Anisotropic hydrogels with high-sensitivity and self-adhesion for wearable sensors†
Received
14th September 2022
, Accepted 21st November 2022
First published on 22nd November 2022
Abstract
Conductive hydrogels (CHs) are widely applied in soft electronic devices. However, the existing CHs excluded self-adhesion and high sensitivity at lower strains, hardly fulfilling the increasing requirements for the next-generation sensors. Inspired by the structure of muscle, an anisotropic hydrogel was designed to address this issue. The gel was prepared from acrylamide (AM), carbon nanotubes (CNTs), and tannic acid (TA), serving as the monomer, conductive filler, and tackifier, respectively. Next, a combination of pre-stretching, air-drying in a confining geometry, and immersion in an aqueous TA solution was adopted to induce the orientation structure. The mechanical strength of the hydrogel along the parallel stretching direction (75.2 kPa) was higher than that along the orthogonal stretching direction (22.2 kPa). Furthermore, the hydrogel showed anisotropic conductivity on account of regular conductive channels. The gauge factor (GF) parallel to the stretching direction was higher than that orthogonal to the stretching direction. The presence of CNTs and ordered structures endowed fast detection (response time of 200 ms) and high sensitivity (GF was 62.2 above 120% strain). In addition, the free catechol groups from TA empowered excellent self-adhesion (adhesion strength = 77.2 kPa). This work provided the basis for the manufacture of high-performance sensors, holding broad potential in flexible sensors, electronic skins, and soft robots.
1. Introduction
Combining the advantages of conductive materials and hydrogels, conductive hydrogels (CHs) have broadened their applications in artificial skin, soft robotics, and wearable sensors, wherein excellent conductive performances and self-adhesion properties are vital for their applications.1–20 Thus far, CHs have been prepared mainly by embedding conductive additives (electrolytes, conducting polymers, metals, and carbon nanomaterials) in polymeric matrixes.21–53 Theoretical studies and extensive experiments have shown that such a design always results in isotropic conductive channels. Given that the strain generated by human activity is usually less than 75%, improving the sensitivity at low strains is very important.10 In addition, conventional CHs lack self-adhesion ability due to the self-lubricating effect of interfacial water molecules, further hindering the detection of subtle physiological changes. Consequently, fabricating CHs simultaneously possessing self-adhesion and high sensitivity at lower strains is essential for their applications.
Although self-adhesive hydrogels and highly sensitive sensors were prepared, this combination has seldom been expanded to conductive hydrogels, which greatly hindered their applications. For example, Zhang41et al. designed a high-sensitive (GF of 52.7 within 300% strain) sensor from polyvinyl alcohol, carbon nanotubes, and graphene. However, it needed tapes and straps to adhere to the human body. To impart adhesion capability, the Fu38 group reported self-adhesive ionic hydrogels that comprised dynamically crosslinked (poly[2-(methacryloyloxy)ethyl]dimethyl-(3-sulfopropyl), PSBMA) and polyvinyl alcohol. The presence of PSBMA endowed adhesion strength of 7.66 kPa. However, its GF within 300% strain was only 1.5, probably because the lack of regular conductive channels inside the hydrogel hindered ion transport to some extent. Therefore, manufacturing CHs with both high sensitivity at low strains and self-adhesive properties remains challenging.
Soft tissues of living organisms, such as muscles and skin, possess well-ordered structures and anisotropic channels to realize signal detection, transmission, and contraction capabilities.54–56 Inspired by the structure of muscle, an anisotropic hydrogel was designed. It was prepared via free radical polymerization of acrylamide monomer in the CNTs-dispersed aqueous solution (Fig. 1), followed by sequentially pre-stretching, air-drying, and soaking in a tannin aqueous solution. Numerous CNTs were entangled and folded in complex networks inside the polyacrylamide (PAM) matrix to form the internal conductive pathway. Pre-stretching drove the PAM chains and CNTs regularly aligned along the direction of the external force while air-drying in a confining geometry and soaking in an aqueous TA solution effectively fixed this orientation structure,57,58 thereby, forming regular electron channels. By doing so, the distance between CNTs was reduced because of the highly ordered arrangement of the conductive hydrogel backbone, resulting in higher conductivity.56 Moreover, it is easier for electrons to transport along conductive channels.55 Therefore, the presence of CNTs and ordered structures endowed high sensitivity (GF was 62.2 above 120% strain). Moreover, the free catechol groups of the TA molecule imparted self-adhesion properties (adhesion strength = 77.2 kPa). These features empower broad applications in the human–machine interface and medical monitoring.
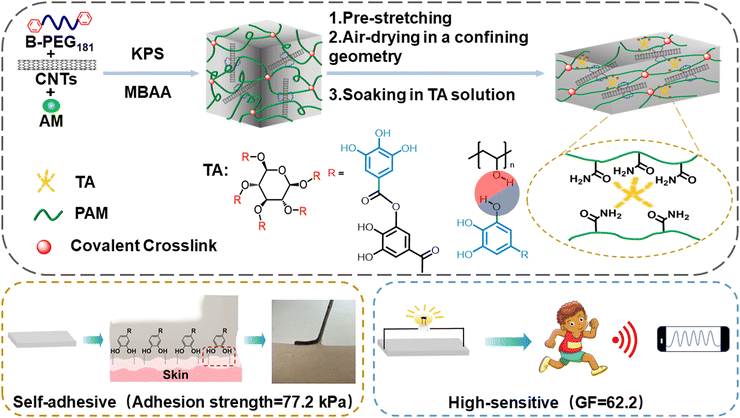 |
| Fig. 1 Schematic diagram of the anisotropic conductive hydrogel. | |
2. Materials and methods
2.1 Materials
Polyethylene glycol (PEG181, the average degree of polymerization was 181), acrylamide (AM), potassium persulfate (KPS), N,N′-methylene bisacrylamide (MBAA), carbon nanotubes (CNTs), and tannic acid (TA, 99%) were provided by Shanghai Aladdin Reagent Co. Ltd.
2.2 Preparation of hydrogels
Bis benzyl-modified polyethylene glycol (B-PEG181) was synthesized following procedures from literatures.34,59,60 Subsequently, B-PEG181 (105 mg, 0.0128 mmol), AM (3.5 g, 49.2 mmol), MBAA (1 mg, 6.5 × 10−3 mmol), and CNTs (mass ratios of CNTs to AM were 0.4%, 0.8%, 1.6%, and 2.4%) were added to 8.16 mL of deionized water. After deoxygenating with N2 and the addition of KPS (35 mg, 0.129 mmol), the solution was transferred to a glass mould and polymerized to prepare the precursor hydrogel. Next, aerogels were obtained by pre-stretching the precursor hydrogel and air drying in a mold at various pre-stretch ratios (strain = 0, 25%, 50%, and 75%) according to previous reports. Finally, the aerogels were immersed in TA solution (mass ratios of TA to water were 0, 5 wt%, and 10 wt%) for several time intervals (10, 20, and 30 h) to obtain the target hydrogel. After optimization, the hydrogel with the best performance was denoted as PACT, where PA, C, and T represent PAM, CNTs, and TA, respectively. The samples parallel and orthogonal to the stretching direction were donated as PACT-P and PACT-O, respectively. The control sample, single network polyacrylamide hydrogel (s-PAM), was also prepared merely from AM and MBAA for comparison.
2.3 Characterization
FT-IR spectra were collected using the Nicolet Avatar 380 instrument.61 Samples were lyophilized and sprayed with gold before taking SEM images (ZEISS Sigma microscope).62 X-Ray diffraction (XRD, Rigaku Smart Lab) data were obtained using Cu Kα radiation with a scan rate of 5° min−1 from 5 to 60° (2θ).63 The chemical compositions of hydrogels were measured using an X-ray photoelectron spectrometer (Thermo Scientific Escalab-250Xi). A monochromatic Al Kα X-ray was used as an excitation source.64 TEM images were obtained using a Tecnai-G2-F30 microscope.60 DLS data were obtained using the Anton Paar LitesizerZM 500 instrument.
2.4 Mechanical properties
The mechanical performances of the hydrogels were characterized using a universal testing machine (HZ-1003B, China) at room temperature. For tensile tests, dumbbell-shaped gels were evaluated with a force of 100 N at a constant speed of 100 mm min−1. The tensile stress (σb) and strain (ε) were calculated from the breaking point.65
2.5 Adhesion properties
At room temperature, the hydrogel was adhered to various substrates with specific properties and the self-adhesion properties of the hydrogel were observed. The lap shear test was performed to measure the adhesive strength of the hydrogels on various substrates with the contacting area of 20 mm × 20 mm at a rate of 100 mm min−1 until full separation.25,26
2.6 Electrical properties
The electrochemical workstation Autolab (CorrTest) was adopted to record the electrical properties of hydrogels with dimensions around 10.0 × 5.0 × 2.0 mm3. To monitor human movements, the hydrogel was placed on the human body, including fingers, face, wrists, elbows, etc., with a frequency from 105 to 10−2 Hz. The relative change of the resistance was calculated using Ohm's law (R = U/I), according to the formula (1): | 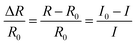 | (1) |
where I0 and I are the initial and real-time currents, respectively.
The strain sensitivity of the hydrogel was evaluated by the gauge factor (GF), which was calculated from the formula (2):
|  | (2) |
where
R0,
R, and
ε are the initial resistance, real-time resistance, and the strain applied during the test, respectively.
Electrochemical impedance spectroscopy was conducted in the frequency range of 10−1–106 Hz to obtain the conductivity (S) using the formula (3):
|  | (3) |
where
S,
L,
R, and
A are the conductivity, thickness of the hydrogel, the value of the impedance obtained, and the cross-sectional area of the hydrogel, respectively.
3. Results and discussion
3.1 Synthesis and characterization of the hydrogel
Conductive hydrogels are usually formed by the homogeneous polymerization of hydrophilic monomers in water with the assistance of conductive fillers, which usually have isotropic structures and functions.1,2 Since the sensitivity (gauge factor) is defined as the relative change in resistance divided by the tensile strain, the sensitivity of traditional conductive hydrogels at lower strains (less than 100%) is usually around 1.0.1,2 To solve this problem, we introduced anisotropic microstructures into traditional PAM hydrogels by the force induced orientation. Carbon nanotubes (CNTs) are prone to aggregate in water due to their intrinsic hydrophobicity,66 which greatly limits their performances. To address this issue, bis benzyl-modified polyethylene glycol (B-PEG181) was prepared and adopted to disperse CNTs in water (Fig. S1, ESI†).60 It is worth noting that the valid orientation of a polymer is a balance between polymer orientation and relaxation.67 The PAM chains are prone to move under external force and realize regular structures. However, they will spontaneously return to their original states after the removal of external forces. To this end, we used a three-step method to tackle this problem. The gel was first prepared from acrylamide, carbon nanotubes (CNTs), and tannic acid (TA), serving as the monomer, conductive filler, and tackifier, respectively. Next, a combination of pre-stretching, air-drying in a confining geometry, and immersion in an aqueous TA solution was adopted to induce the orientation structure. Pre-stretching drove the PAM chains and CNTs regularly aligned along the direction of the external force, while air-drying in a confining geometry and immersion in an aqueous TA solution effectively fixed this orientation structure. Additionally, the free catechol groups from the TA molecule might impart self-adhesion properties.68,69 By doing so, we expect to integrate the self-adhesion and high sensitivity into one system.
Its composition was confirmed from FT-IR spectra, SEM images, XPS, and XRD analyses. As shown in Fig. 2a, the FT-IR spectrum of PACT was similar to that of s-PAM,70 due to its highest composition in the gel matrix (Fig. S2, ESI†). However, the stretching vibration of N–H from s-PAM at 3424 cm−1 and that at 3407 cm−1 for the O–H from TA declined to 3397 cm−1 for the resultant PACT gel, indicating the formation of hydrogen bonds.71,72 This was further confirmed by XPS (Fig. S3, ESI† and Fig. 2b) Clearly, the N1s peaks at 400.1 eV for s-PAM declined by 0.6 eV for the resultant PACT gel, suggesting the formation of hydrogen bonds between PAM and TA.73,74 Due to the low content of CNTs (Fig. S2, ESI†), the sharp diffraction peak of CNTs at 26.0° disappeared in the PACT hydrogel (Fig. 2c). These revealed that TA and CNTs were integrated within the gel matrix. To visually display the anisotropic microstructure of the PACT hydrogel, the cross-sectional morphologies of the s-PAM hydrogel and the PACT hydrogel were observed by scanning electron microscopy (SEM). Since the water content in the PACT gel was 79.9% (Fig. S2, ESI†), s-PAM and PACT gels both displayed porous structures (Fig. S4, ESI†). However, the PACT hydrogel displayed distinct anisotropic microstructures along the stretching direction (Fig. 2d), well consistent with previous reports.16,51,65 Since the mass fraction of CNTs in the PACT hydrogel was 0.12% (Fig. S2, ESI†) and the CNTs were coated by B-PEG181 (Fig. S1, ESI†), it was difficult to distinguish them from PAM chains. Therefore, CNTs were not easily observed in the cross-section SEM images in such gels.32,50,75 These observations indicated that the pre-stretching, air-drying in a confining geometry, and immersion in an aqueous TA solution process successfully induced anisotropy structures into the gel.
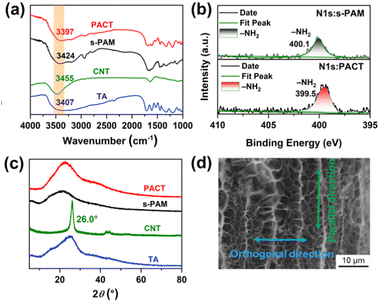 |
| Fig. 2 FT-IR spectra of PACT hydrogel, s-PAM hydrogel, CNTs, and TA (a). N1s spectrum of s-PAM hydrogel and PACT hydrogel (b). XRD patterns of PACT hydrogel, s-PAM hydrogel, CNTs, and TA (c). Muscle-inspired anisotropic microstructures along the stretching direction (d). | |
3.2 Adhesion performances
Skin deformation during body movements could not be fully monitored by traditional sensors, due to their insufficient surface-adaptive adhesion. Therefore, self-adhesion properties are essential for wearable flexible devices to be able to obtain real-time and accurate electrical signals.25,26 Since the successful introduction of TA, the anisotropic hydrogel could spontaneously adhere to various substrates with specific properties, including wood, glass, metal, plastic, and rubber (Fig. S5, ESI†). Later, we investigated the effects of TA concentration and soaking time on the adhesive and conductive properties of PACT hydrogels by the standard lap shear tests.38,76Fig. 3a and b show shear load-displacement curves of the PACT hydrogel adhered to various substrates. The adhesion strengths of the PACT hydrogel to glass, plastic, rubber, cardboard, metal, and wood were 36.6 kPa, 40.8 kPa, 61.4 kPa, 74.6 kPa, 57.3 kPa, and 77.2 kPa, respectively. The multiple hydrogen bonds between TA and PAM increased the cohesive energy of the hydrogel, while the free catechol groups from TA excluded interfacial water and achieved adhesion to various substrates through hydrogen bonds, ionic interactions, and dipole interactions.24,67 The highest adhesion strength to wood was attributed to the roughness of wood, which increased the contact area accordingly.38,77 The conductive properties remained almost constant while the adhesion strength could be largely adjusted by the TA concentration and soaking time (Fig. S6, ESI†). After optimization, maximal sensitivity and adhesion were achieved by soaking the PAM aerogel in 5 wt% TA aqueous solution for 20 hrs. As shown in Fig. 3c, it also exhibited reproducible adhesion on wood because of reversible physical interactions. The adhesion strength was still 74.1 kPa after 5 peel-off/adhesion cycles, with a retention rate of 96.0%, outperforming traditional adhesive gels (Fig. 3d).38,42,78,79 Of particular importance was that the hydrogel adhered firmly to irregular interfaces such as the back of the hand, knuckles, wrists, and elbows, and did not fall off when the finger was repeatedly bent more than 1000 times (Fig. S7, ESI†). Such a stable adhesion is extremely important for accurate signal acquisition.11,29
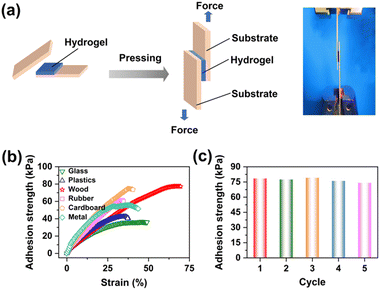 |
| Fig. 3 Self-adhesive properties of hydrogels. Schematic description of the lap shear test (a). Representative shear load-displacement curves of the hydrogels with different substrates (b). Repeated adhesion of the hydrogels to wood (c). | |
3.3 Anisotropic mechanical and conductive properties
The PACT hydrogels exhibited anisotropic mechanical and conductive properties. As shown in Fig. 4a, the tensile stresses of PACT-P hydrogels and PACT-O hydrogels were 75.2 kPa and 22.2 kPa, respectively, due to the oriented arrangement of the polymers. The anisotropy ratio (defined as the strength ratio between parallel and orthogonal directions) was 3.39, similar to literature reports.80,81 Since Young's modulus of human skin was around 25 kPa, PACT hydrogel had skin-comparable Young's modulus (23.4 kPa), which should allow it to act as a wearable sensor.35,82 The electrical conductivity of PACT hydrogel was also anisotropic (Fig. 4b). The conductivities of the hydrogel in a parallel direction and orthogonal direction were 12.8 S m−1 and 7.8 S m−1, respectively. The conductivity anisotropy ratio was 1.64, close to that reported earlier.6,45,83 Bearing anisotropic mechanical and conductive properties, the hydrogel could be adhered to the human skin to monitor wrist bending (Fig. 4c). Clearly, the ΔR/R0 monotonically increased with the increase in the bending angle. Upon repeated wrist bending, ΔR/R0 of the PACT-P hydrogel behaved much more prominently than the PACT-O hydrogel. These results suggested the existence of different electron transport channels, which was consistent with the higher conductivity parallel to the pre-stretching direction (Fig. 4b). Similarly, the sensitivity of PACT-P to monitor elbow joint flexion was better than that of the PACT-O hydrogel (Fig. 4d). Considering that the PACT-P gel had better mechanical and conductive performances, it was adopted as a strain sensor to detect human movements.
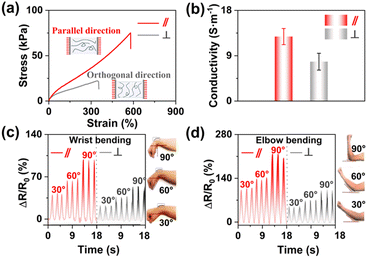 |
| Fig. 4 Parallel and orthogonal directional strengths of the PACT hydrogel (a). Parallel and orthogonal directional conductivity of the PACT hydrogel (b). Relative resistance variation of the anisotropic hydrogel sensor to detect wrist (c) and elbow bending (d). | |
3.4 Electrical performances
Because of the CNTs, the PACT-P hydrogel exhibited potential as a strain sensor (Fig. S8, ESI†). After optimization, maximal sensitivity was achieved at a CNT mass ratio of 0.8% with a pre-stretching ratio of 50% (Fig. S9, ESI†). The PACT-P hydrogel was fabricated by pre-stretching, air-drying in a confining geometry, and soaking in TA solution, thereby forming regular electron channels. When it was performed alongside the pre-stretching direction, the electrons moved from gaps between the CNTs.32,40,69,75 Therefore, hydrogels had excellent electrical conductivity. Fig. 5a shows that the PACT-P hydrogel was highly sensitive to tiny strains. For instance, the relative resistance increased from 0.6% to 9.1% when the strain elevated slightly from 0.2% to 3%. It had high sensitivity not only in the low strains but also in the large strains. For instance, the relative resistance increased from 30.0% to 1736.1% with the increment in strain from 10% to 150%, indicating that the PACT-P hydrogel had great potential in monitoring human body movements (Fig. 5b). The GF of PACT-P could reach 3.2 within the 100% strain range (Fig. 5c), which could make it possible to detect small movements of the human Impressively. The GF was 62.2 when the strain was above 120%. This is a common phenomenon in electronic conductive hydrogels,41,48,49,84,85 mainly due to the structural change in the conductive frameworks under stretching. For example, the GF of the CNT-based sensor jumped sharply from 0.15 to 11.8 when the strain exceeded 100%.85 In our system, numerous CNTs were entangled and folded in the complex networks inside the polyacrylamide matrix to form the internal conductive pathway, leading to good conductivity (Fig. 4b). At small strains (within 100%), a stable contact was formed between CNTs,85 resulting in the moderate GF (Fig. 5c). Under large strains (near 150%), CNTs slid and the distance between them increased. Therefore, CNTs were separated from each other and disconnected accordingly, which obviously broke the conductive pathways, leading to a relatively higher GF.48,49 It could respond quickly with a response and recovery times near 200 ms (Fig. 5d). Since the GF of traditional sensors was around 1.0, GF above 25 was defined as ultra-sensitive.2 As shown in Table S1 (ESI†), the PACT-P hydrogel exhibited better performances than most sensors, in terms of sensitivity and adhesion strength.
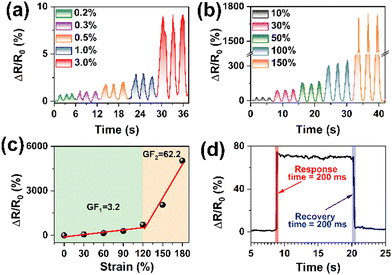 |
| Fig. 5 Relative resistance changes of the PACT-P hydrogel sensor for tiny strain (a) and large strain (b). GF of the PACT-P hydrogel (c). The resistance variation of the PACT-P gel during the loading-unloading process (d). | |
3.5 Sensing performances
Having high sensitivity and self-adhesion properties, it could be directly attached to human skin to detect human movements (Fig. 6a). Fig. 6b shows the ΔR/R0 values of the sensor with the bending angle of the forefinger. When the bending angle increased from 30° to 120° in sequence, the relative resistance monotonically increased.
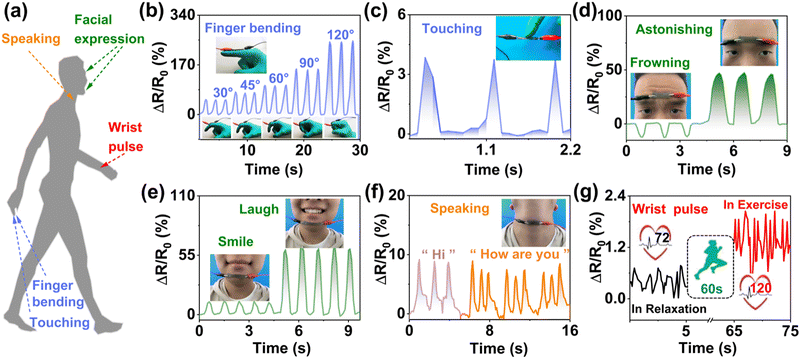 |
| Fig. 6 Real-time monitoring of human motions by the PACT-P hydrogel electronic sensor. Schematic showing the sensing locations (a), finger bending (b), and touching (c). Signals of relative electric resistance during making expressions of frowning and astonishing (d), smiling and laughing (e), speaking different phrases (f), the relative resistance changes vs. time when monitoring the pulse before and after exercise (g). | |
Additionally, it could detect repeated finger pressing with stable response signals (Fig. 6c). Furthermore, it was also applied to detect complex facial muscle motion changes (Fig. 6d and e). The sensor was placed at the forehead and a valley value appeared when frowning. When astonishing, the signal peak appeared, as shown in Fig. 6d. Similarly, expressions of smiling and laughing could be recognized by the disparate intensity of the ΔR/R0 value (Fig. 6e). These results indicate that the prepared strain sensor is reliable and highly sensitive. Taking the advantage of the excellent sensitivity at tiny strains (Fig. 5a), the hydrogel sensor was then exploited to discern subtle human motions. Tiny yet complicated muscle movements during speaking were successfully detected by the strain sensor. Fig. 6f shows the resistance signals obtained based on the vibration of the throat during speech. The image displayed similar features when “Hi” and “How are you” were said three times. As shown in Fig. 6g, by calculating the output signals of the relative resistance, the average blood pulse was 72 beats per minute under regular conditions, which corresponded well with the pulse beat of a healthy man.86 After exercise, the amplitude and frequency of the output signals obviously enhanced, giving an average blood pulse of 120 beats per minute. These results verified the high sensitivity and fast response of the sensor. The above experiments illustrated that the PACT-P hydrogel could efficiently and sensitively recognize signals from human activities, such as finger bending, different expressions, and vocal cord vibrations.
4. Conclusions
In short, an anisotropic sensor was prepared by a combined strategy of pre-stretching, air-drying in a confining geometry, and immersion in an aqueous TA solution to mimic the orientation structure of muscle. The resultant hydrogel showed anisotropic mechanical and conductive performances on account of the regular structures. The tensile strength and gauge factor parallel to the stretching direction were higher than those orthogonal to the stretching direction. The prepared sensor also exhibited anisotropic sensing for multidirectional stress in the strain range of 0.2 to 200%. The presence of CNTs and ordered structures imparted fast response (response time of 200 ms) and high sensitivity (gauge factor was 62.2 above 120% strain). The free catechol groups from TA provided self-adhesion properties (adhesion strength = 77.2 kPa). Consequently, it could spontaneously adhere to the skin, fingers, and elbows, enabling accurate and reliable detection of every nuance of expression, pulses, and vocal-cord vibration. This work provides the basis for the manufacture of high-performance sensors, showing great potential in ionic skin, man-machine interface, wearable sensors, and human health monitoring.
Author contributions
Wentang Wang: data curation, formal analysis, investigation. Xinyue Deng: methodology, formal analysis, investigation. Chunhui Luo: conceptualization, funding acquisition, Writing – review and editing, and supervision.
Conflicts of interest
There are no conflicts to declare.
Acknowledgements
The work was supported by the National Natural Science Foundation of China (52063001), Ningxia low-grade resource high-value utilization and environmental chemical integration technology innovation team project, China, Innovative team for transforming waste cooking oil into clean energy and high value-added chemicals, China, and Foundation of Academic Top-notch Talent Support Program of North Minzu University (2019BGGZ11).
Notes and references
- Z. Chen, Y. Chen, M. S. Hedenqvist, C. Chen, C. Cai, H. Li, H. Liu and J. Fu, J. Mater. Chem. B, 2021, 9, 2561–2583 RSC.
- X. Sun, F. Yao and J. Li, J. Mater. Chem. A, 2020, 8, 18605–18623 RSC.
- C. Yang and Z. Suo, Nat. Rev. Mater., 2018, 3, 125–142 CrossRef CAS.
- Z. Wu, X. Yang and J. Wu, ACS Appl. Mater. Interfaces, 2021, 13, 2128–2144 CrossRef CAS PubMed.
- F. Fu, J. Wang, H. Zeng and J. Yu, ACS Mater. Lett., 2020, 2, 1287–1301 CrossRef CAS.
- J. Chen, Y. Zhu, X. Chang, D. Pan, G. Song, Z. Guo and N. Naik, Adv. Funct. Mater., 2021, 31, 2104686 CrossRef CAS.
- C. Ma, M. G. Ma, C. Si, X. X. Ji and P. Wan, Adv. Funct. Mater., 2021, 31, 2009524 CrossRef CAS.
- H. Yan, Y. Wang, W. Shen, F. Li, G. Gao, T. Zheng, Z. Xu, S. Qian, C. y Chen, C. Zhang, G. Yang and T. Chen, Adv. Funct. Mater., 2022, 32, 2203241 CrossRef CAS.
- D. Chen and Q. Pei, Chem. Rev., 2017, 117, 11239–11268 CrossRef CAS.
- S. Xia, Q. Zhang, S. Song, L. Duan and G. Gao, Chem. Mater., 2019, 31, 9522–9531 CrossRef CAS.
- S. Li, Y. Cong and J. Fu, J. Mater. Chem. B, 2021, 9, 4423–4443 RSC.
- Z. Deng, H. Wang, P. X. Ma and B. Guo, Nanoscale, 2020, 12, 1224–1246 RSC.
- M. Seong, I. Hwang, S. Park, H. Jang, G. Choi, J. Kim, S. K. Kim, G. H. Kim, J. Yeo and H. E. Jeong, Adv. Funct. Mater., 2021, 31, 2107023 CrossRef CAS.
- I. Hwang, H. N. Kim, M. Seong, S. H. Lee, M. Kang, H. Yi, W. G. Bae, M. K. Kwak and H. E. Jeong, Adv. Healthcare Mater., 2018, 7, e1800275 CrossRef.
- G. Li, C. Li, G. Li, D. Yu, Z. Song, H. Wang, X. Liu, H. Liu and W. Liu, Small, 2022, 18, e2101518 CrossRef PubMed.
- C. Qian, T. Higashigaki, T. Asoh and H. Uyama, ACS Appl. Mater. Interfaces, 2020, 12, 27518–27525 CrossRef CAS PubMed.
- E. Prince and E. Kumacheva, Nat. Rev. Mater., 2019, 4, 99–115 CrossRef.
- L. Hsiao, L. Jing, K. Li, H. Yang, Y. Li and P. Chen, Carbon, 2020, 161, 784–793 CrossRef CAS.
- A. Chortos and Z. Bao, Mater. Today, 2014, 17, 321–331 CrossRef CAS.
- E. A. Lumpkin and M. J. Caterina, Nature, 2007, 445, 858–865 CrossRef CAS.
- C. Liu, R. Zhang, P. Li, J. Qu, P. Chao, Z. Mo, T. Yang, N. Qing and L. Tang, ACS Appl. Mater. Interfaces, 2022, 14, 26088–26098 CrossRef CAS.
- Z. Qin, X. Sun, Q. Yu, H. Zhang, X. Wu, M. Yao, W. Liu, F. Yao and J. Li, ACS Appl. Mater. Interfaces, 2020, 12, 4944–4953 CrossRef CAS.
- Y. Wang, H. Huang, J. Wu, L. Han, Z. Yang, Z. Jiang, R. Wang, Z. Huang and M. Xu, ACS Sustainable Chem. Eng., 2020, 8, 18506–18518 CrossRef CAS.
- L. Han, K. Liu, M. Wang, K. Wang, L. Fang, H. Chen, J. Zhou and X. Lu, Adv. Funct. Mater., 2018, 28, 1704195 CrossRef.
- Y. Liang, K. Wang, J. Li, H. Wang, X. Q. Xie, Y. Cui, Y. Zhang, M. Wang and C. S. Liu, Adv. Funct. Mater., 2021, 31, 2107006 CrossRef.
- S. Wang, Y. Fang, H. He, L. Zhang, C. a Li and J. Ouyang, Adv. Funct. Mater, 2020, 31, 2007495 CrossRef.
- Y. Zhao, Z. Li, S. Song, K. Yang, H. Liu, Z. Yang, J. Wang, B. Yang and Q. Lin, Adv. Funct. Mater., 2019, 29, 1901474 CrossRef.
- Y. Zhou, C. Wan, Y. Yang, H. Yang, S. Wang, Z. Dai, K. Ji, H. Jiang, X. Chen and Y. Long, Adv. Funct. Mater., 2019, 29, 1806220 CrossRef.
- Y. Miao, M. Xu and L. Zhang, Adv. Mater., 2021, 33, e2102308 CrossRef.
- H. Zhang, J. Guo, Y. Wang, L. Sun and Y. Zhao, Adv. Sci., 2021, 8, e2102156 CrossRef.
- C. Shan, M. Che, A. Cholewinski, J. Ki Kunihiro, E. K. F. Yim, R. Su and B. Zhao, Chem. Eng. J., 2022, 450, 138256 CrossRef CAS.
- X. Sun, Z. Qin, L. Ye, H. Zhang, Q. Yu, X. Wu, J. Li and F. Yao, Chem. Eng. J., 2020, 382, 122832 CrossRef CAS.
- H. U. Rehman, Y. Chen, Y. Guo, Q. Du, J. Zhou, Y. Guo, H. Duan, H. Li and H. Liu, Composites, Part A, 2016, 90, 250–260 CrossRef CAS.
- C. Luo, S. Xie, X. Deng, Y. Sun, Y. Shen, M. Li and W. Fu, Eur. Polym. J., 2022, 167, 111047 CrossRef CAS.
- Q. Wang, X. Pan, H. Zhang, S. Cao, X. Ma, L. Huang, L. Chen and Y. Ni, J. Mater. Chem. A, 2021, 9, 3968–3975 RSC.
- M. Wu, J. Chen, Y. Ma, B. Yan, M. Pan, Q. Peng, W. Wang, L. Han, J. Liu and H. Zeng, J. Mater. Chem. A, 2020, 8, 24718–24733 RSC.
- S. Hu, L. Zhou, L. Tu, C. Dai, L. Fan, K. Zhang, T. Yao, J. Chen, Z. Wang, J. Xing, R. Fu, P. Yu, G. Tan, J. Du and C. Ning, J. Mater. Chem. B, 2019, 7, 2389–2397 RSC.
- Z. Wang, J. Chen, L. Wang, G. Gao, Y. Zhou, R. Wang, T. Xu, J. Yin and J. Fu, J. Mater. Chem. B, 2019, 7, 24–29 RSC.
- Z. Wang, Y. Cong and J. Fu, J. Mater. Chem. B, 2020, 8, 3437–3459 RSC.
- S. Xia, S. Song, F. Jia and G. Gao, J. Mater. Chem. B, 2019, 7, 4638–4648 RSC.
- Y. Zhang, E. Ren, A. Li, C. Cui, R. Guo, H. Tang, H. Xiao, M. Zhou, W. Qin, X. Wang and L. Liu, J. Mater. Chem. B, 2021, 9, 719–730 RSC.
- J. Yin, S. Pan, L. Wu, L. Tan, D. Chen, S. Huang, Y. Zhang and P. He, J. Mater. Chem. C, 2020, 8, 17349–17364 RSC.
- J. Yu, M. Wang, C. Dang, C. Zhang, X. Feng, G. Chen, Z. Huang, H. Qi, H. Liu and J. Kang, J. Mater. Chem. C, 2021, 9, 3635–3641 RSC.
- X. Pei, H. Zhang, Y. Zhou, L. Zhou and J. Fu, Mater. Horiz., 2020, 7, 1872–1882 RSC.
- X. Huang, X. Zhou, H. Zhou, Y. Zhong, H. Luo and F. Zhang, Nano Res., 2021, 14, 2589–2595 CrossRef CAS.
- Z. Lei, Q. Wang, S. Sun, W. Zhu and P. Wu, Adv. Mater., 2017, 29, 1700321 CrossRef.
- C. Luo, X. Deng and S. Xie, J. Mater. Chem. C, 2021, 9, 17042–17049 RSC.
- H. Liao, X. Guo, P. Wan and G. Yu, Adv. Funct. Mater., 2019, 29, 1904507 CrossRef.
- M. Amjadi, K. U. Kyung, I. Park and M. Sitti, Adv. Funct. Mater., 2016, 26, 1678–1698 CrossRef CAS.
- C. Chen, H. Qin, H. Cong and S. H. Yu, Adv. Mater., 2019, 31, e1900573 CrossRef PubMed.
- T. Hiratani, O. Kose, W. Y. Hamad and M. J. MacLachlan, Mater. Horiz., 2018, 5, 1076–1081 RSC.
- Y. Jiao, Y. Lu, K. Lu, Y. Yue, X. Xu, H. Xiao, J. Li and J. Han, J. Colloid Interface Sci., 2021, 597, 171–181 CrossRef CAS PubMed.
- G. Yan, S. He, S. Ma, A. Zeng, G. Chen, X. Tang, Y. Sun, F. Xu, X. Zeng and L. Lin, Chem. Eng. J., 2022, 427, 131896 CrossRef CAS.
- H. Fan and J. Gong, Macromolecules, 2020, 53, 2769–2782 CrossRef CAS.
- W. Kong, C. Wang, C. Jia, Y. Kuang, G. Pastel, C. Chen, G. Chen, S. He, H. Huang, J. Zhang, S. Wang and L. Hu, Adv. Mater., 2018, 30, e1801934 CrossRef PubMed.
- Y. Feng, H. Liu, W. Zhu, L. Guan, X. Yang, A. V. Zvyagin, Y. Zhao, C. Shen, B. Yang and Q. Lin, Adv. Funct. Mater., 2021, 31, 2105264 CrossRef CAS.
- C. Luo, M. Huang, X. Sun, N. Wei, H. Shi, H. Li, M. Lin and J. Sun, ACS Appl. Mater. Interfaces, 2022, 14, 2638–2649 CrossRef CAS PubMed.
- M. T. I. Mredha, Y. Z. Guo, T. Nonoyama, T. Nakajima, T. Kurokawa and J. Gong, Adv. Mater., 2018, 30, 1704937 CrossRef.
- C. Luo, Y. Liu and Z. Li, Soft Matter, 2012, 8, 2618–2626 RSC.
- X. Deng, S. Xie, W. Wang, C. Luo and F. Luo, Eur. Polym. J., 2022, 178, 111485 CrossRef CAS.
- Z. Ahmadian, A. Correia, M. Hasany, P. Figueiredo, F. Dobakhti, M. R. Eskandari, S. H. Hosseini, R. Abiri, S. Khorshid, J. Hirvonen, H. A. Santos and M.-A. Shahbazi, Adv. Healthcare Mater., 2021, 10, e2001122 CrossRef.
- P. Lin, T. Zhang, X. Wang, B. Yu and F. Zhou, Small, 2016, 12, 4386–4392 CrossRef CAS.
- C. Luo, A. Guo, Y. Zhao and X. Sun, Carbohydr. Polym., 2022, 286, 119268 CrossRef CAS PubMed.
- C. Luo, A. Guo, J. Li, Z. Tang and F. Luo, ACS Appl. Mater. Interfaces, 2022, 14, 35434–35443 CrossRef CAS PubMed.
- W. Yang, H. Furukawa and J. Gong, Adv. Mater., 2008, 20, 4499–4503 CrossRef CAS.
- X. Xie, Y. Mai and X. Zhou, Mater. Sci. Eng., R, 2005, 49, 89–112 CrossRef.
- Q. Wang, Q. Zhang, G. Wang, Y. Wang, X. Ren and G. Gao, ACS Appl. Mater. Interfaces, 2022, 14, 1921–1928 CrossRef CAS.
- H. Fan, J. Wang and Z. Jin, Macromolecules, 2018, 51, 1696–1705 CrossRef CAS.
- H. Jafari, P. Ghaffari-Bohlouli, S. V. Niknezhad, A. Abedi, Z. Izadifar, R. Mohammadinejad, R. S. Varma and A. Shavandi, J. Mater. Chem. B, 2022, 10, 5873–5912 RSC.
- J. Sun, X. Zhao, W. R. K. Illeperuma, O. Chaudhuri, K. H. Oh, D. J. Mooney, J. J. Vlassak and Z. Suo, Nature, 2012, 489, 133–136 CrossRef CAS PubMed.
- K. Kim, M. Shin, M. Koh, J. H. Ryu, M. S. Lee, S. Hong and H. Lee, Adv. Funct. Mater., 2015, 25, 2402–2410 CrossRef CAS.
-
E. Pretsch, P. Buhlmann and M. Badertscher, Structure Determination of Organic Compounds, 2009, pp. 269–336 Search PubMed.
- D. Ishii, T. Nagashima, M. Udatsu, R. Sun, Y. Ishikawa, S. Kawasaki, M. Yamada, T. Iyoda and M. Nakagawa, Chem. Mater., 2006, 18, 2152–2158 CrossRef CAS.
- Y. Xian and Z. Kang, Prog. Org. Coat., 2020, 140, 105486 CrossRef CAS.
- X. Yu, W. Qin, X. Li, Y. Wang, C. Gu, J. Chen and S. Yin, J. Mater. Chem. A, 2022, 10, 15000–15011 RSC.
- K. Zhang, X. Chen, Y. Xue, J. Lin, X. Liang, J. Zhang, J. Zhang, G. Chen, C. Cai and J. Liu, Adv. Funct. Mater., 2021, 32, 2111465 CrossRef.
- X. Yao, S. Zhang, L. Qian, N. Wei, V. Nica, S. Coseri and F. Han, Adv. Funct. Mater., 2022, 32, 2204565 CrossRef CAS.
- S. Hao, C. Shao, L. Meng, C. Cui, F. Xu and J. Yang, ACS Appl. Mater. Interfaces, 2020, 12, 56509–56521 CrossRef CAS.
- Q. Yan, M. Zhou and H. Fu, Composites, Part B, 2020, 201, 108356 CrossRef CAS.
- X. Lin, X. Xing, S. Li, X. Wu, Q. Jia, H. Tu, H. Bian, A. Lu, L. Zhang, H. Yang and B. Duan, Adv. Funct. Mater., 2022, 32, 2112685 CrossRef CAS.
- Y. Chen, C. Jiao, X. Peng, T. Liu, Y. Shi, M. Liang and H. Wang, J. Mater. Chem. B, 2019, 7, 3243–3249 RSC.
- F. Cai, B. Yang, X. Lv, W. Feng and H. Yu, Sci. Adv., 2022, 8, eabo1626 CrossRef CAS.
- G. Yan, S. He, G. Chen, X. Tang, Y. Sun, F. Xu, X. Zeng and L. Lin, Carbohydr. Polym., 2022, 276, 118783 CrossRef CAS.
- L. Zhang, J. Wang, S. Wang, L. Wang and M. Wu, J. Mater. Chem. C, 2022, 10, 4327–4335 RSC.
- Y. Lu, Y. Yue, Q. Ding, C. Mei, X. Xu, Q. Wu, H. Xiao and J. Han, ACS Appl. Mater. Interfaces, 2021, 13, 50281–50297 CrossRef CAS PubMed.
- Y. J. Liu, W. T. Cao, M. G. Ma and P. Wan, ACS Appl. Mater. Interfaces, 2017, 9, 25559–25570 CrossRef CAS PubMed.
|
This journal is © The Royal Society of Chemistry 2023 |