increase further in the subsequent passages. The experimental results demonstrated that the polymers PQA-12 and PTA-6 did not induce resistance in S. aureus, and therefore have certain potential for antibacterial application.
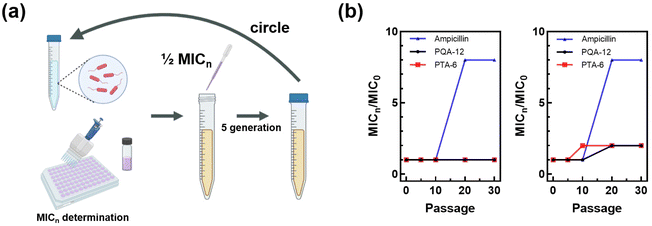 |
| Fig. 5 Overcoming the bacterial drug resistance by PQA-12 and PTA-6. (a) Schematic diagram of the treatment process. (b) Ratios of MICn/MIC0 in S. aureus (left) and E. coli (right) treated with PQA-12, PTA-6 and ampicillin. | |
2.6
In vivo antibacterial activity
Finally, as shown in Fig. 6a, the antibacterial efficacy of PQA-12 and PTA-6 in wound disinfection was evaluated in a MRSA infection model on mouse skin in vivo. During a typical surgical procedure, skin biopsy punches with a diameter of 6 mm were symmetrically created near the hind limb position on the back of rats. After 4 h of MRSA (1 × 107 CFU mL−1) infection, single doses of PQA-12 (15 μL, 1.2 mg mL−1), PTA-6 (15 μL, 0.8 mg mL−1), and vancomycin were administered for treatment. After a 24 h treatment, the bactericidal activity of PQA-12, PTA-6, and vancomycin against MRSA in vivo demonstrated similar potency (1.6 × 105 CFU mL−1) compared to PBS (9 × 105 CFU mL−1). Furthermore, the progress of wound healing indicated that when treated with PQA-12 and PTA-6, the rats exhibited faster recovery than those treated with vancomycin (Fig. 6b and c). During the first 7 days of polymer treatment, the wound shrinkage rate in mice was slightly faster than that of the PBS treatment. However, on the 9th day, the skin of the rats treated with PQA-12 and PTA-6 almost fully healed, while the wounds of the mice treated with PBS still had 35% left to heal. Histological analysis further showed a reduced inflammatory infiltrate in the PQA-12-, PTA-6- and vancomycin-treated wounds (Fig. 6d). This differs from the results from the PBS-treated wounds, which exhibited a pronounced inflammatory infiltrate and visible calcification. In addition, more newly forming collagen and blood vessels were observed in the PQA-12-, PTA-6- and vancomycin-treated wounds than in the PBS ones. Although the potency of PQA-12 and PTA-6 against MRSA is similar to that of vancomycin, they are not antibiotics and their use will not lead to further development of bacterial resistance. These results indicated that PQA-12 and PTA-6 possessed excellent in vivo bacterial killing and wound healing properties.
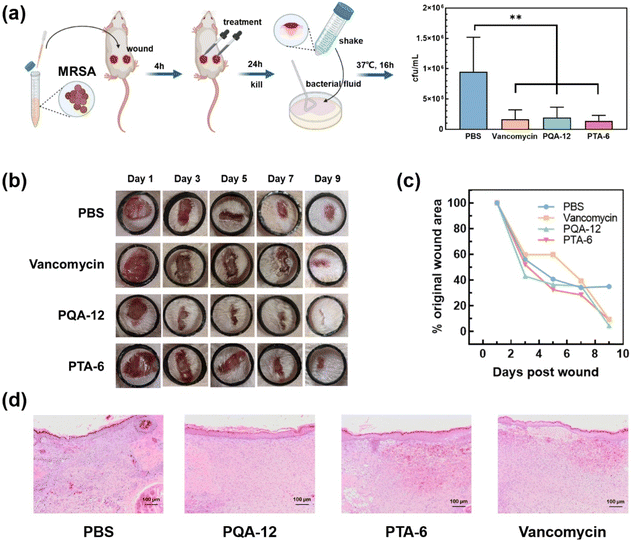 |
| Fig. 6
In vivo antibacterial activity study of PQA-12 and PTA-6 against MRSA using a rat full-thickness wound infection model. (a) Schematic illustration of an MRSA infection model on mouse skin, as well as antimicrobial activity in vivo. (b) The digital images of MRSA-infected mouse skin wounds after being treated with PBS, vancomycin, PQA-12 and PTA-6 at 0, 1, 3, 5, 7, and 9 days. (c) The changes seen in the mouse skin wound size when treated with PBS, vancomycin, PQA-12 and PTA-6. (d) The H&E staining of wound tissue sections at 9 days after treatment with PBS, PQA-12, PTA-6 and vancomycin. | |
3 Conclusion
A novel strategy of designing biodegradable polyurethanes with tertiary amines and quaternary ammonium salts via condensation polymerization and post-functionalization is reported here. The current research shows that the antimicrobial properties of these biodegradable polyurethanes rely on the hydrophilicity/hydrophobicity balance. By optimizing this balance, both polyurethanes with tertiary amines and quaternary ammonium salts exhibit potent antibacterial activity against S. aureus and E. coli. These polymers act quickly and effectively against bacteria and do not induce resistance after repeated use. We also discovered that these polyurethanes effectively prevent the formation of S. aureus biofilms. Systematic investigation suggests that the antibacterial mechanism of these polymers is similar to that of antimicrobial peptides, which can induce membrane disruption. The amphiphilic structure of the polyurethanes is possibly responsible for this mechanism. However, the antibacterial mechanisms against E. coil of biodegradable polyurethanes with tertiary amines and quaternary ammonium salts differ slightly, which requires further investigation of the changes in bacterial gene expression after treatment. In addition, we demonstrated the potent in vivo antibacterial effects of these antimicrobial polyurethanes in a mouse model with MRSA skin infection. These synthetic polyurethanes can be degraded into fragments in the bacterial microenvironment and then metabolized by organisms, being less toxic and more environmentally friendly. And we will further investigate the impact of the degradation products of these polymers on the metabolism of organisms. Our findings suggest that these synthesized polyurethanes with tertiary amines and quaternary ammonium salts are excellent candidates for innovative antimicrobial agents.
Ethical statement
All animal procedures were performed in accordance with the Guidelines for Care and Use of Laboratory Animals of Northwest University of China and approved by the Laboratory Animal Management and Ethics Committee of Northwestern University of China (NWU-AWC-20220409M).
Conflicts of interest
There are no conflicts to declare.
Acknowledgements
This work is supported by the National Key Research & Development Program (2022YFC2603900), the Central Government Guided Local Science and Technology Development Fund (Hebei) (216Z2601G), and the National Natural Science Foundation of China (22175189). The schematic illustrations in Scheme 1 and Fig. 4–6 were created with BioRender.com.
References
- Q. Yao,
et al., Bacterial infection microenvironment-responsive enzymatically degradable multilayer films for multifunctional antibacterial properties, J. Mater. Chem. B, 2017, 5(43), 8532–8541 RSC.
- M. Kishita,
et al., Increase in the frequency of community-acquired methicillin-resistant Staphylococcus aureus clones among inpatients of acute care hospitals in the Kyoto and Shiga regions, Japan, J. Infect. Chemother., 2023, 29(5), 458–463 CrossRef CAS PubMed.
- J. N. Pendleton, S. P. Gorman and B. F. Gilmore, Clinical relevance of the ESKAPE pathogens, Expert Rev. Anti-Infect. Ther., 2013, 11(3), 297–308 CrossRef CAS.
- C. J. L. Murray,
et al., Global burden of bacterial antimicrobial resistance in 2019: a systematic analysis, Lancet, 2022, 399(10325), 629–655 CrossRef CAS.
- U. Hofer, The cost of antimicrobial resistance, Nat. Rev. Microbiol., 2019, 17(1), 3–3 CrossRef CAS PubMed.
- F. Huang,
et al., A dynamic covalent polymeric antimicrobial for conquering drug-resistant bacterial infection, Exploration, 2022, 2(5), 20210145 CrossRef PubMed.
- J. W. Costerton, P. S. Stewart and E. P. Greenberg, Bacterial biofilms: A common cause of persistent infections, Science, 1999, 284(5418), 1318–1322 CrossRef CAS PubMed.
- J. Ding, H. Xiao and X. Chen, Advanced biosafety materials for prevention and theranostics of biosafety issues, Biosaf. Health, 2022, 4(2), 59–60 CrossRef PubMed.
- Y. Zhao,
et al., Advanced bioactive nanomaterials for biomedical applications, Exploration, 2021, 1(3), 20210089 CrossRef PubMed.
- J. Karges, Combination of chemistry and material science to overcome health problems, Biosaf. Health, 2022, 4(2), 64–65 CrossRef PubMed.
- Y. Zhang,
et al., Biosafety materials: Ushering in a new era of infectious disease diagnosis and treatment with the CRISPR/Cas system, Biosaf. Health, 2022, 4(2), 70–78 CrossRef.
- H. Xiao,
et al., Maximizing Synergistic Activity When Combining RNAi and Platinum-Based Anticancer Agents, J. Am. Chem. Soc., 2017, 139(8), 3033–3044 CrossRef CAS.
- L. Huang,
et al., Synergistic Combination of Chitosan Acetate with Nanoparticle Silver as a Topical Antimicrobial: Efficacy against Bacterial Burn Infections, Antimicrob. Agents Chemother., 2011, 55(7), 3432–3438 CrossRef CAS PubMed.
- A. Y. Mansilla,
et al., Evidence on antimicrobial properties and mode of action of a chitosan obtained from crustacean exoskeletons on Pseudomonas syringae pv. tomato DC3000, Appl. Microbiol. Biotechnol., 2013, 97(15), 6957–6966 CrossRef CAS PubMed.
- D. Wei,
et al., Photo-Reduction with NIR Light of Nucleus-Targeting Pt(IV) Nanoparticles for Combined Tumor-Targeted Chemotherapy and Photodynamic Immunotherapy, Angew. Chem., Int. Ed., 2022, 61(20), e202201486 CrossRef CAS PubMed.
- H. Zhou,
et al., Degradable Pseudo Conjugated Polymer Nanoparticles with NIR-II Photothermal Effect and Cationic Quaternary Phosphonium Structural Bacteriostasis for Anti-Infection Therapy, Adv. Sci., 2022, 9(16), 2200732 CrossRef CAS PubMed.
- D. Wei and X. Zhang, Biosynthesis, bioactivity, biotoxicity and applications of antimicrobial peptides for human health, Biosaf. Health, 2022, 4(2), 118–134 CrossRef.
- B. P. Lazzaro, M. Zasloff and J. Rolff, Antimicrobial peptides: Application informed by evolution, Science, 2020, 368(6490), 487 CrossRef PubMed.
- A. Som,
et al., Synthetic mimics of antimicrobial peptides, Biopolymers, 2008, 90(2), 83–93 CrossRef CAS PubMed.
- A. W. Young,
et al., Structure and antimicrobial properties of multivalent short peptides, MedChemComm, 2011, 2(4), 308–314 RSC.
- W. C. Wimley, Describing the Mechanism of Antimicrobial Peptide Action with the Interfacial Activity Model, ACS Chem. Biol., 2010, 5(10), 905–917 CrossRef CAS.
- Z. Geng, Z. Cao and J. Liu, Recent advances in targeted antibacterial therapy basing on nanomaterials, Exploration, 2023, 3(1), 20210117 CrossRef PubMed.
- P. Gilbert and L. E. Moore, Cationic antiseptics: diversity of action under a common epithet, J. Appl. Microbiol., 2005, 99(4), 703–715 CrossRef CAS PubMed.
- R. G. Gaudet,
et al., A human apolipoprotein L with detergent-like activity kills intracellular pathogens, Science, 2021, 373(6552), eabf8113 CrossRef CAS PubMed.
- Y. Xue, H. Xiao and Y. Zhang, Antimicrobial Polymeric Materials with Quaternary Ammonium and Phosphonium Salts, Int. J. Mol. Sci., 2015, 16(2), 3626–3655 CrossRef CAS PubMed.
- J. Sun,
et al., High Antibacterial Activity and Selectivity of the Versatile Polysulfoniums that Combat Drug Resistance, Adv. Mater., 2021, 33(41), e2104402 CrossRef.
- W. Liu,
et al., ECM-mimetic immunomodulatory hydrogel for methicillin-resistant Staphylococcus aureus-infected chronic skin wound healing, Sci. Adv., 2022, 8(27), eabn7006 CrossRef CAS PubMed.
- S. Yan,
et al., Biodegradable Supramolecular Materials Based on Cationic Polyaspartamides and Pillar[5]arene for Targeting Gram-Positive Bacteria and Mitigating Antimicrobial Resistance, Adv. Funct. Mater., 2019, 29(38), 1904683 CrossRef.
- Y. Wang,
et al., Nanoparticle-mediated convection-enhanced delivery of a DNA intercalator to gliomas circumvents temozolomide resistance, Nat. Biomed. Eng., 2021, 5(9), 1048–1058 CrossRef CAS PubMed.
- D. Tang,
et al., Self-Sacrificially Degradable Pseudo-Semiconducting Polymer Nanoparticles that Integrate NIR-II Fluorescence Bioimaging, Photodynamic Immunotherapy, and Photo-Activated Chemotherapy, Adv. Mater., 2022, 34(34), 2203820 CrossRef CAS.
- Y. Yu,
et al., Biodegradable Polymer with Effective Near-Infrared-II Absorption as a Photothermal Agent for Deep Tumor Therapy, Adv. Mater., 2022, 34(4), e2105976 CrossRef.
- L. Zheng,
et al., Flexible Modulation of Cellular Activities with Cationic Photosensitizers: Insights of Alkyl Chain Length on Reactive Oxygen Species Antimicrobial Mechanisms, Adv. Mater., 2023, 35(35), 2302943 CrossRef CAS.
- L. Zheng,
et al., Molecular Sizes and Antibacterial Performance Relationships of Flexible Ionic Liquid Derivatives, J. Am. Chem. Soc., 2020, 142(47), 20257–20269 CrossRef CAS PubMed.
|
This journal is © The Royal Society of Chemistry 2024 |