DOI:
10.1039/D2SD00220E
(Paper)
Sens. Diagn., 2023,
2, 438-444
Towards miniaturized gas sensors based on substrate-integrated hollow waveguides and interband cascade light emitting diodes
Received
12th December 2022
, Accepted 2nd February 2023
First published on 13th February 2023
Abstract
In this study, we report the first combination of mid-infrared (MIR) interband cascade light emitting diodes (ICLEDs) with a central emission wavelength at 4.0 μm and 3.4 μm, respectively, combined with a substrate-integrated hollow waveguide (iHWG) for the detection of CO2 and CH4 analyzing the antisymmetric stretching of CO2 at 2345 cm−1, and the CH stretching rovibrational mode of CH4 at 3020 cm−1. The obtained calibration functions in the concentration range from 1000 to 10
000 ppmV enabled deriving detection limits in the range of 386–407 ppmV for CO2, and of 302–338 ppmV for CH4. The combination of iHWG and ICLED technology is a pioneering step toward a new generation of miniaturized and low-cost mid-infrared optical sensing devices for rapid gas diagnostics and exceedingly small sample volumes.
Introduction
The need for low-cost yet bright light sources in the infrared spectral range from 2 to 20 μm has been growing in recent years. In particular, the 3–6 μm regime is of great importance for the detection of gaseous molecules in environmental analysis, process monitoring and even in biomedical diagnostics. In this spectral window, many relevant gases including but not limited to NH3 (2.1 μm), CH4 (2.35 μm and 3.3 μm), HCl (3.5 μm), N2O (3.9 μm and 4.5 μm), SO2 (4 μm), CO2 (4.25 μm), and CO (2.3 μm and 4.6 μm) have molecule-specific vibrational, rovibrational, and rotational transitions.1
Infrared spectroscopy with its inherent molecular selectivity is ideally suited for the selective, sensitive and rapid detection and/or monitoring of molecular constituents, and represents an effective alternative to conventionally used methods such as e.g., gas chromatography coupled with mass spectrometry or other complex detection schemes. Furthermore, given recent developments in IR waveguide, light source, and detector technology,2–4 infrared spectroscopy shows unrivaled potential for molecular sensor miniaturization serving as a versatile and modular analytical tool.
Nowadays, the specific molecular vibrational, rovibrational, and rotational transitions in the MIR regime in a sensing format are typically excited by narrowband light sources such as quantum-cascade lasers (QCLs). QCLs were first experimentally described in 19945 and are based on intersubband transitions between constructed conduction band states in complex heterostructures instead of electron–hole recombination in a semiconductor bandgap.6,7 These electron transitions lead to efficient photon emission in the MIR range enabling a wide variety of spectroscopic and sensing applications.8–11 These are mostly built on InGaAs/InAlAs material systems, which however are limited to wavelengths λ > 5 μm due to conduction band offset. New QCL material systems have been researched,12–15 but they are less advanced in both fabrication and processing than the common InGaAs/InAlAs materials, and thus QCLs using such new materials are not yet well-developed.
An alternative to QCLs is provided by interband cascade lasers (ICLs), which were proposed and realized at about the same time as QCLs.16,17 ICLs are based on electron–hole recombination of type-II interfaces, where, for example, electrons of the InAs conduction band recombine with the holes of the InGaSb valence band. With this design, it is possible to operate ICLs in continuous wave (cw) mode at room temperature, at exceedingly low required electrical power and within a spectral wavelength range of 3–7 μm.
However, ICLs are not cost-efficient due to their design complexity, and are therefore of limited suitability for low-cost sensing applications. LED-like emitters are a cost-efficient alternative to lasers given their small dimensions, long-term reliability and broadband, incoherent radiation emission characteristics. Interband cascade light diodes (ICLEDs) – similar to ICLs – use electron–hole recombination in type-II structures as their core radiation emission mechanism, however, do not have an upper and lower optical cladding layer. Owing to recent developments, ICLEDs can also be operated at room temperature in cw mode, are characterized by a very low electrical power consumption, and provide output powers in some cases >1 mW in the 3–8 μm range.18–24
In addition to the light source, a compact combination with an appropriate waveguide and an efficient gas cell is required for the development of miniaturized gas sensors. Griffiths et al.25 first reported an applied combination of waveguide and gas cell in the form of a so-called hollow waveguide (HWG) in the 1980s. An innovative alternative providing the combination of an efficient photonic waveguide and a highly miniaturized gas cell was pioneered by Mizaikoff and colleagues.26 So-called substrate-integrated hollow waveguides (iHWGs) integrate photon guidance and gas cell, and enable analyzing exceedingly small gas sample volumes with excellent signal-to-noise ratio.26–28
The scope of the present study was the demonstration of the first ICLED-iHWG combination, which provides a perspective on a potentially miniaturized, cost-effective, small-volume gas sensor for carbon dioxide (CO2) and methane (CH4) based on ICLED light sources.
Material and methods
Experimental setup
The optical setup for gas sample flow-through measurements is illustrated schematically in Fig. 1. The system comprises a thermoelectrically cooled ICLED emitting at a central wavelength of 4.0 μm (2500 cm−1) or 3.4 μm (2941 cm−1), respectively (fabricated by nanoplus Nanosystems and Technologies GmbH, Gerbrunn, Germany) mounted on a TO66 socket (TO66 Heatsink Cube, nanoplus Nanosystems and Technologies GmbH, Germany). For convenience, a Fourier transform infrared spectrometer (FTIR) was used for signal read-out and for spectrally analyzing the radiation after passing through the iHWG focused onto an internal mercury cadmium telluride (MCT) detector cooled with liquid nitrogen (N2). Once the signal characteristics are determined, a dedicated detector matching the footprint of the ICLED-iHWG assembly will be implemented in lieu of the FTIR.
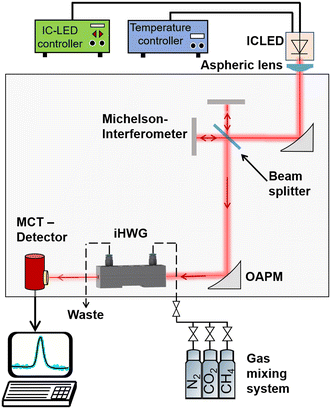 |
| Fig. 1 Schematic of the sensor setup (ICLED: interband cascade laser light emitting diode; aspheric lens; OAPM: off-axis parabolic mirror; iHWG: integrated hollow waveguide; MCT-detector: mercury cadmium telluride detector). | |
IR radiation emitted by the ICLED (red line) was coupled into the interferometer of the FTIR spectrometer (Vertex 70, Bruker Optic GmbH, Ettlingen, Germany) and subsequently coupled into the iHWG located inside the sample chamber of the FTIR system. After propagating through the iHWG, MIR radiation at the distal end (orange line) was focused onto an MCT detector (InfraRed Associates Inc., Stuart, FL, USA) with an active detector element area of 4 mm2 matching the iHWG channel dimensions.
The ICLEDs used in this study had a nine-stage structure grown via molecular beam epitaxy on low-doped GaSb with a 640 μm squared mesa. The flip-chip mounted device can reach a maximum cw output power up to 5.1 mW at room temperature with a driving current of 600 mA. Further information and characterization of the ICLED can be found elsewhere.29 To operate the ICLED, the chip was mounted onto a compact (5 × 4.8 × 5 cm3, L × W × H) heat sink, which allows thermoelectric cooling. For driving current and temperature control, a laser diode controller (TLD001, Thorlabs GmbH, Dachau/Munich, Germany) was used together with a TEC controller (TTC001, Thorlabs GmbH, Dachau/Munich, Germany) to ensure stable driving current and temperature, respectively. The ICLED was operated with a driving current of 200 mA and set to an operating temperature of 25 °C for all measurements.
Measurements procedure
For the gas measurements, CO2 (1.00% carbon dioxide in N2, Westfalen AG, Münster, Germany) and CH4 (1.00% methane in N2, Westfalen AG, Münster, Germany) were used. To produce gas mixtures, a customized gas mixing system (GMS) based on mass flow controllers was used, which was developed in collaboration with Lawrence Livermore National Laboratory (LLNL; Livermore/CA, USA).
Before starting the IR experiments, the iHWG gas flow-through cell was purged for three minutes using N2 gas (99.999% N2, MTI IndustrieGase AG, Neu-Ulm, Germany) at a flow rate of 200 mL min−1. After purging, the IR background spectra was recorded. In the next step, a defined gas mixture of N2 and CO2 or CH4 for the respective ICLED was set at the gas mixing system and the flow-through cell was flushed with the specified sample concentration at a flow rate of 200 mL min−1 for three minutes. In the conducted experiments the CO2 and CH4 concentrations ranged from 1000 to 10
000 ppmV. In the following, three repeated sample measurements were initiated. After recording the IR spectra of the set analyte concentration, the GMS was turned to N2 to restart the purging step, and then the measurement step was repeated with the next higher concentration resulting in a total of 30 data points per measurement series. Three independent series of measurements were performed to verify and reproduce the data.
IR data acquisition and evaluation
For the IR data acquisition and processing the OPUS 8.1 (Bruker Optik GmbH, Ettlingen, Germany) software package was used recording CO2 and CH4 in the spectral range of 3000 to 2000 cm−1 and 4000 to 2600 cm−1, respectively (spectral res. 2 cm−1) using a Blackman–Harris three-term apodization function and averaging 256 scans. Baseline correction was performed in the region 2385–2280 cm−1 for CO2 and 3025–3005 cm−1 for CH4 using the Essential eFTIR spectroscopy software toolbox (Essential FTIR, Madison WI, USA).
The suitability of ICLEDs for the quantification of relevant gases was evaluated by establishing calibration functions, and calculating the limit of detection (LOD), and limit of quantification (LOQ = 3 × LOD) for CO2 and CH4. To determine the LOD and LOQ, the peak areas of the antisymmetric stretching mode of CO2 at 2345 cm−1, and the area of the CH stretching rovibrational mode of CH4 at 3020 cm−1 were integrated using the Essential eFTIR spectroscopy software.
Subsequently, a linear correlation between the integrated peak area and the concentration was established, and the confidence and the prediction interval were calculated according to the approach of Hubaux and Vos.30
Substrate-integrated hollow waveguide
Fig. 2 shows the cross-sectional view of the iHWG (75 × 25 × 20 mm, L × W × H) used in this study. The iHWG was fabricated from aluminum substrates and provides an optical path length of 70 mm with a hollow channel cross-section of 4 × 4 mm2. The cover substrate on top has two threaded ports that serve as gas inlets and outlets into the radiation and gas propagation channel. To achieve high surface reflectance, the iHWG surfaces were polished to a mirror-like finish using commercially available diamond polishing suspensions. The three individual parts of the iHWG were sealed together and bonded with epoxy to ensure a gas-tight interface. Finally, to obtain a miniaturized gas cell, both ends were sealed gas-tight with MIR-transparent BaF2 windows and epoxy, thus creating a highly miniaturized gas cell with a volume of 1120 μL. Details on iHWG technology can be found elsewhere.31–34
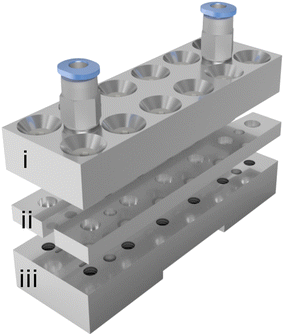 |
| Fig. 2 Cross-sectional view of the iHWG with (i) the top substrate with pneumatic hose for the gas in- and outlet, (ii) middle substrate including the gas and light propagation channel and (iii) the bottom substrate. | |
Results and discussion
Verifying a suitable spectral overlap between the spectral emission of the ICLEDs and the characteristic absorption bands of CO2 and CH4, the emission spectra of the ICLEDs at 200 mA driving current were recorded using the FTIR spectrometer. The obtained spectra were smoothed with the Savitzky–Golay filter for better illustration, and then compared with the FTIR-recorded spectra of CO2 and CH4 using an iHWG. In Fig. 3 the IR spectra of the two ICLEDs radiation spectra (black) are superimposed onto the CO2 and the CH4 spectrum, respectively (red) clearly indicating the suitability of the ICLED emission for sensing CO2 or CH4.
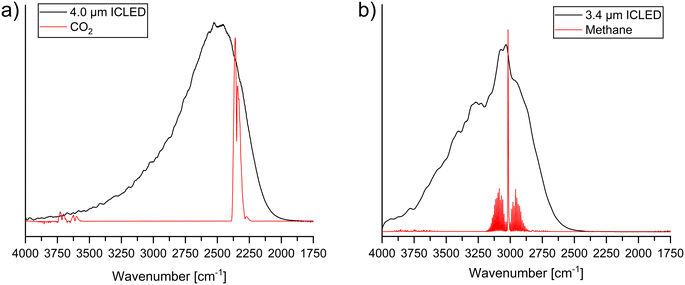 |
| Fig. 3 ICLED spectral emission (black) superimposed with a) FTIR-recorded CO2 absorption spectrum (red) and the 4.0 μm central wavelength ICLED (black) and b) FTIR-recorded CH4 absorption spectrum (red) and the 3.4 μm central wavelength ICLED (black). The ICLED spectral emission spectrum was likewise analyzed via the FTIR and smoothed with the Savitzky–Golay filter for better illustration. | |
Fig. 4 illustrates the integrated peak area of the antisymmetric stretching mode at 2345 cm−1 of CO2 and the CH stretching rovibrational mode of CH4 at 3020 cm−1vs. the analyzed concentrations, respectively. The three independent measurement series for each analyte followed a linear trend with an R2 value of >0.99 confirming the suitability of the established calibration model for quantitative analysis. To estimate the achievable LOD at the previously mentioned conditions, the suitability of ICLEDs was evaluated based on the calculated calibration functions. The resulting LODs were in the range of 386–407 ppmV for CO2, and of 302–338 ppmV for CH4. In addition, the obtained measurements were highly reproducible allowing the detection of small CO2 and CH4 sample volumes via the combination of ICLEDs with iHWGs.
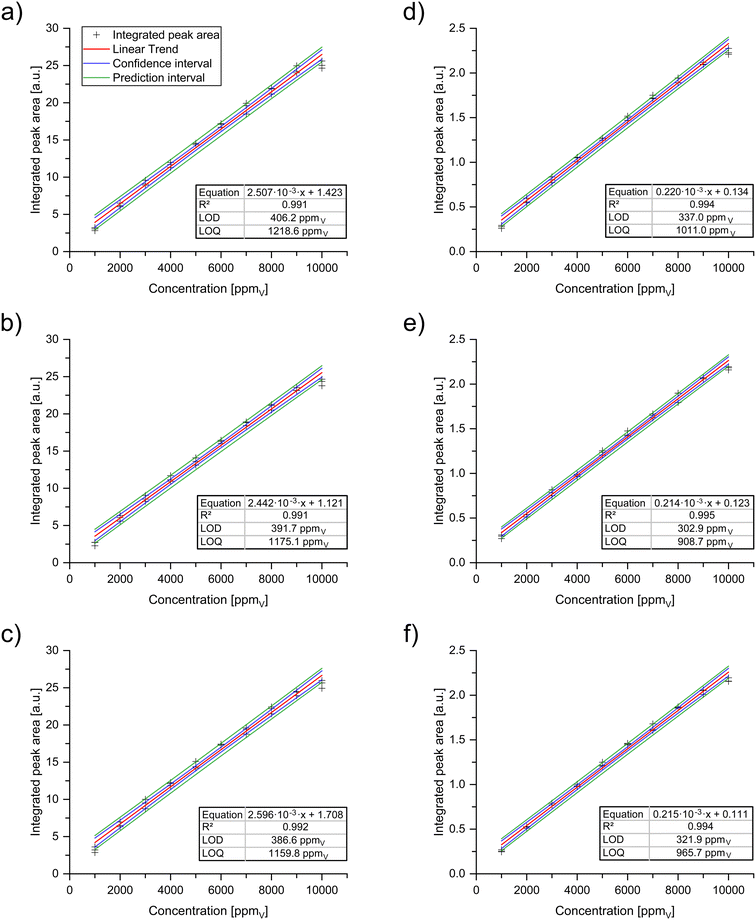 |
| Fig. 4 Comparison of three separately prepared concentration series for CO2 – a)–c) and CH4 – d)–f) – respectively evaluated using the asymmetric stretching mode of CO2 at 2345 cm−1 and the CH stretching rovibrational mode of CH4 at 3020 cm−1. Based on the integrated peak areas, linear trend (red), confidence interval (blue), prediction interval (green) and LOD as well as LOQ were calculated. | |
To further characterize the performance of the sensor concept and the potential influence of stochastic and electronic noise, the signal-to-noise ratio (SNR) for the respective CO2 and CH4 measurements at 1000 ppmV was derived.
Table 1 summarizes the SNRs for CO2 – (a), (b), and (c) shown in Fig. 4, and for CH4 – (d), (e), (f). SNRs of 11.6 to 14.2 for CO2 and 19.0 to 22.0 for CH4 were achieved, which is satisfactory considering that only a small portion of the IR light emitted by the ICLED led to the respective molecular transitions. The difference in SNR between the 4.0 μm ICLED and the 3.4 μm ICLED can be explained by the fact that CH4 absorbs at the central emission wavelength of the 3.4 μm ICLED corresponding to the peak output power, while the CO2 absorption is not located at the central emission wavelength of the 4.0 μm ICLED.
Table 1 Summary of SNR for CO2 and CH4 at 1000 ppmV derived from the data shown in Fig. 4
|
SNR
|
CO2 |
|
(a) |
14.2 |
(b) |
11.6 |
(c) |
13.0 |
CH4 |
|
(d) |
22.0 |
(e) |
19.6 |
(f) |
19.0 |
Comparing our results with recently published miniaturized gas sensor prototypes for CO2 and CH4 detection based on MIR LEDs,35–37 it was determined that the performance of the developed prototypes is at the same order of magnitude compared to the other CO2 MIR LED sensor,35 and CH4 MIR LED sensors.36,37 Last but not least, the performance of the ICLEDs operated in the present study in cw mode was comparable to MIR LEDs operated in previous studies using modulation techniques.
Conclusions
In this study, we demonstrated the first combination of two individual cw ICLEDs emitting at different central IR wavelengths as a low-cost and easy to operate MIR light source coupled with iHWGs serving simultaneously as waveguides and miniaturized gas cells for analyzing minute sample volumes of CO2 and CH4. The quantitative analysis of both gases showed a robust sensor behaviour with reproducible spectra for each analyte. Based on this analysis, the development of possibly integrated iHWG-ICLED-based sensors appears perfectly suitable for e.g., sensing CO2 concentrations in closed and open spaces, as well as the monitoring of the lower explosion limit of CH4 in air with a performance on par with previously published MIR LED-based sensor types.
Future work will address further miniaturization of the optical setup by hybrid integration of the ICLED reflector and lenses together with the iHWG.
Already at this stage of development, the iHWG-ICLED combination has demonstrated that this type of sensor is suitable for the detection of relatively low concentrations of relevant gas phase constituents in the ppmV range in minute sample volumes (i.e., few hundreds of microliters). The modularity, flexibility and adaptability of the iHWG-ICLED combination can contribute significantly to the development of miniaturized and cost-effective gas sensors for a wide range of application scenarios in environmental analysis, process monitoring, and even biomedical diagnostics.
Author contributions
M. H. and N. H. conceived and designed the experiments; M. H. and N. H. performed the experiments; M. H. and N. H. analysed the data; M. H. wrote the article; B. M. proofread the article. All authors have read and agreed to the published version of the manuscript.
Conflicts of interest
There are no competing interests to declare.
Acknowledgements
The authors want to acknowledge valuable support by their colleagues at the Institute of Analytical and Bioanalytical Chemistry (IABC) at Ulm University. The Machine Shop at Ulm University is thanked for support during prototype development. This work was in part funded by the DFG Graduiertenkolleg (GRK) 2203 (PULMOSENS) and has also received partial funding by the European Union's Horizon 2020 research and innovation program under grant agreement No. 101016444.
Notes and references
- I. E. Gordon, L. S. Rothman, C. Hill, R. V. Kochanov, Y. Tan and P. F. Bernath,
et al., The HITRAN2016 molecular spectroscopic database, J. Quant. Spectrosc. Radiat. Transfer, 2017, 203, 3–69 CrossRef CAS , Available from: https://linkinghub.elsevier.com/retrieve/pii/S0022407317301073.
- J. Haas and B. Mizaikoff, Advances in Mid-Infrared Spectroscopy for Chemical Analysis, Annu. Rev. Anal. Chem., 2016, 9(1), 45–68 CrossRef PubMed.
- R. Selvaraj, N. J. Vasa, S. M. S. Nagendra and B. Mizaikoff, Advances in Mid-Infrared Spectroscopy-Based Sensing Techniques for Exhaled Breath Diagnostics, Molecules, 2020, 25(9), 2227 CrossRef CAS PubMed.
- J. Haas, M. Pleyer, J. Nauschütz, J. Koeth, M. Nägele and O. Bibikova,
et al., iBEAM: substrate-integrated hollow waveguides for efficient laser beam combining, Opt. Express, 2019, 27(16), 23059 CrossRef CAS PubMed , Available from: https://www.osapublishing.org/abstract.cfm?URI=oe-27-16-23059.
- J. Faist, F. Capasso, D. L. Sivco, C. Sirtori, A. L. Hutchinson and A. Y. Cho, Quantum Cascade Laser, Science, 1994, 264(5158), 553–566, DOI:10.1126/science.264.5158.553.
- R. Köhler, A. Tredicucci, F. Beltram, H. E. Beere, E. H. Linfield and A. G. Davies,
et al., Terahertz semiconductor-heterostructure laser, Nature, 2002, 417(6885), 156–159 CrossRef PubMed , Available from: https://www.nature.com/articles/417156a.
- B. S. Williams, Terahertz quantum-cascade lasers, Nat. Photonics, 2007, 1(9), 517–525 CrossRef CAS , Available from: https://www.nature.com/articles/nphoton.2007.166.
- M. Sieger, J. Haas, M. Jetter, P. Michler, M. Godejohann and B. Mizaikoff, Mid-Infrared Spectroscopy Platform Based on GaAs/AlGaAs Thin-Film Waveguides and Quantum Cascade Lasers, Anal. Chem., 2016, 88(5), 2558–2562, DOI:10.1021/acs.analchem.5b04144.
- J. Haas, R. Stach, M. Sieger, Z. Gashi, M. Godejohann and B. Mizaikoff, Sensing chlorinated hydrocarbons via miniaturized GaAs/AlGaAs thin-film waveguide flow cells coupled to quantum cascade lasers, Anal. Methods, 2016, 8(36), 6602–6606, 10.1039/C6AY01450J.
- C. Charlton, F. de Melas, A. Inberg, N. Croitoru and B. Mizaikoff, Hollow-waveguide gas sensing with room-temperature quantum cascade lasers, IEE Proc.: Optoelectron., 2003, 150(4), 306 CrossRef CAS.
- K. Wörle, F. Seichter, A. Wilk, C. Armacost, T. Day and M. Godejohann,
et al., Breath Analysis with Broadly Tunable Quantum Cascade Lasers, Anal. Chem., 2013, 85(5), 2697–2702 CrossRef PubMed.
- M. P. Semtsiv, M. Ziegler, S. Dressler, W. T. Masselink, N. Georgiev and T. Dekorsy,
et al., Above room temperature operation of short wavelength (λ=3.8μm) strain-compensated In0.73Ga0.27As–AlAs quantum-cascade lasers, Appl. Phys. Lett., 2004, 85(9), 1478–1480, DOI:10.1063/1.1789246.
- N. Bandyopadhyay, S. Slivken, Y. Bai and M. Razeghi, High power, continuous wave, room temperature operation of λ ∼ 3.4 μm and λ ∼ 3.55 μm InP-based quantum cascade lasers, Appl. Phys. Lett., 2012, 100(21), 212104, DOI:10.1063/1.4719110.
- D. G. Revin, J. W. Cockburn, M. J. Steer, R. J. Airey, M. Hopkinson and A. B. Krysa,
et al., InGaAs/AlAsSb/InP quantum cascade lasers operating at wavelengths close to 3μm, Appl. Phys. Lett., 2007, 90(2), 021108, DOI:10.1063/1.2431035.
- H. Machhadani, P. Kandaswamy, S. Sakr, A. Vardi, A. Wirtmüller and L. Nevou,
et al., GaN/AIGaN
intersubband optoelectronic devices, New J. Phys., 2009, 11 Search PubMed.
- R. Q. Yang, Infrared laser based on intersubband transitions in quantum wells, Superlattices Microstruct., 1995, 17(1), 77–83 CrossRef CAS , Available from: https://linkinghub.elsevier.com/retrieve/pii/S0749603685710178.
- R. Q. Yang, J. D. Bruno, J. L. Bradshaw, J. T. Pham and D. E. Wortman, Interband cascade lasers: progress and challenges, Phys. E, 2000, 7(1–2), 69–75 CrossRef CAS , Available from: https://linkinghub.elsevier.com/retrieve/pii/S1386947799002805.
- A. Joullié and P. Christol, GaSb-based mid-infrared 2–5 μm laser diodes, C. R. Phys., 2003, 4(6), 621–637 CrossRef.
- R. Q. Yang, C. H. Lin, S. J. Murry, S. S. Pei, H. C. Liu and M. Buchanan,
et al., Interband cascade light emitting diodes in the 5–8 μm spectrum region, Appl. Phys. Lett., 1997, 70(15), 2013–2015, DOI:10.1063/1.118806.
- N. C. Das, K. Olver, F. Towner, G. Simonis and H. Shen, Infrared (3.8μm) interband cascade light-emitting diode array with record high efficiency, Appl. Phys. Lett., 2005, 87(4), 041105 CrossRef.
- S. Suchalkin, S. Jung, G. Kipshidze, L. Shterengas, T. Hosoda and D. Westerfeld,
et al., GaSb based light emitting diodes with strained InGaAsSb type I quantum well active regions, Appl. Phys. Lett., 2008, 93(8), 081107 CrossRef.
- C. S. Kim, W. W. Bewley, C. D. Merritt, C. L. Canedy, M. V. Warren and I. Vurgaftman,
et al., Improved mid-infrared interband cascade light-emitting devices, Opt. Eng., 2017, 57(01), 1 Search PubMed.
-
C. D. Merritt, C. S. Kim, M. Kim, C. L. Canedy, W. W. Bewley and M. V. Warren, et al., Effects of ion bombardment on interband cascade laser structures, in Quantum Sensing and Nano Electronics and Photonics XVII, ed. M. Razeghi, J. S. Lewis, G. A. Khodaparast and P. Khalili, SPIE, 2020, p. 61 Search PubMed.
- C. L. Canedy, W. W. Bewley, S. Tomasulo, C. S. Kim, C. D. Merritt and I. Vurgaftman,
et al., Mid-infrared interband cascade light emitting devices grown on off-axis silicon substrates, Opt. Express, 2021, 29(22), 35426 CrossRef CAS PubMed.
-
P. R. Griffiths and J. A. de Haseth, Fourier Transform Infrared Spectrometry, Monitoring Atmospheric Water Vapour, John Wiley & Sons, Inc., Hoboken, NJ, USA, 2007, vol. 10, pp. 95–111 Search PubMed.
- A. Wilk, J. C. Carter, M. Chrisp, A. M. Manuel, P. Mirkarimi and J. B. Alameda,
et al., Substrate-Integrated Hollow Waveguides: A New Level of Integration in Mid-Infrared Gas Sensing, Anal. Chem., 2013, 85(23), 11205–11210 CrossRef CAS PubMed.
- D. Perez-Guaita, V. Kokoric, A. Wilk, S. Garrigues and B. Mizaikoff, Towards the determination of isoprene in human breath using substrate-integrated hollow waveguide mid-infrared sensors, J. Breath Res., 2014, 8(2), 026003 CrossRef CAS PubMed.
- J. Flávio da Silveira Petruci, P. R. Fortes, V. Kokoric, A. Wilk, I. M. Raimundo and A. A. Cardoso,
et al., Monitoring of hydrogen sulfide via substrate-integrated hollow waveguide mid-infrared sensors in real-time, Analyst, 2014, 139(1), 198–203 RSC.
- N. Schäfer, J. Scheuermann, R. Weih, J. Koeth and S. Höfling, High efficiency mid-infrared interband cascade LEDs grown on low absorbing substrates emitting >5 mW of output power, Opt. Eng., 2019, 58(11), 1 Search PubMed , Available from: https://www.spiedigitallibrary.org/journals/optical-engineering/volume-58/issue-11/117106/High-efficiency-mid-infrared-interband-cascade-LEDs-grown-on-low/10.1117/1.OE.58.11.117106.full.
- A. Hubaux and G. Vos, Decision and detection limits for calibration curves, Anal. Chem., 1970, 42(8), 849–855, DOI:10.1021/ac60290a013.
- P. R. Fortes, J. F. da Silveira Petruci, A. Wilk, A. A. Cardoso, I. M. Raimundo and B. Mizaikoff, Optimized design of substrate-integrated hollow waveguides for mid-infrared gas analyzers, J. Opt., 2014, 16(9), 094006 CrossRef CAS.
- B. Mizaikoff, Waveguide-enhanced mid-infrared chem/bio sensors, Chem. Soc. Rev., 2013, 42(22), 8683–8699 RSC.
- E. Tütüncü, V. Kokoric, A. Wilk, F. Seichter, M. Schmid and W. E. Hunt,
et al., Fiber-Coupled Substrate-Integrated Hollow Waveguides: An Innovative Approach to Mid-infrared Remote Gas Sensors, ACS Sens., 2017, 2(9), 1287–1293 CrossRef PubMed.
- R. Stach, J. Haas, E. Tütüncü, S. Daboss, C. Kranz and B. Mizaikoff, polyHWG: 3D Printed Substrate-Integrated Hollow Waveguides for Mid-Infrared Gas Sensing, ACS Sens., 2017, 2(11), 1700–1705 CrossRef CAS PubMed.
- L. Scholz, A. Ortiz Perez, B. Bierer, P. Eaksen, J. Wöllenstein and S. Palzer, Miniature low-cost carbon dioxide sensor for mobile devices, IEEE Sens. J., 2017, 17(9), 2889–2895 CAS.
- V. Wittstock, L. Scholz, B. Bierer, A. O. Perez, J. Wöllenstein and S. Palzer, Design of a LED-based sensor for monitoring the lower explosion limit of methane, Sens. Actuators, B, 2017, 247, 930–939 CrossRef CAS.
- S. H. Huang, Y. J. Huang and H. C. Chui, Trace methane sensor using mid-infrared light emitting diode in hollow-core fiber, Sens. Actuators, B, 2019, 282(May 2018), 599–602 CrossRef CAS.
|
This journal is © The Royal Society of Chemistry 2023 |