DOI:
10.1039/D3RA06536G
(Paper)
RSC Adv., 2023,
13, 35841-35852
Management of virulence in Pseudomonas aeruginosa and Serratia marcescens using environmentally-friendly titanium dioxide nanoparticles
Received
25th September 2023
, Accepted 24th November 2023
First published on 11th December 2023
Abstract
Antimicrobial resistance (AMR), a condition in which the efficacy of antimicrobial drugs in fighting microorganisms is reduced, has become a global challenge. Multidrug resistance (MDR) has been developing in microorganisms, where they can resist multiple medications. In particular, there has been a rise in MDR as well as extensively drug-resistant (XDR) strains of Pseudomonas aeruginosa in some regions, with prevalence rates ranging from 15% to 30%. The application of nanotechnology ranges from diagnostics to drug-delivery systems, revolutionizing healthcare, and improving disease treatment. We aimed to investigate the efficacy of titanium dioxide nanoparticles (TiO2-NPs) against various virulent traits of P. aeruginosa and S. marcescens. More than 50% reduction in the production of virulent pigments of P. aeruginosa was recorded following the treatment of TiO2-NPs. Additionally, elastases and exoproteases were inhibited by 58.21 and 74.36%, respectively. A similar result was observed against the rhamnolipid production and swimming motility of P. aeruginosa. The effect of TiO2-NPs was also validated against another opportunistic pathogen, S. marcescens, where the production of prodigiosin was reduced by 64.78%. Also, a roughly 75% attenuation of proteolytic activity and more than 50% reduction in swarming motility were found. In the control group, the cell surface hydrophobicity was 77.72%, which decreased to 24.67% with the addition of 64 μg ml−1 TiO2-NPs in culture media. The hydrophobicity index of microorganisms is crucial for their initial attachment and the formation of biofilms. In conclusion, TiO2-NPs demonstrated potential in a multi-target approach against P. aeruginosa and S. marcescens, suggesting their advantages in the prevention and treatment of infections. These nanomaterials could have vital importance in the development of novel antibacterial agents to combat drug-resistant bacteria.
1. Introduction
Nanotechnology involves harnessing the unique properties of materials at the nanoscale. When applied to the field of medicine and healthcare, it is referred to as nanomedicine, and it has demonstrated effectiveness in addressing a number of prevalent diseases, including cardiovascular diseases and cancer.1 Nanoscience focuses on the study of the distinctive characteristics of materials within the 1–100 nanometre range. Nanomedicine, in particular, applies nanotechnologies to the realm of healthcare,2 utilizing nanoscale technologies and techniques to prevent, monitor, diagnose, and treat diseases.3 In the field of medicine, nanotechnologies hold immense promise, covering a wide range of applications, including diagnostic tools, imaging methods, tissue-engineered constructs, drug-delivery systems, implants, and pharmaceutical therapies.4 This progress has significantly improved the treatment of various diseases, encompassing cancer, cardiovascular conditions, musculoskeletal disorders, neurodegenerative and psychiatric illnesses, viral and bacterial infections, and diabetes.5 Metal nanoparticles, specifically TiO2-NPs, are extensively manufactured at an industrial level and numerous practical applications have been discovered for them in both industrial processes and daily routines.6 These metal nanoparticles have demonstrated notable efficacy in eradicating and restraining microbial pathogens by targeting multiple sites (Scheme 1). TiO2-NPs exhibit high durability and can function as effective antimicrobial agents against a broad spectrum of bacteria. The antimicrobial efficacy of this metal oxide is contingent upon various factors, such as their shape, size, and crystal structure.7,8 Additionally, the antimicrobial attributes of TiO2-NPs have been attributed to their photocatalytic properties.9 Previous reports have highlighted the significance of oxidative stress, particularly ROS formation, as a crucial component in the antimicrobial mechanism of TiO2-NPs.10 While TiO2-NPs have demonstrated potent antimicrobial properties, it is important to note that they can also pose potential toxicity risks to human health.10 In terms of safety concerns, the Scientific Committee on Consumer Safety (SCCS) determined a maximum level of 25% weight for each UV filter in the nanoform is safe for dermal applications. The overall findings indicate that the penetration of TiO2-NPs into the skin is influenced by various external factors, such as the drug vehicle, dose, and protein reactivity, as well as endogenous factors, like the disease or skin condition, skin age, site of application, etc.6
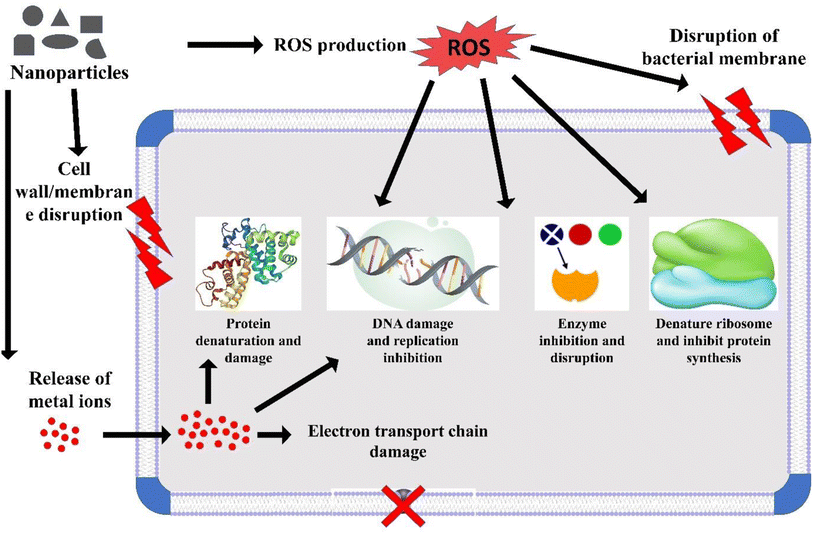 |
| Scheme 1 Various possible targets of TiO2-NPs against microbial pathogens. | |
Antimicrobial resistance (AMR) is the term used to describe the reduced effectiveness of antimicrobial drugs in inhibiting the growth and reproduction of microorganisms.11 In the developing world, a primary challenge in healthcare lies in combating infectious diseases, and antimicrobial agents have proven to be highly effective in this regard. However, the emergence of antimicrobial resistance has become a formidable global issue that demands effective solutions.12 Multidrug resistance (MDR), on the other hand, is characterized by a microorganism's ability to withstand multiple antimicrobial medications, even those with different chemical structures and molecular targets, despite having been sensitive to them in the past.13 In recent years, there has been a noticeable rise in the occurrence of MDR and extensively drug-resistant (XDR) strains of Pseudomonas aeruginosa. In certain geographical regions, the prevalence rates of these resistant strains range from 15% to 30%.14 Data from the United States indicates that MDR P. aeruginosa is responsible for 13% of severe healthcare-associated infections.15 In humans, P. aeruginosa plays a substantial role in several medical conditions, such as burn wounds, chronic wounds,16 corneal infections,17 and respiratory infections in individuals with cystic fibrosis.18 The regulation of many of the extracellular products in P. aeruginosa is controlled by a complex hierarchical quorum sensing (QS) cascade.19 Within this QS system, two complete AHL (N-acylhomoserine lactone) circuits exist, namely RhlR–RhlI and LasR–LasI, each comprising a LuxI-type synthase and a LuxR-type receptor. In addition to the AHL-based quorum sensing system, P. aeruginosa also produces another crucial quorum sensing signal molecule known as the Pseudomonas quinolone signal (PQS).20 The PQS-mediated quorum sensing significantly contributes to the virulence and pathogenicity of P. aeruginosa.21
Serratia species, particularly Serratia marcescens, are noteworthy human pathogens. The initial reported cases shed light on the pathogenic nature of S. marcescens. Many of these infections attributed to S. marcescens were likely to have originated in hospital settings, and this bacterium is frequently isolated from healthcare-associated infections or from patients with underlying medical conditions.22 In newborns, S. marcescens can lead to a wide spectrum of clinical manifestations, ranging from asymptomatic colonization to conditions such as keratitis, urinary tract infections, conjunctivitis, surgical wound infections, pneumonia, sepsis, meningitis, and bloodstream infections.22 The most commonly affected sites of infection are the bloodstream, followed by the respiratory system and the gastrointestinal tract.23
In the past, bacterial cells were conventionally perceived as separate entities, each operating independently with its own self-regulating metabolic and growth systems.21 Conversely, modern scientific understanding has firmly established that bacterial cells engage in a process called quorum sensing (QS).24 In QS, bacterial cells both produce and detect small signalling molecules to facilitate communication. The release and recognition of QS signals lead to two primary outcomes. First, they control the production of various extracellular factors. These factors serve diverse functions, including searching for nutrients, suppressing the immune response, boosting virulence, providing structural support (biofilms), and aiding in mobility.21 Essentially, QS enables individual bacterial cells to monitor and assess the size of their community and empowers them to regulate resource-intensive traits based on the density of cells and the concentration of signalling molecules.25
Given the challenges associated with AMR and the limited discovery of new drugs to combat drug-resistant microbes, there is a compelling need for the development of novel antibiotics or alternative strategies to address AMR. Nanomaterials have demonstrated promising antibacterial properties. In this study, we evaluated the efficacy of titanium dioxide nanoparticles (TiO2-NPs) against various virulent traits of P. aeruginosa and S. marcescens. If proven to be successful, such nanomaterials could play a valuable role in the discovery of innovative antibacterial agents for combating drug-resistant bacteria.
2. Materials and methods
2.1. Synthesis of TiO2-NPs
The titanium dioxide nanoparticles (TiO2-NPs) were produced using an aqueous extract derived from Carum copticum. Detailed information regarding the synthesis and characterization of TiO2-NPs has been published previously.26 Here, we provide a brief description of the TiO2-NPs synthesis procedure used. In summary, 20 ml of extract was combined with 300 ml of a 5 mM titanium(IV) dioxide solution in double-distilled water, and this mixture was agitated using a magnetic stirrer. As the reaction proceeded, 1 ml of 1 mM NaOH was gradually added to the mixture while continuous stirring was maintained. The reaction continued for 6 h at 60 °C. Subsequently, the nanoparticles were separated via centrifugation at 22
000 rcf for 30 min. The TiO2-NPs underwent a series of washes, including three rounds of distilled water rinsing followed by ethanol rinsing, and were finally dried overnight in an oven at 50 °C. The resulting TiO2-NPs were stored in powdered form at room temperature for characterization. Characterization of the nanoparticles was conducted utilizing UV-visible and FTIR spectroscopy, X-ray diffraction, electron microscopy, and energy EDX analysis. The UV-vis spectroscopy displayed a broad absorption band within the range of 385–395 nm, attributed to the presence of titanium oxide nanoparticles.27 TEM analysis revealed an average particle size of 12.01 nm for the TiO2-NPs. The nanoparticles were stored for six months in powdered form to check the stability of the nanoparticles. Upon investigation, we found that the anti-QS properties were negligibly affected, hence confirming the stability of the TiO2-NPs.
2.2. Assays to examine the QS-controlled virulence factors in P. aeruginosa PAO1
The impact of TiO2-NPs on six virulence factors of P. aeruginosa PAO1 at sub-MICs was investigated using the following procedures.
2.2.1. Pyoverdin production. Evaluation of the relative levels of pyoverdin in P. aeruginosa PAO1 followed a previously established protocol.28 In summary, an overnight cultivated culture of P. aeruginosa PAO1, grown both in the absence and presence of 8–64 μg ml−1 TiO2-NPs, was collected. The culture was subjected to centrifugation to obtain the cell-free supernatant. Subsequently, 100 μl of the supernatant was mixed with 0.9 ml Tris–HCl buffer (50 mM and pH 7.4). Using a spectrofluorometer, the fluorescence signals of this solution were measured at 460 nm upon excitation at 400 nm. The percentage data were then calculated in relation to the untreated control.
2.2.2. Pyocyanin production. Estimation of the pyocyanin production was carried out using Pseudomonas broth (PB) medium, which included 1.4 mg ml−1 MgCl2, 20 mg ml−1 peptone, and 10 mg ml−1 K2SO4, known for its ability to enhance pyocyanin production.29 P. aeruginosa PAO1 was cultivated in PB medium under different conditions: without and with varying doses of TiO2-NPs (8, 16, 32, and 64 μg ml−1) for a duration of 18 h. Next, 5 ml of the cultured solution was extracted using 3 ml chloroform, with the aqueous phase discarded. The upper organic phase was then subjected to extraction with 1200 μl of 0.2 N HCl. The absorbance of the resulting aqueous phase was measured at 520 nm against a blank. The quantity of pyocyanin was determined in μg ml−1 using a previously specified method.30
2.2.3. Exoprotease activity. Evaluation of the exoprotease activity in the supernatant of P. aeruginosa PAO1 was conducted using the azocasein degradation protocol, as previously outlined.31 The bacteria were cultured both without TiO2-NPs and with various doses (8, 16, 32, and 64 μg ml−1) of TiO2-NPs for 18 h, with constant shaking at the physiological temperature of 37 °C. Following incubation, the cultures were subjected to centrifugation to remove the bacterial cells, and the resulting cell-free supernatant was collected. Next, 100 μl of the cell-free supernatant from both the control and TiO2-NPs-treated bacterial cultures was mixed with 1000 μl of 0.3% azocasein solution (containing 500 μM calcium chloride in 50 mM Tris–HCl). This mixture was then incubated at 37 °C for 20 min. To halt the reaction, 0.5 ml of 10% TCA was added, and the reaction mixture was subsequently centrifuged for 12 min. The absorbance of the resulting supernatant was measured at 400 nm with the help of a double beam spectrophotometer.
2.2.4. Elastase activity. The elastinolytic activity in the culture supernatant of P. aeruginosa PAO1 was assayed using Elastin Congo Red (ECR) dye.32 The dye was prepared by dissolving 5000 μg ml−1 ECR with 0.001 M CaCl2 in 100 mM Tris. In short, 0.1 ml cell-free supernatant from both the control and TiO2-NPs-treated P. aeruginosa PAO1 cultures was combined with 0.9 ml of ECR buffer. The mixture was incubated at 37 °C for 3 h with gentle mixing. Next, 1000 μl of sodium phosphate buffer was mixed in to stop the reaction, followed by a 30 min cooling period on ice. After centrifugation, the insoluble ECR was removed. The absorbance was measured at 495 nm. The percent inhibition in elastinolytic activity was calculated by comparing it to the untreated control.
2.2.5. Rhamnolipid production. The rhamnolipid assay was utilized with the orcinol method with slight modifications based on a previous protocol.33 To perform the assay, the treatment to P. aeruginosa PAO1 was performed as mentioned in the above sections. Briefly, 300 μl of cell-free supernatant from both the control and TiO2-NPs-treated cultures of P. aeruginosa PAO1 was extracted using 0.6 ml of diethyl ether. After collecting the diethyl ether phase, it was dried at 37 °C and then re-dissolved in 0.1 ml of deionized water. To each sample, 0.9 ml of a 0.19% orcinol solution was added. The samples were heated for 30 min at 80 °C and then cooled for 15 min. Finally, the absorbance was measured at 421 nm.
2.2.6. Swimming motility. For the assessment of the swimming motility, 5 μl of an overnight cultured sample of P. aeruginosa PAO1 was placed onto 0.3% LB agar plates. For the control plates, no treatment with TiO2-NPs was given. In other sets, soft agar plates were prepared by adding 8, 16, 32, and 64 μg ml−1 TiO2-NPs. After spotting P. aeruginosa PAO1 on the soft agar plates, they were allowed to dry under a laminar flow hood.34 Following an 18 h incubation, the diameter of the swarm zone was measured to determine the extent of swimming motility. The swarm zone measurements were recorded in mm, and the data were presented as the percent inhibition relative to the control.
2.3. Assays to examine the QS-controlled virulence factors in S. marcescens MTCC 97
The influence of TiO2-NPs on three S. marcescens MTCC 97 virulence factors was also examined at sub-MICs and the detailed procedures are outlined below.
2.3.1. Prodigiosin production. Serratia marcescens MTCC 97 was grown without and with 8, 16, 32, and 64 μg ml−1 TiO2-NPs as mentioned above in the P. aeruginosa PAO1 section and placed in a shaking incubator for one day. Afterward, the cells were collected through centrifugation and the supernatant was removed. To extract prodigiosin pigment from the pelleted cells, 1000 μl of an acidified ethanol solution was used, which consisted of 4 ml of 1 M HCl mixed with 96 ml ethanol. The level of prodigiosin was quantified by measuring the absorbance of the samples at 534 nm.35
2.3.2. Proteolytic activity. The proteolytic activity in the culture supernatant of S. marcescens MTCC 97 was assayed by azocasein degradation assay as described above in the P. aeruginosa PAO1 section. In summary, a volume of 0.1 ml of supernatant from untreated and TiO2-NPs-treated cultures was combined with 1 ml of a 0.3% azocasein solution and then incubated for 15 min at 37 °C. To stop the reaction, 500 μl of 10% TCA was added. Next, centrifugation of the samples was done for 12 min at 18
000 rcf and the absorbance of the resulting supernatant was measured at 400 nm.
2.3.3. Measurement of the cell surface hydrophobicity. The cell surface hydrophobicity in S. marcescens MTCC 97 was assessed using xylene, following the previously described method.36 In brief, 100 μl of an S. marcescens MTCC 97 overnight grown culture was taken in 1.5 ml microvials and 100 μl xylene was mixed along with varying concentrations of TiO2-NPs. The control group consisted of cells solely with the xylene. The resulting mixture was strongly vortexed and then left for incubation for 10 min to allow for separation of the two phases. Subsequently, the absorbance of the aqueous phase was measured at 530 nm.
2.3.4. Swarming motility. We only assessed the swarming motility in S. marcescens MTCC 97 as this bacterium is highly motile and it is difficult to measure its swimming motility. In short, 5 μl of an overnight culture were point inoculated at the centre of LB soft agar plates containing 0.5% agar. Like for the P. aeruginosa PAO1 motility assay, 0.5% soft agar plates were prepared for both the control and TiO2-NPs-treated groups. The plates were allowed to air dry and then the plates were then incubated for 18 h, after which the swarm zones were measured to assess the motility.
2.4. Statistical analysis
Each assay was performed separately in four independent trials, and these experiments were replicated a minimum of two times. The results reported in this study represent the mean values, accompanied by their corresponding standard deviations. The statistical significance of the treatment groups was assessed by comparing them to the untreated control to calculate the p-values. In cases where * is indicated, it signifies p-values of ≤ 0.05 in comparison to the control, and ** indicates p-values of ≤ 0.01 compared to the control.
3. Results and discussion
3.1. Assays to examine the QS-controlled virulence factors in P. aeruginosa PAO1
Examination of the impact of TiO2-NPs on the virulent traits of P. aeruginosa PAO1 was conducted at sub-MIC levels, and the findings are discussed below.
3.1.1. TiO2-NPs modulation of the virulent pigments of P. aeruginosa PAO1. Pyoverdin, a virulence factor of P. aeruginosa, which is known for its fluorescent properties, plays a crucial function in virulence and subsequent infections.37 Research has indicated that pyoverdin production is regulated by quorum sensing, as P. aeruginosa lacking quorum sensing has exhibited decreased pyoverdin levels.38 This siderophore competes for iron within transferrin proteins, ensuring a sufficient iron supply that is essential for P. aeruginosa's survival in host systems.39 Recent findings have also emphasized pyoverdin's ability to avoid detection by the host's defensive protein, namely neutrophil gelatinase-associated lipocalin, further contributing to P. aeruginosa infections in the lungs of patients with cystic fibrosis.37 Any reduction in this pigment production is a direct indicator of quorum sensing inhibition. Here, treatment with TiO2-NPs led to reduced pyoverdin levels (Fig. 1A). At the lowest dose (8 μg ml−1) of TiO2-NPs, a very low (11.26%) level of inhibition was found that was statistically insignificant (p-value > 0.05). However, reductions of 25.52, 48.10, and 67.80% were observed compared to the control when treated with 16, 32, and 64 μg ml−1 of TiO2-NPs, respectively. These reductions in pigment production demonstrated the effectiveness of TiO2-NPs against quorum sensing-mediated virulence in P. aeruginosa. Previously, green synthesized gold nanoparticles were reported to inhibit the pyocyanin levels in P. aeruginosa by >75% at 200 μg ml−1.40
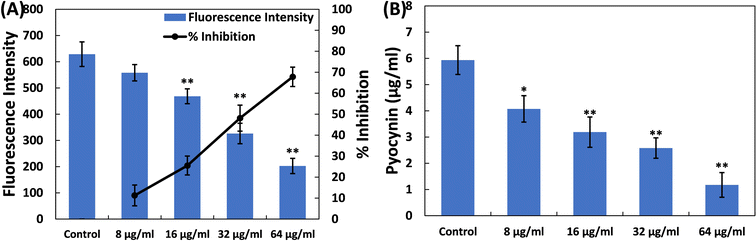 |
| Fig. 1 (A) Inhibition of pyoverdin production in P. aeruginosa PAO1 by TiO2-NPs. Percent inhibition is shown on the secondary y-axis. (B) Inhibition of pyocyanin pigment production in P. aeruginosa PAO1 by TiO2-NPs. Data are presented as the average of four replicates with the standard deviation. In cases where * is indicated, it signifies a p-value ≤ 0.05 in comparison to the control, and ** indicates a p-value ≤ 0.01 compared to the control. | |
Pyocyanin, another pigment with a unique blue-green colour, is directly controlled by quorum sensing in P. aeruginosa, playing a significant role in the bacterium's virulence.41 The synthesis of pyocyanin involves several steps, commencing with the production of autoinducer molecules and the subsequent activation of specific genes (phzA-G). Both phenazine-1-carboxylic acid (a precursor to pyocyanin) and pyocyanin disrupt the normal rhythmic movement of respiratory cilia in humans and affect the expression of immune-regulating proteins, especially in individuals with cystic fibrosis. Additionally, pyocyanin-induced oxidative stress worsens the severity of the disease.42,43 Pyocyanin is also recognized for its involvement in biofilm formation.44,45 This pigment also impacts various cellular processes, primarily due to its redox properties, making it a crucial element in P. aeruginosa's ability to cause disease.46 Here, in the untreated control, the level of pyocyanin was 5.93 ± 0.54 μg ml−1. Treatment with TiO2-NPs led to a decrease in pyocyanin levels in P. aeruginosa PAO1, as depicted in Fig. 1B. At doses of 8, 16, and 32 μg ml−1 TiO2-NPs, there was an inhibition of pyocyanin pigment production by 31.36%, 46.30%, and 56.51%, respectively. In the presence of the highest concentration of TiO2-NPs (64 μg ml−1), >80% inhibition of this pigment was recorded. It is worth mentioning that tin oxide hollow nanoflowers have previously shown the ability to reduce pyocyanin levels in P. aeruginosa PA01 by approximately 60%.47
3.1.2. Inhibition of the virulent enzymes of P. aeruginosa PAO1 by TiO2-NPs. The impact of TiO2-NPs on two virulent enzymes of P. aeruginosa PAO1 was also examined. Proteases and elastases are crucial components contributing to the pathogenicity of Pseudomonas aeruginosa.48 Fig. 2 provides a comprehensive overview of how TiO2-NPs affect the activity of exoproteases. Exoproteases, when released from bacterial cells, facilitate bacterial invasion by breaking down proteins and evading the immune response.49 Proteases secreted by P aeruginosa are well known for their virulence.50 For example, AprA (alkaline protease) is a QS-governed virulence factor that is secreted via the type I secretion system.51 AprA has the capability to break down the complement components TNF-α and IFN-γ, effectively undermining the host's immune system and exacerbating infections.52 Protease IV, another virulent protein, has been notably linked to the corneal virulence of P. aeruginosa.53 Protease IV not only disrupts the host immunity by breaking down essential biological molecules, like complement components, surfactant proteins, and immunoglobulins, but also causes harm to host tissues and amplifies bacterial infection by degrading fibrinogen, transferrin, elastin, and lactoferrin.54–57 Here, while a lower concentration (8 μg ml−1) of TiO2-NPs exhibited only a minimal reduction (less than 20%) in activity, higher concentrations led to significant inhibitions. Specifically, the presence of 16, 32, and 64 μg ml−1 TiO2-NPs inhibited the activity of these azocasein-degrading enzymes by 25.71%, 40.18%, and 74.36%, respectively. Previously, green synthesized gold nanoparticles were found to mitigate proteolytic activity in P. aeruginosa by more than 80% at 200 μg ml−1.40
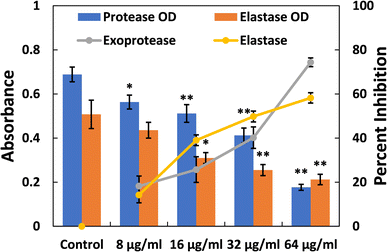 |
| Fig. 2 Inhibition of exoprotease and elastase activity in P. aeruginosa PAO1 by TiO2-NPs. Percent inhibition is shown on the secondary y-axis. Data are presented as the average of four replicates with the standard deviation. In cases where * is indicated, it signifies a p-value ≤ 0.05 in comparison to the control, and ** indicates a p-values ≤ 0.01 compared to the control. | |
Elastases belong to a group of hydrolytic enzymes that not only degrade the tissues of the host but also interfere with the immune system.58 Biofilm formation and virulence factor production in the pathogenicity of P. aeruginosa heavily rely on the expression of the las proteins, which are regulated by QS (quorum sensing). These enzymes also play a crucial role in virulence and biofilm development. Fig. 2 depicts the dose-dependent effect of TiO2-NPs on the activity of elastase. At a lower dose of TiO2-NPs (8 μg ml−1), an insignificant (p-value > 0.05) reduction in elastinolytic activity was found. On the contrary, treatment at higher levels of TiO2-NPs (16, 32, and 64 μg ml−1) resulted in reductions of elastase activity by 39.02%, 49.77%, and 58.21%, respectively. These findings strongly suggest that TiO2-NPs disrupt P. aeruginosa's lasI-lasR quorum sensing system, in line with previous observations.34 Numerous metal nanoparticles, including silver and tin oxide nanoparticles, have also been demonstrated to inhibit the virulent enzymes of P. aeruginosa by targeting quorum sensing.33,47
3.1.3. Inhibition of the rhamnolipid production and swimming motility in P. aeruginosa PAO1. Rhamnolipids, as surfactant molecules, constitute another important virulence factor in Pseudomonas aeruginosa. These glycolipids are found in virulent strains and play a role in regulating human respiratory epithelium, aiding in cellular invasion.59,60 Fig. 3 demonstrates that TiO2-NPs have proven effectiveness in inhibiting the production of rhamnolipids in P. aeruginosa PAO1. When cultured in media containing 8, 16, and 32 μg ml−1 TiO2-NPs, there were reductions in rhamnolipid production by 18.34%, 34.46%, and 47.15%, respectively. In the presence of 64 μg ml−1 TiO2-NPs, the highest sub-MIC concentration tested, there was over 50% inhibition of this surfactant. It is important to note that these surfactants not only initiate biofilm formation but also influence bacterial motility.61 P. aeruginosa produces haemolysins, specifically rhamnolipid and phospholipase-C, which exert highly toxic effects on host cells and contribute to the pathogen's ability to disseminate within the target tissue. These two toxins work in tandem, catalyzing the degradation of lipids and lecithin.62
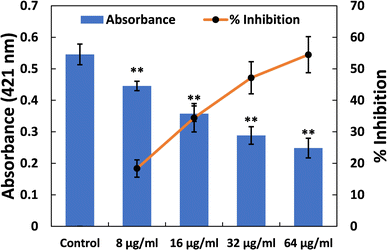 |
| Fig. 3 Inhibition of rhamnolipid production in P. aeruginosa PAO1 by TiO2-NPs. Data are presented as the average of four replicates with the standard deviation. In cases where * is indicated, it signifies a p-value ≤ 0.05 in comparison to the control, and ** indicates a p-value ≤ 0.01 compared to the control. | |
Furthermore, the impact of TiO2-NPs on the swarming motility of P. aeruginosa PAO1 was assessed, with the results presented in Fig. 4A. The mobility of P. aeruginosa, including swarming, swimming, and twitching, is important in spreading infections and the development of biofilms.63 As observed in the control plates, P. aeruginosa PAO1 swarmed across an entire Petri plate after overnight incubation (Fig. 4B). Additionally, there was a characteristic light green colour due to the pigment production in P. aeruginosa PAO1. The presence of TiO2-NPs in soft agar plates resulted in a progressive reduction in bacterial motility. At a lower dose (8 μg ml−1), less than 10% reduction in this bacterial motility was found. However, at concentrations of 16, 32, and 64 μg ml−1 TiO2-NPs, reductions of 25.06%, 32.03%, and 43.17% were observed compared to the control, respectively (Fig. 4C). P. aeruginosa's motility is a critical factor in its ability to spread infections and establish biofilms.63 There is also evidence that silver nanoparticles, synthesized using an extract from Murraya koenigii, significantly reduced the swarming of P. aeruginosa by more than 85%.64 In summary, TiO2-NPs exhibited a multi-target action against P. aeruginosa, indicating their potential benefits in preventing and managing infections.
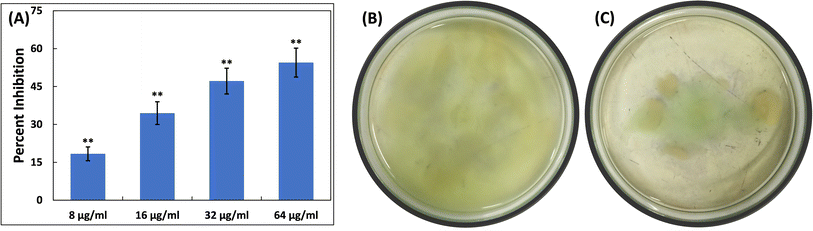 |
| Fig. 4 (A) Inhibition of swimming motility in P. aeruginosa PAO1 by TiO2-NPs. Data are presented as the average of four replicates with the standard deviation. In cases where * is indicated, it signifies a p-value ≤ 0.05 in comparison to the control, and ** indicates a p-value ≤ 0.01 compared to the control. (B) Representative image of an agar plate showing P. aeruginosa PAO1 swimming in the absence of TiO2-NPs. (C) Representative image of an agar plate showing P. aeruginosa PAO1 swimming in the presence of 64 μg ml−1 TiO2-NPs. | |
3.2. Assays to examine the QS-controlled virulence factors in S. marcescens MTCC 97
Examination of the impact of TiO2-NPs on three virulence factors of S. marcescens MTCC 97 was also conducted at sub-MIC levels, and the findings are discussed below.
3.2.1. Inhibition of prodigiosin production. S. marcescens employs a quorum sensing system to produce N-acylhomoserine lactones (AHLs), which in turn regulate several virulence factors, like prodigiosin production, carbapenem resistance, and biofilm formation, along with the production of extracellular enzymes.65 As depicted in Fig. 5, there was a dose-dependent effect on prodigiosin levels when exposed to TiO2-NPs. The treatment with 8, 16, and 32 μg ml−1 TiO2-NPs caused 18.20%, 34.71%, and 64.78% inhibition of this pigment production S. marcescens MTCC 97. At the higher level of treatment (64 μg ml−1 TiO2-NPs) more than 85% inhibition was found. It is documented that prodigiosin production was inhibited by >75% by silver nanoparticles.33 Another study also reported the reduction of prodigiosin level in S. marcescens, in which gold nanoparticles were synthesized from fruits extract of C. annuum, which reduced pigment production by 78.41% at a concentration of 200 μg ml−1.40
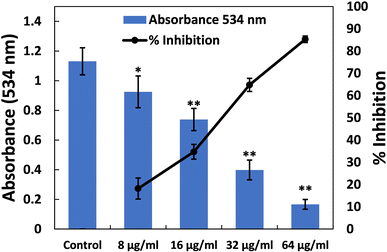 |
| Fig. 5 (A) Inhibition of prodigiosin production in S. marcescens MTCC 97 by TiO2-NPs. Percent inhibition is shown on the secondary y-axis. Data are presented as the average of four replicates with the standard deviation. In cases where * is indicated, it signifies a p-value ≤ 0.05 in comparison to the control, and ** indicates a p-value ≤ 0.01 compared to the control. | |
3.2.2. Inhibition of prodigiosin production proteolytic activity. The effect of TiO2-NPs on exoproteases was also tested. Exoprotease is another crucial virulence factor in S. marcescens, as the secretion of exoproteases plays a pivotal role in modulating the immune and inflammatory responses in the host.66 The haemolytic activity and the secretion of extracellular enzymes like protease in S. marcescens are also regulated by quorum sensing.67 Nearly all S. marcescens strains release a haemolysin, leading to the lysis of both animal and human erythrocytes68 and the liberation of inflammatory mediators from leukocytes.69 Here, the TiO2-NPs exhibited an inhibitory effect on exoprotease activity in S. marcescens MTCC 97 (Fig. 6A). At the lowest dose (8 μg ml−1) of TiO2-NPs, a very low (less than 15%) level of inhibition was found that was too statistically insignificant (p-value > 0.05). However, reductions of 46.14%, 63.39%, and 74.99% were observed compared to the control when treating with 16, 32, and 64 μg ml−1 of TiO2-NPs, respectively.
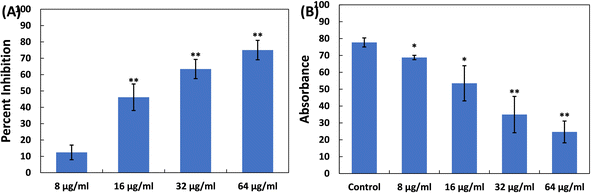 |
| Fig. 6 (A) Inhibition of exoprotease activity in S. marcescens MTCC 97 by TiO2-NPs. (B) Reduction in cell surface hydrophobicity in S. marcescens MTCC 97 by TiO2-NPs. Data are presented as the average of four replicates with the standard deviation. In cases where * is indicated, it signifies a p-value ≤ 0.05 in comparison to the control, and ** indicates a p-value ≤ 0.01 compared to the control. | |
3.2.3. Reduction in cell surface hydrophobicity. The impact of TiO2-NPs on the cell surface hydrophobicity in S. marcescens MTCC 97 was assessed, as the surface hydrophobicity is known to play a pivotal role in the adherence to solid surfaces and the formation of biofilms in bacterial pathogens.70 The TiO2-NPs demonstrated a significant, dose-dependent reduction in cell surface hydrophobicity, as depicted in Fig. 6B. In the untreated control group, the cell surface hydrophobicity was measured at 77.72% for S. marcescens MTCC 97. However, in the presence of TiO2-NPs at concentrations of 8, 16, and 32 μg ml−1, the cell surface hydrophobicity decreased to 68.77%, 53.49%, and 34.95%, respectively. The highest level of treatment (64 μg ml−1) reduced the cell surface hydrophobicity less than 25%, which is remarkable. The hydrophobicity index of microorganisms plays a critical role in the initial attachment and subsequent biofilm formation. Thus, targeting cell surface hydrophobicity presents an alternative strategy for reducing biofilm formation. These findings align with prior research, where the treatment of golf nanoparticles to S. marcescens resulted in a reduction of the surface hydrophobicity.40
3.2.4. Inhibition of swarming motility. Swimming motility is a unique trait of the virulent strains of S. marcescens, and it plays a crucial role in specific nosocomial infections, notably catheter-linked UTIs.70 To assess the impact of TiO2-NPs, soft agar plates were used to evaluate the capability of S. marcescens MTCC 97 swarming and the quantitative data are presented in Fig. 7A. On plates lacking TiO2-NPs, the bacteria spread across the entire plate, resulting in an average zone diameter of roughly 90 mm, as depicted in Fig. 7B. However, in the presence of 8, 16, and 32 μg ml−1 TiO2-NPs, there were notable 15.32%, 35.93%, and 56.54% reductions in the swarm diameter. While the higher tested concentrations (64 μg ml−1) exhibited a >85% inhibition. The flagella-assisted motilities, like swimming, play a pivotal role in regulating the attachment of S. marcescens prior to biofilm formation and are of significant importance in UTIs.71 Previously, gold nanoparticles have been found to reduce the motility of S. marcescens.40
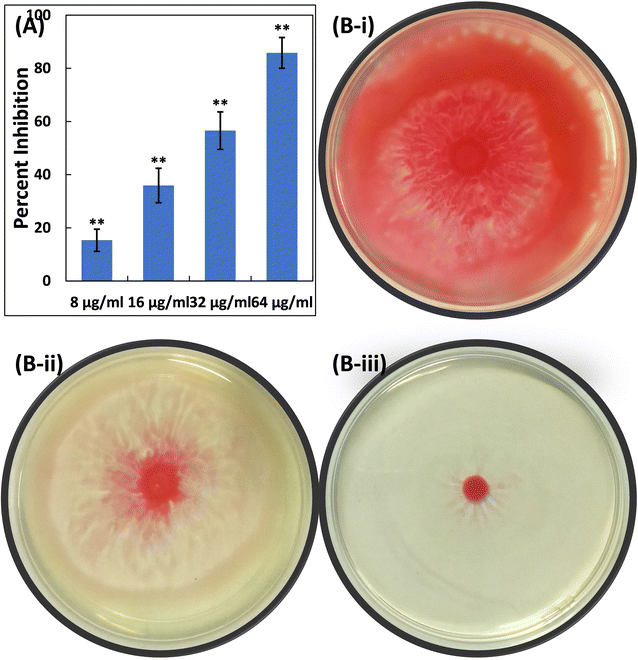 |
| Fig. 7 (A) Inhibition of swarming motility in S. marcescens MTCC 97 by TiO2-NPs. Data are presented as the average of four replicates with the standard deviation. In cases where * is indicated, it signifies a p-value ≤ 0.05 in comparison to the control, and ** indicates a p-value ≤ 0.01 compared to the control. (B(i)) Representative image of an agar plate showing S. marcescens MTCC 97 swarming in the absence of TiO2-NPs. (B(ii)) Representative image of an agar plate showing S. marcescens MTCC 97 swarming in the presence of 16 μg ml−1 TiO2-NPs. (B(iii)) Representative image of an agar plate showing S. marcescens MTCC 97 swarming in the presence of 64 μg ml−1 TiO2-NPs. | |
4. Conclusion
The emergence of AMR has become a global challenge, particularly with the rise of MDR and XDR strains of P. aeruginosa. Nanotechnology, spanning diagnostics to drug-delivery systems, offers innovative solutions in healthcare. In this study, we investigated the efficacy of titanium dioxide nanoparticles (TiO2-NPs) against P. aeruginosa and S. marcescens. The TiO2-NPs demonstrated significant reductions in virulent traits of P. aeruginosa, including pigment production, elastases, exoproteases, rhamnolipid production, and swimming motility. Similar results were observed against S. marcescens, where prodigiosin production, proteolytic activity, and swarming motility were significantly reduced. The TiO2-NPs also affected the cell surface hydrophobicity, a crucial factor in initial attachment and biofilm formation. In conclusion, the multi-target action of TiO2-NPs suggests their potential in preventing and treating infections. If proven effective, these nanomaterials could contribute to the development of innovative antibacterial agents to combat drug-resistant bacteria.
Abbreviations
AHL | Acylhomoserine lactones |
AMR | Antimicrobial resistance |
ECR | Elastin Congo red |
MDR | Multidrug resistance |
QS | Quorum sensing |
TiO2-NPs | Titanium dioxide nanoparticles |
Conflicts of interest
All authors declare that there is no conflict of interest associated with this study.
Acknowledgements
The authors are grateful to the Researchers supporting project number (RSPD2023R972), King Saud University, Riyadh, Saudi Arabia for the support. FAQ is thankful to CSIR [file no. 09/112(0626)2k19-EMR] for providing SRF.
References
- S. Sim and N. Wong, Nanotechnology and Its Use in Imaging and Drug Delivery (Review), Biomed. Rep., 2021, 14, 42, DOI:10.3892/br.2021.1418.
- A. Haleem, M. Javaid, R. P. Singh, S. Rab and R. Suman, Applications of Nanotechnology in Medical Field: A Brief Review, J. Glob. Health, 2023, 7, 70–77, DOI:10.1016/j.glohj.2023.02.008.
- O. Farokhzad and R. Langer, Nanomedicine: Developing Smarter Therapeutic and Diagnostic Modalities, Adv. Drug Delivery Rev., 2006, 58, 1456–1459, DOI:10.1016/j.addr.2006.09.011.
- A. Singh and M. M. Amiji, Application of Nanotechnology in Medical Diagnosis and Imaging, Curr. Opin. Biotechnol., 2022, 74, 241–246, DOI:10.1016/j.copbio.2021.12.011.
- D. Lombardo, M. A. Kiselev and M. T. Caccamo, Smart Nanoparticles for Drug Delivery Application: Development of Versatile Nanocarrier Platforms in Biotechnology and Nanomedicine, J. Nanomater., 2019, 2019, 1–26, DOI:10.1155/2019/3702518.
- E. Bevacqua, M. A. Occhiuzzi, F. Grande and P. Tucci, TiO2-NPs Toxicity and Safety: An Update of the Findings Published over the Last Six Years, Mini-Rev. Med. Chem., 2023, 23, 1050–1057, DOI:10.2174/1389557522666220929152403.
- A. Simon-Deckers, S. Loo, M. Mayne-L'hermite, N. Herlin-Boime, N. Menguy, C. Reynaud, B. Gouget and M. Carrière, Size-, Composition- and Shape-Dependent Toxicological Impact of Metal Oxide Nanoparticles and Carbon Nanotubes toward Bacteria, Environ. Sci. Technol., 2009, 43, 8423–8429, DOI:10.1021/es9016975.
- H. Zhang and G. Chen, Potent Antibacterial Activities of Ag/TiO 2 Nanocomposite Powders Synthesized by a One-Pot Sol−Gel Method, Environ. Sci. Technol., 2009, 43, 2905–2910, DOI:10.1021/es803450f.
- Z. A. Al Othman, M. M. Alam, M. Naushad, I. Inamuddin and M. F. Khan, Inorganic Nanoparticles and Nanomaterials Based on Titanium (Ti): Applications in Medicine, Mater. Sci. Forum, 2013, 754, 21–87, DOI:10.4028/www.scientific.net/MSF.754.21.
- S. Parham, D. H. B. Wicaksono, S. Bagherbaigi, S. L. Lee and H. Nur, Antimicrobial Treatment of Different Metal Oxide Nanoparticles: A Critical Review, J. Chin. Chem. Soc., 2016, 63, 385–393, DOI:10.1002/jccs.201500446.
- A. A. Chiş, L. L. Rus, C. Morgovan, A. M. Arseniu, A. Frum, A. L. Vonica-Tincu, F. G. Gligor, M. L. Mureşan and C. M. Dobrea, Microbial Resistance to Antibiotics and Effective Antibiotherapy, Biomedicines, 2022, 10, 1121, DOI:10.3390/biomedicines10051121.
- H. Qadri, A. H. Shah and M. Mir, Novel Strategies to Combat the Emerging Drug Resistance in Human Pathogenic Microbes, Curr. Drug Targets, 2021, 22, 1424–1436, DOI:10.2174/1389450121666201228123212.
- J. Tanwar, S. Das, Z. Fatima and S. Hameed, Multidrug Resistance: An Emerging Crisis, Interdiscip. Perspect. Infect. Dis., 2014, 2014, 1–7, DOI:10.1155/2014/541340.
- H. S. Sader, M. Castanheira, L. R. Duncan and R. K. Flamm, Antimicrobial Susceptibility of Enterobacteriaceae and Pseudomonas Aeruginosa Isolates from United States Medical Centers Stratified by Infection Type: Results from the International Network for Optimal Resistance Monitoring (INFORM) Surveillance Program, Diagn. Microbiol. Infect. Dis., 2018, 92, 69–74, DOI:10.1016/j.diagmicrobio.2018.04.012.
- J. P. Horcajada, M. Montero, A. Oliver, L. Sorlí, S. Luque, S. Gómez-Zorrilla, N. Benito and S. Grau, Epidemiology and Treatment of Multidrug-Resistant and Extensively Drug-Resistant Pseudomonas Aeruginosa Infections, Clin. Microbiol. Rev., 2019, 32, e00031, DOI:10.1128/CMR.00031-19.
- K. H. Turner, J. Everett, U. Trivedi, K. P. Rumbaugh and M. Whiteley, Requirements for Pseudomonas Aeruginosa Acute Burn and Chronic Surgical Wound Infection, PLoS Genet., 2014, 10, e1004518, DOI:10.1371/journal.pgen.1004518.
- M. E. Zegans, A. DiGiandomenico, K. Ray, A. Naimie, A. E. Keller, C. K. Stover, P. Lalitha, M. Srinivasan, N. R. Acharya and T. M. Lietman, Association of Biofilm Formation, Psl Exopolysaccharide Expression, and Clinical Outcomes in Pseudomonas Aeruginosa Keratitis, JAMA Ophthalmol., 2016, 134, 383, DOI:10.1001/jamaophthalmol.2015.5956.
- E. Mowat, S. Paterson, J. L. Fothergill, E. A. Wright, M. J. Ledson, M. J. Walshaw, M. A. Brockhurst and C. Winstanley, Pseudomonas Aeruginosa Population Diversity and Turnover in Cystic Fibrosis Chronic Infections, Am. J. Respir. Crit. Care Med., 2011, 183, 1674–1679, DOI:10.1164/rccm.201009-1430OC.
- M. Whiteley, S. P. Diggle and E. P. Greenberg, Progress in and Promise of Bacterial Quorum Sensing Research, Nature, 2017, 551, 313–320, DOI:10.1038/nature24624.
- E. C. Pesci, J. B. J. Milbank, J. P. Pearson, S. McKnight, A. S. Kende, E. P. Greenberg and B. H. Iglewski, Quinolone Signaling in the Cell-to-Cell Communication System of Pseudomonas Aeruginosa, Proc. Natl. Acad. Sci. U. S. A., 1999, 96, 11229–11234, DOI:10.1073/pnas.96.20.11229.
- S. Azimi, A. D. Klementiev, M. Whiteley and S. P. Diggle, Bacterial Quorum Sensing During Infection, Annu. Rev. Microbiol., 2020, 74, 201–219, DOI:10.1146/annurev-micro-032020-093845.
- S. D. Mahlen, Serratia Infections: From Military Experiments to Current Practice, Clin. Microbiol. Rev., 2011, 24, 755–791, DOI:10.1128/CMR.00017-11.
- P. Gastmeier, A. Loui, S. Stamm-Balderjahn, S. Hansen, I. Zuschneid, D. Sohr, M. Behnke, M. Obladen, R.-P. Vonberg and H. Rüden, Outbreaks in Neonatal Intensive Care Units—They Are Not like Others, Am. J. Infect. Control, 2007, 35, 172–176, DOI:10.1016/j.ajic.2006.07.007.
- X. Zhao, Z. Yu and T. Ding, Quorum-Sensing Regulation of Antimicrobial Resistance in Bacteria, Microorganisms, 2020, 8, 425, DOI:10.3390/microorganisms8030425.
- M. Schuster, D. Joseph Sexton, S. P. Diggle and E. Peter Greenberg, Acyl-Homoserine Lactone Quorum Sensing: From Evolution to Application, Annu. Rev. Microbiol., 2013, 67, 43–63, DOI:10.1146/annurev-micro-092412-155635.
- M. Altaf, M. T. Zeyad, M. A. Hashmi, S. Manoharadas, S. A. Hussain, M. S. Ali Abuhasil and M. A. M. Almuzaini, Effective Inhibition and Eradication of Pathogenic Biofilms by Titanium Dioxide Nanoparticles Synthesized Using Carum Copticum Extract, RSC Adv., 2021, 11, 19248–19257, 10.1039/D1RA02876F.
- M. Zubair, F. M. Husain, F. A. Qais, P. Alam, I. Ahmad, T. Albalawi, N. Ahmad, M. Alam, M. H. Baig and J.-J. Dong, et al., Bio-Fabrication of Titanium Oxide Nanoparticles from Ochradenus Arabicus to Obliterate Biofilms of Drug-Resistant Staphylococcus Aureus and Pseudomonas Aeruginosa Isolated from Diabetic Foot Infections, Appl. Nanosci., 2021, 11, 375–387, DOI:10.1007/s13204-020-01630-5.
- F. A. Qais, M. S. Khan, I. Ahmad, F. M. Husain, A. A. Al-kheraif, M. Arshad and P. Alam, Plumbagin Inhibits Quorum Sensing-Regulated Virulence and Biofilms of Gram-Negative Bacteria: In Vitro and in Silico Investigations, Biofouling, 2021, 37, 724–739, DOI:10.1080/08927014.2021.1955250.
- F. A. Qais, M. S. Khan and I. Ahmad, Broad-Spectrum Quorum Sensing and Biofilm Inhibition by Green Tea against Gram-Negative Pathogenic Bacteria: Deciphering the Role of Phytocompounds through Molecular Modelling, Microb. Pathog., 2019, 126, 379–392, DOI:10.1016/j.micpath.2018.11.030.
- M. Kurachi, Studies on the Biosynthesis of Pyocyanine. II. Isolation and Determination of Pyocyanine, Bull. Inst. Chem. Res., Kyoto Univ., 1958, 36, 174–187 Search PubMed.
- F. A. Qais, M. S. Khan, I. Ahmad, F. M. Husain, R. A. Khan, I. Hassan, S. A. Shahzad and W. AlHarbi, Coumarin Exhibits Broad-Spectrum Antibiofilm and Antiquorum Sensing Activity against Gram-Negative Bacteria: In Vitro and In Silico Investigation, ACS Omega, 2021, 6, 18823–18835, DOI:10.1021/acsomega.1c02046.
- F. A. Qais, I. Ahmad, F. M. Husain, M. Arshad, A. Khan and M. Adil, Umbelliferone Modulates the Quorum Sensing and Biofilm of Gram − ve Bacteria: In Vitro and in Silico Investigations, J. Biomol. Struct. Dyn., 2023, 1–14, DOI:10.1080/07391102.2023.2229454.
- F. A. Qais, A. Shafiq, I. Ahmad, F. M. Husain, R. A. Khan and I. Hassan, Green Synthesis of Silver Nanoparticles Using Carum Copticum: Assessment of Its Quorum Sensing and Biofilm Inhibitory Potential against Gram Negative Bacterial Pathogens, Microb. Pathog., 2020, 144, 104172, DOI:10.1016/j.micpath.2020.104172.
- F. A. Qais, I. Ahmad, F. M. Husain, S. Y. Alomar, N. Ahmad, F. Albalawi, P. Alam and T. Albalawi, Interference of Quorum Sensing Regulated Bacterial Virulence Factors and Biofilms by Plumbago Zeylanica Extract, Microsc. Res. Tech., 2021, 84, 3150–3160, DOI:10.1002/jemt.23872.
- H. Slater, M. Crow, L. Everson and G. P. C. Salmond, Phosphate Availability Regulates Biosynthesis of Two Antibiotics, Prodigiosin and Carbapenem, in Serratia via Both Quorum-Sensing-Dependent and -Independent Pathways, Mol. Microbiol., 2008, 47, 303–320, DOI:10.1046/j.1365-2958.2003.03295.x.
- M. Rosenberg, D. Gutnick and E. Rosenberg, Adherence of Bacteria to Hydrocarbons: A Simple Method for Measuring Cell-Surface Hydrophobicity, FEMS Microbiol. Lett., 1980, 9, 29–33, DOI:10.1111/j.1574-6968.1980.tb05599.x.
- M. E. Peek, A. Bhatnagar, N. A. McCarty and S. M. Zughaier, Pyoverdine, the Major Siderophore in Pseudomonas Aeruginosa , Evades NGAL Recognition, Interdiscip. Perspect. Infect. Dis., 2012, 2012, 1–10, DOI:10.1155/2012/843509.
- A. Stintzi, K. Evans, J. Meyer and K. Poole, Quorum-Sensing and Siderophore Biosynthesis in Pseudomonas Aeruginosa: LasRllasI Mutants Exhibit Reduced Pyoverdine Biosynthesis, FEMS Microbiol. Lett., 1998, 166, 341–345, DOI:10.1111/j.1574-6968.1998.tb13910.x.
- J. M. Meyer, A. Neely, A. Stintzi, C. Georges and I. A. Holder, Pyoverdin Is Essential for Virulence of Pseudomonas Aeruginosa, Infect. Immun., 1996, 64, 518–523 CrossRef CAS PubMed.
- F. A. Qais, I. Ahmad, M. Altaf and S. H. Alotaibi, Biofabrication of Gold Nanoparticles Using Capsicum Annuum Extract and Its Antiquorum Sensing and Antibiofilm Activity against Bacterial Pathogens, ACS Omega, 2021, 6, 16670–16682, DOI:10.1021/acsomega.1c02297.
- G. W. Lau, D. J. Hassett, H. Ran and F. Kong, The Role of Pyocyanin in Pseudomonas Aeruginosa Infection, Trends Mol. Med., 2004, 10, 599–606, DOI:10.1016/j.molmed.2004.10.002.
- J. L. Fothergill, S. Panagea, C. A. Hart, M. J. Walshaw, T. L. Pitt and C. Winstanley, Widespread Pyocyanin Over-Production among Isolates of a Cystic Fibrosis Epidemic Strain, BMC Microbiol., 2007, 7, 45, DOI:10.1186/1471-2180-7-45.
- R. C. Hunter, V. Klepac-Ceraj, M. M. Lorenzi, H. Grotzinger, T. R. Martin and D. K. Newman, Phenazine Content in the Cystic Fibrosis Respiratory Tract Negatively Correlates with Lung Function and Microbial Complexity, Am. J. Respir. Cell Mol. Biol., 2012, 47, 738–745, DOI:10.1165/rcmb.2012-0088OC.
- D. V. Mavrodi, R. F. Bonsall, S. M. Delaney, M. J. Soule, G. Phillips and L. S. Thomashow, Functional Analysis of Genes for Biosynthesis of Pyocyanin and Phenazine-1-Carboxamide from Pseudomonas Aeruginosa PAO1, J. Bacteriol., 2001, 183, 6454–6465, DOI:10.1128/JB.183.21.6454-6465.2001.
- T. Das, S. K. Kutty, R. Tavallaie, A. I. Ibugo, J. Panchompoo, S. Sehar, L. Aldous, A. W. S. Yeung, S. R. Thomas and N. Kumar, et al., Phenazine Virulence Factor Binding to Extracellular DNA Is Important for Pseudomonas Aeruginosa Biofilm Formation, Sci. Rep., 2015, 5, 8398, DOI:10.1038/srep08398.
- H. Ran, D. J. Hassett and G. W. Lau, Human Targets of Pseudomonas Aeruginosa Pyocyanin, Proc. Natl. Acad. Sci. U. S. A., 2003, 100, 14315–14320, DOI:10.1073/pnas.2332354100.
- N. A. Al-Shabib, F. M. Husain, N. Ahmad, F. A. Qais, A. Khan, A. Khan, M. S. Khan, J. M. Khan, S. A. Shahzad and I. Ahmad, Facile Synthesis of Tin Oxide Hollow Nanoflowers Interfering with Quorum Sensing-Regulated Functions and Bacterial Biofilms, J. Nanomater., 2018, 2018, 1–11, DOI:10.1155/2018/6845026.
- I. A. Holder and C. G. Haidaris, Experimental Studies of the Pathogenesis of Infections Due to Pseudomonas Aeruginosa : Extracellular Protease and Elastase as in Vivo Virulence Factors, Can. J. Microbiol., 1979, 25, 593–599, DOI:10.1139/m79-085.
- R. S. Smith, B. H. Iglewski and H. P. Barbara, Aeruginosa Quorum-Sensing Systems and Virulence, Curr. Opin. Microbiol., 2003, 6, 56–60, DOI:10.1016/S1369-5274(03)00008-0.
- C. Liao, X. Huang, Q. Wang, D. Yao and W. Lu, Virulence Factors of Pseudomonas Aeruginosa and Antivirulence Strategies to Combat Its Drug Resistance, Front. Cell. Infect. Microbiol., 2022, 12, 926758, DOI:10.3389/fcimb.2022.926758.
- S. Bleves, V. Viarre, R. Salacha, G. P. F. Michel, A. Filloux and R. Voulhoux, Protein Secretion Systems in Pseudomonas Aeruginosa: A Wealth of Pathogenic Weapons, Int. J. Med. Microbiol., 2010, 300, 534–543, DOI:10.1016/j.ijmm.2010.08.005.
- T. Matsumoto, K. Tateda, N. Furuya, S. Miyazaki, A. Ohno, Y. Ishii, Y. Hirakata and K. Yamaguchi, Efficacies of Alkaline Protease, Elastase and Exotoxin A Toxoid Vaccines against Gut-Derived Pseudomonas Aeruginosa Sepsis in Mice, J. Med. Microbiol., 1998, 47, 303–308, DOI:10.1099/00222615-47-4-303.
- J. L. Bradshaw, A. R. Caballero, M. A. Bierdeman, K. V. Adams, H. R. Pipkins, A. Tang, R. J. O’Callaghan and L. S. McDaniel, Pseudomonas Aeruginosa Protease IV Exacerbates Pneumococcal Pneumonia and Systemic Disease, mSphere, 2018, 3, e00212, DOI:10.1128/mSphere.00212-18.
- L. S. Engel, J. M. Hill, A. R. Caballero, L. C. Green and R. J. O'Callaghan, Protease IV, a Unique Extracellular Protease and Virulence Factor from Pseudomonas Aeruginosa, J. Biol. Chem., 1998, 273, 16792–16797, DOI:10.1074/jbc.273.27.16792.
- J. L. Malloy, R. A. W. Veldhuizen, B. A. Thibodeaux, R. J. O'Callaghan and J. R. Wright, Pseudomonas Aeruginosa Protease IV Degrades Surfactant Proteins and Inhibits Surfactant Host Defense and Biophysical Functions, Am. J. Physiol. Lung Cell Mol. Physiol., 2005, 288, L409–L418, DOI:10.1152/ajplung.00322.2004.
- Z. Cheng, A Pseudomonas Aeruginosa -Secreted Protease Modulates Host Intrinsic Immune Responses, but How?, BioEssays, 2016, 38, 1084–1092, DOI:10.1002/bies.201600101.
- R. O'Callaghan, A. Caballero, A. Tang and M. Bierdeman, Pseudomonas Aeruginosa Keratitis: Protease IV and PASP as Corneal Virulence Mediators, Microorganisms, 2019, 7, 281, DOI:10.3390/microorganisms7090281.
- M. B. K. Bandara, H. Zhu, P. R. Sankaridurg and M. D. P. Willcox, Salicylic Acid Reduces the Production of Several Potential Virulence Factors of Pseudomonas Aeruginosa Associated with Microbial Keratitis, Investig. Ophthalmol. Vis. Sci., 2006, 47, 4453, DOI:10.1167/iovs.06-0288.
- P. Gupta, R. Gupta and K. Harjai, Multiple Virulence Factors Regulated by Quorum Sensing May Help in Establishment and Colonisation of Urinary Tract by Pseudomonas Aeruginosa during Experimental Urinary Tract Infection, Indian J. Med. Microbiol., 2013, 31, 29–33, DOI:10.4103/0255-0857.108715.
- L. Zulianello, C. Canard, T. Kohler, D. Caille, J.-S. Lacroix and P. Meda, Rhamnolipids Are Virulence Factors That Promote Early Infiltration of Primary Human Airway Epithelia by Pseudomonas Aeruginosa, Infect. Immun., 2006, 74, 3134–3147, DOI:10.1128/IAI.01772-05.
- C. O'May and N. Tufenkji, The Swarming Motility of Pseudomonas Aeruginosa Is Blocked by Cranberry Proanthocyanidins and Other Tannin-Containing Materials, Appl. Environ. Microbiol., 2011, 77, 3061–3067, DOI:10.1128/AEM.02677-10.
- N. M. Ali, S. Chatta, I. Liaqat, S. A. Mazhar, B. Mazhar and S. Zahid, Pseudomonas Aeruginosa Associated Pulmonary Infections and in Vitro Amplification Virulent Rhamnolipid (RhlR) Gene, Braz. J. Biol., 2021, 82, e228009, DOI:10.1590/1519-6984.228009.
- J. W. Newman, R. V. Floyd and J. L. Fothergill, The Contribution of Pseudomonas Aeruginosa Virulence Factors and Host Factors in the Establishment of Urinary Tract Infections, FEMS Microbiol. Lett., 2017, 364, fnx124, DOI:10.1093/femsle/fnx124.
- F. A. Qais, I. Ahmad, M. Altaf, S. Manoharadas, B. F. Al-Rayes, M. S. Ali Abuhasil and Y. A. Almaroai, Biofabricated Silver Nanoparticles Exhibit Broad-Spectrum Antibiofilm and Antiquorum Sensing Activity against Gram-Negative Bacteria, RSC Adv., 2021, 11, 13700–13710, 10.1039/D1RA00488C.
- J.-R. Wei and H.-C. Lai, N-Acylhomoserine Lactone-Dependent Cell-to-Cell Communication and Social Behavior in the Genus Serratia, Int. J. Med. Microbiol., 2006, 296, 117–124, DOI:10.1016/j.ijmm.2006.01.033.
- Y. Kida, H. Inoue, T. Shimizu and K. Kuwano, Serratia Marcescens Serralysin Induces Inflammatory Responses through Protease-Activated Receptor 2, Infect. Immun., 2007, 75, 164–174, DOI:10.1128/IAI.01239-06.
- R. Van Houdt, M. Givskov and C. W. Michiels, Quorum Sensing in Serratia, FEMS Microbiol. Rev., 2007, 31, 407–424, DOI:10.1111/j.1574-6976.2007.00071.x.
- E. Schiebel and V. Braun, Integration of the Serratia Marcescens Haemolysin into Human Erythrocyte Membranes, Mol. Microbiol., 1989, 3, 445–453, DOI:10.1111/j.1365-2958.1989.tb00190.x.
- W. König, Y. Faltin, J. Scheffer, H. Schöffler and V. Braun, Role of Cell-Bound Hemolysin as a Pathogenicity Factor for Serratia Infections, Infect. Immun., 1987, 55, 2554–2561, DOI:10.1128/iai.55.11.2554-2561.1987.
- R. Salini and S. K. Pandian, Interference of Quorum Sensing in Urinary Pathogen Serratia Marcescens by Anethum Graveolens, Pathog. Dis., 2015, 73, ftv038, DOI:10.1093/femspd/ftv038.
- R. Srinivasan, K. R. Devi, A. Kannappan, S. K. Pandian and A. V. Ravi, Piper Betle and Its Bioactive Metabolite Phytol Mitigates Quorum Sensing Mediated Virulence Factors and Biofilm of Nosocomial Pathogen Serratia Marcescens in Vitro, J. Ethnopharmacol., 2016, 193, 592–603, DOI:10.1016/j.jep.2016.10.017.
|
This journal is © The Royal Society of Chemistry 2023 |