DOI:
10.1039/D3BM00854A
(Paper)
Biomater. Sci., 2024,
12, 164-175
Immunogenic dead cells engineered by the sequential treatment of ultraviolet irradiation/cryo-shocking for lung-targeting delivery and tumor vaccination†
Received
17th May 2023
, Accepted 26th October 2023
First published on 10th November 2023
Abstract
Chemoimmunotherapy is a promising strategy in tumor treatments. In this study, immunogenic dead cells were engineered by the sequential treatment of live tumor cells with ultraviolet (UV) irradiation and cryo-shocking. The dead cells could serve as a lung-targeting vehicle and tumor vaccine after differential loading of the chemo-drug 10-hydroxycamptothecin (HCPT) and immune adjuvant Quillaja saponin-21 (QS-21) via physical absorption and chemical conjugation, respectively. After intravenous administration, the dead cells could be trapped in pulmonary capillaries and could fast release HCPT to enhance the drug accumulation in local tissues. Further, the immunogenic dead cells elicited antitumor immune responses together with the conjugated adjuvant QS-21 to achieve the elimination and long-term surveillance of tumor cells. In a lung tumor-bearing mice model, this drug-delivery system achieved synergistic antitumor efficacy and prolonged the survival of mice.
1. Introduction
Lung cancer is a malignant pulmonary disease with a high morbidity and fatality rate.1–6 Every year, lung cancer affects nearly two million individuals around the world and has become the primary reason of cancer-related deaths.7,8 Surgery is commonly the primary choice in lung cancer treatments; however, due to the insidious and atypical clinical symptoms of lung cancer, approximately two-thirds of patients are diagnosed with advanced stage cancer, who are not suitable for conventional surgical operations.9–11 Thus, chemotherapy is still an important standard of care for lung cancer treatments.12,13 Unfortunately, the 5-year survivorship of lung cancer is only 20.5%, mainly due to the severe adverse effects of conventional therapies and a high recurrence rate after the final dose.14–16
In recent years, chemoimmunotherapy, which combines chemotherapy and immunotherapy, has been applied as a way to strengthen traditional chemotherapy via boosting both the auto immune system to kill residual tumor cells and for the long-term surveillance of cancer recurrence.17–23 Among these therapies, tumor vaccines are regarded as a hotspot, and several tumor vaccines in combination with chemotherapy are now in clinical trials for lung cancer treatments.24–27 For instance, the tumor polypeptide antigen vaccine was utilized in combination with pemetrexed, carboplatin, and PD-1 antibody (anti-PD-1) for the treatment of metastatic non-small cell lung cancer in Phase I trials, achieving a decrease in tumor size in at least 21 patients and high immune responses within one year.28 Nevertheless, the therapeutic effects of tumor cell-based vaccines are usually insufficient in vivo because of the poor immunogenicity of tumor cells.29–31 Therefore, how to efficiently improve the immunogenicity of the tumor vaccine and activate antigen presenting cells (APCs) are the critical factors to improve the therapeutic efficacy of tumor cell-based cancer vaccines.
Recent investigations have revealed that several external stimuli, such as chemicals, ultraviolet (UV) irradiation, and X-ray irradiation, might cause immunogenic cell death (ICD) and increase the immunogenicity of tumor cells.32–38 Inspired by this phenomenon, we engineered immunogenic dead cells (UV-Cryo cells) by sequentially treating live cancer cells with UV irradiation and cryo-shocking. Besides serving as a passive lung-targeting delivery vehicle owing to the cellular size, the UV-cryo cells could further strengthen the antigen uptake/presentation by APCs to elicit efficient immune responses as a cancer vaccine.39–44 After differentially loading the antitumor drug 10-hydroxycamptothecin (HCPT) and immunologic adjuvant Quillaja saponin-21 (QS-21) via physical absorption and chemical conjugation, respectively, this system (HCPT&QS-21/UV-Cryo cell) could realize the fast release of chemo-drugs in lung and a long retention of the immune adjuvant, achieving the synergistic efficacy between chemo- and immunotherapy.
2. Materials and methods
2.1 Materials
Egg phospholipids, cholesterol, and octadecylamine were purchased from Aladdin Reagent Co., Ltd (Shanghai, China), and DSPE-PEG-Mal was provided by Ponsure Biotechnology Co., Ltd (Shanghai, China). HCPT and QS-21 were supplied by Nanjing Plant Origin Biological Technology Co., Ltd (Nanjing, China) and Shanghai Yuanye Bio-Technology Co., Ltd (Shanghai, China), respectively. Cell counting kit-8 (CCK-8) was supplied by Dalian Meilun Biotechnology (Dalian, China). Calcein/PI cell viability kit was brought from Beyotime Biotechnology Co., Ltd (Shanghai, China). Annexin V-FITC/PI Apoptosis Detection Kit was supplied by Yeasen Biotechnology Co., Ltd (Shanghai, China). Fluorescence-labeled antibodies of anti-CD11c, anti-CD86, anti-CD80, anti-CD3, anti-CD8, anti-CD4, and enzyme-linked immunosorbent assay (ELISA) kits of IL-6, IFN-γ, and TNF-α were brought from Biolegend (USA). Anti-CRT antibodies were purchased from Aibokang (Beijing) Biomedical Technology Co., Ltd.
Murine lung cancer cell lines LLC and LLC-luc were bought from Shanghai Fuheng Biotechnology Co., Ltd (Shanghai, China), and cultured in DMEM (high glucose) cell culture medium with 10% FBS and 1% penicillin/streptomycin at 37 °C in a 5% CO2 incubator. Murine dendritic cell 2.4 line (DC2.4) was brought from Shanghai Biological Technology Co., Ltd (Shanghai, China) and cultured in RPMI-1640 cell culture medium with 10% FBS and 1% penicillin/streptomycin at 37 °C in a 5% CO2 incubator.
C57BL/6J mice (female, 18–22 g) were supplied by Shanghai University of Traditional Chinese Medicine (Shanghai, China). Shanghai University of Traditional Chinese Medicine's ethical committee assessed and approved the protocols for treating the animals used in this research (Approval No. PZSHUTM211213004). Once the weight loss of the mice reached 15%, the mice were euthanized according to IACUC guidelines.
2.2 Preparation and characterization of UV-Cryo cells
LLC cells were inoculated into culture dishes and exposed to UV irradiation (1.8 mJ cm−2). After culturing for 24 h, the cells were scraped with cell scrapers and dispersed in the cell suspension medium (80% DMEM with 20% FBS) at a density of 1 × 106–1 × 107 cells per mL. Then the cells were quickly immersed in liquid nitrogen for at least 8 h. Before further application, these cells should be quickly thawed at 37 °C water bath and washed by PBS three times.
The structure of the UV-Cryo cells was characterized by confocal laser scanning microscopy (CLSM) and scanning electron microscopy (SEM). In brief, for CLSM characterization, the UV-Cryo cells were stained with rhodamine-labeled phalloidin (30 min) and Hoechst 33342 (10 min). The stained cells were thoroughly rinsed three times with PBS, and then added to the confocal culture dish for observation. Before staining the live cells, 4% paraformaldehyde was added for 15 min for fixation, and 0.1% Triton X-100 was utilized for 20 min to destroy the cell membrane. Then the live cells were stained with rhodamine-labeled phalloidin (30 min) and Hoechst 33342 (10 min). Before adding the cells into the confocal dish, it was necessary to thoroughly wash the cells with PBS to remove residual staining solution.
For SEM detection, 3.5% glutaraldehyde (4 h) and 1% osmic acid (1 h) were both adopted. After fixation, the cells were washed with PBS and dehydrated with gradient ethanol (30%, 50%, 70%, 80%, 90%, and 95% once for 15 min, then 100% twice for 20 min). Then a mixture solution of ethanol and isoamyl acetate (v/v = 1/1) was used to treat the cells for 30 min, and the cells were suspended in pure isoamyl acetate overnight. After drying, the cells were dropped on a silica plate and observed after being sprayed with a thin film of gold.
The cell viability of the UV-Cryo cells was initially assessed through the Calcein/PI cell viability kit, and the cell proliferation capability was assessed using flow cytometry following staining with the Annexin V-FITC/PI Apoptosis Detection Kit, according to the manufacturer's protocol. For quantitative analysis, the cell proliferation capability was further analyzed by CCK-8 assay. In brief, live LLC cells, UV cells, and UV-Cryo cells were all suspended in the cell culture medium (80% DMEM with 20% FBS) and added to 96-well plates (3000 cells per well). After culturing for 0.5, 24, 48, and 72 h, 10 μL CCK-8 solution was added into each well of the 96-well plate. After incubating for another 2 h, the absorbance of each sample was examined at 450 nm using a microplate reader.
2.3 Biosafety evaluation of the UV-Cryo cells
Three groups of healthy C57BL/6 mice were formed at random (n = 5). Saline, live LLC cells, or UV-Cryo cells (2 × 106 cells per mouse) were intravenously (i.v.) injected into the mice, respectively. The survival of the mice in each group was tracked and monitored until day 180. After the mice were sacrificed at day 180, the primary organs (hearts, livers, spleens, lungs, and kidneys) were collected for H&E staining assay.
2.4 Protein expressions of the UV-Cryo cells
The whole cell proteins expressed by UV-Cryo cells were analyzed by SDS-polyacrylamide gel electrophoresis (SDS-PAGE). RIPA lysis and extraction buffer with a protease inhibitor cocktail were added into the cells to extract the proteins. The BCA assay was used to detect the protein concentration. The loaded samples were prepared with Laemmli sample buffer at 20 μg protein per well. The proteins of the loaded samples were denatured for 10 min at 95 °C before being detected by SDS-PAGE under a Stain-Free™ Precast Gel. A Bio-Rad ChemiDoc MP imaging system was applied for the subsequent imaging.
The expression of calreticulin (CRT) on UV-Cryo cells was investigated by flow cytometry and western blotting. UV-Cryo cells were collected and suspended in PBS (with 1% FBS), and then stained with FITC labeled-CRT antibody for 1 h. Flow cytometry was applied to analyze the fluorescence intensity after the cells were washed with PBS.
The relative quantitative expression of CRT was further analyzed by western blotting. After SDS-PAGE assay, the separated proteins on the gel were transferred to a PVDF membrane. After sealing, the membrane was placed into a dish and incubated with the anti-CRT primary antibody at 4 °C for 12 h. Then, TBST was used to wash the membrane with 3 times and the secondary antibody was subsequently adopted to incubate with the membrane at room temperature for 30 min. Finally, ECL luminescent solution was added into the membrane, and the PVDF film was put into a dark box for exposure and protein band observation.
2.5 Preparation of the drug-loaded UV-Cryo cells
Drug-loaded UV-Cryo cells were prepared in two steps typically, namely the preparation of drug-loaded liposome, and incubation/modification of drug-loaded liposome on the UV-Cryo cells.
In brief, drug-loaded liposomes were prepared by a film dispersion method. The lipid composition of the HCPT-loaded liposomes was lecithin, cholesterol, and octadecylamine. After dissolving HCPT and the lipid materials in a mixed solution of CH3OH and CH2Cl2 (1
:
1, v/v), the organic solvent was evaporated by rotary evaporation. Then, the HCPT-loaded liposomes could be obtained after hydration for 1 h at 37 °C and sequential filtration by 0.45 and 0.22 μm filters. Dynamic light scattering (DLS) spectrophotometry was applied to analyze the particle size and ζ-potential of the drug-loaded liposomes.
The preparation of QS-21-loaded liposomes was performed in reference to several previous literature reports.45,46 In brief, the liposomes were prepared based on a similar film dispersion method but with a different lipid composition (8 mg lecithin, 2 mg cholesterol, and 0.2 mg DSPE-PEG-Mal). After dissolving the lipids in CH3OH, the organic solvent was evaporated by rotary evaporation. Then, QS-21-containing aqueous solution (0.5 mg QS-21) was added for hydration for 1 h at 37 °C. To obtain QS-21-loaded liposomes with a relatively uniform particle size, the obtained products were sequentially filtrated through 0.45 and 0.22 μm filters.
The stability of the liposomes was evaluated to reflect the stability of the liposomes through measuring their particle size. Afterwards, UV-Cryo cells were suspended in HCPT/liposome solution and incubated for 30 min. After centrifugation (500g, 5 min) to remove the unloaded liposomes, the HCPT-loaded UV-Cryo cells were obtained. Then QS-21/liposome solution was added into the above HCPT/UV-Cryo cell solution and incubated for a further 30 min. After the click reaction between the maleimide groups on the QS-21/liposome and thiol groups on HCPT/UV-Cryo cells, HCPT and QS-21 co-loaded UV-Cryo cells (HCPT&QS21/UV-Cryo cells) were obtained.
To evaluate the drug-loading state, coumarin 6 and DiI were adopted as the fluorescence drug models of HCPT and QS-21. Coumarin 6 and DiI co-loaded UV-Cryo cells were prepared as stated above and observed by fluorescence inversion microscopy.
2.6 Differential drug release of the HCPT&QS-21/UV-Cryo cells
Coumarin 6 was adopted as the model drug. In detail, cationic coumarin 6-loaded liposome and maleimide-modified coumarin 6-loaded liposome were prepared and further incubated with UV-Cryo cells to mimic the different loading state of the drugs. In vitro drug release was evaluated with a peristaltic pump pretreated with PBS (2% FBS). The shearing stress was adjusted by changing the liquid flow based on Poiseuille's law as follows:47
μ: viscosity coefficient of PBS solution containing 2% FBS (0.01 dyne s cm−2), Q: volume flow (mL min−1), R: radius of silica gel tube.
Coumarin 6-loaded UV-Cryo cells were dispersed in 10 mL PBS and circulated by a peristaltic pump under the shearing stress of 6 Pa (the typical stress of lung capillaries39) at 37 °C. The circulation medium was transferred into a tube at specific timepoints. The fluorescence intensity of the circulation medium was evaluated following filtration with 0.45 μm and 0.22 μm filters, successively. The unreleased drug percentage was calculated by the following equation:
2.7
In vivo distribution of UV-Cryo cells
UV-Cryo cells were labeled with Cy5.5 by incubating UV-Cryo cells in Cy5.5-NHS solution for 1 h. The mice were divided into two groups and received an i.v. injection of Cy5.5-UV-Cryo cells and free Cy5.5 at a Cy5.5 dose of 10 nmol kg−1 (8 μg per mouse), respectively. The mice were separately sacrificed at 0.5, 1, or 1.5 h post-injection (three mice for each point), and an in vivo optical imaging system (IVIS) was applied for the fluorescence analysis of the typical organs (hearts, livers, spleens, lungs, and kidneys).
2.8
In vitro activation of dendritic cells
DC2.4 cells were planted at a density of 1.5 × 105 cells per well on 6-well plates. Saline, QS-21/Cryo cells, and QS-21/UV-Cryo cells were added into the wells (QS-21 0.75 μg per well), respectively. DC2.4 cells were incubated for 48 h before being scraped with cell scrapers, then stained with FITC-CD11c, APC-CD80, and PE-CD86 antibodies, and the CD80+CD86+ cells were analyzed by flow cytometry beneath the gate on CD11c-positive cells.
2.9
In vivo antitumor efficacy
On day 0, 5 × 106 LLC cells were i.v. injected into C57BL/6 mice. On days 7, 9, 11, and 13, various preparations were i.v. administrated to the mice in the saline, HCPT, UV-Cryo cell, QS-21/UV-Cryo cell, and HCPT&QS-21/UV-Cryo cell groups (2.5 mg kg−1 HCPT, 2 × 106 UV-Cryo cells per mouse). The weight change of each mouse during treatment was recorded. On day 17, 200 μL blood samples were collected from the orbit vein, lysed using ACK buffer, and centrifuged at 600g for 8 min to collect T cells. Then the collected cells were suspended in PBS (1% FBS) and stained with APC-CD8, FITC-CD3, and PE-CD4 antibodies for 1 h. The collected T cells were washed with PBS before being analyzed by flow cytometry. Meanwhile, 200 μL blood samples were placed in the centrifuge tube and centrifuged at 600g for 10 min. ELISA kits were applied to determine the serum levels of IFN-γ, IL-12, and TNF-α. After blood collection, the mice were sacrificed and the number of tumor foci in the lungs was subsequently recorded after fixation with Bouin's fixative solution for 10 days, and the lung tissue was then sliced for H&E, TUNEL, Ki67, and immunofluorescence staining analyses. A new batch of mice were used to observe the survival time of mice for each group with the same procedure for the model construction and same drug administration schedule. The fluorescence images of the mice that underwent different treatments were acquired on days 10, 15, and 20 through the IVIS imaging system.
2.10 Statistical analysis
All the research data were presented as the mean ± S.D. The statistical analysis was performed via two-tailed Student's t-test between two groups and ordinary one-way ANOVA for three or more groups, and the statistical significance was expressed as N.S. (No Significance, P > 0.05), *P < 0.05, **P < 0.01, ***P < 0.001, and ****P < 0.0001. The mice survival curves were analyzed via the log-rank (Mantel–Cox) test. All the statistical data were analyzed by GraphPad Prism Software.
3. Results and discussion
3.1 Preparation mechanism of UV-Cryo cells
To obtain the UV-Cryo cells, LLC cells were sequentially treated with UV irradiation and cryo-shocking with liquid nitrogen (Scheme 1A), and the UV exposure dose was screened with the expression of the ICD marker CRT as the standard. Compared with other physical and chemical strategies, UV irradiation is easy to conduct in both labs and factories with simple approaches to UV equipment and negligible concerns of environmental toxicity, which are useful considerations for adoption in large-scale production. As shown in Fig. S1,† after UV irradiation, the expression of CRT was significantly elevated when the exposure dose was higher than 1.8 mJ cm−2. However, the high exposure also resulted in a reduced yield of cells (Fig. S2 and S3†); thus, 1.8 mJ cm−2 was regarded as the most suitable exposure dose for this step. Further, in order to immediately deactivate the tumor cells, all the cells were first dispersed in cell suspension medium (80% DMEM and 20% FBS) and quickly immersed in liquid nitrogen.
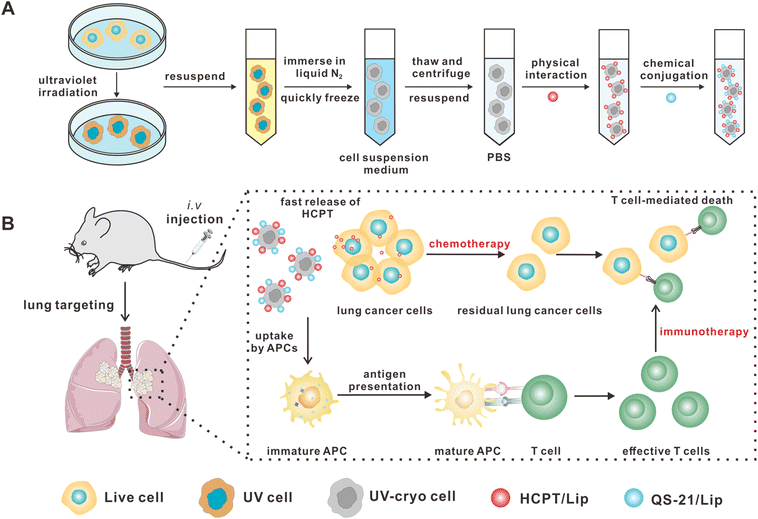 |
| Scheme 1 (A) A schematic illustration of the preparation of HCPT&QS-21/UV-Cryo cells. (B) Boosted antitumor efficacy elicited by HCPT&QS-21/UV-Cryo cells via the fast release of HCPT (chemotherapy) and long retention of QS-21 to achieve co-uptake of the antigen/adjuvant by APCs (immunotherapy). | |
CLSM and SEM were utilized to characterize the structure of the UV-Cryo cells. As shown in Fig. 1A and B, the UV-Cryo cells exhibited almost an intact cellular structure with a round shape with a cell nucleus and membrane. After UV irradiation and cryo-shocking, the cell contour was distinct and all the tumor cells were dead (Fig. 1C). Further, the UV-Cryo cells were characterized by Annexin V-FITC/PI staining, and 97.2% of cells were necrotic-dependent (Fig. 1D and E).
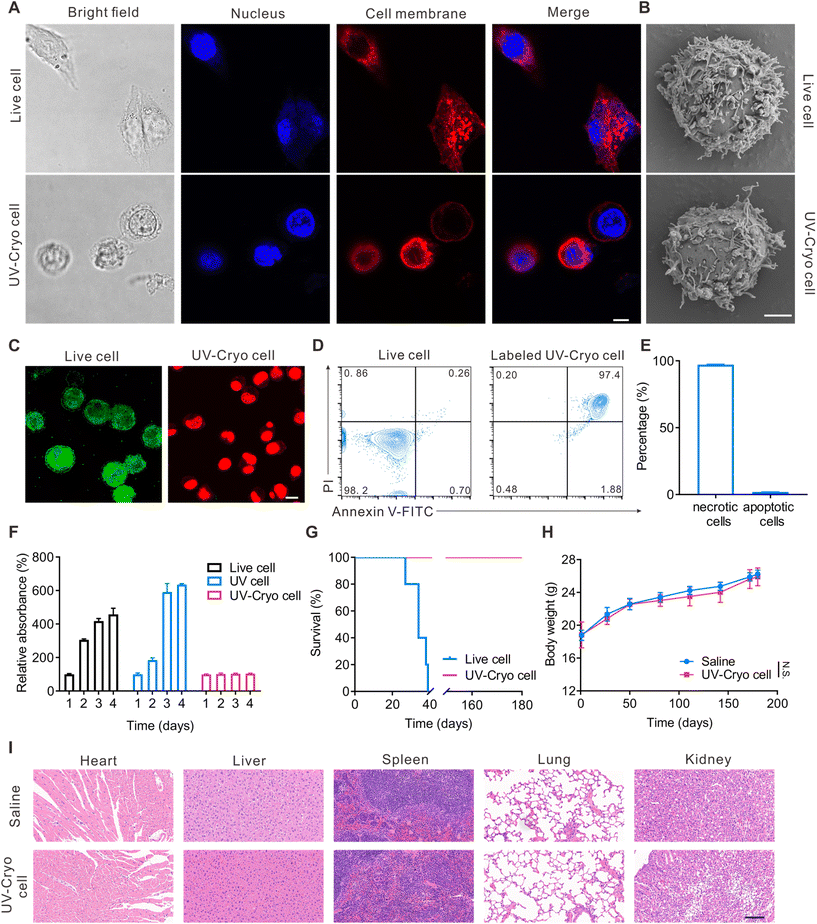 |
| Fig. 1 The characterization of the UV-Cryo cells. (A) The cellular structure of live LLC cells and UV-Cryo cells. The cell nucleus and cytoplasm F-actin were stained by Hoechst 33342 (blue) and rhodamine phalloidin (red), respectively. Scale bar, 10 μm. (B) SEM images of live LLC cells and UV-Cryo cells. Scale bar, 2 μm. (C) The live/dead analysis of live LLC cells and UV-Cryo cells by Calcein/PI cell viability kit staining. The cells were stained with Calcein (green) and PI (red) and imaged by CLSM. The cells with green signals were live cells, and the cells with red signals were dead cells. Scale bars, 10 μm. (D) Typical flow cytometry images of live LLC cells and UV-Cryo cells after Annexin V-FITC/PI staining. (E) The cell percentage of necrotic and apoptotic cells. Necrotic cells referred to Annexin V-FITC+PI+ cells, and apoptotic cells referred to Annexin V-FITC+PI− cells (n = 3). (F) The cell viability analysis of indicated groups by CCK-8 assay (n = 6). (G) The survival situation of the mice after injecting live tumor cells and UV-Cryo cells (n = 5). (H) Body weight of the mice in different groups (n = 5). (I) H&E staining of the heart, liver, spleen, lung, and kidney from the saline- and UV-Cryo cell-treated mice. Scale bar, 100 μm. | |
3.2 Biosafety evaluation of the UV-Cryo cells
In this work, we utilized UV irradiation to enhance the immunogenicity of tumor cells to improve the immune responses. The cell viability of the UV-Cryo cells was evaluated by CCK-8 assay. As shown in Fig. 1F, after UV irradiation alone, the proliferation capability of the remaining cells sharply increased, with the cell number evaluated as 319.7% on day 3 compared with on day 2 post-irradiation, while it was merely 136.6% in the group of untreated cells. In comparison, the cells completely lost their proliferation capability after both UV irradiation and cryo-shocking.
We next studied the biosafety of the UV-Cryo cells in vivo. As exhibited in Fig. 1G, live LLC cells caused the deaths of 100% of the mice within 40 days, while the mice receiving UV-Cryo cell treatment all survived within the observation period of 6 months. Meanwhile, the body weight of the mice undergoing UV-Cryo cell treatment kept rising, and there was no significant difference compared with the normal mice (Fig. 1H). After the 6-month post-injection of UV-Cryo cells, the typical organs were removed from the mice after sacrifice and stained with H&E. According to Fig. 1I, there was no obvious necrosis or inflammation in contrast to the tissues from the normal mice. The in vivo safety evaluation results demonstrated that all the mice treated with UV-Cryo cells exhibited no significant side effects, and no mice died during the whole observation period, supporting the high safety of the proposed UV irradiation/cryo-shocking strategy.
3.3 Differential loading of HCPT and QS-21 in the UV-Cryo cells
The drug dosing sequence in chemoimmunotherapy could influence the therapeutic efficacy.48,49 Generally, chemotherapy needs to be conducted at first to inhibit the proliferation of tumor cells, then further leveraging immunotherapy to elicit body immune responses to recognize and kill residue tumor cells.21,50 Hence in this work, we loaded the chemo-drug (HCPT) and immunological adjuvant (QS-21) differentially in the UV-Cryo cells. As indicated in Fig. S4,† both HCPT/Lip and QS-21/Lip exhibited sufficient storage stability for at least 15 days. In detail, HCPT was attached to the UV-Cryo cells by physical absorption on the basis of the electrostatic forces between the cationic HCPT/Lip (Table S1†) and UV-Cryo cells, while QS-21 was loaded through chemical linkage via the click reaction between the maleimide groups on QS-21/Lip and the thiol groups on the UV-Cryo cells. We adopted the fluorescence dyes of coumarin 6 and DiI as the model agents to characterize the loading of HPCT and QS-21 in the UV-Cryo cells, respectively. As is exhibited in Fig. 2A, obvious coumarin 6 (green) and DiI (red) fluorescence were observed in the UV-Cryo cells with the colocalization coefficient of 0.9316, indicating the successful co-loading of the two drugs in the UV-Cryo cells. Besides, SEM was applied to characterize the structure of the HCPT&QS-21-UV-Cryo cells (Fig. S5†). The drug-loaded UV-Cryo cells were sphere-like particles, similar to the form of UV-Cryo cells.
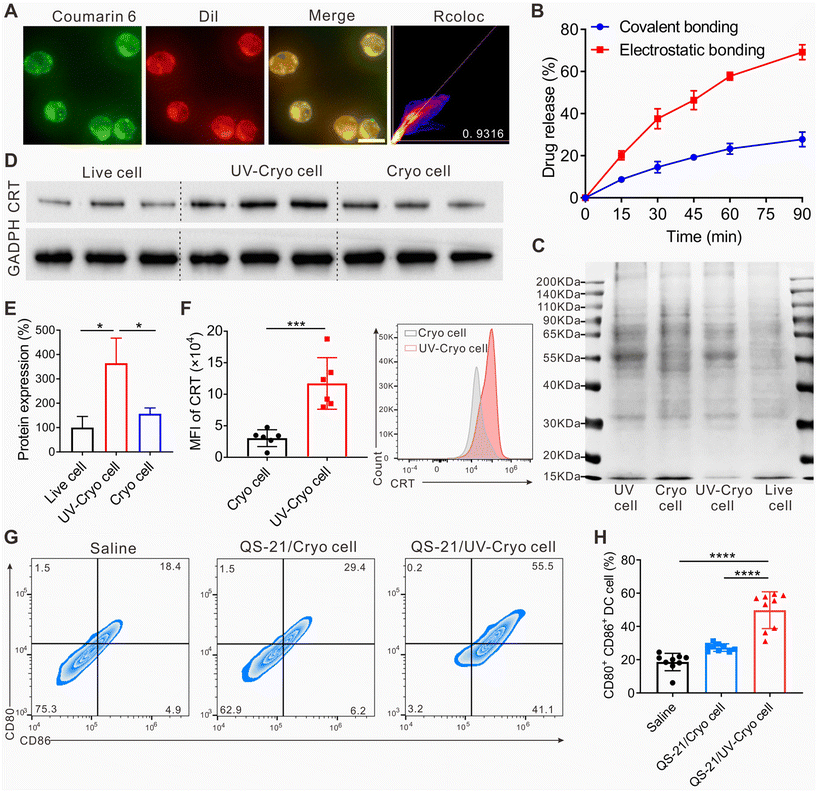 |
| Fig. 2 The characterization of drug-loaded UV-Cryo cells. (A) Fluorescence inverted microscopy images of coumarin 6 and DiI co-loaded UV-Cryo cells. Scale bar, 20 μm. (B) Drug-release determination from UV-Cryo cells (n = 3). (C) SDS-PAGE of whole cell lysate proteins obtained from different groups. (D) Western blotting images and (E) the quantification of CRT in different groups. (F) The CRT expression of Cryo cells and UV-Cryo cell groups 24 hours after the UV treatment analyzed by flow cytometry (n = 6). (G) Typical flow cytometry images of DC maturation under different treatments. (H) The percentage of CD80+/CD86+ DCs (gated on CD11c+ cells) after stimulation in various groups (n = 9). | |
Drug release from the UV-Cryo cells was analyzed with a peristaltic pump to mimic the blood flow in vitro. Under shearing stress of 6 Pa (in accordance with the shear stress of lung capillaries), up to 57.8% HCPT could be quickly released within 1 h, while much less QS-21 was released, with over 72.3% of QS-21 still maintained in the UV-Cryo cells (Fig. 2B). This differential release enabled both the high accumulation of HCPT in the lungs to maximize the chemotherapeutic efficacy, and the long-term retention of QS-21 in the UV-Cryo cells to function as a cancer vaccine after chemotherapy.
3.4 Protein expressions of the UV-Cryo cells and in vitro DC activation
SDS-PAGE was employed to evaluate the protein expressions of UV-Cryo cells at first. As demonstrated in Fig. 2C, the protein expressions of the cells would not be influenced by cryo-shocking, which reflected the similar protein bands between the live cells and Cryo cells, while the protein expressions of the cells were significantly changed after UV irradiation. In detail, the expressed calreticulin in UV-Cryo cells increased significantly (Fig. 2D, E, and F), which was 3.6 times more than that of normal LLC cells.
DCs are the most common and typical APCs in the immune system,51–54 and the antigen presentation and maturation of APCs is important in the activation of immune responses.55 Thus, we analyzed the expression of biomarkers expressed on DCs after QS-21/Cryo cell and QS-21/UV-Cryo cell stimulation. According to Fig. 2G and H, the proportions of mature DCs (CD80+CD86+ DCs) were elevated if incubated with QS-21/Cryo cells or QS-21/UV-Cryo cells. The percentage of CD80+CD86+ DCs were 18.6% (saline group), 27.3% (QS-21/Cryo cell group), and 49.8% (QS-21/UV-Cryo cell group), respectively, indicating that QS-21/UV-Cryo cells could stimulate DC maturation more efficiently compared to QS-21/Cryo cells.
3.5
In vivo biodistribution of UV-Cryo cells
Besides serving as a tumor vaccine, UV-Cryo cells also functioned as lung-targeting vehicle due to their unique cellular size (≥7 μm). The distribution of UV-Cryo cells was evaluated by IVIS after labeling the cells with the fluorescence probe Cy5.5. As depicted in Fig. 3, free Cy5.5 was mainly distributed in the liver after intravenous administration, while Cy5.5/UV-Cryo cells more tended to distribute in the lungs within 1 h post-injection. In the lungs, the fluorescence signal of the Cy5.5/UV-Cryo cell group was substantially higher than that of free dye. It could be inferred from the above phenomenon that the chemotherapy drug HPCT could be precisely transported to the lungs, and fast release was then achieved due to the passive lung-targeting capability of the UV-Cryo cells and the high shearing stress of the lung capillaries, which eventually promoted the drug accumulation at the tumor site in the lung.
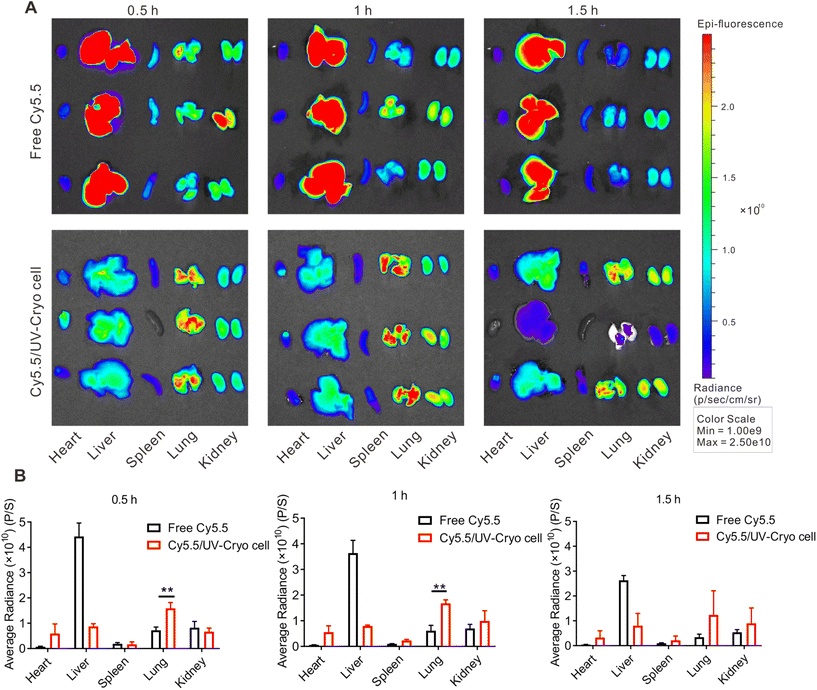 |
| Fig. 3
In vivo distribution of UV-Cryo cells. (A) IVIS images of mice after i.v. injections of Cy5.5 and Cy5.5/UV-Cryo cells. (B) The statistical analysis of isolated organs at 0.5, 1, and 1.5 h post-injection (n = 3). | |
3.6
In vivo antitumor efficacy of HCPT&QS-21/UV-Cryo cells
The antitumor efficacy of HCPT&QS-21/UV-Cryo cells was evaluated in lung tumor-bearing mice based on the therapy scheme illustrated in Fig. 4A. As shown in Fig. 4B, the mice treated in the HCPT&QS-21/UV-Cryo cell group kept alive until day 60, while all the mice died at days 36, 42, 42, and 41 in the saline, HCPT, UV-Cryo cell, and QS-21/UV-Cryo cell groups, respectively. According to Fig. 4C, during the whole therapy process, there was no significant change in body weight of the mice in each group, indicating the excellent safety of each agent. With the development of tumors, the weight of the mice in the other groups showed an obvious decrease, but the weight loss rate of the mice in the HCPT&QS-21/UV-Cryo cell group was much less. The bioluminescence signal (related to LLC-luc cells) around the lungs of the mice in the HCPT&QS-21/UV-Cryo cell group was obviously lower than that for the other groups (Fig. 4D). In addition, the number of tumor nodules of the lung tissue decreased after treatment with the HCPT&QS-21/UV-Cryo cells (Fig. 4E and F); and there was no obvious tumor foci in the lungs in the HCPT&QS-21/UV-Cryo cell group as illustrated in the H&E staining assay (Fig. 4G).
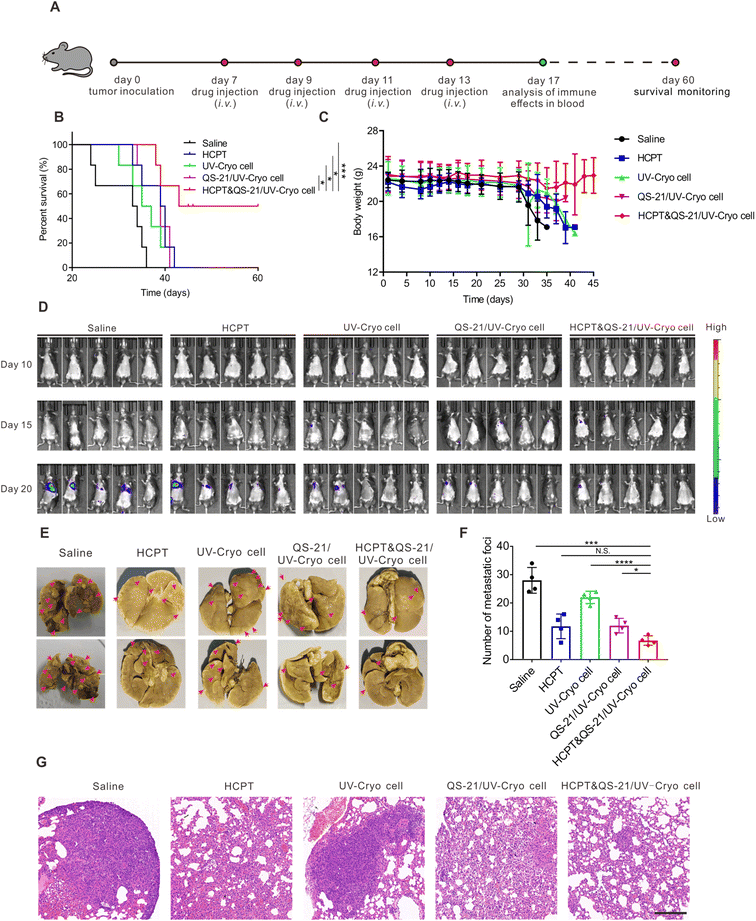 |
| Fig. 4 The in vivo antitumor efficiency determination of HCPT&QS-21/UV-Cryo cells and other treatment groups. (A) A schematic illustration of the model construction and therapy timeline. (B) The survival percentage and (C) body weight of the mice in the various treatment groups (n = 6). (D) Tumor progression of LLC-luc cells-bearing mice of the indicated group detected by IVIS (n = 5). (E) Representative lung photographs after staining with Bouin's fixative solution. Red arrows mark the tumor foci. (F) The number of foci of the indicated group (n = 4). (G) H&E staining of the lung tissue from various groups. Scale bar, 100 μm. | |
Meanwhile, after the therapy based on HCPT&QS-21/UV-Cryo cells, the percentages of CD3+ T cells and CD8+ T cells in peripheral blood were substantially increased to 40.9% and 23.6%, which were significantly higher than in all the other groups (Fig. 5A–D). Moreover, the serum cytokine levels of IFN-γ, IL-6, and TNF-α of the mice were significantly elevated after treatment by the HCPT&QS-21/UV-Cryo cells compared with the other groups (Fig. 5E–G). In addition, immunofluorescence staining revealed obvious signals of CD4+ and CD8+ T cells in the lung tissue of the mice in the HCPT&QS-21/UV-Cryo cell group (Fig. 5H). TUNEL staining assay also proved obvious cell apoptosis in the HCPT&QS-21/UV-Cryo cell group, and the Ki67 staining assay relevant to this group revealed less tumor cell proliferation than the others (Fig. S6†). These above results certified that the chemoimmunotherapy mediated by HCPT&QS-21/UV-Cryo cells could boost the immune responses and achieve efficient in vivo antitumor efficacy.
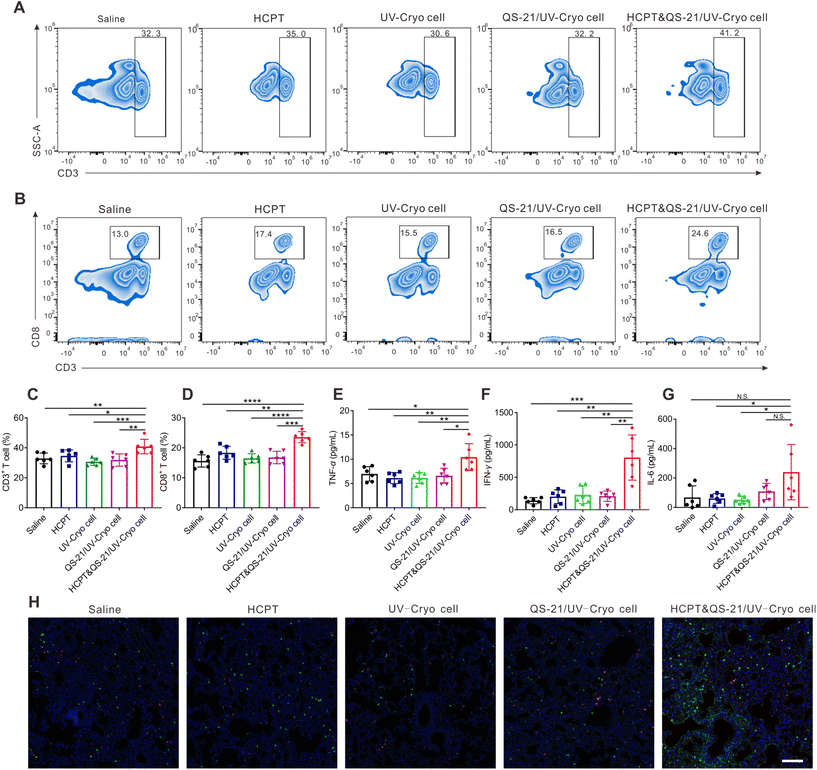 |
| Fig. 5 The in vivo immune-activation analysis of HCPT&QS-21/UV-Cryo cells and other treatment groups. Typical flow cytometry images of the proportion of (A) CD3+ T cells and (B) CD3+/CD8+ T cells in peripheral blood lymphocyte in different groups on day 17. Statistical analysis of (C) CD3+ T cells and (D) CD3+/CD8+ T cells in peripheral blood lymphocytes on day 17 (n = 6). Serum levels of (E) TNF-α, (F) IFN-γ, and (G) IL-6 in various groups on day 17 (n = 6). (H) Immunofluorescence staining of lungs. CD4+ T cells were labeled with Cy5 (red), CD8+ T cells were labeled with Cy3 (green), and cell nuclei were labeled with DAPI (blue). Scale bar, 100 μm. | |
4. Conclusion
In this study, an immunogenic dead cell model of UV-Cryo cells was engineered by the sequential treatment of ultraviolet irradiation and cryo-shocking of tumor cells. Both the in vitro and in vivo results indicated that the UV-Cryo cells possessed outstanding lung-targeting and immune-activation properties, and could serve as a lung-targeting vehicle and cancer vaccine. HCPT&QS-21/UV-Cryo cells could be obtained after the differential loading of HCPT and QS-21 into the UV-Cryo cells. In a lung-tumor-bearing mice model, HCPT&QS-21/UV-Cryo cells mediated synergistic chemoimmunotherapy and effectively prolonged mice survival. In a nutshell, these findings demonstrated that HCPT&QS-21/UV-Cryo cells have promising application prospect in the treatment of lung cancer.
Author contributions
Jing Zang: conceptualization, methodology, experimental work, analyzing and interpreting data, manuscript writing. Jinniu Zhang: methodology, experimental work, data curation. Yijun Mei: data collection, methodology. Yaoxuan Xiong: methodology, experimental work. Tianyuan Ci: conceptualization, supervision, funding acquisition, manuscript review. Nianping Feng: supervision, funding acquisition, manuscript review.
Conflicts of interest
There are no conflicts of interest to declare.
Acknowledgements
This work was supported by National Natural Science Foundation of China (No. 82173984), Shanghai Rising-Star Program (No. 21QA1408800), Program for Shanghai High-Level Local University Innovation Team (SZY20220315).
References
- R. L. Siegel, K. D. Miller, H. E. Fuchs and A. Jemal, CA Cancer J. Clin., 2022, 72, 7–33 CrossRef PubMed.
- B. C. Bade and C. S. Dela Cruz, Clin. Chest Med., 2020, 41, 1–24 CrossRef.
- F. Li, H. Mei, Y. Gao, X. Xie, H. Nie, T. Li, H. Zhang and L. Jia, Biomaterials, 2017, 145, 56–71 CrossRef CAS.
- W. Chen, D. Yu, S.-Y. Sun and F. Li, Acta Biomater., 2021, 129, 258–268 CrossRef CAS.
- X. R. Yin, Y. N. Cui, R. S. Kim, W. R. Stiles, S. H. Park, H. R. Wang, L. Ma, L. Chen, Y. Baek, S. Kashiwagi, K. Bao, A. Ulumben, T. Fukuda, H. Kang and H. S. Choi, Theranostics, 2022, 12, 4147–4162 CrossRef CAS.
- Y. H. Zhu, C. Y. Sun, S. Shen, M. I. U. Khan, Y. Y. Zhao, Y. Liu, Y. C. Wang and J. Wang, Biomater. Sci., 2017, 5, 1612–1621 RSC.
- A. A. Thai, B. J. Solomon, L. V. Sequist, J. F. Gainor and R. S. Heist, Lancet, 2021, 398, 535–554 CrossRef.
- W. H. Lee, C. Y. Loo, D. Traini and P. M. Young, Asian J. Pharm. Sci., 2015, 10, 481–489 CrossRef.
- G. S. Jones and D. R. Baldwin, Clin. Med., 2018, 18, S41–S46 CrossRef PubMed.
- Q.-Y. Hong, G.-M. Wu, G.-S. Qian, C.-P. Hu, J.-Y. Zhou, L.-A. Chen, W.-M. Li, S.-Y. Li, K. Wang, Q. Wang, X.-J. Zhang, J. Li, X. Gong, C.-X. Bai, Chinese Thoracic Soc and Chinese Alliance Against Lung Canc Lung, Cancer, 2015, 121, 3080–3088 CrossRef.
- R. L. Siegel, K. D. Miller and A. Jemal, CA-Cancer J. Clin., 2019, 69, 7–34 CrossRef.
- C. M. Baehr, L. Zhang, Y. Wu, A. Domokos, W. W. Xiao, L. Wang and K. S. Lam, Biomaterials, 2021, 277, 121078 CrossRef CAS PubMed.
- A. El-Hussein, S. L. Manoto, S. Ombinda-Lemboumba, Z. A. Alrowaili and P. Mthunzi-Kufa, Anti-Cancer Agents Med. Chem., 2021, 21, 149–161 CrossRef CAS PubMed.
- H. Y. Lu, Y. J. Chang, N. C. Fan, L. S. Wang, N. C. Lai, C. M. Yang, L. C. Wu and J. A. A. Ho, Biomaterials, 2015, 42, 30–41 CrossRef CAS PubMed.
- A. H. Krist, K. W. Davidson, C. M. Mangione, M. J. Barry, M. Cabana, A. B. Caughey, E. M. Davis, K. E. Donahue, C. A. Doubeni, M. Kubik, C. S. Landefeld, L. Li, G. Ogedegbe, D. K. Owens, L. Pbert, M. Silverstein, J. Stevermer, C. W. Tseng, J. B. Wong and US Preventive Serv Task Force, JAMA, J. Am. Med. Assoc., 2021, 325, 962–970 CrossRef.
- H. Wang, Y. Bo, Y. Liu, M. Xu, K. M. Cai, R. B. Wang and J. J. Cheng, Biomaterials, 2019, 218, 119305 CrossRef CAS PubMed.
- C. G. Da Silva, F. Rueda, C. W. Lowik, F. Ossendorp and L. J. Cruz, Biomaterials, 2016, 83, 308–320 CrossRef CAS PubMed.
- S. Pan, T. Li, Y. Tan and H. Xu, Biomaterials, 2022, 280, 121321 CrossRef CAS PubMed.
- F. Shen, L. Feng, Y. Zhu, D. Tao, J. Xu, R. Peng and Z. Liu, Biomaterials, 2020, 255, 120190 CrossRef CAS PubMed.
- Y. Mei, L. Tang, L. Zhang, J. Hu, Z. Zhang, S. He, J. Zang and W. Wang, Mater. Today, 2022, 60, 52–68 CrossRef CAS.
- C. Q. Ma, Y. C. Duan, C. H. Wu, E. J. Meng, P. P. Li, Z. Z. Zhang, C. H. Zang and X. L. Ren, Asian J. Pharm. Sci., 2021, 16, 653–664 CrossRef.
- J. Gao, H. W. Zhang, F. Q. Zhou, B. Hou, M. W. Chen, Z. G. Xie and H. J. Yu, Chin. Chem. Lett., 2021, 32, 1929–1936 CrossRef CAS.
- J. H. Li, L. J. Huang, H. L. Zhou, Y. M. Shan, F. M. Chen, V. P. Lehto, W. J. Xu, L. Q. Luo and H. J. Yu, Acta Pharmacol. Sin., 2022, 43, 2749–2758 CrossRef CAS.
- M. Reck, J. Remon and M. D. Hellmann, J. Clin. Oncol., 2022, 40, 373 Search PubMed.
- M. Saxena, S. H. van der Burg, C. J. M. Melief and N. Bhardwaj, Nat. Rev. Cancer, 2021, 21, 360–378 CrossRef CAS PubMed.
- G. N. Shi, C. N. Zhang, R. Xu, J. F. Niu, H. J. Song, X. Y. Zhang, W. W. Wang, Y. M. Wang, C. Li, X. Q. Wei and D. L. Kong, Biomaterials, 2017, 113, 191–202 CrossRef CAS.
- Y. Sui, J. Li, J. Q. Qu, T. Fang, H. Y. Zhang, J. Zhang, Z. R. Wang, M. Y. Xia, Y. H. Dai and D. K. Wang, Asian J. Pharm. Sci., 2022, 17, 583–595 CrossRef PubMed.
- C. S. K. Leung and B. J. Van den Eynde, Cancer Cell, 2022, 40, 903–905 CrossRef CAS.
- Y. Nakahara, T. Kouro, Y. Igarashi, M. Kawahara and T. Sasada, Expert Rev. Vaccines, 2019, 18, 703–709 CrossRef CAS PubMed.
- E. Rijavec, F. Biello, C. Genova, G. Barletta, C. Maggioni, M. G. Dal Bello, S. Coco, A. Truini, I. Vanni, A. Alama, S. Beltramini, M. A. Grassi, F. Boccardo and F. Grossi, Expert Opin. Biol. Ther., 2015, 15, 1371–1379 CrossRef CAS.
- M. Peng, Y. Mo, Y. Wang, P. Wu, Y. Zhang, F. Xiong, C. Guo, X. Wu, Y. Li, X. Li, G. Li, W. Xiong and Z. Zeng, Mol. Cancer, 2019, 18, 128 CrossRef.
- M. Obeid, T. Panaretakis, N. Joza, R. Tufi, A. Tesniere, P. van Endert, L. Zitvogel and G. Kroemer, Cell Death Differ., 2007, 14, 1848–1850 CrossRef CAS PubMed.
- T. Panaretakis, N. Joza, N. Modjtahedi, A. Tesniere, I. Vitale, M. Durchschlag, M. Durchschlag, G. M. Fimia, O. Kepp, M. Piacentini, K. U. Froehlich, P. van Endert, L. Zitvogel, F. Madeo and G. Kroemer, Cell Death Differ., 2008, 15, 1499–1509 CrossRef CAS.
- T. Panaretakis, O. Kepp, U. Brockmeier, A. Tesniere, A.-C. Bjorklund, D. C. Chapman, M. Durchschlag, N. Joza, G. Pierron, P. van Endert, J. Yuan, L. Zitvogel, F. Madeo, D. B. Williams and G. Kroemer, EMBO J., 2009, 28, 578–590 CrossRef CAS PubMed.
- D. Brusa, S. Garetto, G. Chiorino, M. Scatolini, E. Migliore, G. Camussi and L. Matera, Vaccine, 2008, 26, 6422–6432 CrossRef CAS.
- J. Zhang, X. Sun, X. Zhao, C. Yang, M. Shi, B. Zhang, H. Hu, M. Qiao, D. Chen and X. Zhao, Acta Pharm. Sin. B, 2022, 12, 3694–3709 CrossRef CAS.
- E. B. Golden and L. Apetoh, Semin. Radiat. Oncol., 2015, 25, 11–17 CrossRef PubMed.
- Y. Moon, M. K. Shim, J. Choi, S. Yang, J. Kim, W. S. Yun, H. Cho, J. Y. Park, Y. Kim, J. K. Seong and K. Kim, Theranostics, 2022, 12, 1999–2014 CrossRef CAS PubMed.
- Z. Zhao, A. Ukidve, V. Krishnan, A. Fehnel, D. C. Pan, Y. Gao, J. Kim, M. A. Evans, A. Mandal, J. Guo, V. R. Muzykantov and S. Mitragotri, Nat. Biomed. Eng., 2021, 5, 441–454 CrossRef CAS.
- Z. Zhao, L. Fang, P. Xiao, X. Sun, L. Zhou, X. Liu, J. Wang, G. Wang, H. Cao, P. Zhang, Y. Jiang, D. Wang and Y. Li, Adv. Mater., 2022, 34, 2205462 CrossRef CAS.
- X. Liu, J. Xu, T. Yao, J. Ding, S. Li, R. Su, H. Zhang, H. Li, Q. Yue and X. Gao, ACS Appl. Mater. Interfaces, 2023, 15, 67–76 CrossRef CAS.
- Z. Q. Sun, J. T. Chen, G. C. Chen, C. Zhang and C. Y. Li, Biomed. Mater., 2022, 17, 022002 CrossRef.
- X. Y. Zhao, J. T. Zhang, B. B. Chen, X. K. Ding, N. N. Zhao and F. J. Xu, Small Methods, 2023, 7, 2201595 CrossRef CAS PubMed.
- M. Saeed, F. M. Chen, J. Y. Ye, Y. Shi, T. Lammers, B. G. De Geest, Z. P. Xu and H. J. Yu, Adv. Mater., 2021, 33, 2008094 CrossRef CAS.
- O. Jobe, J. Kim, D. O. Pinto, Z. Villar, T. Hewitt, E. H. Duncan, A. Anderson, N. Gohain, H. Gong, C. Tucker, C. R. Alving, G. R. Matyas, E. Bergmann-Leitner and M. Rao, Sci. Rep., 2022, 12, 7570 CrossRef CAS.
- K. AboulFotouh, H. Xu, C. Moon, R. O. Williams, III and Z. Cui, Int. J. Pharm., 2022, 622, 121825 CrossRef CAS PubMed.
- Y. Xin, X. Chen, X. Tang, K. Li, M. Yang, W. C.-S. Tai, Y. Liu and Y. Tan, Biophys. J., 2019, 116, 1803–1814 CrossRef CAS PubMed.
- T. A. Yap, E. E. Parkes, W. Peng, J. T. Moyers, M. A. Curran and H. A. Tawbi, Cancer Discovery, 2021, 11, 1368–1397 CrossRef CAS.
- K. M. Heinhuis, W. Ros, M. Kok, N. Steeghs, J. H. Beijnen and J. H. M. Schellens, Ann. Oncol., 2019, 30, 219–235 CrossRef CAS.
- C. Zhu, Y. Shi, Q. Li, L. Luo, X. Li, Z. Luo, Y. Lu, J. Zhang, M. Jiang, B. Qin, W. Qiu, Z. Jin, X. Jiang, J. Xiao and J. You, J. Controlled Release, 2022, 341, 769–781 CrossRef CAS PubMed.
- M. Collin, N. McGovern and M. Haniffa, Immunology, 2013, 140, 22–30 CrossRef CAS PubMed.
- X. D. Fan, T. X. Yue, A. D. Liu, X. W. Xie, W. X. Fang, Y. H. Wei, H. S. Zheng, H. Y. Zheng, M. Q. Zhou, J. G. Piao and F. Z. Li, Asian J. Pharm. Sci., 2022, 17, 713–727 CrossRef PubMed.
- S. S. Gou, S. Wang, W. W. Liu, G. Y. Chen, D. Y. Zhang, J. F. Du, Z. Y. Yan, H. F. Wang, W. J. Zhai, X. H. Sui, Y. H. Wu, Y. M. Qi and Y. F. Gao, Theranostics, 2021, 11, 7308–7321 CrossRef CAS PubMed.
- K. Schuhladen, L. Stich, J. Schmidt, A. Steinkasserer, A. R. Boccaccini and E. Zinser, Biomater. Sci., 2020, 8, 2143–2155 RSC.
- Z. Yu, M. Wu, Y. Huang, Y. Wang, Y. Chen, Q. Long, Z. Lin, L. Xue, C. Ju and C. Zhang, Biomater. Sci., 2022, 11, 263–277 RSC.
|
This journal is © The Royal Society of Chemistry 2024 |
Click here to see how this site uses Cookies. View our privacy policy here.