DOI:
10.1039/D2SD00156J
(Critical Review)
Sens. Diagn., 2023,
2, 46-77
Endocrine disrupting chemicals in water and recent advances on their detection using electrochemical biosensors
Received
31st August 2022
, Accepted 26th October 2022
First published on 6th December 2022
Abstract
The ever increasing anthropogenic activities have been producing an undesired group of substances called endocrine disrupting chemicals (EDCs), which can pose a serious threat to the health of human beings and wildlife once they are released into natural water environments. Herein, recent advances on the use of electrochemical biosensors for the determination of EDCs especially in water are thoroughly summarized. Initially, different categories of EDCs with important guidelines are briefly introduced, followed by a simple discussion of non-electrochemical detection methods. Electrochemical techniques including theoretical background and reported studies are then evaluated as the highlight of the review. Finally, current issues, technical bottlenecks, and prospects of this field are critically discussed. This review is composed of following subsections: (i) systematical classification of common EDCs with their toxicities towards human beings and animals, (ii) water contamination events, safety guidelines, and legislations, (iii) non-electrochemical methods for detection of EDCs, (iv) electrochemical monitoring systems, (v) receptors for biorecognition, (vi) detection of EDCs using electrochemical biosensors, and (vii) recent progress, issues, and further development. This review, with a strong interdisciplinary nature, across nanotechnology, biology, material science, and electrochemistry, can offer comprehensive academic assistance for future studies on the detection of EDCs using electrochemical biosensors.
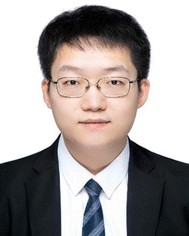 Lue Wang | Dr Lue Wang is currently doing postdoctoral research at the Department of Pharmaceutical, Tsinghua University, China. His research has been mainly focused on graphene or other 2D material-based biosensors for environmental monitoring and medical applications. He received his BSc (2015) in Pharmaceutical Engineering from Fuzhou University, China, and both his MSc (2016) in Chemical Engineering and PhD (2021) in Nanotechnology from Swansea University, UK. After the doctoral study at Swansea University, he joined the Department of Pharmaceutical, Tsinghua University in 2022. |
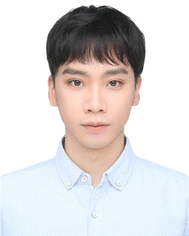 Haoyun Wang | Haoyun Wang is currently a PhD candidate at the Institute of Integrated Micro and Nano Systems, University of Edinburgh, UK. His research area is mainly focused on the simulation, micro-fabrication and characteristics of micro electromechanical system (MEMS) sensors based on ZnO nanowires or other 1D materials for gas sensing and force sensing. He received his BEng (2018) in Chemical Engineering from Tianjin University, China, as well as his MSc (2021) in Nanotechnology from Swansea University, UK. After the postgraduate study at Swansea University, he started his PhD at the Institute for Micro and Nano Systems in 2022. |
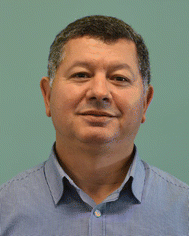 Chedly Tizaoui | Chedly Tizaoui, FIChemE, is a Professor in the Department of Chemical Engineering and he leads the Water and Resources Recovery Research (WR3) Lab at the Faculty of Science and Engineering, Swansea University. His research focuses on water and resources recovery. He applies advanced oxidation and separation processes to address challenging issues related to water and resources including the removal of micropollutants and valorisation of waste. |
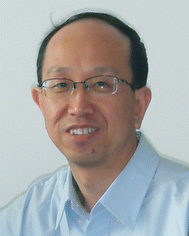 Yuesuo Yang | Yang Yuesuo is a professor in contaminant hydrogeology, at Jilin University, China. He has been undertaking research on theory and practical environmental hydrogeology. He has published about two hundred peer-reviewed journal papers, and he is a PI for various research projects including NSFC, MoST Advanced Sci/Tech Development (863). Recently he has mainly focused upon the contamination processes and remediation techniques of organics and heavy metals in soil and groundwater, in particular, some interesting work on the physical, chemical and biological processes of emerging contaminants (e.g. steroid estrogens) in the subsurface environment. |
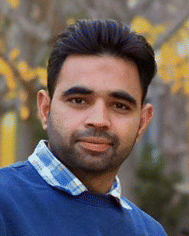 Jafar Ali | Dr Jafar Ali received his Ph.D. degree from Research Center for Eco-Environmental Sciences (RCEES), Chinese Academy of Sciences, China in 2020. At present he is a post-doctoral at College of New Energy and Environment, Jilin University. He was also a postdoc researcher in United Arab Emirates University, UAE between 2020 and 2021. He is also Assistant Professor in the University of Sialkot, Pakistan. His research interests include bioelectrochemistry, microbial fuel cells, renewable energy, nanostructured electrode materials and modified electrode surfaces, electrochemical sensors, and pollution remediation. |
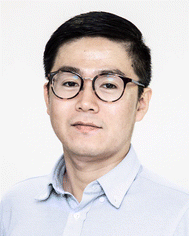 Wei Zhang | Dr Wei Zhang is currently a lecturer at the Department of Chemical Engineering, Swansea University. His main research interest lies in developing novel water treatment and monitoring technologies. During his research career, he was the recipient of many prestigious awards, including Australian Endeavour fellowship in 2014, Japan Society for Promotion of Science (JSPS) overseas fellowship in 2015, and Horizon 2020 Marie Skłodowska-Curie Actions (MSCA) fellowship in 2017. He also serves as a topic editor for journal Biosensors and Frontiers in Sensors. |
1. Introduction
In recent years, endocrine disrupting compounds (EDCs) have aroused public concern due to their adverse effects on the endocrine systems of some aquatic and terrestrial animals. Rapid industrialization such as food packaging, plastic manufacture, pharmaceuticals, and pesticides are mainly responsible for the increased level of EDCs in the environment and wastewater. Endocrine disrupting compounds (EDCs) often mimic endogenic hormones based on their structural similarity or interfere with normal activities of hormonal receptors by altering their behaviors. Even a trace concentration in EDC-contaminated water can induce acute or chronic health impacts on human beings or animals. As a big family, bisphenols (BPs), polychlorinated biphenyls (PCBs), phthalate esters, alkylphenols, and natural and synthetic estrogens, are the commonly reported categories of EDCs, and each category has many subtypes.1 Moreover, a growing number of artificial and naturally occurring compounds are considered as suspected EDCs because of equivalent harmful consequences; therefore effective control and management is essential. Several critical reviews in relation to the classifications and toxicity mechanisms of EDCs have been published during the last few years. From a clinical perspective, Diamanti-Kandarakis et al.2 elaborated an overview on health risks after EDC exposure aiming to provide readers with an in-depth understanding of endocrine disorders, organ failures, and carcinogenesis.
EDC detection results acquired from various chromatography methods are widely regarded as gold standards by worldwide authorities. Omar et al.3 published a review paper concerning the determination of EDCs primarily achieved by liquid chromatography mass spectrometry-mass spectrometry (LC-MS-MS), gas chromatography-mass spectrometry (GC-MS) and other bioanalytical approaches. Nevertheless, these methods always have some drawbacks such as the use of costly equipment, complicated operation, and long analyzing time. Furthermore, specimens ready for testing usually need to be sent to assigned laboratories, which are, to some extent, not feasible for large scale and point-of-use applications. Hence, it has become imperative to develop low-cost, simple, and sensitive monitoring platforms with miniaturized devices for rapid determination of EDCs in water and wastewater.
To date, a variety of sensing technologies have been applied for EDC detection. Scognamiglio et al.4 conducted a review on commonly used monitoring tools for EDCs, including in vitro bioassays and chromatographic analysis, with biosensing techniques discussed at greater length. Similarly, Rodriguez-Mozaz et al.5 reviewed over 100 publications regarding the detection of EDCs using biosensors, where the monitoring of endocrine disorders or signals captured after the receptor–analyte conjugation were described in more detail. Compared with the conventional methods, Jaffrezic-Renault et al.6 paid more attention to the detection of EDCs by electrochemical techniques. However, advances summarized in their review were mostly reported within the years 2016–2019. As an extended version of this, Azzouz et al.7 referenced more literature associated with EDC determination via electrochemical strategies on nanomaterial-based platforms. Moreover, Sofen et al.8 provided a review article related to the screening of endocrine disrupting pollutants using electrochemical sensors. Despite the above good perspectives, there is really a need to expand the scale of the evaluation by analyzing more references to support each type of EDC.
This review begins with the discussion of EDCs especially for common compounds of each type, along with their sources and toxicities. Suspected EDCs are also mentioned to help readers have an entire picture. Historical water pollution and regulation issues are stated reflecting the urgency in quality control of water sources. As a methodological comparison, EDCs detected using non-electrochemical methods are firstly discussed. Analyses of electrochemical biosensors include several subsections, starting from electrochemical techniques, commonly used electrodes, surface functional layers, biorecognition elements to electrochemical biosensors for EDCs. Finally, conclusive statements regarding electrochemical biosensors for EDCs, and current challenges as well as future development are also presented.
2. Endocrine disrupting chemicals
EDCs can be roughly divided into naturally occurring products and artificial compounds, where the latter cover the larger proportion ranging from industrial additives to daily-consumed utensils, aliments, and medications. EDC contaminated effluents eventually reach natural water environments via different routes, increasing the health risk. Toxicity mechanisms and corresponding adverse effects after exposure are depicted in Table 1. Unlike other water pollutants, EDCs consist of multitudinous types and subvarieties. Commonly found EDCs include bisphenols, polychlorinated biphenyls, phthalate esters, alkylphenols, and natural and artificial estrogens. Other less studied and suspected EDCs are also included in this review. Common chemical structures regarding each type of common EDC are presented in Fig. 1.
Table 1 Common EDCs with their toxicities and adverse effects
EDCs |
Toxicology |
Adverse health effects |
Bisphenols |
Acting on estrogen, androgen, and thyroid receptors |
Cancers, diabetes, heart diseases, and reproductive dysfunction |
Polychlorinated biphenyls |
Interfering with cerebral glands, thyroid receptors, and reproductive systems |
Carcinogenic, mutagenic, teratogenic effects and immunotoxicity |
Phthalate esters |
Altering cerebral, peripheral, and reproductive hormone secretion balance |
Diabetes, hepatotoxicity, precociousness, low birth weight, abortion, and respiratory issues |
Alkylphenols |
Abnormally affecting ERK signaling pathways |
Tumorigenicity, immune and metabolic system disturbance |
Natural and synthetic estrogens |
Mimicking estrogenic hormones due to high structural similarity |
Irregular reproductive activities, carcinogenicity, cardiovascular risks, headache, and other physiological pains |
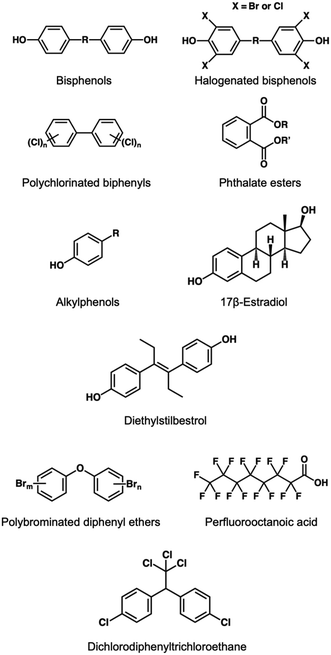 |
| Fig. 1 General chemical structures regarding each type of common EDC. | |
2.1 Bisphenols
Bisphenols (BPs) are the most prevalent EDCs that are always composed of two phenolic bodies linked together with a bridging component. The central connection is an essential part to differentiate from other variants. To date, BPs have approximately 20 variants. Bisphenol A (2,2-bis(4-hydroxyphenol) propane, BPA) is the most notorious BP over other analogs and even in the entire EDC family. BPA can bind to the pregnane X receptor and different estrogen receptors (ERs) such as ERα, ERβ, and estrogen related receptor γ (ERRγ), resulting in a variety of endocrine disrupting effects like immune system damage, neurological toxicities, metabolic disorders, and reproductive dysfunctions in the human body.9 Furthermore, BPA also exhibits toxic behavior towards wild animals particularly for the aquatic life when the concentration rises to 1–10 μg mL−1.10 Due to the serious consequences, other bisphenol analogs have replaced the use of BPA. Bisphenol S (4,4-sulfonyldiphenol, BPS) is one of the ideal BPA substitutions and is extensively used in many applications. Unfortunately, recent studies reveal that BPS can induce heart problems by influencing ERβ (ref. 11) and has strong toxicity to antiandrogenic activities.12 As another alternative of BPA, bisphenol AP (4,4′-(1-phenylethylidene) bisphenol, BPAP) is also evidenced as a toxic chemical. Xiao et al.13 demonstrated that exposure to BPAP in trace amounts might lead to anti-estrogenic effects and blood glucose level disorders. Bisphenol AF (2,2-bis(4-hydroxyphenyl) hexafluoropropane) belongs to a halogenated BPA, where hydrogen atoms on the two methyl radicals are fully replaced by fluorine atoms. Unlike BPA, BPAF can activate ERα while presenting antagonistic behavior towards ERβ, without the need for ERRγ. In terms of toxicity, several research groups have confirmed that BPAF can negatively impact heart and liver tissues in zebrafish.14,15 BPA and BPS are commonly used industrial chemicals in the manufacture of epoxy resins and polycarbonate products reaching their ubiquity in many hard plastic containers.8,11 BPAP and BPAF are essential components for polymer processing and can be widely found in rubber products, thermals papers, cosmetics, and electronic parts.11,14 There are some special BPs, where the bridging part remains the same as BPA, but has halogenated replacement (i.e., chlorinated, or brominated reaction) on both phenolic rings, including tetrabromobisphenol A (TBBPA), tetrabromobisphenol S (TBBPS), and tetrachlorobisphenol A (TCBPA). Bioassays showed that these halogenated BPA derivatives are capable of interfering not only with ERs,16 but also glucocorticoid receptors (GRs), androgen receptors (ARs),17 peroxisome proliferator-activated receptor γ (PPARγ), and thyroid receptors (TRs).18,19 However, adverse health effects on human beings need to be further investigated. Very recent studies on the carcinogenic nature of TCBPA and genotoxicity of TBBPA and TBBPS were elaborated by Lei et al.20 and Barańska et al.,21 respectively. TBBPA, TBBPS and TCBPA are known as common brominated flame retardants, and they are also often used in plastic paints, synthetic textiles, and electrical devices.22,23
2.2 Polychlorinated biphenyls
Polychlorinated biphenyls (PCBs), another class of man-made EDCs, have 209 congeners, and all of them follow a general formula C12H10−xClx. Every single PCB is identified by a specific BZ congener number according to the position and number of chlorine atoms on a biphenyl body and is usually named PCBx. Among them, tetrachlorobiphenyls such as 2,3′,5,5′-tetrachlorobiphenyl (PCB72) and 3,3′,4,4′-tetrachlorobiphenyl (PCB77) are frequently reported. Dioxin-like PCBs can bind to aryl hydrocarbon receptor (AhR) and are considered as the most hazardous and anti-estrogen chemicals, consisting of PCB77 and other 11 congeners.24 Moreover, non-dioxin-like PCBs like PCB28, PCB101, and PCB180 are also frequently reported. Non-dioxin-like PCBs prefer to interfere with ERs and ARs, leading to various adverse effects.25 Since many congeners have similar structures, PCBs mostly exist in a mixture. Aroclor, one of the commercially available products, contains over 100 variants of PCBs. Aroclor 1254, a C12H5Cl5-dominanted PCB mixture, consists of four different congeners which are identified by the four to seven substitutions of chlorine atoms on the biphenyl.26 PCBs are widely used in the manufacture of electrical parts, or industrial reagents such as lubricants, thermal transfer fluids, and coolants.27 The persistence in the natural environment makes PCBs (especially heavier congeners) prone to accumulation in organisms through the food chain. Their strong carcinogenic nature has been verified by the International Agency for Research on Cancer (IARC).28
2.3 Phthalate esters
Phthalate esters are also known as phthalates or phthalic acid esters (PAEs). They are often used as plasticizers to reduce the brittleness of raw polyvinyl chloride (PVC) products, but also can be found in many daily necessities and baby toys.29 Phthalate esters usually have 26 derivatives, with molecular weights (MW) varying from 194 to 531 g mol−1. Common phthalate esters incorporate dimethyl phthalate (DMP), dibutyl phthalate (DBP), and di(2-ethylhexyl)phthalate (DEHP). DEHP can be also named as dioctyl phthalate (DOP), which is different from di(n-octyl) phthalate (DNOP), although they have equivalent MW. Phthalate esters with short carbon chains (e.g., DMP) are more volatile than the longer ones, thus increasing the risk of respiratory symptoms such as asthma.30 DEHP is a typical long-chain phthalate ester that tends to exist in water or food rather than air due to the heavier MW. Careless exposure to phthalate esters results in imbalance on hormonal levels and therefore has toxic effects on the brain, liver, and reproductive organs, which is highly detrimental to pregnant women, infants, and adolescents.30
2.4 Alkylphenols
Alkylphenols, a class of organic chemicals obtained from the alkylation of phenols, can also induce endocrine disruption in the event of exposure. Alkylphenols contain 9 subtypes that are defined according to the number of carbon atoms on the alkyl chain. Like the isomerism in phthalate esters, each of alkylphenol subtype has its own isomers. Alkylphenols have higher stability and can remain in natural environments for a long period. Octylphenols (OPs) and nonylphenols (NPs) are frequently found in water sources. Priac et al.31 not only described these two subtypes, but also emphasized their polyethoxylated precursors namely alkylphenol ethoxylates (APEOs). APEOs are not very stable and are easily subjected to a metabolic process to produce alkylphenols.30 The toxicity of alkylphenols is derived from the disruption of signaling pathways associated with extracellular signal-regulated kinases (ERKs), and thus abnormally interferes with membrane estrogen receptors (mERs). ERK signaling pathways are closely related to many cellular activities such as division, differentiation, apoptosis, and carcinogenesis.32 Long chain alkylphenols are often applied as non-ionic surfactants, which are the main ingredient in detergents or cleaners. Moreover, they are also widely used in the production of oily chemicals, rubber industries, paper manufacture, or used as an alternative of flame-retardant materials.31
2.5 Natural and synthetic estrogens
Organisms can naturally produce a variety of estrogens in their bodies, including estrone (E1), (17β-) estradiol (E2), estriol (E3), and estetrol (E4). These estrogens can be recognized by nuclear estrogen receptors (nERs) and mERs.31,33 Estrogens released at a reasonable level can maintain the normal working of reproductive and cardiovascular systems and develop cerebral and osseous tissues.31 The unusual release of these sex hormones can also cause endocrine disrupting consequences. Diethylstilbestrol (DES) belongs to synthetic estrogen, of which usage can be traced back to 1940. DES is initially treated as a medical reagent for pregnant women to prevent miscarriage or to solve other pregnancy-related problems. Due to the highly similar structure, DES can pretend to be an endogenic estrogen interacting with receptors in the body, leading to endocrine disruption.
2.6 Less studied and suspected EDCs
Polybrominated diphenyl ethers (PBDEs) are another class of artificial organic halides. Like PCBs, PBDEs are also a big family that have 209 congeners. They are identified depending on the number of bromine atoms in the molecule. Common PBDEs include tetra-, penta-, octa-subtypes, and decaBDEs. As another widespread flame-resistant material, PBDEs are often added in the manufacture of polymeric foams (e.g., polyurethane foams), plastics, electronics, and textiles.34 PBDEs are proven EDCs that have negative effects on estrogenic and thyroid receptors, resulting in a chaotic level of corresponding hormones.35 Perfluorooctanoic acid (PFOA) belongs to a side product in the manufacture of fluoropolymer-related products, and widely used as an industrial surfactant. PFOA exists in soil, sediment, and is more likely to be in water due to the carboxylic group. It is regarded as an EDC that shows the strongest resistance against the degradation of the natural environment.36 Some health reports indicate that PFOA is closely related to thyroid diseases and carcinogenesis.37 However, more theoretical data is still required to interpret its in-depth toxicity mechanism. Dichlorodiphenyltrichloroethane (DDT) is one of the most famous organochlorides that has been extensively used as a pesticide and insecticide. It exhibits strong hydrophobicity and therefore prefers to seep into soil, sediment, and accumulate in lipids. Like PFOA, DDT is supposed to have carcinogenicity but needs to be confirmed by more studies.38
Organic compounds with similar chemical structures or hormone-disordering effects to EDCs but have not been proven yet are classified as suspected EDCs. Many problems, such as bioaccumulation in organisms, toxicities, environmental contamination records, and official guidelines, still lack conclusive answers to date. Suspected EDCs include polychlorinated dibenzo derivatives, polycyclic aromatic hydrocarbons (PAHs), mycotoxins, pharmaceuticals, personal care products, and broad-ranging agricultural chemicals. Polychlorinated dibenzo derivatives consist of polychlorinated dibenzo dioxins (PCDDs) and polychlorinated dibenzo furans (PCDFs), which are unwanted impurities in the production of chlorinated products (e.g., PCBs).39 PAHs refer to the hydrocarbons with two and more benzene rings and are normally produced from the incomplete combustion in cooking (e.g., use of coal and oil), smoking (e.g., tobacco), and driving (e.g., petrol).40 Mycotoxins are a group of xenoestrogens produced by fungi and are sometimes also called mycoestrogens. Zearalenone (ZEN), a typical type of mycotoxin, is generated by the estrogenic metabolism of Fusarium and Gibberella species in many cereal crops.41 17α-Ethinylestradiol (EE2) is an artificial compound with a similar chemical structure to E2, which is used as a contraceptive medication coupled with progestins. EE2 can induce various side effects on human beings. It has also been demonstrated that EE2 presents serious neurotoxicity towards fishes (e.g., zebrafish larvae) when it is released into natural aquatic areas.42 Oxybenzone is created naturally in many flowering plants and is often applied as the main constituent of sunscreen creams (i.e., one of the common personal care products).43 However, skin allergies and other adverse effects caused by oxybenzone have been increasingly reported in recent years,44,45 making this sunscreen active ingredient more controversial. Moreover, suspected EDCs also incorporate many agricultural chemicals such as pesticides (e.g., endosulfan and kepone), herbicides (e.g., atrazine), and fungicides (e.g., vinclozolin). The endocrine disrupting effect regarding these chemicals requires more studies.
2.7 Contamination events, official warnings, and safety guidelines
The strong reliance on polycarbonate plastics and epoxy products worldwide leads to the extremely high use of BPA. China accounted for one fifth of the world's total consumption of BPA in 2013. Lin et al.46 selected Yuyao, Taizhou, and Wenzhou City in Zhejiang Province, the main areas for plastic production and sale in China, to explore the influence of plastic factories on surface soils, nearby rivers and aquatic organisms. The study revealed that the level of BPA from the river in Yuyao City was 0.24–5.68 ng mL−1, which was higher than the maximum quantified BPA concentrations in Asia of 317 pg mL−1, North America, and Europe of 99 and 14 pg mL−1, respectively.47 On the other hand, the wide existence of BPA in baby products, such as milk bottles and infant formula cans, was demonstrated by the United States Food and Drug Administration (USFDA).48 Many organizations or countries have implemented legislations on prohibition of BPA. Canada was the first country to ban BPA in the production of baby bottles in 2010, followed by the European Union (EU), China, and Malaysia issued similar regulations to increase the stringency upon BPA.49 As an alternative of BPA, BPS and BPF have been widely used in many countries to make ‘BPA-free’ products. Yamazaki et al.50 investigated the level of BPS in water sources from China, Japan, South Korea, and India, of which the highest concentration was found in the Adyar River of 7.2 ng mL−1, Chennai, India. In addition, the amount of BPF in water samples was also provided by their group, indicating higher concentrations in China, Japan, and South Korea, especially the Tamagawa River of 2.85 ng mL−1, Japan, to which great attention should be paid.49 Liu et al.51 noted that the water specimens from the Taihu Lake in Jiangsu Province, China, not only had common BPs (i.e., BPA, BPF, and BPS), but also had BPB, BPZ, BPAF, and BPAP with comparable contents, among which an apparently high concentration of BPAF was determined in a range of 0.11–0.14 ng mL−1, with a median of 0.11 ng mL−1. Liu et al.52 confirmed the detection results of surface water samples given by Yamazaki et al. and Liu et al., and comprehensively evaluated the BPs in sewage samples from wastewater treatment plants (WWTPs), showing that BPA, BPS, and BPF were the main pollutants in China and the United States, while BPF in India was below the detection limit. Interestingly, BPFA was only detected in samples collected from China.51
Earlier electrical devices contain high levels of PCBs, where some of them are still being used at present. Natural environments will suffer from great pressure on decomposition if PCB-containing electrical devices are improperly recycled or abandoned after they reach their lifetimes. PCBs not only exist in water environment, air, and soil, but also are highly prone to be accumulated in fatty tissues due to the strong lipophilic property.53 Bench et al. found that PCBs had been accumulated up to 10 million times in rainbow trout in Lake Ontario, which resulted in the closure of local fisheries.53 Additionally, the impact of PCBs on seawater was proposed in the report as the pollution initially stored in phytoplankton could become more serious in advanced organisms through the food chain, and ultimately threaten human health.53 Owing to increasing worldwide pollution concern, many countries have started taking actions. On PCBs, Canada passed a law in 1977 to ban the import, manufacture, and sale of PCBs. After that, the US, UK, Australia, and EU successively banned the use of PCBs in 1979, 1981, 1986, and 1987, respectively. To strengthen the quality management of drinking water, the United States Environmental Protection Agency (USEPA) set the highest acceptable concentration for PCBs of 500 pg mL−1.54 In 2001, governments around the world signed the Stockholm Convention aiming to eliminate PCBs by 2028. As one of the signatories to the convention, China was committed to proper inventory and harmless disposal of PCBs.55
Like BPs, phthalate esters as plasticizers are also detected in a range of plastic products. The weak behavioral and cognitive abilities of young children can be derived from more exposure to phthalates than adults, and therefore it is significant to control the phthalate content in baby products. The EU has banned six phthalates, including butyl benzyl phthalate (BBP), di-isodecyl phthalate (DIDP), di-isononyl phthalate (DINP), DBP, DEHP, and DNOP.56 An investigation into the health risk of phthalates was conducted by Abtahi et al.,56 showing that DMP and DEHP were the primary contaminants found in different water samples from Tehran, Iran, along with other phthalates detected in relatively low levels, involving BBP, DBP, diethyl phthalate (DEP), and DNOP. The estimation on the burden of disease after drinking water contaminated by DEHP was also performed by the group based on the theory of disability-adjusted life year (DALY).56 Paying equivalent emphasis on the health of children, the Minnesota Department of Health (MDH) listed BBP, DBP, and DEHP as Toxic Free Kids Act Priority Chemicals in 2011, with corresponding safety guidance of drinking water for BBP (100
000 pg mL−1) and DBP (20
000 pg mL−1) issued in 2012 as well as DEHP (7000 pg mL−1) in 2013.57 The guideline value of DEHP was 1000 pg mL−1 higher than the maximum contaminant level (MCL) provided by the FDA (6000 pg mL−1) but was 1000 pg mL−1 lower than the concentration set by the World Health Organization (WHO) and EU (8000 pg mL−1).58 The pollution of BBP, DBP, DEP, DEHP, DMP, and DNOP from other countries (i.e., Canada, Spain, South Africa, China, and India) was reviewed by Ghosh et al.,59 which showed that the content of nominated phthalates in water samples was not higher than 7 ng mL−1.
A total amount of 500
000 tons of APEOs are annually produced worldwide to meet the demand for non-ionic surfactants and other applications.60 Alkylphenols are the product of APEOs after biodegradation. Municipal wastewaters and industrial effluents (e.g., factories for paper and textile production) are usually the main sources of environmental contamination.62 Uğuz et al.62 published a comprehensive review on the pollution of alkylphenols and their health threat to living organisms. In their work, they found that nonylphenol ethoxylates (NPEs) and NPs widely existed in water and sediment, and pointed out that the concentration of NPs in rivers from the United States, United Kingdom, Canada was much higher (i.e., ranging from ug L−1 to mg L−1) than the level for inland surface waters of 300 pg mL−1 from the Directive 2008/105/EC.63 Although the current concentration of NPs in various natural waters is not high enough to cause death, the bioaccumulation in living creatures can still reach a lethal level as time passes, which should be paid more attention.62 Moreover, the maximum permissible standard of 7000 pg mL−1 for 4-NP in ocean water was confirmed by the USPEA to ensure the normal growth of most marine animals.64 Lei et al.65 investigated several estrogens (i.e., E1, E2, E3, EE2, and DES) in water samples from the Beijing–Tianjin–Hebei region, of which the total concentration in wastewater was 0.468 ± 0.0272 ng mL−1 and was 0.219 ± 0.023 ng mL−1 in river water. Natural or synthetic estrogens have negative effects on surface waters, particularly for fish species.64 Similarly, Wedekind et al.66 focused the analysis on the change of the sex ratio of fish caused by the presence of estrogens and endeavored to find the reason for the strong adaptation in estrogen-contaminated water in some fish populations. However, more efforts on this field were suggested to be taken in these two papers due to the current less-supporting evidence. As a major pollutant of estrogen, E2 was listed in the Water Framework Directive (WFD) of the EU in 2013, along with a safety guideline of 0.4 pg mL−1.67 It has been confirmed that PBDEs can accumulate in milk products (e.g., human breast milk68) and fatty tissues. They also mainly exist in surface water, soil, and sediment. Surface waters containing PBDEs, of which the concentrations range from a few pg L−1 to hundreds of ng L−1, have been registered in countries from all over the world, including Switzerland (river and lake water), Canada (lake water), the United States (lake water and estuary), Paris (river water), and the UK (river water).34 PBDEs were banned in the US and EU in 2004.69,70 Until recently, there is no specific maximum acceptable level for PBDEs in drinking water. PFOA is widely found in surface waters,71 coastal waters,72 and seawaters.73 The potential adverse effects of PFOA on human beings and natural environments, especially for infants and aquatic lives, respectively, have been of significant concern, although relevant toxic events are unknown.36 Aiming to reduce the extensive exposure, PFOA was discontinuously produced and gradually phased out in the United States in 2015. Canada set the maximum acceptable concentration (MAC) for PFOA in drinking water of 200 pg mL−1.74 The health advisory for PFOA in drinking water in China was firstly claimed by Zhang et al.,75 as 85 pg mL−1. Likewise, DDT has been banned in many countries due to its strong toxicity. However, it is still being used in some developing countries or regions to fight against malaria. Ochoa-Rivero et al.76 reported that DDT with a total amount of 2804 ng mL−1 was found in agricultural areas in Mexico. The value was extremely higher than those detected in water sources from other areas in Mexico or other countries where DDT was used.76 With the consideration upon the continuous consumption of DDT, the WHO issued a guideline value of 1000 pg mL−1 in drinking water.77
2.8 Non-electrochemical methods for detection of EDCs
Traditional determination of EDCs was normally performed using bioanalytical methods such as cell assays (i.e., recombinant yeast incubation, cell proliferation, and in vitro cell bioluminescence tests) and enzyme-based assays like luciferase induction.78 However, these bioassays are always complicated in terms of manipulation and require lengthy analyzing duration. Recently, more advanced analytical techniques, including enzyme-linked immunosorbent assay (ELISA), surface enhanced Raman spectroscopy (SERS), and biofluorescence, have been developed to improve test efficiency and reduce batch-to-batch variation. ELISA is a bioassay where the antibody–antigen interaction is usually achieved on 96-well microplates. Biorecognition results are obtained after the introduction of signal-indicated substrate solutions. ELISA is easy to follow, and the analysis can be conducted in different specimens. Commercial ELISA kits have been available for the quantitative determination of EDCs. This method retains its predominance and is sometimes considered as a golden standard for comparing the results obtained from other approaches, although the method often suffers from a main drawback of short data-collection time.79 EDCs measured using SERS are reported in some publications. For example, Tu et al.58 developed aptamer-functionalized magnetic particles to provide binding sites for the competitive DEHP that were attached to the surface of SERS silica particles and the target DEHP. The magnetic beads were then aggregated by a magnet to yield the supernatant of silica particles containing Raman receptor molecules. Raman spectra with characteristic peaks of DEHP in different intensities were clearly observed after different concentrations of DEHP were introduced.58 However, this analysis can be only achieved in laboratories using a bulky analytical instrument and is not suitable for on-site tests.
Another commonly reported method for the detection of EDCs is biofluorescence. It combines biorecognition elements with fluorescent substances such as dyes, labels, or quantum dots, indicating the analyte concentration according to the change in fluorescence intensity. A non-equilibrium rapid replacement aptamer (NERRA) assay was conducted by Kim et al.80 based on a portable device, where the phthalate esters were detected in the solution containing free PoPo3 dye attached aptamers. Results of biofluorescence were highly comparable to those of chromatographic methods, but the analyzing time was dramatically reduced to 30 seconds. The experiment focused only on detecting the mixture of phthalate esters rather than a single chemical.80 Aptamer-based biosensors with fluorescence characteristics account for a large percentage of the biofluorescence technology. Lim et al.81 reported an aptasensor connected with two types of quantum dots (QDs) to detect DEHP. QDs 565 were immobilized on magnetic beads while QDs 655 dissociated from the DNA probes after DEHP binding to the aptamer. Apart from phthalate esters, fluorescent aptasensors are also widely used to detect other EDCs like BPs82 and PCBs.83,84 Instead of fluorescent aptamers, Tang et al.85 fabricated an antibody-based biosensor based on optical fibers for the monitoring of eight phthalate esters. The antibodies coupled to fluorescent tags reacted with the analyte to create inhibition signals. In comparison to Kim et al.,80 their immunosensors featured the sensitive detection of a single phthalate ester (i.e., DMP), demonstrating the reliability of the protocol.84
In recent years, chromatographic or chromatographic-spectrometric combined analyses used for the detection of EDCs, such as gas chromatography coupled to mass spectroscopy (GC-MS),86 high-performance liquid chromatography (HPLC),87 and liquid chromatography coupled to mass spectrometry (LC-MS),88 have been acknowledged by more and more environmental agencies or health organizations due to their excellent sensitivity and accuracy. These methodologies enable the identification of a series of EDC variants at a single measurement, and are therefore of great significance in multi-analyte monitoring. Nevertheless, limitations regarding these techniques need to be taken into consideration at the same time, for instance, expensive apparatus, scheduled maintenance, and demand for experienced technicians. Moreover, pre-concentration, extraction (micro-extraction), and derivatization of specimens are other inconveniences when using these chromatographic methods, which often make the experiment unsuitable for in situ applications.89 In fact, the complexity of EDCs worsens the limitations on the use of bioassays, chromatographical analyses, and other non-electrochemical approaches, pushing the advent of a highly efficient and miniaturized setup working under a simple detection mechanism but capable of providing competitive results. The developed prototype tends to present more friendliness toward real samples in a point-of-care application. Compared with the conventional detection methods, biosensors in combination with electrochemical techniques have been increasingly used as a delicate sensing systems to determine EDCs in water sources relying on the higher selective and sensitive nature. Electrochemical biosensors equipped with different biorecognition components will be elucidated at the latter section of the review.
3. Electrochemical biosensing of EDCs in water
Biosensing is a process that achieves the detection of the target analyte using a device where the surface is covered with biorecognition components. Electrochemical biosensing allows biochemical activities to occur at an electrode–solution interface, followed by a signal transduction to yield readable electrical responses that are presented as original curves via a data processing system (Fig. 2). This technique integrates superior sensitivity with advantages of shorter analyzing duration, inexpensiveness, and miniaturization feasibility, which has been known as a promising method for the determination of small molecules. Commonly used electrochemical biosensors can be divided into immunosensors, aptasensors, and enzymatic sensors. Each class of biosensors has its own unique principle for detection. Immunosensors are a class of bioelectrodes where the surface is functionalized with antibodies, which can selectively recognize antigens via the antibody–antigen interaction. The affinity offered by aptasensors is derived from the chain-like structure that has strong locking effect upon the analyte. Electrodes decorated with a bioactive enzyme exhibit electrocatalysis towards the target molecules. Some natural (i.e., cyclodextrins) or artificial (i.e., molecularly imprinted polymers) recognition elements have attracted tremendous interest in the past decade because of their biomimetic properties. The surface of molecularly imprinted polymer (MIP)-based sensors can provide cavities with an identical shape of the analyte, therefore showing enhanced specificity in the sensing process, while cyclodextrin-related sensors capture the target via their adsorptive chambers. The electrochemical biosensing system consisting of various electrochemical techniques, electrodes and surface functional supports will be firstly introduced in this section, followed by different bioreceptors, especially the binding schemes of antibodies in immunosensors will be discussed in more detail.
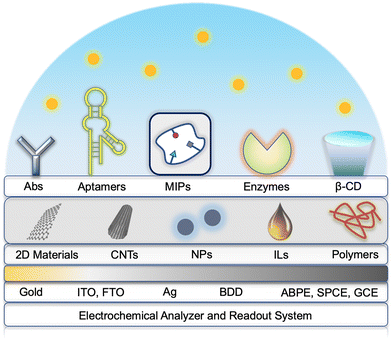 |
| Fig. 2 Schematic diagram of electrochemical biosensors used for detection of EDCs in water (among them, images of 2D materials, CNTs, and ILs were reprinted with the permission from ref. 193–195, respectively). | |
3.1 Electrochemical monitoring system
An electrochemical monitoring system is normally constructed by connecting a biofunctionalized electrode with an electrochemical analyzer. The analyte-containing solution is then allowed to be in contact with the specific sensing area of the transducer, triggering the conversion from biological events to electrical responses as amperometric and impedimetric forms (Fig. 3). All these measurements take place after application of a potential on the working electrode to produce the corresponding current. Voltammetry is a method to analyze current changes over an assigned potential scanning under linear (e.g., linear sweep voltammetry), periodical (e.g., cyclic voltammetry), and pulsed (e.g., differential pulse voltammetry and square wave voltammetry) behaviors. Linear sweep voltammetry (LSV) is the most fundamental electrochemical technique with a simple working principle in which the potential linearly changes with time.90 An advanced version of the technique called second-order derivative linear sweep voltammetry (SDLSV) produces electrochemical results often with lower background noise and larger peak current due to the more complicated mathematical analysis.91 Cyclic voltammetry (CV) is regarded as a derivative of LSV. The linear potential in CV scans starting from a higher level to the switching point (i.e., lower level), followed by a reversing of the potential from the switching point back to the initial level.90 The workflow can be performed in multi-cycles under a fixed scan rate. In most cases, the resulting current varies as the potential scans forming a closed voltammogram that contains an oxidation and a reduction peak. CV is one of the most common electrochemical methodologies utilized not only in the sensing process, but also for the assessment of electrode modification from untreated up to a fully functionalized situation.92 Differential pulsed voltammetry (DPV) is another commonly reported electrochemical technique. Unlike LSV and CV, DPV embeds voltage pulses on a linearly changed potential, creating two momentary currents right before and after the pulsed voltage changes. The final current difference instead of an absolute value versus an assigned range of potential contributes to a characteristic DPV voltammogram.93,94 Like DPV, voltage pulses in square wave voltammetry (SWV) are superimposed with a staircase-shaped potential, and the current difference is measured based on the two current values recorded right before and after the pulsed voltage changes.95 Amperometry is another frequently used electrochemical technique. It is different from voltammetry as a constant potential is usually applied on the working electrode to generate a transient current in relation to the oxidation or reduction reaction of redox couples. Amperometric measurement can diminish the undesired noises for the interferants or impurities in a tested solution by adjusting the impressed voltage, therefore possessing high sensitivity towards the detection of interest.96,97 Photoelectrochemistry (PEC) is an emerging technique that has been used for biosensing. Following the three-electrode set-up, a PEC system contains a working electrode modified with photosensitive semiconductor materials, coupled with a reference and counter electrode. In this method, a lamp with a medium wavelength is always required as an irradiation source. After a specific voltage is applied to the working electrode, a photocurrent is generated and varies with time. The change of photocurrent density within a light-on duration corresponds to the concentration of the analyte. PEC can produce responsive curves with a distinctive difference even in ultralow concentrations, which has been demonstrated in many studies.162,163,166,167,193
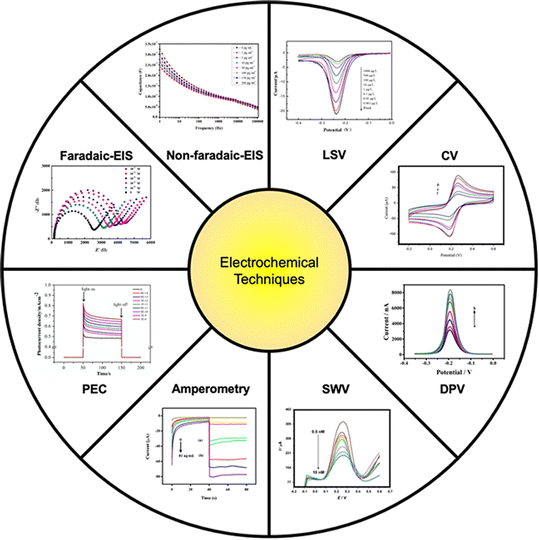 |
| Fig. 3 Electrochemical techniques used for the detection of EDCs (reprinted with the permission from ref. 141 for LSV, ref. 164 for CV, ref. 153 for DPV, ref. 137 for SWV, ref. 129 for amperometry, ref. 161 for PEC, ref. 150 for faradaic-EIS, and ref. 136 for non-faradaic-EIS). | |
Impedimetric measurement applies a certain potential to the working electrode to generate a current and calculates the resulting resistance or impedance as a final readout. Resistance can be expressed as the slope of the I–V curve according to Ohm's law. The equivalent circuit merely contains a resistor that serves as the only element subjected to a fixed potential. However, the sample environment in actual electrochemical biosensing usually includes capacitive behaviors and Warburg diffusion (Zw), thus it is much more complicated than the model of simple resistance.98 Electrochemical impedance spectroscopy (EIS) is a typical representative of impedimetric methods that has received a great amount of attention in a recent couple of years due to simple operation, short response time, high sensitivity, and device portability.99 In theory, an alternating potential (e.g., sinusoidal potential) is introduced into the system producing a current in the same waveform but with a phase difference.100 The potential is then divided by the current to yield the impedance under each certain frequency.98 Impedance is a characteristic EIS parameter presented in a complex expression and can be drawn in the Nyquist plot, where the real part normally reflects the charge transfer resistance against redox couples moving from the bulky solution toward the surface of the electrode, and the imaginary part refers to the total capacitance generated at the electrode–solution interface.98,101,102 In other words, resistance is a special case of an impedance that lacks the imaginary part. Therefore, analyzing impedance or its parts is vital for the illustration of chemical or biological events (e.g., target molecules binding to the biorecognition element) occurring at the specific sensing area.
3.1.1 Electrodes for electrochemical analysis. Electrodes are the fundamental of signal transduction in electrochemical biosensors. The three-electrode system is widely employed for electrochemical detection, consisting of a working electrode, reference electrode, and counter electrode. The working electrode refers to a sensing platform with functional supports and bioreceptors, which is ready for capturing target molecules. Unlike the two-electrode system (i.e., only consisting of a working electrode and counter electrode), the reference electrode in the three-electrode system has a constant potential and negligible current, that can define the working potential after a certain voltage applied, sharing a part of the work of the counter electrode.103 Ag/AgCl is the commonly used material for reference electrodes. The counter electrode, or sometimes called auxiliary electrode, forms a closed path for current with the working electrode. Counter electrodes are often made of platinum. Electrodes fitted in electrochemical biosensors have the following characteristics: 1) good conductivity, 2) high durability, 3) large surface area, and 4) long-term stability. Conductivity is very crucial as it improves the sensitivity and accuracy of signal transduction. Durability increases the electrode tolerance to any damage (e.g., bending, scraping, and dropping). The surface area relates to the amount of bioreceptor loading that determines the specificity level. Stability ensures the electrode to be available under various conditions (i.e., temperature, pressure, and pH), which is beneficial to storage or transportation. Glassy carbon electrodes (GCEs) are the most used electrodes in electrochemical biosensors due to their low cost, good conductivity, and stability. Solutions containing biorecognition elements, or antigens, or functional compounds, are often drop-casted on the surface of polished GCEs. It is ready to use after a certain length of incubation.104 Carbon rod electrodes have a large surface area because of the special morphology, allowing the functionalization of bioreceptors with a high density.105 Other highly conductive electrodes like carbon paste electrodes (CPE),160,180 carbon electrodes (CE),171 and silver electrodes137 are also reported, but with fewer cases. Electrodes made of boron-doped diamond (BDD) possess advantages of high chemical stability and low background noise.151,164,179 Acetylene black paste electrodes (ABPE) mixed with paraffin are used as a sensing platform that integrates excellent electrical conductivity with high durability.157 A unique carbon electrode can be directly created by laser-induced carbonization on a polyimide (PI) substrate.106 The method for the electrode production does not require additional carbon materials and the surface patterns of the electrode can be drawn as the design of interest. Gold electrodes are another class of common electrodes with outstanding conductivity and stability due to the inherent properties of gold. Gold surfaces prefer to form gold-thiol complexes, and therefore are capable of binding cysteamine, thiolated self-assembled monolayers (SAMs), or biorecognition components. As a noble metal, it is worth exploring the methods on how to effectively use gold in the fabrication of electrodes. For example, Yu et al.142 prepared a film electrode via sputtering gold onto a polyethylene terephthalate (PET) substrate. The loading of the gold material was well controlled using this technique. Moreover, printing methods (e.g., screen printing) are advantageous for large volume production and depositing electrode materials (e.g., gold107 and carbon108,109,153,176,182) on a wide range of cheap substrates. Some transparent materials used in solar cells such as fluorine-doped tin oxide (FTO)162,163 and indium tin oxide (ITO)166,167 are also applied to make the electrode of electrochemical biosensors. Field-effect transistors (FET) are mostly used in semiconductors, where Si/SiO2 is often used as an underlayer substate.110 Titanium or other composites (e.g., HfO2/F16CuPc111) are then coated on top of the substrate serving as a dielectric layer, with the gold drain-source electrode finally created on the dielectric layer through a lithography technique. Recent advances show that FET systems can be used in electrochemical biosensors for detection of EDCs in water, but the reports are very limited.
3.1.2 Functional supporting on electrode surfaces. Biological events occurring on a bare electrode (i.e., untreated) often lead to undesired sensing results because of the limited conductivity, small specific area, low stability, and less biocompatibility to biorecognition elements. Hence, the introduction of a functional support on electrode surfaces can rectify these issues, which is regarded as another highly crucial step in the fabrication of electrochemical biosensors. Carbon materials such as graphene (Gr),129 graphene nanoribbons (GNRs),172 nitrogen-doped graphene nanoribbons (NGNRs),169 graphene nanoplatelets (GNPs),187 carbon nanotubes (CNTs),130,139,141,159,164,165,168,183 and carbon nanofibers (CNFs)138 are usually deposited on the surface of the electrode as a conductive underlayer to facilitate electron transfer, increase the surface area, and improve the mechanical strength. Alternatively, the surface area can be enlarged by adding porous nanostructures like cobalt phosphide decorated porous carbon microspheres (CoxP/NC)149 and nanoporous gold leaf (NPGL).181 Porous three-dimensional graphene (3D-GN) can evenly load functional substances (e.g., Cu and Fe3O4) to prevent them from aggregation owing to their surface energy.174 Derikvandi et al.140 used polyethyleneimine (PEI) to offer a similar microenvironment for the immobilization of platinum nanoparticles (PtNPs). Another purpose of increasing the surface area is to provide sufficient space for the functionalization of biorecognition elements and hence to enhance selectivity and specificity. Metal nanoparticles such as gold nanoparticles (AuNPs),159,161,164,165,169,185,188 silver nanoparticles (AgNPs),132 PtNPs,140 nickel nanoparticles (NiNPs),160 bimetallic hybrids like gold/palladium nanorods (Au@Pd NRs),136 and gold/platinum (AuPt),133 or metal/metal oxide composites, including amino-functionalized magnetite and gold nanoparticles (H2N–Fe3O4/Au NPs),144 CoFe2O4,136 functional copper magnetic nanoparticles (CuFe2O4–Pr–SH),141 and Ag–Cu2O,148 not only promote electron transportation, but also have strong electrochemical activity and enzyme-like catalytic or electrocatalytic ability, which belong to another class of common functional supports. Additionally, biochar nanoparticles (BCNPs) can also exhibit excellent electrocatalytic properties as well as a large surface area and have been well-developed in electrochemical biosensing.177,178 Quantum dots are a type of nanomaterial with a size less than 100 nanometers. Graphene quantum dots (GQDs)156 and palladium quantum dots (PdQDs)163 have been used to modify the electrode due to their good water solubility and biocompatibility. Apart from graphene, other two-dimensional (2D) materials have been discovered in recent years. Some studies have confirmed that the use of MoS2 in combination with graphene can effectively improve the sensitivity of the detection platform.112,152 As a novel planar material, MXene has been applied to support electrodes because of its large specific surface area, high electron mobility, and hydrophilic nature.113,173Electrode-supporting substances that contain functional ends are also frequently involved to present binding sites for biorecognition components. Instead of pristine graphene, graphene oxide (GO) is often employed to bind to other electrode additions such as ionic liquids (ILs),153 3-aminopropyltriethoxysilane (APTES),158 and metal nanoparticles,184 relying on its abundant oxygen-containing functional groups. However, the limited conductivity of GO usually leads to poor sensitivity in biosensing. Facing this challenge, the GO after being loaded with functional compounds tends to be chemically133,136,147,148,150,175,186 or electrochemically157,160,171,185,187 converted into reduced graphene oxide (rGO) to maximally recreate the original conductivity of graphene. Multi-walled carbon nanotubes (MWCNTs) with carboxylic groups can provide reaction sites for antigens,130 deoxyribonucleic acid (DNA) sequences,140,144 enzymes,179 and cyclodextrins.182 SAMs are sometimes introduced onto metal electrodes, where the functional terminals are able to couple with receptors, for instance, cysteamine grown on a gold surface to support MIP,169 mercaptoacetic acid fixed on a gold surface for antibody immobilization,135 and mercaptoundecanoic acid (11-MUA) grafted at a silver surface for the surface anchoring of an antibody.137 Serving as a specific organic linker for carboxylic groups, ethyl (dimethylamino propyl) carbodiimide (EDC)140 or ethyl (dimethylamino propyl) carbodiimide/N-hydroxysuccinimide (EDC/NHS)135,137,144,178 is often included in an experiment to achieve activation and protection from carboxylic radicals. However, based on the conclusion of Zhang et al.,114 Liang et al.134 utilized graphene oxide nanoribbons/multi-walled carbon nanotubes (GONRs/MWCNTs) hybrids to implant antigens without using linkage chemicals. Some polymers containing amino groups such as poly (1,5-diaminonaphthalene) (P(1,5-DAN))129 and polyaniline (PANI),132 can bind to antibodies through a direct and glutaraldehyde (GA)-linked interaction, respectively. AgNPs are capable of fixing thiol-terminated aptamers via the Ag–S bond,132 while AuNPs have the ability to fix antigens,130 thiolated aptamers150,152,153 or complementary chains149via the Au–S bond. Moreover, Beiranvand et al.139 functionalized Fe3O4/AuNPs with amino groups to react with GA at first, leaving free aldehyde groups that were used to combine with non-thiolated aptamers. Redox probes can elevate electrochemical activity and amplify sensing signals. Methylene blue (MB)142,143,145,153,154,181,185 is the most familiar redox probe applied to electrochemical biosensing, along with others like toluidine blue (TB),130 ferrocene (Fc),146,150 thionine (Thi),152 and biotin,148 are also reported. Cetyltrimethylammonium bromide (CTAB) is a cationic surfactant, can either adsorb nanoparticles by virtue of electrostatic effects,173 or preserve the activity of biological enzymes in the event of substrate reactions.179 The deposition of chitosan134,175 or Nafion168,176,177 on a biofunctionalized surface enables the electrode durability to be upgraded. ILs have the ability to form films as well and exhibit intense adsorption effects on the analyte of interest.153,170 Blocking agents such as bovine serum albumin (BSA)130,131,133,135,136,147 and 6-mercapto-1-hexanol (MCH),141–143,148,149,152–155 are always used as a final functional layer on the surface of immunosensors and aptasensors, respectively. These chemicals can cover unbound active sites to protect target molecules from any non-specific interactions, thus enhancing the sensitivity.
3.2 Receptors for biorecognition of estrogens
Biorecognition elements are the core constituents that contribute to electrochemical biosensors. Receptors that are commonly used to electrochemically detect EDCs in water, including antibodies (Abs), aptamers, MIPs, enzymes, and cyclodextrins, are often reported.
3.2.1 Antibody-based receptors. Antibodies belong to one of the most utilized biorecognition components and can be further classified into polyclonal and monoclonal subtypes. Electrodes functionalized with antibodies are usually called immunosensors. Polyclonal antibodies (pAbs) are the proteins generated after an exotic immunogen stimulation towards the immune system in living organisms (e.g., rabbit129 or chicken107). pAbs can conjugate with multiple epitopes on a single antigen, which can easily trigger a cross-reaction in complex sample environments that contain more than one analyte.115 The production of monoclonal antibodies (mAbs) normally undergoes a half in vivo (i.e., immunization activated after antigen injection) and a half in vitro (i.e., B cell-myeloma hybridoma culture) process.116 mAbs are mostly obtained from mice,117,129,135 which are different from pAbs as they interact only with a single epitope on an antigen. mAbs have the advantages of batch-to-batch reproducibility and negligible cross-reactivity but they are time-consuming to prepare and more expensive.118 Recent advances present that pAbs are more prevalent than mAbs used as biological receptors in electrochemical immunosensors because they can offer comparable sensitive results with a lower cost. The same as ELISA, the antibody–antigen conjugation in electrochemical immunosensing can also be divided into direct-, directly competitive-, indirectly competitive-, and sandwich schemes (Fig. 4). The direct scheme is the simplest method to detect the analyte. However, the non-specific binding of target molecules often occurs during a biorecognition process to interfere with the normal binding signals. Direct competition is another common method, where the experiment often introduces an antigen with different concentrations and a labeled competitor at a fixed concentration to simultaneously bind to the antibody. The directly competitive method, which reflects the analyte concentration by quantifying the amount of antibody-labeled competitor conjugation, avoids the interference of non-specific binding, and thus improves sensitivity and accuracy. Most of the EDCs belong to small molecules as their molecular weights are below 900 Dalton. It is hard to form a stable dual antibody binding (i.e., sandwich scheme) when the target molecules are small molecules. This is the reason for fewer reports on the sandwich-type detection method. However, the determination of small molecules can be realized by indirect competition. An indirectly competitive method usually contains two stages: 1) the introduced analyte, and the competitor immobilized on the electrode surface competitively bind to the primary antibody (Ab1); 2) the remaining Ab1-competitor continuously bind to the labeled secondary antibody (Ab2) after the removal of Ab1-analyte composites. It is noted that indirect competition can also cause cross-reaction due to the use of two types of antibodies, in which extra concern should be taken especially in the detection of multi-targets.119 Lu et al.120 reported an immunosensing conducted via a displacement method. In their work, the competitive antigen with a butyric acid-horseradish peroxidase (HRP) was firstly recognized by the antibody, followed by a replacement of the competitor after the analyte was introduced. A lower LOD for BPA was obtained using the proposed method in comparison to the conventional direct competition.120
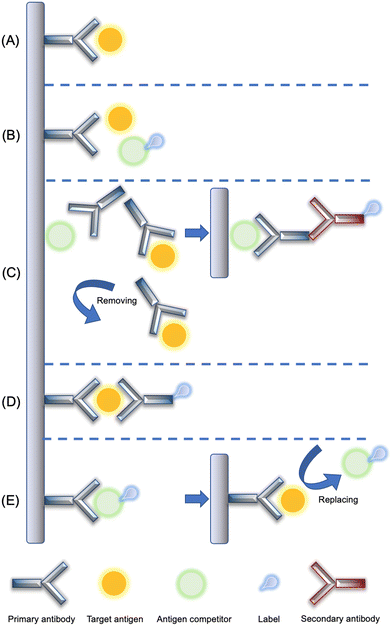 |
| Fig. 4 Different binding schemes between antibodies and antigens in electrochemical immunosensing: (A) direct, (B) direct competition, (C) indirect competition, (D) sandwich, and (E) displacement. | |
3.2.2 Aptamer-based receptors. Aptamers are single-stranded DNA (ss DNA) or ribonucleic acid (RNA) sequences that are selected from the ssDNA or RNA pool by virtue of the systematic evolution of ligands by exponential enrichment (SELEX).121 Aptamers can effectively bind to target molecules due to the structural change of the chains, which are also a type of common biorecognition element. Electrodes loaded with aptamers are often called aptasensors. Some aptamers or complementary DNA (cDNA) strands possess functional ends that can be immobilized on the surface of the electrode through different bonds. Aptamer-related detections are achieved following three methods. The first one is that aptamers directly capture the target molecules causing the increase of steric hindrance against the electron transfer from the bulky solution towards the electrode surface. The second one firstly allows a cDNA fixed on the electrode surface, followed by a helical binding with the aptamer. After involving the analyte, the aptamer is not dissociated with the cDNA, yielding a larger steric hindrance. These two methods provide decreased responses with the increase of analyte concentration. The only difference of the third method from the second one is the aptamer dissociated from cDNA after forming the aptamer–analyte conjugation. Steric resistance is reduced in this method and the electrochemical responses are positively correlated with the target concentration. Abnous et al.122 constructed a special aptasensor for monitoring of BPA (see Fig. 5). The aptamer and a short complementary sequence initially attached to the electrode surface, and subsequently bridged through poly T that was catalyzed by terminal deoxynucleotidyl transferase (TdT), forming a physical barrier against the redox couple (i.e., Fe (CN)63−/4−). Chemical events happened at the gold surface since the aptamer length extension reaction was not triggered after the analyte was involved.122
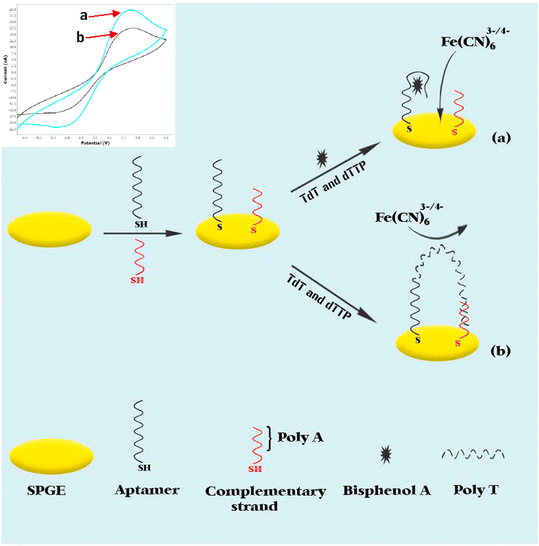 |
| Fig. 5 Schematic illustration of the electrochemical aptasensor for BPA detection based on nontarget-induced extension of aptamer length triggered by TdT (reprinted with the permission from ref. 121). | |
3.2.3 Molecularly imprinted polymer-based receptors. MIPs are a promising alternative of biorecognition elements that can exhibit strong specificity like antibodies, owing to the use of biocompatible polymers and organic functional reagents. In the past decade, more and more research groups focus their attention on studying of MIPs. A molecular imprinting process usually requires a specific monomer, cross-linker, and template molecule. The polymeric matrix is then built after a few cycles of polymerization activated by an initiator. Tailored cavities are subsequently obtained by the removal of template molecules. According to the cited papers in this review, functional monomers including pyrrole,123,156,159,160,171 1H-pyrrole-3-carboxylic acid (py-3-COOH),173 methacrylic acid (MAA),124,165,166 chitosan,157o-phenylenediamine (o-PD),167 acrylamide,111 3,4-ethylenedioxythiophene (EDOT),172 and 4-aminothiophenol (4-ATP or p-ATP)164 are often used. Some research groups prefer to use a comonomer to create the MIP, including MAA/4-ATP,161 3-aminopropyltriethoxysilane (APTES)/phenyltrimethoxysilane (PTMS),168 2-aminothiophenol (2-ATP)/4-ATP169 and o-phenylenediamine/o-toluidine.170 Ethylene glycol dimethacrylate (EGDMA) and azodiisobutyronitrile (AIBN) are common chemicals for a cross-linker and initiator, respectively.158,161,165,166 There is an exception reported in Venkatesh et al.,111 where the cross-linking and activation were conducted in the presence of N,N-methylene-bisacrylamide and tetramethylethylenediamine (TEMED), respectively. Zhang et al.168 applied tetraethoxysilane (TEOS) as a special linkage reagent (see Fig. 6). Chemicals such as methanol/acetic acid,156,158,159,161,165,168–170,172 ethanol/acetic acid,160,171 hydrochloric acid,157,164 and sodium hydrate mixed with ethanol173 are used to remove template molecules after the imprinted matrix was generated. For some photoelectrochemical MIPs, the template molecules are normally removed by water with high temperature annealing,156 ethanol,163 deionized water/ethanol,166 and sodium hydrate.167
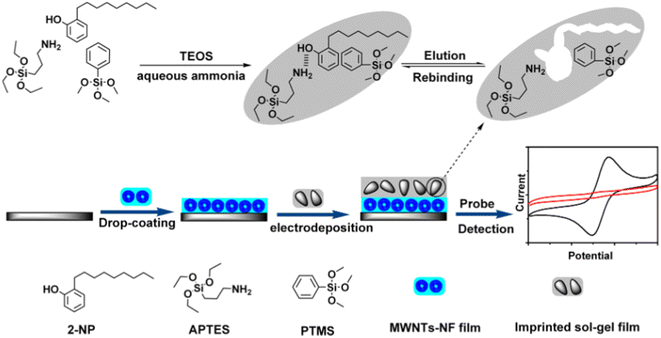 |
| Fig. 6 Schematic representation of the preparation procedure of MIP/sol–gel/MWNT-NF/GCE (reprinted with the permission from ref. 167). | |
3.2.4 Enzymatic and cyclodextrin-based receptors. Enzymes can also play the role of biorecognition element in electrochemical biosensors. A biological enzyme is a protein that shows strong selectivity and specificity towards target molecules. Enzymes can dramatically reduce the activation energy of chemical events to accelerate the reaction process, presenting excellent electrocatalytic nature. Common enzymes used for electrochemical detection of EDCs in water are tyrosinase (Tyr) and laccase (Lac). Tyr is a copper-containing enzyme mainly obtained from plant or animal tissues (e.g., mushroom). The primary function of Tyr is to catalyze the oxidation of tyrosine and control the production of melanin and other pigments. BPA determined using Tyr-based biosensors is normally subjected to a primary oxidation to form catechol, followed by a secondary oxidation of catechol to form o-quinone. The reduction of o-quinone forms an enzymatic reaction loop to regenerate catechol, yielding reductive currents that indicate the concentrations of BPA.125,177–179 Lac is different from Tyr as it belongs to a multi-copper-containing oxidase family. Lac can be obtained from various species of fungi, plants, insects, and bacteria.126 The oxidation in Lac-induced reactions usually transfers only one electron, while molecular oxygen or a redox probe often serves as an electron acceptor contributing to the reduction.176,181 Cyclodextrins are a class of barrel-like products naturally formed through the circular cross-linking of oligosaccharides. The resulting homopolymer always with a hexagonal, heptagonal, or octagonal structure depends on the number of glucose monomers incorporated, corresponding to α-, β-, and γ-cyclodextrin, respectively (see Fig. 7).127 Cyclodextrins have a general property of outer-hydrophilicity and inner-hydrophobicity that present adsorption onto target molecules to establish stable ‘host–guest’ inclusion complexes.128 Among them, β-cyclodextrin (β-CD) is the one that has been widely used in electrochemical biosensors for detection of EDCs in water.
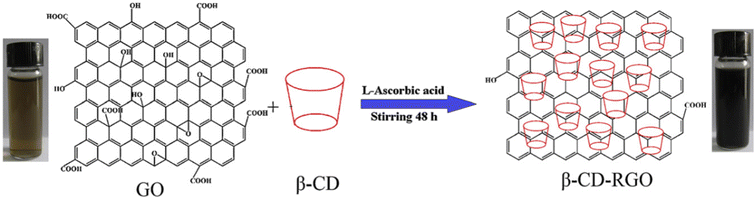 |
| Fig. 7 Illustration of the preparation procedure of β-CD-RGO (reprinted with the permission from ref. 126). | |
3.3 Detection of EDCs using electrochemical biosensors
In this section, detection results of EDCs in water using various electrochemical biosensors are summarized in several tables depending on different biorecognition elements applied: Table 2 for electrochemical immunosensors, Table 3 for electrochemical aptasensors, Table 4 for MIP-based electrochemical sensors, and Table 5 for enzymatic and β-CD-based electrochemical sensors.
Table 2 Electrochemical immunosensors for detection of EDCs in water
EDC |
Recognition biocomponent |
Detection scheme |
Electrode |
Electrode functional support from bottom to top |
Electrochemical technique |
Dynamic range (ng mL−1) |
LOD (pg mL−1) |
Real water sample analysis |
Ref. |
BPA |
mAb, pAb |
Sandwich |
GCE |
Gr/P(1,5DAN), α1BPA, BPA antigen, α2BPA, Gr-AgNPs |
SWV |
0.228–1.824 |
136.8 |
Drinking water |
129 |
TBBPA |
Ab |
Indirect competitive |
GCE |
MWCNTs/Au-TB, competitor; antigen, BSA, Ab1, analyte, Ab2 with label; MnO2/Pd |
Amperometry |
0–81 |
170 |
Pure water, tap water, pond water, and lake water |
130 |
TBBPA |
Ab |
Indirect competitive |
GCE |
MWCNTs, competitor; antigen, BSA, Ab1, analyte, Ab2 with label; AuPd–NH2–fMnO2 |
Amperometry |
0–243 |
100 |
Pure water, tap water, pond water, and lake water |
131 |
PCB 28 |
pAb |
Direct |
GCE |
AgNPs, PANI, GA |
SWV |
0.2–1.2 |
63 |
Tap water |
132 |
DMP |
Ab |
Direct competitive |
GCE |
AuPt-GS, anti-DMP, BSA, competitor; PtPb(tag)-BSA-DMP |
DPV |
1–1000 |
330 |
Tap water and lake water |
133 |
DBP |
Ab |
Indirect competitive |
GCE |
MWCNTs@GONRs, chitosan, competitor; antigen, Ab1, analyte, Ab2 with label; AuNPs |
EIS |
0.005–0.5 |
7000 |
Pure water, tap water, pond water, and river water |
134 |
E2 |
mAb |
Direct competitive |
Gold |
Mercaptoacetic acid, EDC/NHS, BSA, competitor; Cu2S-BSA-E2 |
SWV |
0.025–7.5 |
7.5 |
Water sample |
135 |
E2 |
Ab |
Direct |
GCE |
CoFe2O4/rGO, Au@Pd NRs, BSA |
Amperometry |
0.01–18 |
3.3 |
River water |
136 |
E2 |
mAb |
Direct |
Silver |
11-MUA, EDC/NHS |
EIS |
0.005–0.2 |
1 |
Water sample |
137 |
Table 3 Electrochemical aptasensors for detection of EDCs in water
EDC |
Recognition biocomponent |
Electrode |
Electrode functional support from bottom to top |
Electrochemical technique |
Dynamic range (ng mL−1) |
LOD (pg mL−1) |
Real water sample analysis |
Ref. |
BPA |
Thiolated aptamer |
GCE |
CNFs/AgNPs |
SWV |
0.0228–2.28 |
88.92 |
Real water sample |
138 |
BPA |
Aptamer |
GCE |
MWCNTs/Fe3O4/AuNPs-NH2/GA |
DPV |
0.228–136.8 |
68.4 |
Environmental water sample |
139 |
BPA |
Aptamer |
GCE |
PEI/PtNPs/MWCNTs-COOH/EDC/ssDNA2 |
DPV |
0.228–91.2 |
47.88 |
Environmental water sample |
140 |
BPA |
Thiolated aptamer |
GCE |
MWCNTs/Au/CuFe2O4-Pr-SH, MCH |
DPV |
0.0114–2.052 |
5.746 |
Mineral water |
141 |
BPA |
Thiolated aptamer |
Au/PET |
MB, MCH |
LSV |
0.001–100 |
0.4 |
Tap water |
142 |
BPA |
Thiolated aptamer |
Gold |
cDNA, MB, MCH |
CV |
0.0001–1 |
0.284 |
Drinking water |
143 |
BPA |
Aptamer |
GCE |
MWCNTs-COOH/EDC/NHS, NH2–Fe3O4 NPs/AuNPs, cDNA, SWCNTs |
DPV |
2.28 × 10−11–2.28 × 10−6 |
1.824 × 10−8 |
Lake water |
144 |
PCB 72 |
Thiolated aptamer |
Gold |
MB@CaCO3, PAH, EDTA |
DPV |
10−8–0.01 |
1 |
Tap water and lake water |
145 |
PCB 77 |
Thiolated aptamer |
Gold |
Fc |
DPV |
0.2–200 |
10 |
Tap water |
146 |
PCB 77 |
Aptamer |
Gold |
rGO/NiHCF NPs, BSA |
DPV |
0.001–0.1 |
0.22 |
Tap water and lake water |
147 |
PCB 77 |
Aptamer |
Gold |
Ag–Cu2O/rGO, thiolated HPDNA, MCH, biotin, SA-MB@CeO2/AuPt |
DPV |
0.0001–1 |
0.069 |
Tap water |
148 |
PCB 77 |
Aptamer |
Gold |
CoxP/NC, AuNPs, thiolated cDNA, MCH, AuNRs/MB |
DPV |
0.00001–100 |
0.059 |
Tap water |
149 |
PCB 77 |
Thiolated aptamer |
Gold |
RGO-AuNPs, Fc |
DPV |
10−6–10 |
0.0001 |
Tap water |
150 |
PCB 77 |
Thiolated aptamer |
BDD |
T-Au-NPs |
EIS |
2.92 × 10−7–2.92 × 10−3 |
9.344 × 10−5 |
Lake water |
151 |
PCB 77 |
Thiolated aptamer |
GCE |
MoS2-rGO/Thi/AuNPs, MCH |
DPV |
3 × 10−7–0.1 |
8×10−5 |
River water and tap water |
152 |
DBP |
Thiolated aptamer |
SPCE |
IL/GO, AuNPs, MCH, MB |
DPV |
0.00014–7 |
0.042 |
River water |
153 |
DEHP |
Aptamer |
Gold |
Thiolated CP, DNA junction, MCH, MB |
DPV |
0.1–5000 |
40 |
Tap water |
154 |
DEHP |
Aptamer |
Gold |
MCH |
EIS |
0.0076–2000 |
0.103 |
Tap water and lake water |
155 |
Table 4 Electrochemical MIP sensors for detection of EDCs in water
EDC |
Biorecognition component |
Electrode |
Electrode functional support from bottom to top |
Electrochemical technique |
Dynamic range (ng mL−1) |
LOD (pg mL−1) |
Real water sample analysis |
Ref. |
BPA |
MIP |
GCE |
GQDs |
DPV |
22.8–11 400 |
9120 |
Seawater and bottled water |
156 |
BPA |
MIP |
ABPE |
rGO |
Second-order derivative LSV |
1.824–4560 |
1368 |
Drinking water and beverages |
157 |
BPA |
MIP |
GCE |
GO/APTES |
DPV |
1.368–4560 |
684 |
Mineral water |
158 |
TBBPA |
MIP |
GCE |
MWCNTs-AuNPs |
DPV |
0.272–544 |
130.56 |
Seawater and tap water |
159 |
TBBPA |
MIP |
GCE |
rGO/NiNPs |
DPV |
0.272–5440 |
70.72 |
Tap water, rainwater, and lake water |
160 |
TBBPS |
MIP |
CPE |
AuNPs |
DPV |
0.0566–5.66 |
16.414 |
Tap water, drinking water, and lake water |
161 |
PCB 101 |
MIP |
FTO |
TiO2 NRs |
Photoelectrochemistry |
2.6 × 10−5–9.78 |
0.00326 |
River water |
162 |
PCB 101 |
MIP |
FTO |
Pd QDs@TiO2 NRs |
Photoelectrochemistry |
3.26 × 10−5–0.163 |
0.0163 |
Lake water |
163 |
DBP |
MIP |
BDD |
MWCNTs/AuNPs |
DPV |
2.78–2780 |
917.4 |
Lake water and tap water |
164 |
DBP |
MIP |
GCE |
MWCNTs/AuNPs |
CV |
0.1–10 000 |
5.09 |
Tap water |
165 |
DOP |
MIP |
ITO |
Cu3(BTC)2@Cu2O |
Photoelectrochemistry |
0.00975–39 |
3.5685 |
Bottled water |
166 |
DOP |
MIP |
ITO |
Bi2S3 |
Photoelectrochemistry |
0.000195–0.0273 |
0.039 |
Plastic bottled water, and rainwater |
167 |
2-NP |
MIP |
GCE |
MWCNTs-Nafion/sol–gel |
DPV |
44–79 200 |
13 200 |
Tap water and river water |
168 |
4-NP |
MIP |
Gold |
TiO2–NH2/AuNPs/Cysteamine |
Amperometry |
209–105 600 |
70 400 |
Tap water |
169 |
4-NP |
MIP |
GCE |
NGNRs-IL |
LSV |
8.8–1320 |
1760 |
Lake water, river water, and tap water |
170 |
4-NP |
MIP |
CE |
GP |
DPV |
0.01–10 |
3.5 |
Rainwater and lake water |
171 |
4-tert-OP |
MIP |
GCE |
GNRs |
LSV |
4.12–1648 |
206 |
River water and bottled water |
172 |
DES |
MIP |
GCE |
Ti3C2Tx/Cu2O NPs/CNT-CTAB |
DPV |
2.68–18 760 |
1608 |
Lake water |
173 |
Table 5 Electrochemical enzymatic and cyclodextrin sensors for detection of EDCs in water
EDC |
Biorecognition component |
Electrode |
Electrode functional support from bottom to top |
Electrochemical technique |
Dynamic range (ng mL−1) |
LOD (pg mL−1) |
Real water sample analysis |
Ref. |
BPA |
Lac |
GCE |
(3D-GN)/Cu/Fe3O4 |
DPV |
1641.6–4104 |
387 600 |
Tap water |
174 |
BPA |
Lac |
GCE |
rGO-Fe3O4 NPs/Chit95 |
Amperometry |
6–228 |
4104 |
Bottled water |
175 |
BPA |
Tyr |
SPCE |
Au leaf-like microstructures, Nafion |
CV |
114–11 400 |
17 556 |
Tap water, and mineral water |
176 |
BPA |
Tyr |
GCE |
BCNPs, Nafion |
Amperometry |
4.56–2280 |
725.04 |
Ground water |
177 |
BPA |
ML-Tyr |
GCE |
Mag-BCNPs-COOH, EDC/NHS |
Amperometry |
2.28–230.28 |
633.84 |
Environmental water |
178 |
BPA |
Tyr |
BDD |
MWCNTs-diazonium |
CV |
0.00228–22.8 |
2.28 |
River water |
179 |
4-NP |
Lac |
CPE |
CTAB |
Amperometry |
1100–66 000 |
200 |
Water sample |
180 |
BPA |
β-CD-SH |
Gold |
NPGL, MB |
SWV |
68.4–22 800 |
13 680 |
Tap water |
181 |
BPA |
β-CD |
SPCE |
MWCNTs |
LSV |
28.5–6840 |
3137.28 |
Lake water and tap water |
182 |
BPS |
β-CD |
GCE |
MWCNTs |
SWV |
125.135–15016.2 |
12513.5 |
Drinking water and tap water |
183 |
BPA |
PCD |
GCE |
GO, AuAgPt |
DPV |
BPA: 1.368–912 |
BPA: 456 |
River water |
184 |
BPS |
BPS: 2–2000 |
BPS: 667.5 |
PCB 77 |
β-CD-SH |
GCE |
AuNPs/GR, MB |
DPV |
14.6–2920 |
8176 |
Canal water and lake water |
185 |
DEHP |
β-CD |
GCE |
Graphene |
EIS |
780–7020 |
46 800 |
Wastewater |
186 |
DEHP |
β-CD |
GCE |
DAD/rGO |
EIS |
78–468 |
3900 |
River water |
187 |
4-NP |
β-CD |
GCE |
GNP/sol–gel, AuNPs |
CV |
2.2–220 |
660 |
Lake water |
188 |
3.3.1 Electrochemical immunosensors. Antibody–antigen conjugation was often used in electrochemical immunosensors for detection of EDCs in water (Table 2). Nguyen et al.129 fabricated an electrochemical immunosensor following a sandwich determination protocol. After the antigen conjugated with the monoclonal anti-BPA antibody (αBPA) on the electrode surface, polyclonal anti-BPA antibody (α2BPA) coated graphene-silver nanoparticles (Gr-AgNPs) were introduced to accomplish the sandwich-type biosensing. Under the SWV analysis, the immunosensor had a limit of detection (LOD) of 136.8 pg mL−1. However, the detection range lay only within 0.228–1.824 ng mL−1.130 Yakubu et al.130 created an indirect competitive immunosensor where the antigen was fixed on the transducer surface serving as the competitor to TBBPA. The competition was induced when the primary antibody (Ab1) and analyte molecules were involved. After washing off the TBBPA-bound Ab1, the remaining Ab1-antigen combination was successively recognized by the secondary antibody (Ab2) coupled to the MnO2/Pd label. Amperometric results presented a linear range of 0–81 ng mL−1, and a LOD of 170 pg mL−1. The test for stability was performed by measuring current responses of the biosensor every three days, and a recovery of 86.85% was obtained from the one stored at 4 °C for two weeks. The reproducibility assay was conducted on five electrodes and showed a small relative standard deviation (RSD) of 3.17%.130 From the same research group, the structure of the label was improved as AuPd decorated MnO2 nanoflowers (AuPd–NH2–fMnO2), followed by the identical biosensing method to reduce the LOD to 100 pg mL−1.131 Zhang et al.136 provided a direct competitive sensing platform in which the analyte (i.e., E2) and Cu2S-BSA labeled E2 competitively bound to the monoclonal antibody on the gold surface. The labeled E2-antibody composite was eventually measured reflecting the analyte concentration. A linear range of 0.025–7.5 ng mL−1 was achieved and the minimum detection for E2 was 7.5 pg mL−1.136 In contrast to the competition scheme, two papers focused on the electrochemical detection of E2 using direct antibody–antigen interaction. Zhang et al.136 prepared a label-free electrochemical immunosensor supported by the underlayer of spinel oxide-loaded rGO and non-biological enzyme (i.e., Au@Pd NRs). A wider dynamic range of 0.01–18 ng mL−1 and a lower LOD of 3.3 pg mL−1 were acquired using amperometric measurement.136 Based on this, Singh et al.137 further improved the LOD to 1 pg mL−1, using non-faradaic EIS.Apart from BPs and estrogens, antibody-based electrochemical biosensors were also frequently used for monitoring of PCBs and phthalate esters in water. Nevertheless, there are few comparative studies on a single EDC. Khesuoe et al.132 reported a direct immunosensor for PCB 28. However, cross-reactivity among the analyte, PCB 180, and benzyl chloride (BnCl) was noted because of their similar structures, leaving poor specificity. A direct competitive assay in between the target DMP and the PtPb tagged DMP (i.e., competitor) was conducted by Liu et al.133 on the surface of a GCE. The DPV result was compared with those obtained from chromatographic and SERS methods, evidencing a lower LOD of 330 pg mL−1. Liang et al.134 fabricated an impedimetric immunosensor for the determination of DBP relying on the mechanism of indirect competition. AuNPs enhanced Ab2 was added into the testing system after the removal of DBP-Ab1 complexes to amplify the electrochemical signals. A detection limit of 7000 pg mL−1 was achieved, which was ten times lower than that yielded by the conventional ELISA approach.134 A new class of biosensors has emerged which do not need separate labeling steps for their preparation and can provide rapid and in situ sensing in fields. In this regard an ultrasensitive immunosensor was fabricated by electropolymerization over a graphene screen printed electrode (SPE). The PANI/Gr-SPE-devices displayed linear responses to low molecular weight, poorly water soluble, estrogenic compounds, in EIS assays, from 0.0975 ng L−1 to 200 ng L−1 in water samples. This method was considered better compared with previous reports due to low detection limits of 0.043 pg L−1 for E1, 0.19 ng L−1 for E2 and 0.070 pg L−1 for EE2.31 Another label free impedimetric biosensor was developed by immobilizing both estrogen receptor-α and bovine serum albumin on gold electrode surfaces for detecting 17β-estradiol. The binding of the estrogen contaminant on the surface of the label free sensor increased the charge transfer resistance which was proven by EIS analysis The present biosensor gives a linear response (r2 = 0.992) for 17β-estradiol concentration from 1.0 × 10−13 to 1.0 × 10−9 M with a detection limit of 1.0 × 10−13 M (S/N = 3).32 In a similar study, ER-a was coupled onto the gold electrode through its 6-His tag and NTA-copper complex to prepare a label free biosensor for estrogen. After interaction of estradiol with ER-a, the biosensor presents a well-defined peak at +500 mV due to estradiol oxidation (E17 peak). These rapid and innovative sensors were used to detect estradiol from hospital effluents.94
3.3.2 Electrochemical aptasensors. Aptamer-based electrochemical biosensors have been widely reported in the past decade. Electrochemical aptasensors used for detection of EDCs in water are summarized in Table 3. Tsekeli et al.138 developed an electrochemical aptasensor for BPA. The electron transfer of the redox couple near the electrode surface was monitored using SWV. Weakened electrical signals were observed when the BPA concentration was increased due to the enlarged steric hindrance caused by the aptamer–antigen complex. The biosensor reached a LOD of 88.92 pg mL−1 and a good selectivity was verified by mixing BPA with other six interferents.138 Similar studies were also performed by Beiranvand et al.139 and Derikvandi et al.,140 where the biosensors exhibited a slightly broader detection range and improved LODs. MCH was sometimes introduced to the electrode surface after the functionalization of aptamers to avoid any non-specific adsorption. This principle was applied in the aptasensor provided by Baghayeri et al.,141 of which a well-improved LOD of 5.746 pg mL−1 was achieved. Thiolated aptamers were also directly immobilized on gold electrodes. In the presence of MCH, these gold-based aptasensors presented better performances like wider dynamic ranges (e.g., four or five orders of magnitude) and LODs below 1 pg mL−1.142,143 For ultrasensitive biosensing, Zhao et al.144 reported an aptasensor in which the electrochemical signal was significantly amplified as single-walled carbon nanotubes (SWCNTs) attached to the cDNA in lieu of the aptamer after BPA was introduced. Under the DPV measurement, the aptasensor offered a linear response from 2.28 × 10−11 to 2.28 × 10−6 ng mL−1, with an ultralow LOD of 1.824 × 10−8 pg mL−1.144Other than BPs, aptamer-based electrochemical biosensors used for the detection of PCBs (e.g., PCB 77) in a variety of water samples have also been reported. Utilizing a PCB 77 specific DNA sequence as the bioreceptor, Wu et al.146 developed an aptasensor with a resulting LOD of 10 pg mL−1. The proposed biosensor successfully detected PCB 77 in real tap water and displayed good selectivity to the analyte even in the presence of other PCB congeners involving PCB 81, PCB 126, PCB 169, and PCB 189. Moreover, the electrochemical biosensing only took 10 seconds, which was much faster than traditional GC methods of about 10 minutes.146 A lower LOD (i.e., 1 pg mL−1) was achieved by Fan et al.,147 because the nickel hexacyanoferrate nanoparticles (NiHCF NPs) exerted a synergistic effect with BSA on enhancing the aptasensor sensitivity. Some aptamers were immobilized on the electrode surface via their complementary parts (e.g., hairpin DNA148 and cDNA149), while the other ends of the chains were connected by a signal generator namely streptavidin (SA)-MB@CeO2/AuPt (ref. 148) and gold nanorods (AuNRs)@MB,149 respectively. Such signal generator-aptamer hybrids were prone to dissociate from the electrode surface and bind to the target molecules, leading to weakened signals. This process can significantly enlarge the range of detection and reduce the LOD but needs to prepare many functional components resulting in tedious experimental procedures. Another sensing mechanism was based on the spatial change of aptamers to shorten the distance between the signal probe (always attaching to the end of aptamers) and the electrode surface, thus improving the sensitivity. This method involved fewer electrode supports but exhibited even better electrochemical performance.149 Yuan et al.151 achieved an ultrasensitive determination of PCB 77 on the twice sputtered and twice annealed gold nanoparticle (T-Au-NPs) covered BBD electrode. Under faradaic EIS, visible differences can be observed in the Nyquist plot ranging from 2.92 × 10−7 to 2.92 × 10−3 ng mL−1 with a small standard deviation in each concentration, accompanied by an ultralow LOD of 9.344 × 10−5 pg mL−1.151 Another aptamer-induced biosensing in virtue of thionine was performed by Mohammadi et al.152 with similar ultrasensitive outcomes. The selectivity test was carried out by introducing structural analogs of PCB 77 as well as heavy metal ions and the ratio of DPV responses after introducing interferants was highly close to that of the control group (i.e., PCB 77 only).152 Han et al.145 constructed a special dendritic aptasensor used for the detection of PCB 72 in water. The aptamers were firstly allowed to grow on the skeleton of CaCO3 particles loaded with MB. Ethylenediaminetetraacetic acid (EDTA) was then used to dissolve the CaCO3 framework, leaving aptamer-based microcapsules. MB was finally released from the capsules after introducing the analyte. This biodevice possessed a LOD of 1 pg mL−1 and a broad dynamic range with a six-order-of-magnitude span (i.e., 10−8–10−2 ng mL−1).145
The detection of phthalate esters in water samples was sometimes reported using aptamer-based electrochemical biosensors, but mainly focused on DEHP. Chen et al.154 fabricated an aptasensor for DEHP based on the gold surface. The target-specific aptamers initially combined with the capture probe (CP). Subsequently, the analyte bound to the aptamers and formed a free composite, while the CP continuously combined with a DNA junction comprising of three hairpin DNA and a quarter sequence. The biosensor had a linear range of 0.1–5000 ng mL−1 and a LOD of 40 pg mL−1.154 A simple biosensor that only contained aptamers and a blocking agent (i.e., MCH) was made by Lu et al.,155 where the use of EIS resulted in a wider range of detection from 0.0076–2000 ng mL−1 and a lower LOD of 0.103 pg mL−1, further verifying the high reliability of impedimetric methods. The prepared biosensor also had an outstanding selectivity towards DEHP in a mixture of other nine phthalate esters.155 Contrary to the sensing principle of Wu et al.,150 decreased responses were observed by Gurudatt et al.,153 since the target molecules bound to the aptamers creating a larger distance between MB and the electrode surface. This biosensor realized an ultrasensitive determination of DBP in river water from South Korea.
3.3.3 Electrochemical MIP sensors. MIPs are another type of customarily used recognition element for the electrochemical detection EDCs in water (Table 4). Tan et al.156 created a MIP sensor for the monitoring of BPA and gained a detection range of 22.8–11
400 ng mL−1 with a not very low LOD of 9120 pg mL−1. Real water analysis was implemented by spiking BPA in seawater and bottled water with recoveries comparable to those of HPLC. In addition, the influence of MIP thickness on performance of the proposed sensor was also discussed in their group. Increasing the thickness enlarges the surface area of the polymeric matrix, leading to adequate room for cavity production. Nevertheless, excessive thickness will result in the difficulty on the removal of template molecules. Meanwhile, cavities created in the deep inner area make the analyte unable to bind effectively because of the high mass-transfer resistance. Therefore, the thickness of MIP should be in a reasonable range via controlling the cycle of polymerization.156 Deng et al.157 prepared a MIP sensor based on the ABPE electrode. Compared with Tan et al.,156 the sensor had a competitive dynamic range and a significantly reduced LOD of 1368 pg mL−1, under the measurement of SDLSV. Dadkhah et al.158 further lowered the LOD to 684 pg mL−1. The high specificity to BPA was derived from the amine groups of APTES attached to the surface of GO. Also, DPV results provided by the MIP sensor were found to be approximately five times larger than those of the non-imprinted polymer (NIP) sensor under the same conditions, demonstrating the usefulness of MIPs.158 Wu et al.159 and Chen et al.160 developed MIP sensors for the screening of TBBPA in water samples. Using the same electrochemical technique (i.e., DPV), a wider dynamic range as well as a lower detection limit was obtained by the latter group, probably owing to the modification of amine-terminated benzenediazonium (NBD) upon the GCE. Sarpong et al.161 successfully detected TBBPS in water samples using a dual-monomer polymerized MIP modified on CPE. However, the detection span was only from 0.0566 to 5.66 ng mL−1 (two orders of magnitude), and there is only one paper focusing on the detection of TBBPS in water using electrochemical MIP sensors, leaving the demand for more parallel research.Huang et al.169 applied amine-terminated TiO2 (TiO2–NH2) nanoparticles to cover the gold electrode, where the resulting surface was used to absorb AuNPs. A 4-nonylphenol (4-NP) imprinted polymer was then built on top of cysteamine self-assembled nanoparticles under polymerization with thioglycolic acid involved as a stabilizer. However, the detection results were not as low as expected. This is probably due to the use of TiO2 nanoparticles that are likely to display semiconductive properties but are not very conductive as carbon materials. Like Sarpong et al.,161 Pan et al.170 also prepared a MIP sensor for 4-NP based on two monomers. A highly conductive functional composite (i.e., NGNRs-IL) was placed in between the MIP and GCE, serving as an excellent path for electron transfer. Compared with Huang et al.,169 the LOD was decreased by an order of magnitude, 1760 pg mL−1. Chen et al.171 reported a simple MIP sensor for the sensitive detection of 4-NP based on a home-made CE. The amine-terminated graphene oxide was electrochemically reduced and covalently bound to the electrode acting as a conductive underlayer. DPV results exhibited a dynamic range of 0.01–10 ng mL−1 with a remarkably lowered minimal detection level of 3.5 pg mL−1.171 As an isomer of 4-NP, the monitoring of 2-nonylphenol (2-NP) in environmental water samples was manifested by Zhang et al.168 on a sol–gel MIP sensor, reaching a wide range of detection (i.e., 44–79
200 ng mL−1). However, the detection limit was still over ten thousand pg mL−1. Pan et al.172 constructed two MIP-based sensing devices for 4-tert-OP, one of which used EDOT as a monomer, the other used AuNPs-captured EDOT. The experimental results revealed that the MIP incorporating gold nanoparticles presented a higher sensitivity, wider detection window, and lower LOD than the one without gold nanoparticles.172
Additionally, there are also a few reports on the detection of PCBs, phthalate esters, and estrogens in water making use of electrochemical MIP sensors. Shi et al.162 developed a special MIP sensor, where the TiO2 sol was initially deposited on the FTO substrate via spin-coating and subjected to a hydrothermal process with template molecules (i.e., PCB 101) to generate cavity-embedded titanium dioxide nanorods (TiO2 NRs). In combination with the photoelectrochemical technique, the sensor was able to determine PCB 101 with an ultralow LOD of 0.00326 pg mL−1. Another similar work was also performed by the same group, in which the TiO2 NRs were further decorated with palladium quantum dots (Pd QDs) and yielded a comparable LOD.163 Based on these two studies, they demonstrated that photoelectrochemistry could enable a very broad detection. In the experiment performed by Wu et al.,164 DBP was determined by the imprinted cavities on a BDD electrode ranging from 2.78–2780 ng mL−1. The analyte was interfered with other structural analogs such as BPA, DMP, DEP, and DOP, to evaluate the selectivity. The peak current of DPV (RSD <3.5%) verified that the presence of interferants had a negligible effect on the electrochemical sensing of DBP.164 Wang et al.165 constructed a MIP sensing platform based on silica nanospheres. Equipped with the same functional supports as Wu et al.,164 this sensor exhibited an extremely wide detection range of 0.1–10
000 ng mL−1 and a lower LOD of 5.09 pg mL−1, which was because of the large surface area provided by nanospheres. The low efficiency of charge transfer upon silica was also claimed by the research group. The issue can be solved by adding more functional substances with good electrical conductivity on the electrode surface.165 MIP sensors developed on the ITO substrate were used to detect DOP in water in combination with photoelectrochemistry. A cuprous oxide–copper organic framework heterostructure (Cu3(BTC)2@Cu2O) and bismuth sulphide (Bi2S3) were applied by Gao et al.166 and Li et al.,167 respectively, to improve the photoelectrochemical responses. It is observed that the range of detection and LOD are mostly at ultralow concentration, suggesting again that photoelectrochemistry can achieve ultrasensitive determination for EDCs in water. Xia et al.173 introduced titanium carbide MXene composites (Ti3C2Tx), a type of novel 2D-material, to prepare an MIP sensor for the detection of DES in water. Cuprous oxide nanoparticles (Cu2O NPs) were adsorbed by the CTAB-CNT composite through the electrostatic effect and generated another characteristic peak in the DPV voltammogram, showing a proportional relationship with the peak of the analyte.173
3.3.4 Electrochemical enzymatic and cyclodextrin sensors. In Table 5, recent progress shows that enzyme-based biosensors are mostly used to detect BPs (e.g., BPA) in water. Lou et al.174 prepared a laccase-modified biosensor for BPA, where DPV was used to analyze the sensing results after different concentrations of BPA were introduced, offering a linear range of 1641.6–4104 ng mL−1 and a LOD of 387
600 pg mL−1. Furthermore, they also found that the pH of phosphate buffer saline (PBS) solution of 4.0 enables laccase to have the highest electrocatalytic activity.174 A similar enzymatic sensing platform was also reported by Fernandes et al.,175 displaying a wider detection range of 6–228 ng mL−1 and a two-order-of-magnitude reduced LOD of 4104 pg mL−1. The obvious improvement in detection performance was attributed to the high activity of laccase preserved by the good biocompatibility and unique spatial structure of chitosan.189 Moreover, laccase-based electrochemical biosensors can be also used for the determination of 4-NP in water. Liu et al.180 evaluated the influence of temperature (i.e., 5–40 °C) and pH (i.e., 4.0–7.0) on laccase. The results revealed that the enzyme showed the best catalytic ability at room temperature of 25 °C and pH of 5.5. The detection threshold of 4-NP was 200 pg mL−1 obtained under optimized conditions.180 In fact, electrochemical biosensors functionalized with tyrosinase as the biorecognition center are more common than the ones functionalized with laccase. For the detection of BPA, Inroga et al.176 developed an enzymatic biosensor where tyrosinase was deposited on the leaf-like gold microstructures. The results yielded by the biosensing device had a wider detection range of 114–11
400 ng mL−1 in comparison to those obtained by the abovementioned biosensors equipped with laccase. However, limited progress was made on LOD with a value of 17
556 pg mL−1.175 Liu et al.177 realized a LOD to an extent as low as 725.04 pg mL−1 on the BCNP coated electrode using the amperometric technique. He et al.178 invented a multi-layered enzymatic bioelectrode through the precipitation and cross-linking of tyrosinase, and acquired similar lower results (i.e., LOD of 633.84 pg mL−1). After the optimization, they learned that tyrosinase provided maximum electrochemical responses at 25 °C and pH of 7.0.178 Zehani et al.179 published an ultrasensitive tyrosinase-based biosensor for BPA, with a LOD of 2.28 pg mL−1 (see Fig. 8). The detection in the ultralow range (i.e., 0.00228–22.8 ng mL−1) could be interpreted as the small capacitive background current generated on the diazonium-modified BDD electrode, evidencing high applicability for trace level monitoring.190
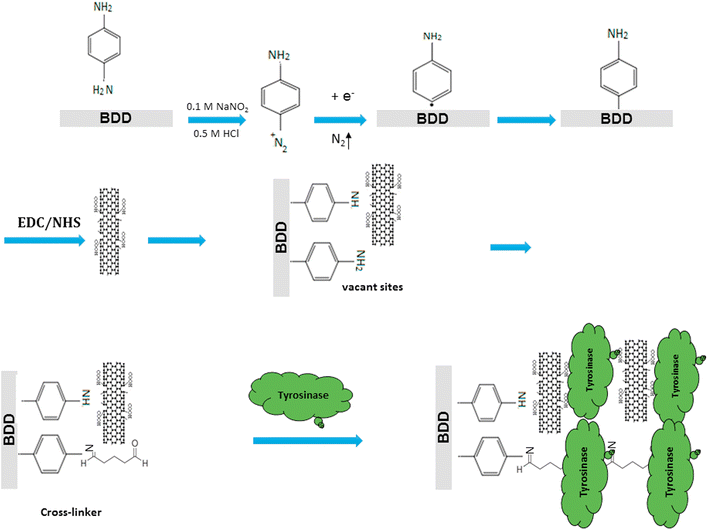 |
| Fig. 8 Schematic illustration of the functionalizing procedure and enzyme attachment on the boron-doped diamond (BDD) surface (reprinted with the permission from ref. 178). | |
Cyclodextrin-based (i.e., β-CD) electrochemical sensors were also used to screen BPs,181–183 PCBs,185 phthalate esters,186,187 and alkylphenols188 in water sources through the adsorption of hydrophobic chambers. Ye et al.184 applied a GCE modified with gold–silver–platinum ternary alloy nanocrystals (AuAgPt) to simultaneously recognize BPA and BPS using polymerized β-CD. The sensor provided LODs below 1000 pg mL−1 for these two BPs, along with satisfactory findings in practical applications.184 Su et al.188 elaborated a sensor for 4-NP, where β-CDs were mixed with a sol–gel resulting in a stable and bioactive composite, which reached a LOD of 660 pg mL−1. However, there are few parallel studies on the detection of a specific EDC. Also, most of the LODs reported in this class of sensors are not low enough (i.e., thousands to tens of thousands pg mL−1) compared with those obtained from the biosensors with antibodies, aptamers, and MIPs. These problems indicate that cyclodextrin-based sensors require more investigation to improve the electrochemical sensing performance. The stability of biological components is a major issue of biobased sensors. These challenges can be addressed using new class of nanozymes which will not only increase the shelf life but will also reduce the cost of sensing devices.
4. Current status and further development
Electrochemical biosensing of EDCs in water has been remarkably improved during the past decade. Research teams worldwide have made great efforts from the application of various biorecognition elements, electrode materials, and functional composites, to electrochemical techniques, to improve the ultra-sensitivity, high selectivity, long-term stability, and commercial availability of electrochemical biosensors.
Electrochemical immunosensors (i.e., antibody-based biosensors) are often used to determine EDCs. Competitive immunosensing provides higher sensitivity and accuracy compared with direct antibody–antigen binding protocols. Commonly employed labels like enzymes and metallic nanoparticles are usually modified on the antigen competitor in direct competitive biosensing or on the antibody in the indirect competitive one, reflecting different analyte concentrations. However, like sandwich-type biosensing, indirect competition requires more reagents and time-consuming preparation. Other widespread biodevices for EDCs are electrochemical aptasensors (i.e., aptamer-based biosensors). Specific ssDNA or RNA sequences are simply immobilized on an electrode surface as usual or via binding to cDNA chains to form double-helical structures. Steric hindrance upon redox couples increases after more aptamer–analyte complexes are formed. Recently, a novel CNT induced ‘signal on’ aptasensor has been reported.144 The aptamers dissociated from their cDNA chains when the analyte was introduced. Free ssDNA sequences preferred to encircle the target molecules while the complementary parts were subsequently connected with CNTs through π–π stacking, leading to amplified signals. In our opinion, this sensing method allows the analyte to be detected in a wider dynamic range coupled with a very low LOD, which is suitable for the ultrasensitive detection of EDCs in water. Electrodes functionalized with enzymes are also applied in electrochemical biosensing via electrocatalytic features. Apart from tyrosinase and laccase, Messaoud et al.191 fabricated a xanthine oxidase (XOD) based biosensor for the monitoring of BPA, with a LOD of 228 pg mL−1 obtained using the enzyme inhibition principle. Other recognition elements like MIPs and β-CDs that exhibit strong affinity towards EDCs have received tremendous attention. Tailored cavities are created after the polymerization of different monomers with template molecules, equipping MIP sensors with excellent reusability and stability. In addition, MIPs can be integrated with aptamers to produce super sensitive biosensors. Ensafi et al.123 reported an aptasensor where the p-63 sequences were embedded in the BPA imprinted polymeric matrix. The resulting biosensor reached a broad detection range from 1.14 × 10−7 to 1.14 × 10−3 ng mL−1 and an ultralow LOD of 1.824 × 10−5 pg mL−1. Liang et al.192 provided an electrochemical sensor decorated with reduced graphene oxide (RGO), PtPd bimetallic nanoparticles (PtPd NPs), and cationic pillar [5]arene (CP5) used as the receptor for BPA. Like β-cyclodextrin, CP5 has a barrel-shaped structure with a pentagonal opening, presenting similar ‘host–guest’ adsorption towards target analytes. A five orders of magnitude linear range (i.e., 2.28–2.28 × 105 ng mL−1) and a detection limit of 752.4 pg mL−1 were obtained after the DPV measurement. This device was finally utilized to successfully detect BPA in real water samples with good recoveries of 97.75–104.70%.186
Electrodes are the essential part in electrochemical biosensors. Carbon (e.g., carbon rod, glassy carbon, screen-printed carbon, and carbon paste) and gold (e.g., Au substrate, screen-printed Au, and sputtered Au) are still the main materials for electrode fabrication. The limited electrochemical performance of bare electrodes can be significantly improved through various surface modifications. A wide range of functional compounds or composites are usually deposited on electrode surfaces via covalent binding, non-covalent adsorption, van der Waals force, electrostatic effects, and hydrogen bonding, to prepare micro-bioenvironments catering for different bioreceptors. In general, functional supports for electrodes have the following capacities: 1) increasing the surface area to accommodate more active binding sites, 2) improving the electrode conductivity and electrocatalytic activity, and 3) enhancing film forming and electrode stability. Moreover, the miniaturization of electrochemical biosensing systems (i.e., electrode in combination with a data analyzer) is the direction for future development. Low cost, simple preparation, and portability are key factors in the commercialization of point-of-use electrochemical biosensors.
So far, a variety of electrochemical techniques have been used for biosensing of EDCs, including current varied with voltage (e.g., LSV, DPV, and SWV), current varied with time (e.g., amperometry and PEC), and impedance varied with frequency (e.g., EIS). Some research groups believe that non-faradaic EIS (i.e., capacitance as the typical response) is more promising than faradaic EIS (i.e., charge transfer resistance as the typical response) because of the higher sensitivity and simpler preparation (e.g., without the use of a redox couple), although faradaic EIS is still an active domain of investigation.137 Cheng et al.106 performed a capacitive aptasensing based on the ac electroosmotic (ACEO) effect. Under an alternating electric field, the ACEO flows enriched target molecules relying on the charge transfer in the electrical double layer (EDL) at the electrode–solution interface. Hence, their work integrated the preconcentration of the analyte with determination, which simplified the operation procedures. Interfacial capacitance was measured at a certain frequency and transient ac voltage, yielding an ultralow LOD of 1.329 × 10−5 pg mL−1 and a linear range of 2.28 × 10−8–2.28 × 10−4 ng mL−1.106 Additionally, ultrasensitive detection of EDCs in water using a PEC technique has been demonstrated by many groups. An issue that is of concern is that some materials with a large band gap may not be appropriate for the fabrication of PEC electrodes as light with higher energy (i.e., light with short wavelength) is often required to generate the photocurrent.193
In conclusion, the recent development of electrochemical biosensing of EDCs in water sources has been reviewed. The sensing capabilities regarding the five types of biosensors (i.e., immunosensors, aptasensors, MIP sensors, enzymatic sensors, and β-CD sensors) follow an order of aptasensors > immunosensors ≈ MIP sensors > enzymatic sensors ≈ β-CD sensors. Meanwhile, the recent advances on this field also expose some problems: 1) for immunosensing, only limited chemical variants in BPs, PCBs, phthalate esters, and estrogens are reported in the listed papers. More parallel research should be performed to increase the reliability of the result comparison. Examples for aptasensing enables comparable analyses on BPs (i.e., BPA) and PCBs (i.e., PCB 77). However, this group of biosensing exhibits the lowest detection diversity. In contrast, the detection diversity is improved in the cases of MIP and β-CD based electrochemical sensing, including BPS, TBBPS, PCB 101, DOP, DES, and alkylphenols (e.g., 2-NP, 4-NP, and 4-tert-OP). However, the same issue as less comparable studies on each type of EDC should be noted. EDC determination achieved by enzymatic biosensors has only a few papers and is mostly limited to the detection of BPA. Lu et al.125 used a copper-organic framework (CuMOF) modified enzymatic sensor to detect five BPs (i.e., BPA, BPB, BPE, BPF, and BPZ), expanding the range of EDC detection, to some extent. Nonetheless, the LODs needed to be further improved. Moreover, some studies claimed that the specificity of the prepared electrochemical biosensors was not good enough to differentiate the target compound from its structural analogs, especially for PCBs132 and alkylphenols,108 from which the detection result only reflected a total concentration of the mixture. Furthermore, other EDCs, like PBDEs, PFOA, DDT as well as a wider range of suspected EDCs have no report on water sample detection using electrochemical biosensors. There is still a long way to discover, study, and evaluate EDCs and their electrochemical screening methods. It is envisaged that more profound understanding, more mature detection techniques, and more standardized management of EDCs will offer stronger protection for human beings and natural environments.
Development of smart sensors for real-time monitoring of EDCs in environmental samples remains challenging due to the complex nature of wastewater samples. Moreover, the increasing demand for electrochemical sensors will influence the cost of electrode materials. Combined efforts are needed from scientists of various fields such as analytical chemistry, bioelectrochemistry, and environmental engineers for designing reliable and cost-effective sensors for field applications. Nanozyme based sensors will open new gateways for compact and portable devices.
Abbreviations
2-ATP | 2-Aminothiophenol |
2D | Two-dimensional |
2-NP | 2-Nonylphenol |
3D-GN | Three-dimensional graphene |
4-ATP | 4-Aminothiophenol |
4-NP | 4-Nonylphenol |
4-tert-OP | 4-tert-Octylphenol |
11-MUA | Mercaptoundecanoic acid |
Ab1 | Primary antibody |
Ab2 | Secondary antibody |
ABPE | Acetylene black paste electrodes |
Abs | Antibodies |
ACEO | AC electroosmotic |
AIBN | Azodiisobutyronitrile |
AgNPs | Silver nanoparticles |
AhR | Aryl hydrocarbon receptor |
APEOs | Alkylphenol ethoxylates |
APTES | 3-Aminopropyltriethoxysilane |
ARs | Androgen receptors |
AuAgPt | Gold–silver–platinum ternary alloy nanocrystals |
AuNPs | Gold nanoparticles |
AuNRs | Gold nanorod |
AuPd–NH2–fMnO2 | AuPd decorated MnO2 nanoflowers |
Au@Pd NRs | Gold/palladium nanorods |
AuPt | Gold/platinum |
BBP | Butyl benzyl phthalate |
BCNPs | Biochar nanoparticles |
BDD | Boron doped diamond |
Bi2S3 | Bismuth sulphide |
BnCl | Benzyl chloride |
BPs | Bisphenols |
BPA | Bisphenol A |
BPAF | Bisphenol AF |
BPAP | Bisphenol AP |
BPB | Bisphenol B |
BPE | Bisphenol E |
BPF | Bisphenol F |
BPS | Bisphenol S |
BPZ | Bisphenol Z |
BSA | Bovine serum albumin |
cDNA | Complementary DNA |
CE | Carbon electrode |
Chit | Chitosan |
CNFs | Carbon nanofibers |
CNTs | Carbon nanotubes |
CoxP/NC | Cobalt phosphide nanoparticles embedded in three-dimensional carbon microspheres |
CP | Capture probe |
CP5 | Cationic pillar [5]arene |
CPE | Carbon paste electrode |
CTAB | Cetyltrimethylammonium bromide |
Cu3(BTC)2@Cu2O | Cuprous oxide–copper organic framework heterostructure |
CuFe2O4-Pr-SH | Thiol-functionalized copper magnetic nanoparticles |
CuMOF | Copper-organic framework |
Cu2O NPs | Cuprous oxide nanoparticles |
CV | Cyclic voltammetry |
DAD1 | 10-Diaminodecane |
DALY | Disability-adjusted life year |
DBP | Dibutyl phthalate |
DDT | Dichlorodiphenyltrichloroethane |
DEHP | Di(2-ethylhexyl)phthalate |
DEP | Diethyl phthalate |
DES | Diethylstilbestrol |
DIDP | Di-isodecyl phthalate |
DINP | Di-isononyl phthalate |
DMP | Dimethyl phthalates |
DNA | Deoxyribonucleic acid |
DNOP | Di(n-octyl)phthalate |
DPV | Differential pulsed voltammetry |
DOP | Dioctyl phthalate |
E1 | Estrone |
E2 | (17β-) estradiol |
E3 | Estriol |
E4 | Estetrol |
EDC | Ethyl(dimethylamino propyl)carbodiimide |
EDC/NHS | Ethyl(dimethylamino propyl)carbodiimide/N-hydroxysuccinimide |
EDCs | Endocrine disrupting chemicals |
EDL | Electrical double layer |
EDOT | 3,4-Ethylenedioxythiophene |
EDTA | Ethylenediaminetetraacetic acid |
EE2 | 17α-Ethinylestradiol |
EGDMA | Ethylene glycol dimethacrylate |
EGFET | Extended gate field effect transistor |
EIS | Electrochemical impedance spectroscopy |
ELISA | Enzyme-linked immunosorbent assay |
ERKs | Extracellular signal-regulated kinases |
ERRγ | Estrogen related receptor gamma |
ERs | Estrogen receptors |
EU | European Union |
Fc | Ferrocene |
FET | Field-effect transistors |
FTO | Fluorine-doped tin oxide |
GA | Glutaraldehyde |
GCE | Glassy carbon electrode |
GC/MS | Gas chromatography coupled to mass spectrometry |
GNP | Graphene nanoplatelets |
GNRs | Graphene nanoribbons |
GO | Graphene oxide |
GONRs/MWCNTs | Graphene oxide nanoribbons/multi-walled carbon nanotubes |
GQDs | Graphene quantum dots |
GR or Gr | Graphene |
Gr–AgNPs | graphene–silver nanoparticles |
GRs | Glucocorticoid receptors |
H2N–Fe3O4/Au NPs | Amino-functionalized magnetite and gold nanoparticles |
HDSW | Heavily doped silicon wafer |
HPDNA | Hairpin DNA |
HPLC | High-performance liquid chromatography |
HRP | Horseradish peroxidase |
IARC | International Agency for Research on Cancer |
ILs | Ionic liquids |
ITO | Indium tin oxide |
Lac | Laccase |
LC-MS | Liquid chromatography coupled to mass spectroscopy |
LOD | Limit of detection |
LOQ | Limit of quantification |
LSV | Linear sweep voltammetry |
MAA | Methacrylic acid |
mAbs | Monoclonal antibodies |
MAC | Maximum acceptable concentration |
Mag | Magnetic |
MB | Methylene blue |
MCH | 6-Mercapto-1-hexanol |
MCL | Maximum contaminant level |
MDH | Minnesota Department of Health |
mERs | Membrane estrogen receptors |
MIPs | Molecular imprinted polymers |
ML | Multi-layered |
MOF | Metal–organic framework |
MS | Mass spectroscopy |
MW | Molecular weight |
MWCNTs | Multi-walled carbon nanotubes |
NBD | Amine-terminated benzenediazonium |
NERRA | Non-equilibrium rapid replacement aptamer |
nERs | Nuclear estrogen receptors |
NGNRs | Nitrogen-doped graphene nanoribbons |
NiHCF NPs | Nickel hexacyanoferrate nanoparticles |
NiNPs | Nickel nanoparticles |
NIP | Non-imprinted polymer |
NPEs | Nonylphenol ethoxylates |
NPGL | Nanoporous gold leaf |
NPs | Nanoparticles or nonylphenols |
o-PD | o-Phenylenediamine |
OPs | Octylphenols |
P(1,5-DAN) | Poly(1,5-diaminonaphthalene) |
pAbs | Polyclonal antibodies |
PAEs | Phthalic acid esters |
PAH | Poly(allylamine hydrochloride) |
PAHs | Polycyclic aromatic hydrocarbons |
PANI | Polyaniline |
PBDEs | Polybrominated diphenyl ethers |
PBS | Phosphate buffer saline |
PCBs | Polychlorinated biphenyls |
PCD | β-Cyclodextrin polymer |
PCDDs | Polychlorinated dibenzo dioxins |
PCDFs | Polychlorinated dibenzo furans |
PdQDs | Palladium quantum dots |
PEC | Photoelectrochemistry |
PEI | Polyethyleneimine |
PET | Polyethylene terephthalate |
PFOA | Perfluorooctanoic acid |
PI | Polyimide |
PPARγ | Peroxisome proliferator-activated receptor γ |
PTMS | Phenyltrimethoxysilane |
PtNPs | Platinum nanoparticles |
PtPd NPs | PtPd bimetallic nanoparticles |
PVC | Poly(vinyl chloride) |
py-3-COOH | 1H-Pyrrole-3-carboxylic acid |
QDs | Quantum dots |
rGO or RGO | Reduced graphene oxide |
RNA | Ribonucleic acid |
RSD | Relative standard deviation |
SA | Streptavidin |
SAMs | Self-assembled monolayers |
SDLSV | Second-order derivative linear sweep voltammetry |
SELEX | Systematic evolution of ligands by exponential enrichment |
SERS | Surface enhanced Raman spectroscopy |
SPCE | Screen printed carbon electrode |
ssDNA | Single-stranded DNA |
SWCNT | Single-walled carbon nanotube |
SWV | Square wave voltammetry |
T-Au-NPs | Twice sputtered and twice annealed gold nanoparticles |
TB | Toluidine blue |
TBBPA | Tetrabromobisphenol A |
TBBPS | Tetrabromobisphenol S |
TCBPA | Tetrachlorobisphenol A |
TdT | Terminal deoxynucleotidyl transferase |
TEMED | Tetramethylethylenediamine |
TEOS | Tetraethoxysilane |
Thi | Thionine |
Ti3C2Tx | Titanium carbide MXene composites |
TiO2-NH2 | Amine-terminated TiO2 |
TiO2 NRs | Titanium dioxide nanorods |
TRs | Thyroid receptors |
Tyr | Tyrosinase |
USEPA | United States Environmental Protection Agency |
USFDA | United States Food and Drug Administration |
WFD | Water Framework Directive |
WHO | World Health Organization |
WWTPs | Wastewater treatment plants |
XOD | Xanthine oxidase |
ZEN | Zearalenone |
Z w | Warburg diffusion |
αBPA | Monoclonal anti-BPA antibody |
α2BPA | Polyclonal anti-BPA antibody |
β-CD | β-Cyclodextrin |
β-CD-SH | Thiolated β-cyclodextrin |
Conflicts of interest
There are no conflicts to declare.
References
- L. Mansouri, C. Tizaoui, S. U. Geissen and L. Bousselmi, A comparative study on ozone, hydrogen peroxide and UV based advanced oxidation processes for efficient removal of diethyl phthalate in water, J. Hazard. Mater., 2019, 363, 401–411 CrossRef PubMed.
- E. Diamanti-Kandarakis, J. P. Bourguignon, L. C. Giudice, R. Hauser, G. S. Prins, A. M. Soto, R. T. Zoeller and A. C. Gore, Endocrine-disrupting chemicals, an Endocrine Society scientific statement, Endocr. Rev., 2009, 30(4), 293–342 CrossRef PubMed.
- T. F. T. Omar, A. Ahmad, A. Z. Aris and F. M. Yusoff, Endocrine disrupting compounds (EDCs) in environmental matrices, Review of analytical strategies for pharmaceuticals, estrogenic hormones, and alkylphenol compounds, TrAC, Trends Anal. Chem., 2016, 85, 241–259 CrossRef.
- V. Scognamiglio, A. Antonacci, L. Patrolecco, M. D. Lambreva, S. C. Litescu, S. A. Ghuge and G. Rea, Analytical tools monitoring endocrine disrupting chemicals, TrAC, Trends Anal. Chem., 2016, 80, 555–567 CrossRef.
- S. Rodriguez-Mozaz, M. P. Marco, M. J. Lopez de Alda and D. Barceló, Biosensors for environmental monitoring of endocrine disruptors, a review article, Anal. Bioanal. Chem., 2004, 378(3), 588–598 CrossRef PubMed.
- N. Jaffrezic-Renault, J. Kou, D. Tan and Z. Guo, New trends in the electrochemical detection of endocrine disruptors in complex media, Anal. Bioanal. Chem., 2020, 412(24), 5913–5923 CrossRef CAS PubMed.
- A. Azzouz, S. K. Kailasa, P. Kumar, E. Ballesteros and K. H. Kim, Advances in functional nanomaterial-based electrochemical techniques for screening of endocrine disrupting chemicals in various sample matrices, TrAC, Trends Anal. Chem., 2019, 113, 256–279 CrossRef CAS.
- L. E. Sofen and A. L. Furst, Perspective—electrochemical sensors to monitor endocrine disrupting pollutants, J. Electrochem. Soc., 2019, 167(3), 037524 CrossRef.
- F. Acconcia, V. Pallottini and M. Marino, Molecular mechanisms of action of BPA, Dose-Response, 2015, 13(4), 1559325815610582 CrossRef PubMed.
- X. Niu, W. Yang, G. Wang, J. Ren, H. Guo and J. Gao, A novel electrochemical sensor of bisphenol A based on stacked graphene nanofibers/gold nanoparticles composite modified glassy carbon electrode, Electrochim. Acta, 2013, 98, 167–175 CrossRef CAS.
- M. Ferguson, I. L. Schmidt and W. G. Pyle, Bisphenol S rapidly depresses heart function through estrogen receptor-β and decreases phospholamban phosphorylation in a sex-dependent manner, Sci. Rep., 2019, 9(1), 1–12 CrossRef CAS PubMed.
- Q. Wang, D. Zhang, L. Yang and L. Zhang, Constructed ILs@ hollow porous spherical Ni-loaded CdFe2O4 modified electrode for highly sensitive simultaneous electrochemical analysis of bisphenols, Sens. Actuators, B, 2017, 246, 800–808 CrossRef CAS.
- X. Xiao, J. Li, T. Yu, L. Zhou, X. Fan, H. Xiao, Y. Wang, L. Yang, J. Lv, X. Jia and Z. Zhang, Bisphenol AP is anti-estrogenic and may cause adverse effects at low doses relevant to human exposure, Environ. Pollut., 2018, 242, 1625–1632 CrossRef CAS PubMed.
- J. Moreman, O. Lee, M. Trznadel, A. David, T. Kudoh and C. R. Tyler, Acute toxicity, teratogenic, and estrogenic effects of bisphenol A and its alternative replacements bisphenol S, bisphenol F, and bisphenol AF in zebrafish embryo-larvae, Environ. Sci. Technol., 2017, 51(21), 12796–12805 CrossRef CAS.
- J. Shi, Z. Jiao, S. Zheng, M. Li, J. Zhang, Y. Feng, J. Yin and B. Shao, Long-term effects of bisphenol AF (BPAF) on hormonal balance and genes of hypothalamus-pituitary-gonad axis and liver of zebrafish (Danio rerio), and the impact on offspring, Chemosphere, 2015, 128, 252–257 CrossRef CAS PubMed.
- K. E. Pelch, Y. Li, L. Perera, K. A. Thayer and K. S. Korach, Characterization of estrogenic and androgenic activities for bisphenol A-like chemicals (BPs), in vitro estrogen and androgen receptors transcriptional activation, gene regulation, and binding profiles, Toxicol. Sci., 2019, 172(1), 23–37 CrossRef CAS.
- K. R. Beck, T. J. Sommer, D. Schuster and A. Odermatt, Evaluation of tetrabromobisphenol A effects on human glucocorticoid and androgen receptors, A comparison of results from human-with yeast-based in vitro assays, Toxicology, 2016, 370, 70–77 CrossRef CAS.
- V. Delfosse, M. Grimaldi, A. Le Maire, W. Bourguet and P. Balaguer, Nuclear receptor profiling of bisphenol-A and its halogenated analogues, Vitam. Horm., 2014, 94, 229–251 CAS.
- S. Kitamura, T. Suzuki, S. Sanoh, R. Kohta, N. Jinno, K. Sugihara, S. Yoshihara, N. Fujimoto, H. Watanabe and S. Ohta, Comparative study of the endocrine-disrupting activity of bisphenol A and 19 related compounds, Toxicol. Sci., 2005, 84(2), 249–259 CrossRef CAS PubMed.
- B. Lei, Q. Tang, S. Sun, X. Zhang, Y. Huang and L. Xu, Insight into the mechanism of tetrachlorobisphenol A (TCBPA)-induced proliferation of breast cancer cells by GPER-mediated signaling pathways, Environ. Pollut., 2021, 275, 116636 CrossRef CAS PubMed.
- A. Barańska, A. Woźniak, K. Mokra and J. Michałowicz, Genotoxic Mechanism of Action of TBBPA, TBBPS and Selected Bromophenols in Human Peripheral Blood Mononuclear Cells, Front. Immunol., 2022, 1617 Search PubMed.
- A. Liu, Z. Shen, Y. Tian, R. Shi, Y. Liu, Z. Zhao and M. Xian, Thin-layer chromatography coupled with high performance liquid chromatography for determining tetrabromobisphenol A/S and their derivatives in soils, J. Chromatogr. A, 2017, 1526, 151–156 CrossRef CAS.
- Q. Huang, Y. Chen, L. Lin, Y. Liu, Y. Chi, Y. Lin, G. Ye, H. Zhu and S. Dong, Different effects of bisphenol a and its halogenated derivatives on the reproduction and development of Oryzias melastigma under environmentally relevant doses, Sci. Total Environ., 2017, 595, 752–758 CrossRef CAS.
- M. R. Bell, Endocrine-disrupting actions of PCBs on brain development and social and reproductive behaviors, Curr. Opin. Pharmacol., 2014, 19, 134–144 CrossRef CAS.
- A. J. Baars, M. I. Bakker, R. A. Baumann, P. E. Boon, J. I. Freijer, L. A. P. Hoogenboom, R. Hoogerbrugge, J. D. van Klaveren, A. K. D. Liem, W. A. Traag and J. de Vries, Dioxins, dioxin-like PCBs and non-dioxin-like PCBs in foodstuffs, occurrence and dietary intake in The Netherlands, Toxicol. Lett., 2004, 151(1), 51–61 CrossRef CAS.
- S. Alsefri, T. Balbaied and E. Moore, Electrochemical Development of an Immunosensor for Detection Polychlorinated biphenyls (PCBs) for Environmental Analysis, Chemosensors, 2021, 9(11), 307 CrossRef CAS.
- M. D. Erickson and R. G. Kaley, Applications of polychlorinated biphenyls, Environ. Sci. Pollut. Res., 2011, 18(2), 135–151 CrossRef CAS PubMed.
- B. Lauby-Secretan, D. Loomis, Y. Grosse, F. El Ghissassi, V. Bouvard, L. B. Tallaa, N. Guha, R. Baan, H. Mattock, K. Straif and WHO Iarc Working Group, Carcinogenicity of polychlorinated biphenyls and polybrominated biphenyls, Lancet Oncol., 2013, 14(4), 287–288 CrossRef CAS.
- A. A. Moahammadi, S. S. H. Davarani, M. Jafari and A. Mehdinia, Preparation and evaluation of a new solid-phase microextraction fiber based on polythionine for analysis of phthalate esters in aqueous samples, J. Iran. Chem. Soc., 2021, 18(2), 385–391 CrossRef CAS.
- Y. Wang and H. Qian, Phthalates and their impacts on human health, Healthcare, 2021, 9, 603 CrossRef.
- A. Priac, N. M. Crini, C. Druart, S. Gavoille, C. Bradu, C. Lagarrigue, G. Torri, P. Winterton and G. Crini, Alkylphenol and alkylphenol polyethoxylates in water and wastewater, a review of options for their elimination, Arabian J. Chem., 2017, 10, S3749–S3773 CrossRef CAS.
- H. Barton, W. M. B. Filho, S. Consuegra, L. Francis, C. Tizaoui, R. S. Conlan and S. R. Teixeira, Ultrasensitive environmental assessment of xeno-estrogens in water samples using label-free graphene immunosensors, Anal. Biochem., 2018, 548, 102–108 CrossRef CAS.
- B. K. Kim, J. Li, J. E. Im, K. S. Ahn, T. S. Park, S. I. Cho, Y. R. Kim and W. Y. Lee, Impedometric estrogen biosensor based on estrogen receptor alpha-immobilized gold electrode, J. Electroanal. Chem., 2012, 671, 106–111 CrossRef CAS.
- B. Winid, Environmental Threats of Natural Water Contamination with Polybrominated Diphenyl Ethers (PBDEs), Pol. J. Environ. Stud., 2015, 24(1), 47–55 CrossRef CAS PubMed.
- L. G. Costa and G. Giordano, Is decabromodiphenyl ether (BDE-209) a developmental neurotoxicant?, Neurotoxicology, 2011, 32(1), 9–24 CrossRef CAS.
- G. B. Post, P. D. Cohn and K. R. Cooper, Perfluorooctanoic acid (PFOA), an emerging drinking water contaminant, a critical review of recent literature, Environ. Res., 2012, 116, 93–117 CrossRef CAS PubMed.
- W. Nicole, PFOA and cancer in a highly exposed community, new findings from the C8 science panel, Environ. Health Perspect., 2013, 121(11–12), A340 Search PubMed.
- A. M. Soto and C. Sonnenschein, DDT, endocrine disruption and breast cancer, Nat. Rev. Endocrinol., 2015, 11(9), 507–508 CrossRef CAS.
- S. Safe, Polychlorinated biphenyls (PCBs), dibenzo-p-dioxins (PCDDs), dibenzofurans (PCDFs), and related compounds, environmental and mechanistic considerations which support the development of toxic equivalency factors (TEFs), Crit. Rev. Toxicol., 1990, 21(1), 51–88 CrossRef CAS PubMed.
- H. I. A. Shafy and M. S. M. Mansour, A review on polycyclic aromatic hydrocarbons, source, environmental impact, effect on human health and remediation, Egypt. J. Pet., 2016, 25(1), 107–123 CrossRef.
- J. Liu and T. Applegate, Zearalenone (ZEN) in livestock and poultry, dose, toxicokinetics, toxicity and estrogenicity, Toxins, 2020, 12(6), 377 CrossRef CAS PubMed.
- A. Nasri, A. Mezni, P. A. Lafon, A. Wahbi, N. Cubedo, P. Clair, A. H. Harrath, H. Beyrem, M. Rossel and V. Perrier, Ethinylestradiol (EE2) residues from birth control pills impair nervous system development and swimming behavior of zebrafish larvae, Sci. Total Environ., 2021, 770, 145272 CrossRef CAS.
- S. L. Schneider and H. W. Lim, Review of environmental effects of oxybenzone and other sunscreen active ingredients, J. Am. Acad. Dermatol., 2019, 80(1), 266–271 CrossRef CAS.
- E. Scheuer and E. Warshaw, Sunscreen allergy, a review of epidemiology, clinical characteristics, and responsible allergens, Dermatitis, 2006, 17(1), 3–11 CrossRef PubMed.
- X. M. Zhang, M. Nakagawa, K. Kawai and K. Kawai, Erythema-multiforme-like eruption following photoallergic contact dermatitis from oxybenzone, Contact Dermatitis, 1998, 38(1), 43–44 CrossRef.
- Z. Lin, L. Wang, Y. Jia, Y. Zhang, Q. Dong and C. Huang, A study on environmental bisphenol A pollution in plastics industry areas, Water, Air, Soil Pollut., 2017, 228(3), 1–9 Search PubMed.
- S. M. Arnold, K. E. Clark, C. A. Staples, G. M. Klecka, S. S. Dimond, N. Caspers and S. G. Hentges, Relevance of drinking water as a source of human exposure to bisphenol A, J. Exposure Sci. Environ. Epidemiol., 2013, 23(2), 137–144 CrossRef.
- J. E. Biles, T. P. McNeal and T. H. Begley, Determination of bisphenol A migrating from epoxy
can coatings to infant formula liquid concentrates, J. Agric. Food Chem., 1997, 45(12), 4697–4700 CrossRef.
- P. Jing, X. Zhang, Z. Wu, L. Bao, Y. Xu, C. Liang and W. Cao, Electrochemical sensing of bisphenol A by graphene-1-butyl-3-methylimidazolium hexafluorophosphate modified electrode, Talanta, 2015, 141, 41–46 CrossRef PubMed.
- E. Yamazaki, N. Yamashita, S. Taniyasu, J. Lam, P. K. Lam, H. B. Moon, Y. Jeong, P. Kannan, H. Achyuthan, N. Munuswamy and K. Kannan, Bisphenol A and other bisphenol analogues including BPS and BPF in surface water samples from Japan, China, Korea and India, Ecotoxicol. Environ. Saf., 2015, 122, 565–572 CrossRef CAS PubMed.
- Y. Liu, S. Zhang, N. Song, R. Guo, M. Chen, D. Mai, Z. Yan, Z. Han and J. Chen, Occurrence, distribution and sources of bisphenol analogues in a shallow Chinese freshwater lake (Taihu Lake), Implications for ecological and human health risk, Sci. Total Environ., 2017, 599, 1090–1098 CrossRef.
- J. Liu, L. Zhang, G. Lu, R. Jiang, Z. Yan and Y. Li, Occurrence, toxicity and ecological risk of Bisphenol A analogues in aquatic environment–A review, Ecotoxicol. Environ. Saf., 2021, 208, 111481 CrossRef CAS.
- D. W. Bench, PCBs, mining, and water pollution, United States Environmental Protection Agency, Region VIII, 1999 Search PubMed.
- I. Ahmad, J. Weng, A. J. Stromberg, J. Z. Hilt and T. D. Dziubla, Fluorescence based detection of polychlorinated biphenyls (PCBs) in water using hydrophobic interactions, Analyst, 2019, 144(2), 677–684 RSC.
- IPEN and G. Beagle, China chemical safety case study, PCBs pollution in Ziyang, Sichuan Province, 2004 Search PubMed.
- M. Abtahi, S. Dobaradaran, M. Torabbeigi, S. Jorfi, R. Gholamnia, A. Koolivand, H. Darabi, A. Kavousi and R. Saeedi, Health risk of phthalates in water environment, occurrence in water resources, bottled water, and tap water, and burden of disease from exposure through drinking water in Tehran, Iran, Environ. Res., 2019, 173, 469–479 CrossRef CAS.
- Phthalates Drinking water, Minnesota Department of Health, 2013, https://www.state.mn.us Search PubMed.
- D. Tu, J. T. Garza and G. L. Coté, A SERS aptasensor for sensitive and selective detection of bis (2-ethylhexyl) phthalate, RSC Adv., 2019, 9(5), 2618–2625 RSC.
- S. Ghosh and M. Sahu, Phthalate pollution and remediation strategies, a review, J. Hazard. Mater. Adv., 2022, 6, 100065 CrossRef.
- R. Renner, European bans on surfactant trigger transatlantic debate, Environ. Sci. Technol., 1997, 31(7), 316A–320A CrossRef PubMed.
- C. Tyler, S. Jobling and J. P. Sumpter, Endocrine disruption in wildlife, a critical review of the evidence, Crit. Rev. Toxicol., 1998, 28(4), 319–361 CrossRef.
- C. Uğuz, M. İşcan and İ. Togan, Alkylphenols in the environment and their adverse effects on living organisms, Kocatepe Vet. J., 2009, 2(1), 49–58 Search PubMed.
- Directive 2008/105/EC of the European Parliament and of the Council on environmental quality standards in the field of water policy, amending and subsequently repealing Council Directives 82/176/EEC, 83/513/EEC, 84/156/EEC, 84/491/EEC, 86/280/EEC and amending Directive 2000/60/EC of the European Parliament and of the Council, Off. J. Eur. Communities: Legis., 2008, L 348/84 Search PubMed.
- National Recommended Water Quality Criteria – Aquatic Life Criteria Table, EPA, 2015, https://www.epa.gov/wqc/national-recommended-water-quality-criteria-aquatic-life-criteria-table Search PubMed.
- K. Lei, C. Y. Lin, Y. Zhu, W. Chen, H. Y. Pan, Z. Sun, A. Sweetman, Q. Zhang and M. C. He, Estrogens in municipal wastewater and receiving waters in the Beijing-Tianjin-Hebei region, China, occurrence and risk assessment of mixtures, J. Hazard. Mater., 2020, 389, 121891 CrossRef PubMed.
- C. Wedekind, Fish populations surviving estrogen pollution, BMC Biol., 2014, 12(1), 1–3 CrossRef PubMed.
- E. Nazari and F. Suja, Effects of 17β-estradiol (E2) on aqueous organisms and its treatment problem, a review, Rev. Environ. Health, 2016, 31(4), 465–491 Search PubMed.
- Y. Lind, P. O. Darnerud, S. Atuma, M. Aune, W. Becker, R. Bjerselius, S. Cnattingius and A. Glynn, Polybrominated diphenyl ethers in breast milk from Uppsala County, Sweden, Environ. Res., 2003, 93(2), 186–194 CrossRef PubMed.
- Study, Banned Since 2004, Toxic Flame Retardants Persist in U.S. Newborns, https://www.ewg.org/news-insights/news/study-banned-2004-toxic-flame-retardants-persist-us-newborns Search PubMed.
- EU-banned flame retardants still present in consumer products and found in humans, https://chemycal.com/news/84914007-4e61-4a85-83f9-f84e39ce9fc9/EU-banned_flame_retardants_still_present_in_consumer_products_and_found_in_humans_ Search PubMed.
- K. S. Betts, Perfluoroalkyl acids, what is the evidence telling us?, Environ. Health Perspect., 2007, 115(5), A250–A256 CrossRef PubMed.
- N. Yamashita, K. Kannan, S. Taniyasu, Y. Horii, G. Petrick and T. Gamo, A global survey of perfluorinated acids in oceans, Mar. Pollut. Bull., 2005, 51(8–12), 658–668 CrossRef PubMed.
- R. Renner, Aerosols complicate PFOA picture, Environ. Sci. Technol., 2008, 42(11), 3908 CrossRef PubMed.
- Guideline Technical Document-Perfluorooctanoic Acid (PFOA), https://www.canada.ca/en/health-canada/services/publications/healthy-living/guidelines-canadian-drinking-water-quality-technical-document-perfluorooctanoic-acid.html Search PubMed.
- S. Zhang, Q. Kang, H. Peng, M. Ding, F. Zhao, Y. Zhou, Z. Dong, H. Zhang, M. Yang, S. Tao and J. Hu, Relationship between perfluorooctanoate and perfluorooctane sulfonate blood concentrations in the general population and routine drinking water exposure, Environ. Int., 2019, 126, 54–60 CrossRef PubMed.
- J. M. Ochoa-Rivero, A. V. R. Fierro, M. D. R. P. Pérez, F. J. Z. D. de la Serna, L. B. Casarrubias, I. Salmerón, H. R. Arias and B. A. R. Gutierrez, Levels and distribution of pollutants in the waters of an aquatic ecosystem in Northern Mexico, Int. J. Environ. Res. Public Health, 2017, 14(5), 456 CrossRef.
- DDT and its Derivatives in Drinking-water, Background document for development of WHO Guidelines for Drinking-water Quality, WHO/SDE/WSH/03.04/89, https://cdn.who.int/media/docs/default-source/wash-documents/wash-chemicals/ddt.pdf?sfvrsn=1d7f988e_4 Search PubMed.
- W. Xia, Y. Li, Y. Wan, T. Chen, J. Wei, Y. Lin and S. Xu, Electrochemical biosensor for estrogenic substance using lipid bilayers modified by Au nanoparticles, Biosens. Bioelectron., 2010, 25(10), 2253–2258 CrossRef PubMed.
- S. Sakamoto, W. Putalun, S. Vimolmangkang, W. Phoolcharoen, Y. Shoyama, H. Tanaka and S. Morimoto, Enzyme-linked immunosorbent assay for the quantitative/qualitative analysis of plant secondary metabolites, J. Nat. Med., 2018, 72(1), 32–42 CrossRef PubMed.
- D. Kim, H. J. Lim, Y. G. Ahn, B. Chua and A. Son, Development of non-equilibrium rapid replacement aptamer assay for ultra-fast detection of phthalic acid esters, Talanta, 2020, 219, 121216 CrossRef PubMed.
- H. J. Lim, A. R. Kim, M. Y. Yoon, Y. You, B. Chua and A. Son, Development of quantum dot aptasensor and its portable analyzer for the detection of di-2-ethylhexyl phthalate, Biosens. Bioelectron., 2018, 121, 1–9 CrossRef PubMed.
- J. Feng, Y. Tao, X. Shen, H. Jin, T. Zhou, Y. Zhou, L. Hu, D. Luo, S. Mei and Y. I. Lee, Highly sensitive and selective fluorescent sensor for tetrabromobisphenol-A in electronic waste samples using molecularly imprinted polymer coated quantum dots, Microchem. J., 2019, 144, 93–101 CrossRef.
- Y. Wang, J. Bai, B. Huo, S. Yuan, M. Zhang, X. Sun, Y. Peng, S. Li, J. Wang, B. Ning and Z. Gao, Upconversion fluorescent aptasensor for polychlorinated biphenyls detection based on nicking endonuclease and hybridization chain reaction dual-amplification strategy, Anal. Chem., 2018, 90(16), 9936–9942 CrossRef PubMed.
- S. Xu, H. Yuan, S. Chen, A. Xu, J. Wang and L. Wu, Selection of DNA aptamers against polychlorinated biphenyls as potential biorecognition elements for environmental analysis, Anal. Biochem., 2012, 423(2), 195–201 CrossRef PubMed.
- M. Tang, Y. Wu, D. Deng, J. Wei, J. Zhang, D. Yang and G. Li, Development of an optical fiber immunosensor for the rapid and sensitive detection of phthalate esters, Sens. Actuators, B, 2018, 258, 304–312 CrossRef.
- L. Brossa, R. M. Marcé, F. Borrull and E. Pocurull, Determination of endocrine-disrupting compounds in water samples by on-line solid-phase extraction–programmed-temperature vaporisation–gas chromatography–mass spectrometry, J. Chromatogr. A, 2003, 998(1–2), 41–50 CrossRef PubMed.
- X. Ma, Q. Li and D. Yuan, Determination of endocrine-disrupting compounds in water by carbon nanotubes solid-phase microextraction fiber coupled online with high performance liquid chromatography, Talanta, 2011, 85(4), 2212–2217 CrossRef PubMed.
- R. Jeannot, H. Sabik, E. Sauvard, T. Dagnac and K. Dohrendorf, Determination of endocrine-disrupting compounds in environmental samples using gas and liquid chromatography with mass spectrometry, J. Chromatogr. A, 2002, 974(1–2), 143–159 CrossRef PubMed.
- A. M. Gurban, L. Rotariu, V. E. Marinescu and C. Bala, Determination of Xenoestrogenic Compounds Using a Nanostructured Biosensing Device, Electroanalysis, 2012, 24(12), 2371–2379 CrossRef.
- T. M. Nahir, R. A. Clark and E. F. Bowden, Linear-sweep voltammetry of irreversible electron transfer in surface-confined species using the Marcus theory, Anal. Chem., 1994, 66(15), 2595–2598 CrossRef.
- Q. He, Y. Tian, Y. Wu, J. Liu, G. Li, P. Deng and D. Chen, Facile and ultrasensitive determination of 4-nitrophenol based on acetylene black paste and graphene hybrid electrode, Nanomaterials, 2019, 9(3), 429 CrossRef PubMed.
- Y. Chen, Y. Li, D. Deng, H. He, X. Yan, Z. Wang, C. Fan and L. Luo, Effective immobilization of Au nanoparticles on TiO2 loaded graphene for a novel sandwich-type immunosensor, Biosens. Bioelectron., 2018, 102, 301–306 CrossRef PubMed.
- W. Zhang, M. B. Dixon, C. Saint, K. S. Teng and H. Furumai, Electrochemical biosensing of Algal toxins in water, The current state-of-the-art, ACS Sens., 2018, 3(7), 1233–1245 CrossRef PubMed.
- W. Zhang, L. Wang, Y. Yang, P. Gaskin and K. S. Teng, Recent advances on electrochemical sensors for the detection of organic disinfection by-products in water, ACS Sens., 2019, 4, 1138–1150 CrossRef PubMed.
- Z. Guo, N. Zine, P. Balaguer, A. Zhang, P. Namour, F. Lagarde and N. J. Renault, Electrochemical Estrogen Receptor α based Biosensor for Label-Free Detection of Estradiol, Electroanalysis, 2013, 25(7), 1765–1772 CrossRef.
- F. A. Settle, Handbook of Instrumental Techniques for Analytical Chemistry, Prentice Hall, 1st edn, 1997 Search PubMed.
- S. Samavat, J. S. Lloyd, L. O'Dea, W. Zhang, E. Preedy, S. Luzio and K. S. Teng, Uniform sensing layer of immiscible enzyme-mediator compounds developed via a spray aerosol mixing technique towards low cost minimally invasive microneedle continuous glucose monitoring devices, Biosens. Bioelectron., 2018, 118, 224–230 CrossRef.
- Basics of Electrochemical Impedance Spectroscopy, https://www.gamry.com/application-notes/EIS/basics-of-electrochemical-impedance-spectroscopy/ Search PubMed.
- S. M. Park and J. S. Yoo, Peer reviewed, electrochemical impedance spectroscopy for better electrochemical measurements, Anal. Chem., 2003, 75(21), 455A–461A CrossRef PubMed.
- L. Wang, W. Zhang, S. Samavat, D. Deganello and K. S. Teng, Vertically aligned graphene biosensor prepared by photonic annealing for ultra-sensitive biosensor, ACS Appl. Mater. Interfaces, 2020, 12(31), 35328–35336 CrossRef.
- W. Zhang, B. Jia and H. Furumai, Fabrication of graphene film composite electrochemical biosensor as a pre-screening algal toxin detection tool in the event of water contamination, Sci. Rep., 2018, 8, 10686 CrossRef.
- W. Zhang, C. Han, B. Jia, C. Saint, M. Nadagouda, P. Falaras, L. Sygellou, V. Vogiazi and D. Dionysiou, A 3D graphene-based biosensor as an early microcystin-LR screening tool in sources of drinking water supply, Electrochim. Acta, 2017, 236, 319–327 CrossRef.
- J. W. Chen and H. T. Tien, A comparative study between a two-compartment cell and the standard electrode system, J. Electroanal. Chem. Interfacial Electrochem., 1988, 253(3), 491–497 CrossRef.
- W. E. Van der Linden and J. W. Dieker, Glassy carbon as electrode material in electro-analytical chemistry, Anal. Chim. Acta, 1980, 119(1), 1–24 CrossRef CAS.
- M. H. Piao, H. B. Noh, M. A. Rahman, M. S. Won and Y. B. Shim, Label-Free Detection of Bisphenol A Using a Potentiometric Immunosensor, Electroanalysis, 2008, 20(1), 30–37 CrossRef.
- C. Cheng, S. Wang, J. Wu, Y. Yu, R. Li, S. Eda, J. Chen, G. Feng, B. Lawrie and A. Hu, Bisphenol a sensors on polyimide fabricated by laser direct writing for onsite river water monitoring at attomolar concentration, ACS Appl. Mater. Interfaces, 2016, 8(28), 17784–17792 CrossRef PubMed.
- S. Alsefri, T. Balbaied and E. Moore, Electrochemical Development of an Immunosensor for Detection Polychlorinated biphenyls (PCBs) for Environmental Analysis, Chemosensors, 2021, 9(11), 307 CrossRef.
- G. A. Evtugyn, S. A. Eremin, R. P. Shaljamova, A. R. Ismagilova and H. C. Budnikov, Amperometric immunosensor for nonylphenol determination based on peroxidase indicating reaction, Biosens. Bioelectron., 2006, 22(1), 56–62 CrossRef.
- M. L. S. Benuzzi, J. Raba, G. J. S. Illia, R. J. Schneider and G. A. Messina, Novel electrochemical paper-based immunocapture assay for the quantitative determination of ethinylestradiol in water samples, Anal. Chem., 2018, 90(6), 4104–4111 CrossRef PubMed.
- N. Belkhamssa, J. P. da Costa, C. I. Justino, P. S. Santos, S. Cardoso, A. C. Duarte, T. R. Santos and M. Ksibi, Development of an electrochemical biosensor for alkylphenol detection, Talanta, 2016, 158, 30–34 CrossRef PubMed.
- S. Venkatesh, C. C. Yeung, Q. J. Sun, J. Zhuang, T. Li, R. K. Li and V. A. Roy, Selective and sensitive onsite detection of phthalates in common solvents, Sens. Actuators, B, 2018, 259, 650–657 CrossRef CAS.
- Y. Han, R. Zhang, C. Dong, F. Cheng and Y. Guo, Sensitive electrochemical sensor for nitrite ions based on rose-like AuNPs/MoS2/graphene composite, Biosens. Bioelectron., 2019, 142, 111529 CrossRef CAS.
- M. Sajid, MXenes, are they emerging materials for analytical chemistry applications? a review, Anal. Chim. Acta, 2021, 1143, 267–280 CrossRef PubMed.
- X. Zhang, G. Shen, Y. Shen, D. Yin and C. Zhang, Direct immobilization of antibodies on a new polymer film for fabricating an electrochemical impedance immunosensor, Anal. Biochem., 2015, 485, 81–85 CrossRef PubMed.
- F. Mollarasouli, S. Kurbanoglu and S. A. Ozkan, The role of electrochemical immunosensors in clinical analysis, Biosensors, 2019, 9(3), 86 CrossRef PubMed.
- N. S. Lipman, L. R. Jackson, L. J. Trudel and F. W. Garcia, Monoclonal versus polyclonal antibodies, distinguishing characteristics, applications, and information resources, ILAR J., 2005, 46(3), 258–268 CrossRef PubMed.
- B. S. He and J. W. Li, Synthesis of gold nanocubes/PEI-wrinkled CoSe2 nanomaterials and its application in electrochemical immunosensors for detection of dipropyl phthalate, Rare Met., 2021, 40(5), 1099–1109 CrossRef.
- S. Loisel, M. Ohresser, M. Pallardy, D. Daydé, C. Berthou, G. Cartron and H. Watier, Relevance, advantages and limitations of animal models used in the development of monoclonal antibodies for cancer treatment, Crit. Rev. Oncol. Hematol., 2007, 62(1), 34–42 CrossRef PubMed.
- W. Xu, Y. Qing, S. Chen, J. Chen, Z. Qin, J. Qiu and C. Li, Electrochemical indirect competitive immunoassay for ultrasensitive detection of zearalenone based on a glassy carbon electrode modified with carboxylated multi-walled carbon nanotubes and chitosan, Microchim. Acta, 2017, 184(9), 3339–3347 CrossRef.
- Y. Lu, M. Li, M. Ding, G. Liu, Y. Zhang and S. Wang, Detection of bisphenol-A using electrochemical immunosensor, Comparison between competition and displacement format assay, J. Electroanal. Chem., 2016, 779, 34–38 CrossRef.
- W. Zhong, Y. Pu, W. Tan, J. Liu, J. Liao, B. Liu, K. Chen, B. Yu, Y. Hu, Y. Deng, J. Zhang and H. Liu, Identification and application of an aptamer targeting papillary thyroid carcinoma using tissue-SELEX, Anal. Chem., 2019, 91(13), 8289–8297 CrossRef PubMed.
- K. Abnous, N. M. Danesh, M. Ramezani, M. Alibolandi and S. M. Taghdisi, A novel electrochemical sensor for bisphenol A detection based on nontarget-induced extension of aptamer length and formation of a physical barrier, Biosens. Bioelectron., 2018, 119, 204–208 CrossRef PubMed.
- A. A. Ensafi, M. Amini and B. Rezaei, Molecularly imprinted electrochemical aptasensor for the attomolar detection of bisphenol A, Microchim. Acta, 2018, 185(5), 1–7 CrossRef PubMed.
- Z. Zhang, L. Luo, R. Cai and H. Chen, A sensitive and selective molecularly imprinted sensor combined with magnetic molecularly imprinted solid phase extraction for determination of dibutyl phthalate, Biosens. Bioelectron., 2013, 49, 367–373 CrossRef PubMed.
- X. Lu, X. Wang, L. Wu, L. Wu, L. Dhanjai, Y. Fu and J. Chen Gao, Response characteristics of bisphenols on a metal–organic framework-based tyrosinase nanosensor, ACS Appl. Mater. Interfaces, 2016, 8(25), 16533–16539 CrossRef PubMed.
- L. Arregui, M. Ayala, X. G. Gil, G. G. Soto, C. E. H. Luna, M. H. de Los Santos, L. Levin, A. R. Domínguez, D. R. Martínez, M. C. N. Saparrat, M. A. T. Roldán and N. A. V. Cruz, Laccases, structure, function, and potential application in water bioremediation, Microb. Cell Fact., 2019, 18(1), 1–33 CrossRef PubMed.
- D. Lu, S. Lin, L. Wang, X. Shi, C. Wang and Y. Zhang, Synthesis of cyclodextrin-reduced graphene oxide hybrid nanosheets for sensitivity enhanced electrochemical determination of diethylstilbestrol, Electrochim. Acta, 2012, 85, 131–138 CrossRef.
- H. Liu, C. Liu, X. Yang, S. Zeng, Y. Xiong and W. Xu, Uniformly sized β-cyclodextrin molecularly imprinted microspheres prepared by a novel surface imprinting technique for ursolic acid, Anal. Chim. Acta, 2008, 628(1), 87–94 CrossRef.
- H. L. Nguyen, V. A. Nguyen, D. T. Bui, N. T. Pham, Q. P. Do, D. T. Nguyen, H. H. Cao and T. D. Lam, Graphene Decorated with Silver Nanoparticles as Electrocatalytic Labels in Non-Enzymatic Bisphenol-A Immunosensor, J. Cluster Sci., 2022, 33, 2277–2285 CrossRef.
- S. Yakubu, B. Jia, Y. Guo, Y. Zou, N. Song, J. Xiao, K. Liang, Y. Bu and Z. Zhang, Indirect competitive-structured electrochemical immunosensor for tetrabromobisphenol A sensing using CTAB-MnO2 nanosheet hybrid as a label for signal amplification, Anal. Bioanal. Chem., 2021, 413(16), 4217–4226 CrossRef.
- S. Yakubu, J. Xiao, J. Gu, J. Cheng, J. Wang, X. Li and Z. Zhang, A competitive electrochemical immunosensor based on bimetallic nanoparticle decorated nanoflower-like MnO2 for enhanced peroxidase-like activity and sensitive detection of Tetrabromobisphenol A, Sens. Actuators, B, 2020, 325, 128909 CrossRef.
- M. P. Khesuoe, F. O. Okumu and M. C. Matoetoe, Development of a silver functionalised polyaniline electrochemical immunosensor for polychlorinated biphenyls, Anal. Methods, 2016, 8(39), 7087–7095 RSC.
- Y. Liu, S. Zhang, X. Ren, Y. Wang, L. Yan, Q. Wei and B. Du, Synthesis of PtPb hollow nanoparticles and their application in an electrochemical immunosensor as signal tags for detection of dimethyl phthalate, RSC Adv., 2015, 5(71), 57346–57353 RSC.
- Y. R. Liang, Z. M. Zhang, Z. J. Liu, K. Wang, X. Y. Wu, K. Zeng, H. Meng and Z. Zhang, A highly sensitive signal-amplified gold nanoparticle-based electrochemical immunosensor for dibutyl phthalate detection, Biosens. Bioelectron., 2017, 91, 199–202 CrossRef.
- S. Zhang, Y. Wang, Y. Zhang, T. Yan, L. Yan, Q. Wei and B. Du, An ultrasensitive electrochemical immunosensor for determination of estradiol using coralloid Cu 2 S nanostructures as labels, RSC Adv., 2015, 5(9), 6512–6517 RSC.
- Y. Zhang, J. Li, Z. Wang, H. Ma, D. Wu, Q. Cheng and Q. Wei, Label-free electrochemical immunosensor based on enhanced signal amplification between Au@ Pd and CoFe2O4/graphene nanohybrid, Sci. Rep., 2016, 6(1), 1–8 CrossRef PubMed.
- A. C. Singh, G. Bacher and S. Bhand, A label free immunosensor for ultrasensitive detection of 17β-Estradiol in water, Electrochim. Acta, 2017, 232, 30–37 CrossRef.
- T. R. Tsekeli, L. Tshwenya, T. I. Sebokolodi, T. Ndlovu and O. A. Arotiba, An electrochemical aptamer biosensor for bisphenol A on a carbon nanofibre-silver nanoparticle immobilisation platform, Electroanalysis, 2021, 33(9), 2053–2061 CrossRef.
- S. Beiranvand and A. Azadbakht, Electrochemical switching with a DNA aptamer-based electrochemical sensor, Mater. Sci. Eng., C, 2017, 76, 925–933 CrossRef PubMed.
- Z. Derikvandi, A. R. Abbasi, M. Roushani, Z. Derikvand and A. Azadbakht, Design of ultrasensitive bisphenol A–aptamer based on platinum nanoparticles loading to polyethyleneimine-functionalized carbon nanotubes, Anal. Biochem., 2016, 512, 47–57 CrossRef.
- M. Baghayeri, R. Ansari, M. Nodehi, I. Razavipanah and H. Veisi, Label-free electrochemical bisphenol A aptasensor based on designing and fabrication of a magnetic gold nanocomposite, Electroanalysis, 2018, 30(9), 2160–2166 CrossRef.
- Z. Yu, Y. Luan, H. Li, W. Wang, X. Wang and Q. Zhang, A disposable electrochemical aptasensor using single-stranded DNA–methylene blue complex as signal-amplification platform for sensitive sensing of bisphenol A, Sens. Actuators, B, 2019, 284, 73–80 CrossRef.
- F. Xue, J. Wu, H. Chu, Z. Mei, Y. Ye, J. Liu, R. Zhang, C. Peng, L. Zhang and W. Chen, Electrochemical aptasensor for the determination of bisphenol A in drinking water, Microchim. Acta, 2013, 180(1), 109–115 CrossRef.
- Z. Zhao, J. Zheng, E. P. Nguyen, D. Tao, J. Cheng, H. Pan, L. Zhang, N. J. Renault and Z. Guo, A novel SWCNT-amplified “signal-on” electrochemical aptasensor for the determination of trace level of bisphenol A in human serum and lake water, Microchim. Acta, 2020, 187(9), 1–11 CrossRef.
- T. Han, S. Wang, F. Sheng, S. Wang, T. Dai, X. Zhang and G. Wang, Target triggered ultrasensitive electrochemical polychlorinated biphenyl aptasensor based on DNA microcapsules and nonlinear hybridization chain reaction, Analyst, 2020, 145(10), 3598–3604 RSC.
- L. Wu, P. Qi, X. Fu, H. Liu, J. Li, Q. Wang and H. Fan, A novel electrochemical PCB77-binding DNA aptamer biosensor for selective detection of PCB77, J. Electroanal. Chem., 2016, 771, 45–49 CrossRef CAS.
- L. Fan, G. Wang, W. Liang, W. Yan, Y. Guo, S. Shuang, C. Dong and Y. Bi, Label-free and highly selective electrochemical aptasensor for detection of PCBs based on nickel hexacyanoferrate nanoparticles/reduced graphene oxides hybrids, Biosens. Bioelectron., 2019, 145, 111728 CrossRef CAS.
- B. Zhang, J. He, P. Tian, L. Lv, H. Zhu, L. Xie, X. Liu and B. He, Ultrasensitive Electrochemical Aptasensor Based on Ag-Cu2O/rGO and CeO2/AuPt Nanocomposites for PCB77 Detection, J. Electron. Mater., 2022, 51, 3831–3842 CrossRef.
- P. Tian, B. Zhang, L. Lv, L. Xie, H. Chen and B. He, An electrochemical aptasensor-based Co x P-decorated porous carbon microspheres and AuNRs labelled methylene blue as signal labels for the sensitive detection of PCB77, Anal. Methods, 2020, 12(37), 4579–4587 RSC.
- L. Wu, X. Lu, X. Fu, L. Wu and H. Liu, Gold nanoparticles dotted reduction graphene oxide nanocomposite based electrochemical aptasensor for selective, rapid, sensitive and congener-specific PCB77 detection, Sci. Rep., 2017, 7(1), 1–7 CrossRef PubMed.
- X. Yuan, Z. Jiang, Q. Wang, N. Gao, H. Li and Y. Ma, Polychlorinated Biphenyl Electrochemical Aptasensor Based on a Diamond–Gold Nanocomposite to Realize a Sub-Femtomolar Detection Limit, ACS Omega, 2020, 5(35), 22402–22410 CrossRef PubMed.
- A. Mohammadi, E. H. Bafrooei, M. M. Foroughi and M. Mohammadi, Electrochemical aptasensor for ultrasensitive detection of PCB77 using thionine-functionalized MoS2-rGO nanohybrid, Microchem. J., 2020, 155, 104747 CrossRef.
- N. G. Gurudatt, K. Lee, W. Heo and H. I. Jung, Simple ultrasensitive electrochemical detection of DBP plasticizer for the risk assessment of the South Korean River water, Analyst, 2022, 147, 3525–3533 RSC.
- Q. Chen, M. Du and X. Xu, A label-free and selective electrochemical aptasensor for ultrasensitive detection of Di (2-ethylhexyl) phthalate based on self-assembled DNA nanostructure amplification, J. Electroanal. Chem., 2022, 914, 116300 CrossRef CAS.
- Q. Lu, X. Liu, J. Hou, Q. Yuan, Y. Li and S. Chen, Selection of aptamers specific for DEHP based on ssDNA library immobilized SELEX and development of electrochemical impedance spectroscopy aptasensor, Molecules, 2020, 25(3), 747 CrossRef CAS PubMed.
- F. Tan, L. Cong, X. Li, Q. Zhao, H. Zhao, X. Quan and J. Chen, An electrochemical sensor based on molecularly imprinted polypyrrole/graphene quantum dots composite for detection of bisphenol A in water samples, Sens. Actuators, B, 2016, 233, 599–606 CrossRef CAS.
- P. Deng, Z. Xu and Y. Kuang, Electrochemical determination of bisphenol A in plastic bottled drinking water and canned beverages using a molecularly imprinted chitosan–graphene composite film modified electrode, Food Chem., 2014, 157, 490–497 CrossRef CAS PubMed.
- S. Dadkhah, E. Ziaei, A. Mehdinia, T. B. Kayyal and A. Jabbari, A glassy carbon electrode modified with amino-functionalized graphene oxide and molecularly imprinted polymer for electrochemical sensing of bisphenol A, Microchim. Acta, 2016, 183(6), 1933–1941 CrossRef CAS.
- M. Wu, X. Wang, J. Shan, H. Zhou, Y. Shi, M. Li and L. Liu, Sensitive and selective electrochemical sensor based on molecularly imprinted polypyrrole hybrid nanocomposites for tetrabromobisphenol A detection, Anal. Lett., 2019, 52(16), 2506–2523 CrossRef CAS.
- H. Chen, Z. Zhang, R. Cai, W. Rao and F. Long, Molecularly imprinted electrochemical sensor based on nickel nanoparticles-graphene nanocomposites modified electrode for determination of tetrabromobisphenol A, Electrochim. Acta, 2014, 117, 385–392 CrossRef CAS.
- K. A. Sarpong, K. Zhang, Y. Luan, Y. Cao and W. Xu, Development and application of a novel electrochemical sensor based on AuNPS and difunctional monomer-MIPs for the selective determination of Tetrabromobisphenol-S in water samples, Microchem. J., 2020, 154, 104526 CrossRef CAS.
- H. Shi, J. Zhao, Y. Wang and G. Zhao, A highly selective and picomolar level photoelectrochemical sensor for PCB 101 detection in environmental water samples, Biosens. Bioelectron., 2016, 81, 503–509 CrossRef CAS.
- H. Shi, Y. Wang, J. Zhao, X. Huang and G. Zhao, Cathodic photoelectrochemical detection of PCB101 in environmental samples with high sensitivity and selectivity, J. Hazard. Mater., 2018, 342, 131–138 CrossRef CAS PubMed.
- X. Wu, F. Jia and Z. Fu, Construction of dibutyl phthalate molecularly imprinted electrochemical sensor based on multi-walled carbon nanotubes-modified boron-doped diamond electrode, J. Phys.: Conf. Ser., 2021, 2079, 012012 CrossRef.
- S. Wang, M. Pan, K. Liu, X. Xie, J. Yang, L. Hong and S. Wang, A SiO2@ MIP electrochemical sensor based on MWCNTs and AuNPs for highly sensitive and selective recognition and detection of dibutyl phthalate, Food Chem., 2022, 381, 132225 CrossRef CAS.
- P. W. Gao, Y. Z. Shen, C. Ma, Q. Xu and X. Y. Hu, High-sensitivity photo-electrochemical heterostructure of the cuprous oxide-metal organic framework for a dioctyl phthalate molecularly imprinted sensor, Analyst, 2021, 146(20), 6178–6186 RSC.
- X. Li, L. Zhong, R. Liu, X. Wei and J. Li, A molecularly imprinted photoelectrochemical sensor based on the use of Bi2S3 for sensitive determination of dioctyl phthalate, Microchim. Acta, 2019, 186(11), 1–8 Search PubMed.
- J. Zhang, Y. Niu, S. Li, R. Luo and C. Wang, A molecularly imprinted electrochemical sensor based on sol–gel technology and multiwalled carbon nanotubes–Nafion functional layer for determination of 2-nonylphenol in environmental samples, Sens. Actuators, B, 2014, 193, 844–850 CrossRef CAS.
- J. Huang, X. Zhang, S. Liu, Q. Lin, X. He, X. Xing, W. Lian and D. Tang, Development of molecularly imprinted electrochemical sensor with titanium oxide and gold nanomaterials enhanced technique for determination of 4-nonylphenol, Sens. Actuators, B, 2011, 152(2), 292–298 CrossRef CAS.
- Y. Pan, L. Shang, F. Zhao and B. Zeng, A novel electrochemical 4-nonyl-phenol sensor based on molecularly imprinted poly (o-phenylenediamine-co-o-toluidine)− nitrogen-doped graphene nanoribbons− ionic liquid composite film, Electrochim. Acta, 2015, 151, 423–428 CrossRef CAS.
- H. J. Chen, Z. H. Zhang, R. Cai, X. Chen, Y. N. Liu, W. Rao and S. Z. Yao, Molecularly imprinted electrochemical sensor based on amine group modified graphene covalently linked electrode for 4-nonylphenol detection, Talanta, 2013, 115, 222–227 CrossRef CAS.
- Y. Pan, F. Zhao and B. Zeng, Electrochemical sensors of octylphenol based on molecularly imprinted poly (3, 4-ethylenedioxythiophene) and poly (3, 4-ethylenedioxythiophene–gold nanoparticles), RSC Adv., 2015, 5(71), 57671–57677 RSC.
- Y. Xia, X. Hu, Y. Liu, F. Zhao and B. Zeng, Molecularly imprinted ratiometric electrochemical sensor based on carbon nanotubes/cuprous oxide nanoparticles/titanium carbide MXene composite for diethylstilbestrol detection, Microchim. Acta, 2022, 189(4), 1–10 CrossRef.
- C. Lou, T. Jing, J. Tian, Y. Zheng, J. Zhang, M. Dong, C. Wang, C. Hou, J. Fan and Z. Guo, 3-Dimensional graphene/Cu/Fe3O4 composites, immobilized laccase electrodes for detecting bisphenol A, J. Mater. Res., 2019, 34(17), 2964–2975 CrossRef CAS.
- P. Fernandes, J. M. Campiña and A. F. Silva, A layered nanocomposite of laccase, chitosan, and Fe3O4 nanoparticles-reduced graphene oxide for the nanomolar electrochemical detection of bisphenol A, Microchim. Acta, 2020, 187(5), 1–10 CrossRef PubMed.
- F. A. D. Inroga, M. O. Rocha, V. Lavayen and J. Arguello, Development of a tyrosinase-based biosensor for bisphenol A detection using gold leaf–like microstructures, J. Solid State Electrochem., 2019, 23(6), 1659–1666 CrossRef CAS.
- Y. Liu, L. Yao, L. He, N. Liu and Y. Piao, Electrochemical enzyme biosensor bearing biochar nanoparticle as signal enhancer for bisphenol a detection in water, Sensors, 2019, 19(7), 1619 CrossRef CAS.
- L. He, Y. Yang, J. Kim, L. Yao, X. Dong, T. Li and Y. Piao, Multi-layered enzyme coating on highly conductive magnetic biochar nanoparticles for bisphenol A sensing in water, Chem. Eng. J., 2020, 384, 123276 CrossRef CAS.
- N. Zehani, P. Fortgang, M. S. Lachgar, A. Baraket, M. Arab, S. V. Dzyadevych, R. Kherrat and N. J. Renault, Highly sensitive electrochemical biosensor for bisphenol A detection based on a diazonium-functionalized boron-doped diamond electrode modified with a multi-walled carbon nanotube-tyrosinase hybrid film, Biosens. Bioelectron., 2015, 74, 830–835 CrossRef CAS PubMed.
- Y. Q. Liu, J. J. Ding, Y. Liang, W. H. Qiu, F. Luo and H. Deng, A Novel Method to Determinate Nonylphenol Contents in Water Samples Based on Laccases Cetyltrimethyl Ammonium Bromide-Carbon Paste Electrode, Adv. Mater. Res., 2012, 550–553, 2009–2013 CAS.
- R. Zhang, Y. Zhang, X. Deng, S. Sun and Y. Li, A novel dual-signal electrochemical sensor for bisphenol A determination by coupling nanoporous gold leaf and self-assembled cyclodextrin, Electrochim. Acta, 2018, 271, 417–424 CrossRef CAS.
- M. Y. Ali, A. U. Alam and M. M. R. Howlader, Fabrication of highly sensitive Bisphenol A electrochemical sensor amplified with chemically modified multiwall carbon nanotubes and β-cyclodextrin, Sens. Actuators, B, 2020, 320, 128319 CrossRef CAS.
- H. Filik, A. A. Avan and E. K. Yetimoğlu, Multiwalled carbon nanotubes β-cyclodextrin modified electrode for electrochemical determination of bisphenol S in water samples, Russ. J. Electrochem., 2019, 55(2), 70–77 CrossRef CAS.
- Z. Ye, Q. Wang, J. Qiao, B. Ye and G. Li, Simultaneous detection of bisphenol A and bisphenol S with high sensitivity based on a new electrochemical sensor, J. Electroanal. Chem., 2019, 854, 113541 CrossRef CAS.
- W. Chen, Y. Jiang, J. Jiang, L. Qian and W. Gu, Determination of 3, 3′, 4, 4′-Polychlorinated biphenyls by an electrochemical sensor based on a methylene blue probe, J. Electrochem. Soc., 2019, 166(12), B908–B915 CrossRef CAS.
- S. Xiong, J. Cheng, L. He, M. Wang, X. Zhang and Z. Wu, Detection of di (2-ethylhexyl) phthalate through graphene–β-cyclodextrin composites by electrochemical impedance spectroscopy, Anal. Methods, 2014, 6(6), 1736–1742 RSC.
- S. Xiong, J. Cheng, L. He, D. Cai, X. Zhang and Z. Wu, Fabrication of β-cyclodextrin/graphene/1, 10-diaminodecane composite on glassy carbon electrode and impedimetric method for Di (2-ethyl hexyl) phthalate determination, J. Electroanal. Chem., 2015, 743, 18–24 CrossRef CAS.
- D. Su, Y. Zhang, Z. Wang, Q. Wan and N. Yang, Decoration of graphene nano platelets with gold nanoparticles for voltammetry of 4-nonylphenol, Carbon, 2017, 117, 313–321 CrossRef CAS.
- S. S. Li, K. H. Tu, C. C. Lin, C. W. Chen and M. Chhowalla, Solution-processable graphene oxide as an efficient hole transport layer in polymer solar cells, ACS Nano, 2010, 4(6), 3169–3174 CrossRef CAS.
- Y. Zhou and J. Zhi, The application of boron-doped diamond electrodes in amperometric biosensors, Talanta, 2009, 79(5), 1189–1196 CrossRef CAS.
- N. B. Messaoud, M. E. Ghica, C. Dridi, M. B. Ali and C. M. A. Brett, A novel amperometric enzyme inhibition biosensor based on xanthine oxidase immobilised onto glassy carbon electrodes for bisphenol A determination, Talanta, 2018, 184, 388–393 CrossRef.
- H. Liang, Y. Zhao, H. Ye and C. P. Li, Ultrasensitive and ultrawide range electrochemical determination of bisphenol A based on PtPd bimetallic nanoparticles and cationic pillar [5] arene decorated graphene, J. Electroanal. Chem., 2019, 855, 113487 CrossRef CAS.
- Y. Wang, F. Chen, X. Ye, T. Wu, K. Wu and C. Li, Photoelectrochemical immunosensing of tetrabromobisphenol A based on the enhanced effect of dodecahedral gold nanocrystals/MoS2 nanosheets, Sens. Actuators, B, 2017, 245, 205–212 CrossRef CAS.
- C. Liu, X. Huang, Y. Y. Wu, X. Deng, Z. Zheng, Z. Xu and D. Hui, Advance on the dispersion treatment of graphene oxide and the graphene oxide modified cement-based materials, Nanotechnol. Rev., 2021, 10(1), 34–49 CrossRef CAS.
- S. Khazalpour, M. Yarie, E. Kianpour, A. Amani, S. Asadabadi, J. Y. Seyf, M. Rezaeivala, S. Azizian and M. A. Zolfigol, Applications of phosphonium-based ionic liquids in chemical processes, J. Iran. Chem. Soc., 2020, 17(8), 1775–1917 CrossRef CAS.
|
This journal is © The Royal Society of Chemistry 2023 |
Click here to see how this site uses Cookies. View our privacy policy here.