DOI:
10.1039/D3SD00007A
(Paper)
Sens. Diagn., 2023,
2, 445-456
An electrochemiluminescent sensor based on hydrophilic CsPbBr3/TDPA nanocrystals for sensitive detection of nitrobenzene
Received
6th January 2023
, Accepted 7th February 2023
First published on 9th February 2023
Abstract
The extreme sensitivity of perovskite nanoparticles to water has seriously hindered their application. At present, all inorganic perovskite nanocrystals (NCs) have received extensive attention due to their excellent photoelectric properties. A highly hydrophilic composite CsPbBr3/TDPA NCs was synthesized in the presence of oleylamine molecules, which greatly enhanced its water stability. The electrochemiluminescence (ECL) intensity and stability of the composites are much higher than those of single CsPbBr3 quantum dots (QDs). For the first time, we have developed a sensor for the specific detection of nitrobenzene using the ECL method. It was found that nitrobenzene could significantly enhance the ECL signal of CsPbBr3/TDPA NCs. The ECL sensor showed a good linear relationship with the concentration of nitrobenzene in the range of 1 mM to 0.1 μM, and the detection limit was as low as 0.05 μM (S/N = 3). This work provides a new idea for the development of nitrobenzene ECL sensors.
With the development of science and technology, environmental pollution is becoming more and more serious due to the mass production and wide application of various chemical raw materials. Nitrobenzene compounds are widely used in chemical raw materials. For example, nitrobenzene is used in the manufacture of explosives, fuels, pesticides, drugs and other raw materials.1 There are also some enterprises that use nitrobenzene as a solvent, used in coatings, shoes, floor materials and other production activities.2 However, nitrobenzene can poison people and animals, damage the blood circulation and nervous system, and cause anemia and even death.3 Nitrobenzene is usually stable in water due to the strong electron-absorbing properties of the nitro group of the aromatic ring.4 The detection methods of nitrobenzene mainly include fluorescence (FL)5 and scanning tunneling microscope break junction technique (STM-BJ).6 Nevertheless, these methods may lack a certain degree of sensitivity and their costs are not easily estimated. At present, the ECL detection method of nitrobenzene has not been reported. ECL analysis technology is widely used in the detection field because of its advantages of low detection limit and high sensitivity.
Due to their excellent photoelectric properties, lead halide perovskites have attracted extensive attention in recent years. Perovskite is a direct bandgap semiconductor and has excellent carrier transport characteristics, and its optical bandgap can be adjusted by changing the type and proportion of halogen atoms.7 At present, all inorganic perovskite nanocrystals (NCs) have been widely used in the preparation of high-efficiency solar cells,8 light-emitting diodes,9 lasers10 and photodetectors.11 They also have many applications in the field of analysis and testing, such as fluorescent probes,12 ECL,13 photoelectric chemistry14 and so on. However, the stability of all inorganic perovskite nanoparticles is poor, especially in water, which greatly limits their practical application. Ligand engineering and coating with inert shells have proved to be effective methods to improve the stability of perovskites. In the absence of any amines, ligands reduce non-radioactive recombination pathways from surface defects. Therefore, oleamine ligands were identified to contribute to reaction kinetics. They have the ability to interact with the surface of cesium bromide NCs through the formation of hydrogen bonds, via either direct ligand addition or substitution of the surface Cs+ cations. Alkyl phosphinic acids, which have a strong affinity for Pb2+, contain two chelating sites that allow the ligand to interact strongly with the surface of perovskite nanoparticles and have been used to replace oleic acid.15 For example, Kovalenko et al. first prepared CsPbX3 perovskite NCs by hot injection of cesium stearate in PbBr2 solution at 170 °C with the assistance of long-chain organic ligands such as octadecene and oleylamine.16 Wu et al. synthesized sodium (Na) passivated CsPbBr3 nanocrystals at room temperature using a modified ligand-assisted reprecipitation method and dispersed them in water/n-hexane solution.17 However, after some surface modification, they still have inherent fragility in terms of stability. This not only hinders the application of perovskite NCs in photochemistry, but also becomes a major obstacle to their future application in electrochemiluminescence.
Electrochemiluminescence (ECL) is a kind of light radiation produced by electrochemically induced energy relaxation of excited states.18 It has the advantages of high sensitivity, low background signal and easy control.19 CsPbBr3 QDs have excellent photoelectric performance, and many researchers have begun to explore their ECL performance. Zhu et al. obtained strong and stable ECL from CsPbBr3 QDs by hydrolyzing tetramethyl orthosilicate while encapsulating CsPbBr3 QDs and a coreactant (CoR) into an in situ generated SiO2 matrix.20 Wang et al. used hydrogen peroxide as the co-reactive agent for the first time to investigate the charge transfer related anodic redox and ECL of all-inorganic perovskite CsPbBr3 NCs in aqueous media.21 However, most studies based on CsPbBr3 QDs only focus on anode ECL, and there are few reports on cathode ECL of perovskites. Cathodic ECL also has unique advantages, such as expanding the possible types of ECL reactions, eliminating the oxidation products of the emitter, and reducing the interference of compounds present in the sample.22 Therefore, it is of great significance to explore the cathode ECL properties of all inorganic perovskites.
The application of self-assembled alkyl phosphate monolayers on metal or metal oxide surfaces has been used as a stabilization method to protect metal surfaces and interfaces against corrosion.23 In this work, we synthesized tetradecylphosphonic acid (TDPA) coated CsPbBr3 nanocrystals by supersaturated crystallization rather than by hot injection. This method has the advantages of simple and controllable synthesis conditions, cost saving and easy to carry out large-scale synthesis. We systematically studied the cathodic ECL performance of CsPbBr3/TDPA NCs in aqueous solution. The results show that the NCs still maintained good ECL performance after one week of dispersion in water. CsPbBr3 QD based ECL analysis has been performed in the aqueous phase, expanding its application in biological analysis. Compared with pure CsPbBr3 NCs, the material overcomes the luminescence instability defect of CsPbBr3 QDs and has better ECL signal intensity. A series of classical semiconductor nanocrystals, such as CdSe,24 CdS,25 and ZnS,26 have ECL emission characteristics. However, they have been criticized for the stability of their ECL response signal, especially the instability of NCs in the ECL process with high excitation electrochemical potential. Moreover, the low ECL signal intensity of some two-dimensional nanosheets also limits their application in ECL analysis. For example, the ECL emission of a g-C3N4 film rapidly degrades at the applied potential range of 0 to −1.5 V.27 This can be ascribed to electrode passivation occurring immediately after one cyclic potential scan, leading to a sharp decrease in ECL emission at subsequent potential scans. Although a stable ECL signal can be obtained by using small cathodic potentials, the ECL intensity is apparently too weak for analytical application. Nevertheless, the combined action of TDPA and oleylamine molecules improved the surface defect state of CsPbBr3 QDs and promoted the charge transfer recombination of quantum dots and excited states, as well as greatly enhancing the ECL signal intensity and luminescence stability. However, CsPbBr3/TDPA NCs have not been used in the application of ECL research right now. This material overcomes the characteristics of poor conductivity of traditional CsPbBr3 QDs, and has excellent electrochemical activity and electron transport ability. Moreover, nitrobenzene can specifically enhance the ECL signal of CsPbBr3, which is believed to have broad development prospects.
Experimental section
Chemicals and materials
Caesium bromide (99.5%), lead bromide (99.0%), and oleylamine (OAm, 80–90%) were obtained from Aladdin. Toluene (99.8%) was purchased from Aldrich. N,N-Dimethylformamide (DMF, 99.8%), sodium chloride (AR, 99.5%), potassium chloride (99.5%), sodium phosphate dibasic (99.99% metals basis), potassium phosphate monobasic (99.5%), potassium persulfate (99.5%), nitrobenzene (AR, 99%), 1-tetradecylphosphonic acid (TDPA, 98%), and oleic acid (OA, AR) were obtained from Macklin.
Apparatus
X-ray polycrystalline diffractometer (XRD, Smartlab, 9 kW, Japan), X-ray photoelectron spectroscopy (XPS, ESCALAB 250, the United States), fluorescence spectrometer (PL, F-2500, Japan), Fourier transform infrared spectrometer (FTIR, NEXUS-870, Japan), ultraviolet visible absorption spectrometer (UV-1750, Shimadzu, Japan), scanning electron microscope (SEM, REGULUS8230, Japan), transmission electron microscope (TEM, JEM-2100, Japan). All electrochemical measurements were carried out on a CHI 660E electrochemical workstation (Shanghai CH Instrument Co., Ltd., China) and the measurements of electrochemiluminescence (ECL) were taken on an MPI-E electrochemiluminescence analyzer (Xi'An Remax Electronic Science & Technology Co. Ltd., Xi'An, China). During the test, a Ag/AgCl electrode was selected as the reference electrode, a platinum wire electrode as the auxiliary electrode and a glassy carbon electrode (GCE) as the working electrode. The photomultiplier tube voltage is set to 600 V. Electrochemical impedance spectroscopy (EIS) measurements were carried out on an AutoLab electrochemical analysis instrument (Holland).
Preparation of CsPbBr3 QDs
Traditional CsPbBr3 QDs were prepared according to methods reported in the literature.28 In brief, 0.1 mmol of CsBr and 0.1 mmol of PbBr2 were dissolved in 2 mL of DMF, and then 80 μL of OAm and 250 μL of OA were added with vigorous stirring. Then, strong green emission was observed when 200 μL precursor solution was dispersed into 3 mL toluene solvent.
Preparation of CsPbBr3/TDPA NCs
CsPbBr3/TDPA NCs were synthesized according to the literature.29 Some details have been slightly changed. In brief, 0.1 mmol of CsBr, 0.1 mmol of PbBr2, 25 mg of TDPA and 0.125 mL of OAm were dissolved in 2.5 mL of DMF and stirred vigorously. Then, 200 μL of the precursor solution was dispersed into 2 mL toluene solvent and centrifuged at 9000 rpm for 10 min. At last, the NCs were washed with cyclohexane several times, and dried in a vacuum drying oven. The synthesis process of CsPbBr3/TDPA NCs is shown in Scheme 1(A).
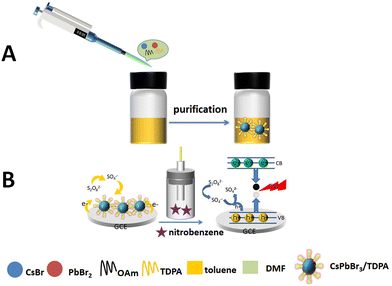 |
| Scheme 1 (A) The synthesis process of CsPbBr3/TDPA NCs. (B) Construction and detection of ECL sensors. | |
Construction of the sensor
The assembly process of the sensor is shown in Scheme 1(B). Firstly, the glassy carbon electrode was polished with alumina powder, and then sonicated in ultrapure water, ethanol and ultrapure water, and finally the surface of the GCE was dried with nitrogen. 10 μL of 0.5 mg mL−1 CsPbBr3/TDPA NCs were dropped on the electrode surface and dried in air for electrochemical testing. The prepared sensor was placed in 5 mL test liquid containing 0.12 M K2S2O8 and 10 mM PBS (pH = 7.4). The scanning potential range varies from 0 to −2.0 V, the scanning rate is 100 mV s−1, and the high voltage of the photomultiplier tube is set at 600 V.
Results and discussion
Morphological and structural characterization
Fig. 1A shows the TEM images of CsPbBr3/TDPA NCs aqueous solution at the initial stage, in which the NCs presented the shape of regular nanocubes. In addition, the size uniformity of nanoparticles coated with TDPA and OAm is also fabulous. The high-resolution TEM (HR-TEM) image (Fig. 1B) of the NCs showed a d-spacing of 2.9 Å, which was consistent with the (200) crystal face of cubic phase CsPbBr3.30 As shown in Fig. 1C, the as-prepared CsPbBr3 showed a nanoplate shape with good size uniformity.
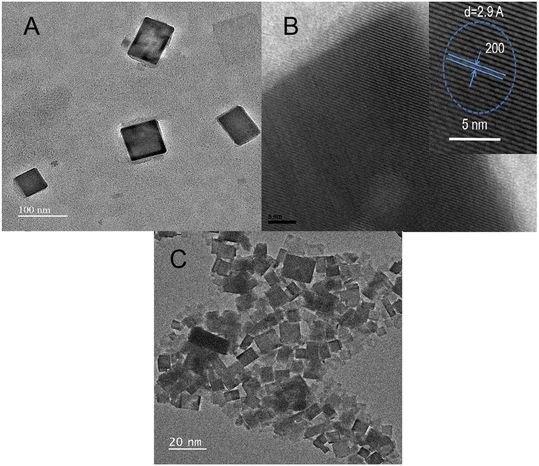 |
| Fig. 1 The (A) TEM and (B) HR-TEM images of CsPbBr3/TDPA NCs. (C) The TEM image of CsPbBr3 QDs. | |
We further analyzed the crystal phase structure of CsPbBr3/TDPA NCs powder by XRD, as shown in Fig. 2A. There are also broad peaks and some impurity peaks on the curve of CsPbBr3/TDPA NCs, which can be confirmed as the peak of TDPA by comparing with the XRD spectrum of TDPA. At the same time, the main diffraction peaks of NCs correspond to the (100), (110), (200), (210), (211) and (202) crystal planes of cubic phase CsPbBr3, respectively, indicating that the CsPbBr3/TDPA NCs have a cubic crystal structure.31 We further investigated the crystal structure changes of nanoparticles dispersed in water for different periods of time (Fig. 2B). After one or even 5 days of CsPbBr3/TDPA NCs dissolution in water, the position of the XRD peaks almost did not change, indicating that the NCs basically maintained the original cubic phase structure. However, individual small peaks also appeared at 2θ = 23.5° and 2θ = 26.4°, which may be due to the recrystallization of the nanoparticles in water, resulting in a change in crystal orientation. At the same time, the prepared materials were characterized by Fourier transform infrared spectroscopy, and the results are shown in Fig. 2C. Curve a is the infrared spectrum of CsPbBr3/TDPA NCs, and curve b is the infrared spectrum of TDPA. By comparing the FTIR spectra of TDPA and CsPbBr3/TDPA NCs, the peaks at 2933 cm−1 and 2858 cm−1 can be summed up as the characteristic stretching vibration peaks of C–H. Meanwhile, curve b shows that the peaks at 1000 cm−1 and 962 cm−1 can be attributed to the characteristic stretching vibration peaks of P–O–H.32 Notably, curve a shows that the P
O tensile vibration at 1230 cm−1 is replaced by a broad peak at 1000–900 cm−1, indicating that the P
O and P–O–H bonds gradually break and produce Pb–O–P bonds.33 The successful synthesis of the NCs was confirmed. The UV-vis absorption spectra and photoluminescence spectra of the synthesized CsPbBr3/TDPA NCs and CsPbBr3 QDs solution are shown in Fig. 2D. The maximum absorption peak and fluorescence emission peak of CsPbBr3/TDPA are located at 512 nm and 519 nm, respectively. The CsPbBr3 QDs exhibit an absorption onset at 509 nm and an emission peak at 518 nm. A red shift was observed in CsPbBr3 NCs in both cases of absorption and emission upon loading into TDPA, which may be attributed to an increase in the size of NCs after engaging with TDPA. In this work, we used TDPA and OAm as ligands and it was found that the fluorescence intensity of CsPbBr3 synthesized with TDPA as the ligand was stronger than that with OA and OAm as ligands. This should be due to the more efficient surface passivation of CsPbBr3 NCs by TDPA, resulting in reduced surface defect density.
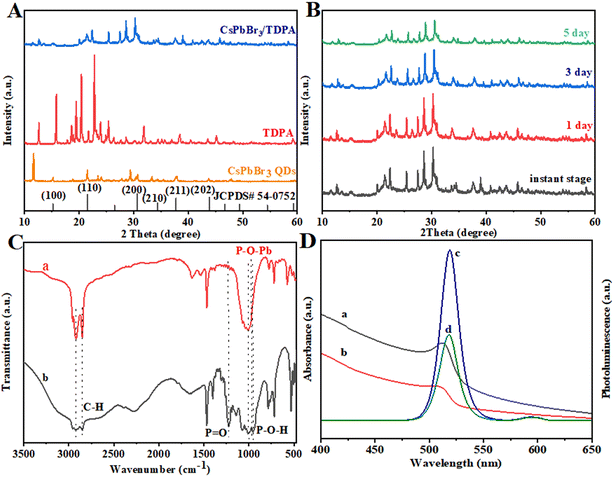 |
| Fig. 2 (A) XRD patterns of TDPA, CsPbBr3 QDs and CsPbBr3/TDPA NCs. (B) XRD patterns of CsPbBr3/TDPA NCs dispersed in water for different times. (C) The FTIR spectra of (a) CsPbBr3/TDPA NCs and (b) TDPA. (D) Absorbance spectra of CsPbBr3/TDPA NCs (a) and CsPbBr3 QDs (b); photoluminescence spectra of CsPbBr3/TDPA NCs (c) and CsPbBr3 QDs (d). | |
The valence states of surface elements of CsPbBr3/TDPA NCs were characterized by X-ray photoelectron spectroscopy. The results are shown in Fig. 3. Fig. 3A shows that the Cs, Pb, Br, C, N, O and P elements exist in CsPbBr3/TDPA NCs. The peaks of Pb 4f are located at 143 and 138.1 eV, corresponding to Pb 4f5/2 and Pb 4f7/2 in Fig. 3, respectively. Additionally, the Br 3d peaks could be fitted to two peaks (3d5/2, 3d3/2) with binding energies of 68.4 and 69.4 eV (Fig. 3C). Meanwhile, the peaks of O 1s are located at 529.8, 530.3 and 530.9 eV (Fig. 3D), corresponding to bulk O, Pb–O, and Pb–O–P bonds in CsPbBr3/TDPA NCs, respectively. The above results prove that we have successfully synthesized CsPbBr3/TDPA NCs.
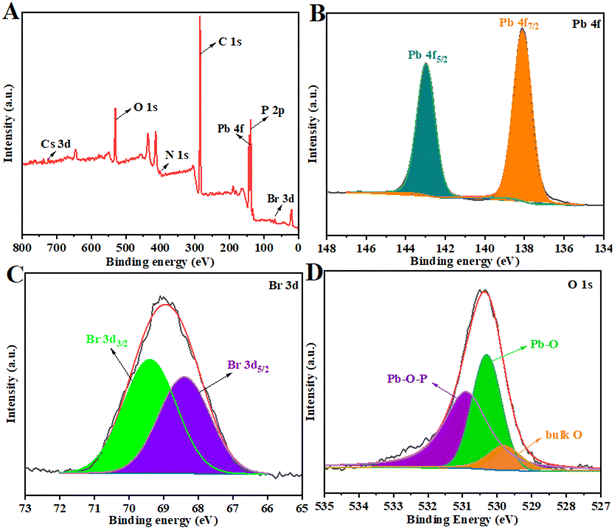 |
| Fig. 3 XPS analysis of the full region of CsPbBr3/TDPA NCs (A) and different elements Pb 4f (B), Br 3d (C), and O 1s (D). | |
Stability of CsPbBr3/TDPA NCs
Due to the poor stability of perovskite materials, it is difficult to maintain their original structure and optical properties due to the influence of environmental and interface factors. And the instability of perovskite-type nanomaterials in aqueous solution greatly limits their electrochemical applications. Therefore, the water stability of CsPbBr3/TDPA NCs was investigated by measuring their ECL. The CsPbBr3/TDPA NCs were dispersed in water until a clear homogeneous solution was formed. Then, 0.5 mg mL−1 aqueous CsPbBr3/TDPA NCs solution was modified on a GCE to detect the ECL intensity at different periods. As shown in Fig. 4, after one day of CsPbBr3/TDPA NCs dispersion in water, the ECL intensity still remained at 90% of the initial intensity. Compared to a previous study,34 it is worth noting that after a week of dispersion in water, the ECL intensity of the NCs was still 40% of the initial intensity, indicating that the water stability of the NCs was excellent. We conclude that the combined action of TDPA and OAm can improve the water stability of ECL and play an important role in delaying the degradation rate of CsPbBr3 NCs in water.
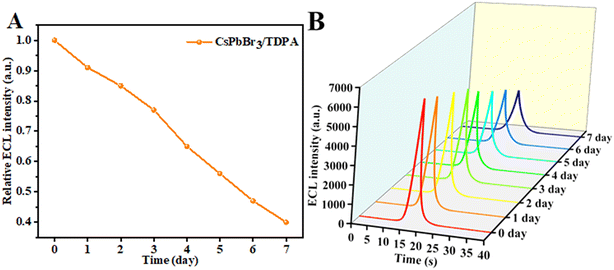 |
| Fig. 4 (A) The relative ECL intensity of 0.5 mg mL−1 NCs as a function of time in water. (B) Effects of aqueous storage time on the ECL peak intensity of CsPbBr3/TDPA NCs|GCE. | |
Feasibility of electrochemical sensors
In order to further explore the feasibility of the constructed sensor, electrochemical impedance spectroscopy (EIS), cyclic voltammetry (CV) and ECL tests were used to characterize the modification process of the electrode surface. EIS was used to monitor the changes of interface properties of different electrode surfaces with 0.05 mmol L−1 Fe(CN)63−/4−. The impedance spectrum includes a semicircle part and a linear part. The semi-circular part with higher frequency corresponds to the electron transfer limiting process, while the linear part with lower frequency represents the diffusion limiting process.35 The bare GCE has the smallest impedance value (curve a, Fig. 5A), indicating that the GCE has good electrical conductivity. The resistance of the GCE modified by CsPbBr3 QDs (Fig. 5B) is very large, indicating that its electrical conductivity is very poor. Compared with the bare GCE, the TDPA-modified GCE had higher impedance values (curve c in Fig. 5A). This is because TDPA, as a long organic small molecule, has a low electrical conductivity. Notably, the electron transfer resistance of CsPbBr3/TDPA NCs was significantly reduced after surface modification of the GCE. The alkyl phosphonic acid and perovskite divalent cations (lead ions) have a strong interaction, and the change of the Pb–Br skeleton structure and composition mainly regulates the electronic properties. When TDPA interacts with the perovskite, the interface of the CsPbBr3/TDPA NCs creates an electric potential that results in the spatial separation of carriers at the interface, which prevents the photoinduced radiative recombination of carriers and results in changes in their electrochemical properties. The results show that the prepared nanoparticles have very efficient electron transfer and excellent electrochemical activity. Fig. 5C shows the CV responses of the different modified electrodes. The exposed glassy carbon electrode (curve a) shows a pair of obvious Fe(CN)63−/4− redox peaks, indicating its excellent electron transport capacity. After modification of CsPbBr3/TDPA NCs on the electrode surface (curve b), the peak current of the Fe(CN)63−/4− redox pair decreased slightly. When TDPA was modified on the electrode surface (curve c), the separation degree of redox peaks gradually increased, and the peak current decreased successively. In particular, the anode peak and cathode peak significantly decreased when the electrode is modified by CsPbBr3 QDs (curve d), which just corresponds to the EIS results.
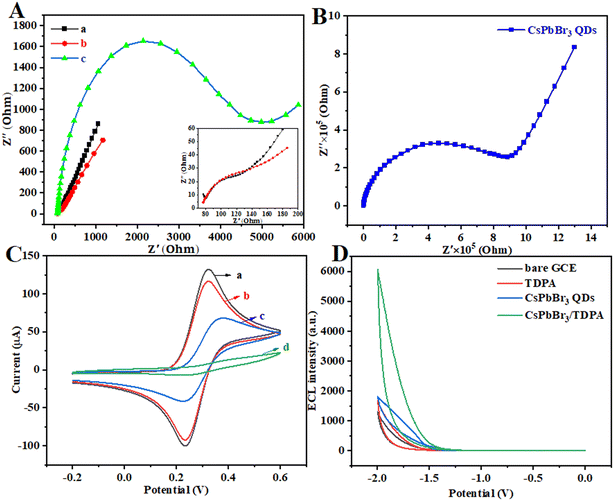 |
| Fig. 5 (A) EIS characterization of (a) bare GCE, (b) CsPbBr3/TDPA NCs and (c) TDPA. (B) EIS characterization of CsPbBr3 QDs. (C) CV characterization of (a) bare GCE, (b) CsPbBr3/TDPA NCs, (c) TDPA and (d) CsPbBr3 QDs. (D) ECL–potential curves of the different modified electrodes. | |
At present, the ECL properties of all inorganic perovskite nanocrystals are mostly concentrated on anodic ECL, and some related reports have shown that the ECL intensity of these materials in organic phases is very low. In this experiment, we measured the ECL response of a GCE modified at each step using K2S2O8 as a coreactant (Fig. 5D). As expected, the ECL response of bare GCE is small and negligible. However, it is worth noting that weak ECL emission can be observed in CsPbBr3 QDs|GCE. Moreover, after loading TDPA on the surface of the GCE, relatively weak ECL emission can also be observed due to its weak conductivity as a small organic molecule. Under the combined action of OAm and TDPA, a strong ECL signal was obtained at CsPbBr3/TDPA|GCE. Compared to that of unmodified CsPbBr3 QDs, the ECL signal intensity of CsPbBr3/TDPA is significantly increased by about 3.4 times, and the ECL luminous efficiency is greatly improved.
ECL mechanism of electrochemical sensors
Hole and electron injection processes play an important role in the generation of ECL of CsPbBr3 NCs. In previous reports, CsPbBr3 NCs or their redox products may interact with S2O82− to promote the generation of cathodic electrochemical luminescence. Therefore, we hypothesized that the ECL emission mechanism is caused by electron transfer annihilation between the SO4˙− oxidation product CsPbBr3/TDPA+ and the electroreduction product CsPbBr3/TDPA−. Subsequently, the electron transfer produces an excited state CsPbBr3/TDPA*, which is then relaxed by emission. The possible ECL mechanisms are as follows:36
S2O82− + e− → SO42− + SO4˙− |
SO4˙− + CsPbBr3/TDPA → SO42− + CsPbBr3/TDPA+ |
CsPbBr3/TDPA + e− → CsPbBr3/TDPA− |
CsPbBr3/TDPA+ + CsPbBr3/TDPA− → CsPbBr3/TDPA* |
CsPbBr3/TDPA* → CsPbBr3/TDPA + hv |
Based on previous reports, there is an interaction between perovskite colloidal particles and nitrobenzene.37 π-Conjugated molecules tend to have excellent electrical conductivity, and good rigidity and planarity, due to the existence of π-electrons. It was found that the introduction of conjugated groups can greatly increase the contribution of organic components to the band edge, and reduce the narrow band gap to a certain extent, so as to promote the electronic coupling between the inorganic and organic layers of low-dimensional perovskites, and further promote the carrier transport.38 Nitrobenzene is a compound rich in π-electrons, and its nitro group has a π–π interaction with the benzene ring. There is a strong interaction of π electrons between such conjugated molecules and the perovskite octahedron, that is, the nitrocellulose group can interact with the PbBr6 cage of the perovskite, which may produce a passivation effect and promote the phase stability of the low-dimensional perovskite. The phenyl group is hydrophobic and has the potential to prevent water from entering. Therefore, in the process of continuous ECL scanning, the interaction between the redox products of CsPbBr3/TDPA and S2O82− is further promoted due to the effect of defect passivation and water osmosis inhibition. This process results in more and more catalytic products in the electrolyte, and also increases the contact area with K2S2O8. Fig. 6 depicts the CV responses of bare GCE and CsPbBr3/TDPA in an air-saturated water medium with K2S2O8 as a coreactant. The obvious reduction peak of bare GCE at −0.97 V indicates that the strong oxidant SO4˙− is formed by electrochemical reduction of S2O82−. Compared with that of the GCE, the reduction current of the CsPbBr3/TDPA modified electrode was significantly increased in the presence of nitrobenzene, and the reduction potential was −0.74 V, which confirmed that nitrobenzene could indeed enhance the cathodic ECL of CsPbBr3/TDPA in aqueous solution.
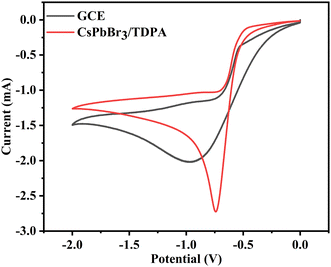 |
| Fig. 6 CV responses of bare GCE and CsPbBr3/TDPA NCs in 10 mM PBS containing 0.12 M K2S2O8 and 0.1 mM nitrobenzene. | |
Optimization of experimental conditions
In order to obtain optimal ECL response results, various experimental conditions were optimized as shown in Fig. 6. First, the scanning rate was optimized to select the best ECL response signal value between 60 and 140 mV s−1 (Fig. 7A). The resulting surface, without changing the rest of the conditions, can reach steady state when the scanning rate reaches 100 mV s−1, with the highest ECL intensity. However, when the scanning rate exceeds 100 mV s−1, the polarization speed of the electrode increases and the ECL intensity decreases. Secondly, the effect of K2S2O8 concentration on ECL intensity was also investigated (Fig. 7B). The intensity of ECL increases with increasing concentration of K2S2O8. When the concentration of K2S2O8 reaches 120 mM, the ECL intensity reaches the maximum. However, when the concentration of K2S2O8 exceeds 120 mM, too much SO4˙− will be produced on the electrode surface, leading to the gradual reduction of ECL intensity. In addition, the effect of ligand concentration on ECL intensity was also investigated (Fig. 7C). When the ligand concentration is less than 8 mg mL−1, the ECL intensity is low, which may be due to the poor passivation effect on the surface of CsPbBr3 QDs. However, when the ligand concentration was greater than 8 mg mL−1, the intensity of ECL decreased gradually. This is because the steric hindrance caused by the high concentration of TDPA leads to the generation of more unaligned atoms on the surface. Therefore, 8 mg mL−1 was chosen as the optimal ligand concentration. Finally, the effect of solution pH on ECL intensity was studied, and the experiment was carried out in the pH range of 5.5–8.5 (Fig. 7D). As the pH increases, the number of negatively charged species increases. In addition, the electron transfer speed is greatly accelerated, and the ECL intensity will also increase. At the same time, when the pH is greater than 7.4, too much negative charge will be deposited on the electrode surface, and the ECL intensity will also decrease. Therefore, 7.4 was chosen as the optimal pH.
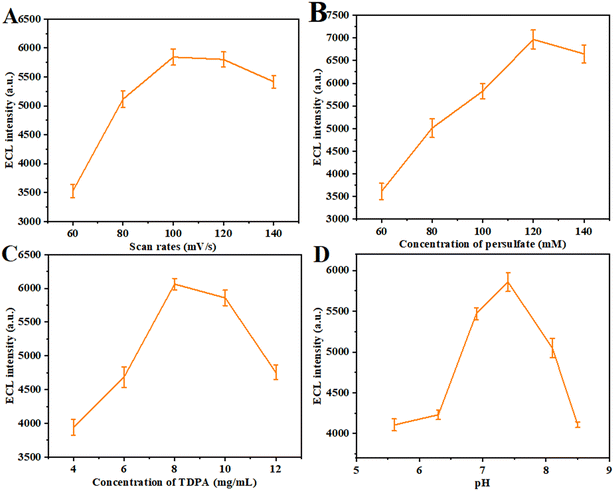 |
| Fig. 7 Optimization of the experimental conditions: (A) scan rates, (B) concentration of persulfate, (C) concentration of TDPA, and (D) pH. | |
Analytical performance of the ECL sensors
The ECL sensor was tested with different concentrations of nitrobenzene under the best experimental conditions. By the single variable method, the prepared CsPbBr3/TDPA/GCE sensor platform was placed in a mixture of 120 mM K2S2O8 and 10 mM PBS containing different concentrations of nitrobenzene for ECL analysis. As shown in Fig. 8A, with the increase of target concentration, the ECL response signal of the sensor gradually increases. In the range of nitrobenzene concentrations from 1 mM to 0.1 μM, the intensity of the ECL response signal was linearly related to the logarithm of nitrobenzene concentration (Fig. 8B). The linear fitting equation was I = 188.1301
log
C + 19
865, the correlation coefficient was 0.997, and the detection limit was 0.05 μM (S/N = 3). In fact, the LOD was calculated according to 3σ/S, where σ is the standard deviation of three independent blank sample responses and S is the slope of the analytical curve. Compared with previous studies (Table 1), the established sensor has better performance.
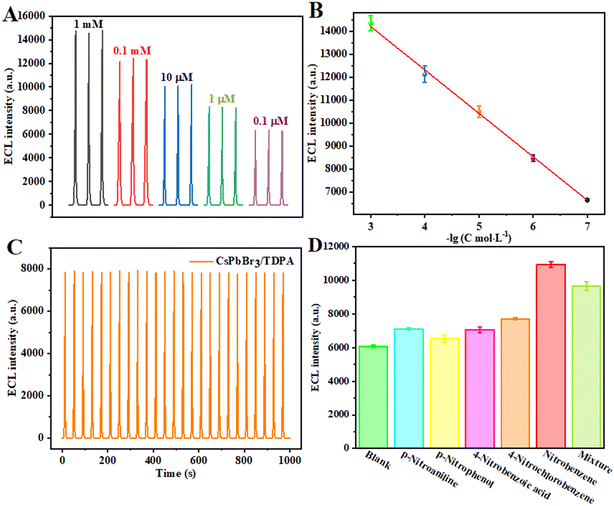 |
| Fig. 8 (A) Calibration curve and (B) ECL response for different nitrobenzene levels. (C) The stability of the constructed ECL sensing platform for 25 scanning cycles. (D) The selectivity of the fabricated sensing platform for various targets. | |
Table 1 Comparison of this method with other methods reported in the literature
Material |
Method |
Linear range |
LOD |
Ref. |
Zn-TDPAT |
Fluorescence |
50–5 × 103 ppm |
50 ppm |
39
|
[Ln(mtpc)1.5(DMA)(H2O)]·2H2O |
Fluorescence |
15–150 ppm |
15 ppm |
40
|
Ln-MOFs |
Fluorescence |
0–0.7 mM |
1.46 μM |
41
|
Co-NC-800 |
DPV |
0.1–63 μM |
0.086 μM |
42
|
EDAS/(g-C3N4-Ag)NC |
CV |
5–50 μM |
2 μM |
43
|
CsPbBr3/TDPA |
ECL |
0.1–1 × 103 μM |
0.05 μM |
This work |
Stability and selectivity of the sensor
It is well known that selectivity and stability are essential properties of excellent sensors. After 25 consecutive CV scanning cycles, the ECL intensity basically remained stable, indicating that the sensor had good stability (Fig. 8C). Meanwhile, in order to test the selectivity of the sensor, the ECL response characteristics of the sensor platform CsPbBr3/TDPA/GCE were used to detect a variety of different substances. We measured and compared the ECL signal responses of p-nitroaniline, p-nitrophenol, 4-nitrobenzoic acid, 4-nitrochlorobenzene and nitrobenzene (Fig. 8D). The concentration of all substances was 10 μM. Compared with the ECL intensity of the blank solution, the ECL intensity increased slightly with the interfering substances. At the same time, the ECL intensity of nitrobenzene increased obviously. In the detection of mixed samples, the signal corresponding to the ECL sensor is equivalent to the signal of the 10 μM nitrobenzene sample. Nitrobenzene has greater flexibility and smaller steric hindrance than the other detected substances. The orientation of other interfering materials may deviate substantially from the vertical direction, disrupting π–π stacking and reducing electron delocalization. At the same time, nitrobenzene, as a compound rich in π electrons, has a stronger interaction with the surface of the perovskite, and the intercalation configuration is less affected, so the constructed sensor has good selectivity. The results show that the CsPbBr3/TDPA/GCE sensor has good selectivity to nitrobenzene.
Detection of real samples
The sensor platform CsPbBr3/TDPA/GCE was used for the detection of real samples to evaluate the practical detection capability of the sensor platform. A tap water sample was tested, and a recovery experiment was carried out by the standard addition method, and then three different concentrations of samples were measured under the same conditions and the recovery of nitrobenzene was calculated. The results are shown in Table 2, and the recoveries are 97%, 101% and 103%, respectively, which proves that the sensor has high accuracy in real environmental samples.
Table 2 Measurement of TDPA in spiked samples
Serum sample |
Added (μM) |
Found (μM) |
Recovery |
RSD (%, n = 3) |
1 |
0 |
0.11 |
0 |
2.87 |
2 |
1 |
0.97 |
0.97 |
0.96 |
3 |
10 |
10.3 |
1.03 |
2.56 |
4 |
100 |
10.1 |
1.01 |
0.63 |
Conclusion
In this work, TDPA-coated CsPbBr3 QDs were synthesized by recrystallization at room temperature. Compared with those of unmodified CsPbBr3 QDs, the water stability and ECL response signal of the composite are greatly improved, and the ECL stability and reproducibility are also enhanced. ECL analysis based on CsPbBr3 QDs is performed in the aqueous phase, which expands its application in biological analysis. More importantly, it greatly improves the electrochemical performance of CsPbBr3 QDs and has more excellent electrical conductivity. It is found that nitrobenzene can effectively enhance the ECL response signal generated in a CsPbBr3/TDPA–K2S2O8 system. Based on this, an ECL sensor platform for nitrobenzene detection was prepared. In this work, the cathodic ECL of perovskite nanocrystals in aqueous solution was explored, and its potential application in ECL sensors and nanodevices was expanded.
Conflicts of interest
There are no conflicts to declare.
Acknowledgements
This work was supported by the National Natural Science Foundation of China (21976001), the University Collaborative Innovation Project of Anhui Province (GXXT-2019-023) and the Natural Science Research Projects of Universities in Anhui Province (KJ2021A0030).
References
- Y. Salinas, R. Martínez-Máñez, M. D. Marcos, F. Sancenón, A. M. Costero, M. Parra and S. Gil, Optical chemosensors and reagents to detect explosives, Chem. Soc. Rev., 2012, 41(3), 1261–1296 RSC.
- X. Yan, H. Wang, C. E. Hauke, T. R. Cook, M. Wang, M. L. Saha, Z. Zhou, M. Zhang, X. Li, F. Huang and P. J. Stang, A Suite of Tetraphenylethylene-Based Discrete Organoplatinum(II) Metallacycles: Controllable Structure and Stoichiometry, Aggregation-Induced Emission, and Nitroaromatics Sensing, J. Am. Chem. Soc., 2015, 137(48), 15276–15286 CrossRef CAS PubMed.
- A. Ayati, B. Tanhaei, F. F. Bamoharram, A. Ahmadpour, P. Maydannik and M. Sillanpää, Photocatalytic degradation of nitrobenzene by gold nanoparticles decorated polyoxometalate immobilized TiO2 nanotubes, Sep. Purif. Technol., 2016, 171, 62–68 CrossRef CAS.
- B. Thirumalraj, S. Palanisamy, S.-M. Chen, K. Thangavelu, P. Periakaruppan and X.-H. Liu, A simple electrochemical platform for detection of nitrobenzene in water samples using an alumina polished glassy carbon electrode, J. Colloid Interface Sci., 2016, 475, 154–160 CrossRef CAS PubMed.
- L. Zhai, Z.-X. Yang, W.-W. Zhang, J.-L. Zuo and X.-M. Ren, Dual-emission and thermochromic luminescence alkaline earth metal coordination polymers and their blend films with polyvinylidene fluoride for detecting nitrobenzene vapor, J. Mater. Chem. C, 2018, 6(26), 7030–7041 RSC.
- P. Yu, L. Chen, Y. Zhang, S. Zhao, Z. Chen, Y. Hu, J. Liu, Y. Yang, J. Shi, Z. Yao and W. Hong, Single-Molecule Tunneling Sensors for Nitrobenzene Explosives, Anal. Chem., 2022, 94(35), 12042–12050 CrossRef CAS PubMed.
- Z. Liu, W. Qi and G. Xu, Recent advances in electrochemiluminescence, Chem. Soc. Rev., 2015, 44(10), 3117–3142 RSC.
- M.-F. Lo, Z.-Q. Guan, T.-W. Ng, C.-Y. Chan and C.-S. Lee, Electronic Structures and Photoconversion Mechanism in Perovskite/Fullerene Heterojunctions, Adv. Funct. Mater., 2015, 25(8), 1213–1218 CrossRef CAS.
- G. Li, F. W. R. Rivarola, N. J. L. K. Davis, S. Bai, T. C. Jellicoe, F. de la Peña, S. Hou, C. Ducati, F. Gao, R. H. Friend, N. C. Greenham and Z.-K. Tan, Highly Efficient Perovskite Nanocrystal Light-Emitting Diodes Enabled by a Universal Crosslinking Method, Adv. Mater., 2016, 28(18), 3528–3534 CrossRef CAS PubMed.
- Q. Liao, K. Hu, H. Zhang, X. Wang, J. Yao and H. Fu, Perovskite Microdisk Microlasers Self-Assembled from Solution, Adv. Mater., 2015, 27(22), 3405–3410 CrossRef CAS PubMed.
- H. Zhang, Q. Liao, X. Wang, K. Hu, J. Yao and H. Fu, Controlled Substitution of Chlorine for Iodine in Single-Crystal Nanofibers of Mixed Perovskite MAPbI3-xClx, Small, 2016, 12(28), 3780–3787 CrossRef CAS PubMed.
- Y. Liu, X. Tang, T. Zhu, M. Deng, I. P. Ikechukwu, W. Huang, G. Yin, Y. Bai, D. Qu, X. Huang and F. Qiu, All-inorganic CsPbBr3 perovskite quantum dots as a photoluminescent probe for ultrasensitive Cu2+ detection, J. Mater. Chem. C, 2018, 6(17), 4793–4799 RSC.
- Z. Li, Q. Kang, L. Chen, B. Zhang, G. Zou and D. Shen, Enhancing aqueous stability and radiative-charge-transfer efficiency of CsPbBr3 perovskite nanocrystals via conductive silica gel coating, Electrochim. Acta, 2020, 330, 135332 CrossRef CAS.
- C. Zhao, Z. He, P. Wangyang, J. Tan, C. Shi, A. Pan, L. He and Y. Liu, Bidentate Ligand-Induced Oriented Transformation of CsPbBr3 Perovskite Nanocrystals into Nanowires for X-ray Photodetectors, ACS Appl. Nano Mater., 2022, 5(10), 13737–13744 CrossRef CAS.
- B. Zhang, L. Goldoni, J. Zito, Z. Dang, G. Almeida, F. Zaccaria, J. de Wit, I. Infante, L. De Trizio and L. Manna, Alkyl Phosphonic Acids Deliver CsPbBr3 Nanocrystals with High Photoluminescence Quantum Yield and Truncated Octahedron Shape, Chem. Mater., 2019, 31(21), 9140–9147 CrossRef CAS.
- L. Protesescu, S. Yakunin, M. I. Bodnarchuk, F. Krieg, R. Caputo, C. H. Hendon, R. X. Yang, A. Walsh and M. V. Kovalenko, Nanocrystals of Cesium Lead Halide Perovskites (CsPbX3, X = Cl, Br, and I): Novel Optoelectronic Materials Showing Bright Emission with Wide Color Gamut, Nano Lett., 2015, 15(6), 3692–3696 CrossRef CAS PubMed.
- L. Wu, M. Zhang, S. Yang, R. Wu, S. Gong, Q. Han and W. Wu, Spectral and dynamic analysis of CsPbBr3 perovskite nanocrystals with enhanced water stability using sodium passivation, J. Alloys Compd., 2021, 889, 161721 CrossRef.
- K. A. Fähnrich, M. Pravda and G. G. Guilbault, Recent applications of electrogenerated chemiluminescence in chemical analysis, Talanta, 2001, 54(4), 531–559 CrossRef PubMed.
- L. Li, Y. Chen and J.-J. Zhu, Recent Advances in Electrochemiluminescence Analysis, Anal. Chem., 2017, 89(1), 358–371 CrossRef CAS PubMed.
- L. Li, Z. Zhang, Y. Chen, Q. Xu, J.-R. Zhang, Z. Chen, Y. Chen and J.-J. Zhu, Sustainable and Self-Enhanced Electrochemiluminescent Ternary Suprastructures Derived from CsPbBr3 Perovskite Quantum Dots, Adv. Funct. Mater., 2019, 29(32), 1902533 CrossRef.
- Y. Huang, X. Long, D. Shen, G. Zou, B. Zhang and H. Wang, Hydrogen Peroxide Involved Anodic Charge Transfer and Electrochemiluminescence of All-Inorganic Halide Perovskite CsPbBr3 Nanocrystals in an Aqueous Medium, Inorg. Chem., 2017, 56(17), 10135–10138 CrossRef CAS PubMed.
- J. Ballesta-Claver, R. Rodríguez-Gómez and L. F. Capitán-Vallvey, Disposable biosensor based on cathodic electrochemiluminescence of tris(2,2-bipyridine)ruthenium(II) for uric acid determination, Anal. Chim. Acta, 2013, 770, 153–160 CrossRef CAS PubMed.
- Q. Jun-e, C. Geng, W. Hai-ren and C. Zhi-yong, The protection of TDPA deposited from mixed solutions with different water/ethanol ratios for aluminum alloy against corrosion in NaCl solution, Anti-Corros. Methods Mater., 2017, 64(2), 233–240 CrossRef.
- H. Asbaghian-Namin, P. Karami, H. Naghshara, D. Gholamin and M. Johari-Ahar, Electrochemiluminescent immunoassay for the determination of CA15-3 and CA72-4 using graphene oxide nanocomposite modified with CdSe quantum dots and Ru(bpy)3 complex, Microchim. Acta, 2021, 188(7), 238 CrossRef CAS PubMed.
- X. Wang, H. Liu, H. Qi, Q. Gao and C. Zhang, Highly efficient electrochemiluminescence of ruthenium complex-functionalized CdS quantum dots and their analytical application, J. Mater. Chem. B, 2020, 8(16), 3598–3605 RSC.
- L. Fu, X. Gao, S. Dong, J. Jia, Y. Xu, D. Wang and G. Zou, Coreactant-Free and Direct Electrochemiluminescence from Dual-Stabilizer-Capped InP/ZnS Nanocrystals: A New Route Involving n-Type Luminophore, Anal. Chem., 2022, 94(2), 1350–1356 CrossRef CAS PubMed.
- L. Chen, X. Zeng, P. Si, Y. Chen, Y. Chi, D.-H. Kim and G. Chen, Gold Nanoparticle-Graphite-Like C3N4 Nanosheet Nanohybrids Used for Electrochemiluminescent Immunosensor, Anal. Chem., 2014, 86(9), 4188–4195 CrossRef CAS PubMed.
- H. Gao, S. Liu, Z. Xue, W. Liu, Y. Nie, G. Chen and X. Li, Synthesis and photoluminescence properties of CsPbBr3 quantum dots by using para-xylene as the anti-solvent, J. Lumin., 2019, 215, 116584 CrossRef CAS.
- T. Xuan, X. Yang, S. Lou, J. Huang, Y. Liu, J. Yu, H. Li, K.-L. Wong, C. Wang and J. Wang, Highly stable CsPbBr3 quantum dots coated with alkyl phosphate for white light-emitting diodes, Nanoscale, 2017, 9(40), 15286–15290 RSC.
- C. Sun, Y. Zhang, C. Ruan, C. Yin, X. Wang, Y. Wang and W. W. Yu, Efficient and Stable White LEDs with Silica-Coated Inorganic Perovskite Quantum Dots, Adv. Mater., 2016, 28(45), 10088–10094 CrossRef CAS PubMed.
- F. Wang, H. Wang, A. Ali, Y. Zhang, X. Cui and Y. Liu, In-situ one-step electrospray fabrication of polyvinylidene fluoride encapsulated CsPbBr3 spheres with high stability and cell imaging application, Inorg. Chem. Commun., 2019, 106, 99–103 CrossRef CAS.
- J.-E. Qu, G. Chen, H.-R. Wang and D.-J. Nie, Effect of water content on corrosion inhibition behavior of self-assembled TDPA on aluminum alloy surface, Trans. Nonferrous Met. Soc. China, 2013, 23(10), 3137–3144 CrossRef CAS.
- H.-D. Nguyen, C. C. Lin and R.-S. Liu, Waterproof Alkyl Phosphate Coated Fluoride Phosphors for Optoelectronic Materials, Angew. Chem., Int. Ed., 2015, 54(37), 10862–10866 CrossRef CAS PubMed.
- J. Han, M. Sharipov, S. Hwang, Y. Lee, B. T. Huy and Y.-I. Lee, Water-stable perovskite-loaded nanogels containing antioxidant property for highly sensitive and selective detection of roxithromycin in animal-derived food products, Sci. Rep., 2022, 12(1), 3147 CrossRef CAS PubMed.
- H. Dai, X. Wu, Y. Wang, W. Zhou and G. Chen, An electrochemiluminescent biosensor for vitamin C based on inhibition of luminol electrochemiluminescence on graphite/poly(methylmethacrylate) composite electrode, Electrochim. Acta, 2008, 53(16), 5113–5117 CrossRef CAS.
- K. Hills-Kimball, H. Yang, T. Cai, J. Wang and O. Chen, Recent Advances in Ligand Design and Engineering in Lead Halide Perovskite Nanocrystals, Adv. Sci., 2021, 8(12), 2100214 CrossRef CAS PubMed.
- A. Ioakeimidis and S. A. Choulis, Nitrobenzene as Additive to Improve Reproducibility and Degradation Resistance of Highly Efficient Methylammonium-Free Inverted Perovskite Solar Cells, Materials, 2020, 13(15), 3289 CrossRef CAS PubMed.
- J. Xue, R. Wang, X. Chen, C. Yao, X. Jin, K.-L. Wang, W. Huang, T. Huang, Y. Zhao, Y. Zhai, D. Meng, S. Tan, R. Liu, Z.-K. Wang, C. Zhu, K. Zhu, M. C. Beard, Y. Yan and Y. Yang, Reconfiguring the band-edge states of photovoltaic perovskites by conjugated organic cations, Science, 2021, 371(6529), 636–640 CrossRef CAS PubMed.
- D. Ma, B. Li, X. Zhou, Q. Zhou, K. Liu, G. Zeng, G. Li, Z. Shi and S. Feng, A dual functional MOF as a luminescent sensor for quantitatively detecting the concentration of nitrobenzene and temperature, Chem. Commun., 2013, 49(79), 8964–8966 RSC.
- X.-M. Cao, N. Wei, L. Liu, L. Li and Z.-B. Han, Luminescent lanthanide-organic polyrotaxane framework as a turn-off sensor for nitrobenzene and Fe3+, RSC Adv., 2016, 6(23), 19459–19462 RSC.
- Z. Sun, J. Sun, L. Xi, J. Xie, X. Wang, Y. Ma and L. Li, Two Novel Lanthanide Metal-Organic Frameworks: Selective Luminescent Sensing for Nitrobenzene, Cu2+, and MnO4−, Cryst. Growth Des., 2020, 20(8), 5225–5234 CrossRef CAS.
- S. An, N. Shang, J. Zhang, A. Nsabimana, M. Su, S. Zhang and Y. Zhang, Fabrication of electrocatalytically active, cobalt-embedded nitrogen-doped ordered macroporous carbon for sensitive detection of nitrobenzene, Colloids Surf., A, 2022, 653, 130078 CrossRef CAS.
- C. Pandiyarajan, P. Rameshkumar, S. Murugesan and M. Selvaraj, Silver nanoparticles-supported graphitic-like carbon nitride for the electrochemical sensing of nitrobenzene and its derivatives, J. Mater. Sci.: Mater. Electron., 2021, 32(14), 19912–19924 CrossRef CAS.
|
This journal is © The Royal Society of Chemistry 2023 |