DOI:
10.1039/D3RA03790H
(Paper)
RSC Adv., 2023,
13, 22079-22087
Enzyme and pH dual responsive linear-dendritic block copolymer micelles based on a phenylalanyl–lysine motif and peripherally ketal-functionalized dendron as potential drug carriers†
Received
6th June 2023
, Accepted 12th July 2023
First published on 21st July 2023
Abstract
Stimuli–responsive linear-dendritic block copolymers (LDBCs) have attracted significant research attention as novel drug carriers. We report here three generations of new enzyme and pH dual responsive linear-dendritic block copolymers (LDBCs) with a phenylalanyl–lysine (Phe–Lys) dipeptide linking hydrophilic linear poly(N-vinylpyrrolidone) (PNVP) and a hydrophobic peripherally ketal-functionalized dendron derived from 2,2′-bis(hydroxymethyl)propionic acid (bis-MPA). The LDBCs are synthesized via a combination of interchange of xanthates/reversible addition–fragmentation chain transfer (MADIX/RAFT) polymerization of N-vinylpyrrolidone (NVP) and “chain-first” strategy. Their structures are confirmed by 1H NMR spectra. The gel permeation chromatograph (GPC) analysis revealed that the LDBCs have a narrow molecular weight distribution (PDI ≤ 1.25). The amphiphilic LDBCs can self-assemble into spherical nanomicelles in aqueous solution. The presence of enzyme or/and the change of pH cause disassembly of micelles to release encapsulated cargos. The release rates of the guest molecules are faster in buffer solution at pH 5.0 than those upon the addition of the activating enzyme and can be fine-tuned by changing the generation of bis-MPA dendrons. The combination of enzyme and pH dual stimuli results in significantly accelerated and more complete release of the loaded hydrophobic guests. The cell viability assay confirmed the favorable biocompatibility until the LDBC micelle concentration reached 800 μg mL−1. These results indicate that the LDBCs can be considered as a good candidate for targeting drug delivery.
Introduction
Stimuli–responsive linear-dendritic block copolymers (LDBCs) have drawn increasing research interest in recent years because of combining the ability to respond to various stimuli (such as light, temperature, pH and enzymes) and different polymeric architectures for a broad range of applications, including drug delivery, gene therapy and materials science.1–8 In this regard, enzyme responsive LDBCs are especially intriguing owing to high selectivity and favorable efficiency of enzyme-catalyzed reactions.9 Moreover, since some enzymes are overexpressed in diseased states, by incorporating enzyme labile linkages, polymeric nanoparticles can be constructed to release the loaded drug at desired sites, and are excellent candidates for drug targeted delivery.10–12 Amir's group has reported a series of LDBCs based on a linear hydrophilic polyethyleneglycol (PEG) and a dendron with enzyme-cleavable hydrophobic end groups.9,13–15 Our group obtained some LDBCs composed of a hydrophilic linear enzyme responsive poly(hydroxyethyl L-glutamine) (PHEG) and a hydrophobic dendron derived from cysteamine or 2,2′-bis(hydroxymethyl)propionic acid (bis-MPA).16 Some LDBCs based on linear poly(N-vinylpyrrolidone) (PNVP) and enzyme responsive dendritic phenylalanyl–lysine (Phe–Lys) dipeptides have also been synthesized.17 The enzyme responsive moieties of these LDBCs have been incorporated into their linear chain, dendritic blocks and periphery of dendrons. However, to date, there has been no any report on an enzyme–responsive motif is introduced at the junction point between the hydrophilic linear chain and hydrophobic dendritic block of amphiphilic LDBCs.
In the past decade, pH–responsive LDBCs have been developed for the application of targeted and controlled drug delivery since the numerous pH gradients exist in both normal and pathophysiological states.18 Many pH–responsive units such as carboxylic acid,19,20 amino-ester,21 amino acid,22 amines,23 acetal groups5,24 and hydrazone bonds25 have been incorporated into LDBCs. The pH–responsive polymers with highly sensitive ketal linkages have generated significant interest as promising candidates for tumor-targeted drug delivery. This is attributed to their distinctive features that ketal linkages hydrolyze into neutral alcohols and ketones could avoiding inflammatory problems compared with the acidic degradation products of polyesters and polyorthoesters.26 Moreover, ketal linkages were more sensitive to the lower pH environment of tumors or phagosomes than hydrazones but were more stable than these linkages in the pH 7.4 of the blood.27 However, to the best of our knowledge, the pH–responsive LDBCs with ketal peripheries have so far not been reported.
Although many single stimulus responsive LDBCs have been obtained, dual responsive LDBCs have been relatively underexplored, especially systems that exhibit enzyme responsive behavior.24,28,29 There are large variations in physiological conditions between normal microenvironments and diseased sites, thus single responsive polymer materials could not achieve the desired goals in a complex physiological microenvironment. The integration of several responsive moieties within one polymer is highly desired for biomedical applications.30–32 In particular, the combination of enzyme and pH stimuli is appropriate to design polymer nanocarriers for tumour targeted delivery of drugs since lower pH values in tumours relative to normal tissues.33–35 Additionally, it is well known that some enzymes (such as matrix metallo proteinases,36 cathepsin B,37 alkaline phosphatase,38 trypsin39) are overexpressed in tumor cells due to the needs of proliferation.
In this study, an enzyme cleavable phenylalanyl–lysine (Phe–Lys) dipeptide is used as a linkage between hydrophilic linear poly(N-vinylpyrrolidone) (PNVP) and a hydrophobic peripherally ketal-functionalized dendron derived from 2,2′-dimethylolpropionic acid (bis-MPA) to obtain enzyme and pH dual responsive LDBCs. Their self-assembly behavior in aqueous solution and the release kinetics of the encapsulated guest molecules in response to enzyme or pH stimulus and combination of dual stimuli have been investigated. The cytotoxicity of the LDBCs was tested in human lung epithelial cells (BEAS-2B) and human liver cancer cells (SMMC-7721). The LDBCs could self-assemble into spherical nanomicelles and disassembly to release loaded drug in various microenvironment which typically exist in cancer cells (Scheme 1).
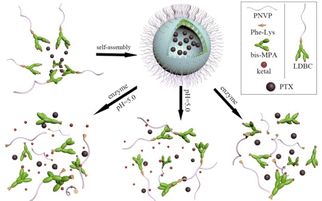 |
| Scheme 1 Illustration of self-assembly and disassembly of the LDBCs to release encapsulated cargos in presence of enzyme or/and the change of pH. | |
Experimental
Materials
α,α-Azobisisobutyronitrile (AIBN) (98%, Aldrich) was recrystallized twice from methanol. N-Vinylpyrrolidone (NVP) (98%, Sinopharm Chemical Reagent) was distilled under reduced pressure and then stored at 4 °C. Isopropylidene-2,2-bis(oxymethyl)propionic anhydride was prepared according to literature method.40,41
Characterization
1H NMR spectra were obtained on a Bruker DRX-500 spectrometer in CDCl3 or D2O. GPC analyses were performed on a Waters 2690D separations module and a Waters 2414 refractive index detector (RI) with Styragel HR3 and HR4 columns (Waters) using DMF as eluent at 40 °C at a flow rate of 0.3 mL min−1. The system was calibrated with poly(methyl methacrylate) standards. Transmission electron microscopy (TEM) images were obtained using a JEM-2100 transmission electron microscope operating at an accelerating voltage of 200 kV. Size and distribution of the nanoparticles were characterized by ZetaPALS particle sizing systems (Brookhaven instruments).
Synthesis of PNVP-Phe-NH2
PNVP-Phe-NH2 was synthesized according to the method reported in our previous work.15 1H NMR in CDCl3, δ (ppm): 1.19–1.28 (m, CH3), 1.52–1.89 (m, CH2), 1.89–2.15 (m, CH2), 2.15–2.96 (m, O
CCH2), 2.99–3.48 (m, NCH2), 3.48–4.08 (m, NCH), 7.01–7.53 (m, C6H5).
Synthesis of PNVP-Phe-Lys-NHBoc
To a solution of (Fmoc)lys(Boc)-OH (0.18 g, 0.30 mmol) in 10 mL of DMF was added PNVP-Phe-NH2 (1.2 g, 0.1 mmol) and 1-hydroxybenzotriazole (HOBt) (13.51 mg, 0.1 mmol). After stirring for 30 min, N,N-diisopropylcarbodiimide (DIC) (0.06 mL, 0.4 mmol) was added. The reaction mixture was stirred at room temperature for 24 h. The crude product were precipitated and washed by cold ether. The precipitate was dissolved in distilled water, dialyzed for 3 days, and lyophilized to give a white solid. The yield was 97.5%. 1H NMR in CDCl3, δ (ppm): 1.18–1.29 (m, CH3), 1.51–1.89 (m, CH2), 1.89–2.15 (m, CH2), 2.15–2.95 (m, O
CCH2), 2.95–3.47 (m, NCH2), 3.47–4.10 (m, NCH), 7.02–7.54 (m, Ar–H).
Synthesis of PNVP-Phe-Lys-NH2
To a solution of PNVP-Phe-Lys-NHBoc (0.1 mmol) in 4 mL of DMF was added trifluoroacetic acid to adjust the pH value of the solution to 4–5. After stirring for 6 h, the reaction mixture was neutralized with NaOH solution. After filtration and evaporation of the solvent, the residue was dissolved in water, dialyzed for 3 days, and then lyophilized to obtain a white solid product in a yield of 84.4%. 1H NMR in CDCl3, δ (ppm): 1.18–1.29 (m, CH3), 1.52–1.89 (m, CH2), 1.89–2.14 (m, CH2), 2.14–2.95 (m, O
CCH2), 2.95–3.48 (m, NCH2), 3.48–4.09 (m, NCH), 7.02–7.53 (m, Ar–H).
Synthesis of PNVP-Phe-Lys-b-G1
PNVP-Phe-Lys-NH2 (1.25 g, 0.1 mmol), isopropylidene-2,2-bis(oxymethyl)propionic anhydride (49.6 mg, 0.15 mmol) and DMAP (2.4 mg, 0.02 mmol) were dissolved in 20 mL of DMF/NEt3 solution (v/v = 3/1). After stirring for 40 h at room temperature, the reaction mixture was precipitated three times by cold ether. The precipitate was collected and dissolved in distilled water, dialyzed for 3 days, and then lyophilized to obtain a white solid product in a yield of 92.3%. 1H NMR in CDCl3, δ (ppm): 1.19–1.29 (m, CH3), 1.42 (s, CH3), 1.50–1.88 (m, CH2), 1.88–2.14 (m, CH2), 2.14–2.63 (m, O
CCH2), 2.91–3.48 (m, NCH2), 3.48–4.09 (m, NCH), 7.01–7.52 (m, Ar–H).
Synthesis of PNVP-Phe-Lys-b-G1-OH
1.27 g (0.1 mmol) of PNVP-Phe-Lys-b-G1 was dissolved in 50 mL of THF/methanol solution (v/v = 4/3). H2SO4 was added to adjust the pH value of the solution to 3. After stirring for 20 h at room temperature, the reaction mixture was neutralized with NH3·H2O and filtered to remove ammonium sulfate. The solvent was distilled off and the residue was added to distilled water, dialyzed for 3 days, and then lyophilized to obtain a white solid in a yield of 80.1%. 1H NMR in CDCl3, δ (ppm): 1.20–1.30(m, CH3), 1.50–1.89 (m, CH2), 1.89–2.14 (m, CH2), 2.14–2.65 (m, O
CCH2), 2.92–3.47 (m, NCH2), 3.48–4.08 (m, NCH), 4.24–4.39 (m, OCH2), 7.02–7.50 (m, Ar–H).
Synthesis of PNVP-Phe-Lys-b-G2
PNVP-Phe-Lys-b-G1-OH (1.27 g, 0.1 mmol), isopropylidene-2,2-bis(oxymethyl)propionic anhydride (0.40 g, 1.2 mmol) and (0.15 g, 1.2 mmol) of DMAP were dissolved in 5 mL of pyridine/CH2Cl2 solution (v/v = 3/2). After stirring for 48 h at room temperature, the reaction mixture was precipitated three times by cold ether. The precipitate was collected and dissolved in water, dialyzed for 3 days, and then lyophilized to obtain a white solid product in a yield of 89.2%. 1H NMR in CDCl3, δ (ppm): 1.19–1.30 (m, CH3), 1.42 (s, CH3), 1.52–1.89 (m, CH2), 1.89–2.14 (m, CH2), 2.14–2.62 (m, O
CCH2), 2.94–3.48 (m, NCH2), 3.48–4.09 (m, NCH), 4.22–4.38 (m, OCH2), 7.02–7.52 (m, Ar–H).
Synthesis of PNVP-Phe-Lys-b-G2-OH
PNVP-Phe-Lys-b-G2-OH was synthesized by the same general procedure described for the synthesis of PNVP-Phe-Lys-b-G1-OH. From PNVP-Phe-Lys-b-G2 (1.27 g, 0.1 mmol), THF (32 mL), methanol (24 mL) and H2SO4 were obtained as a white solid in a yield of 76.0% (0.99 g). 1H NMR in CDCl3, δ (ppm): 1.21–1.31 (m, CH3), 1.51–1.89 (m, CH2), 1.89–2.14 (m, CH2), 2.14–2.62 (m, O
CCH2), 2.96–3.48 (m, NCH2), 3.49–4.09 (m, NCH), 4.24–4.41 (m, OCH2), 7.02–7.52 (m, Ar–H).
Preparation of PNVP-Phe-Lys-b-G3
PNVP-Phe-Lys-b-G3 was synthesized by the same general procedure described for the synthesis of PNVP-Phe-Lys-b-G2. From DMAP (0.29 g, 2.4 mmol), pyridine (3 mL), CH2Cl2 (3 mL), PNVP-Phe-Lys-b-G2-OH (1.29 g, 0.1 mmol) and isopropylidene-2,2-bis(oxymethyl)propionic anhydride (0.79 g, 2.4 mmol) were obtained as a white solid in a yield of 97.0% (1.25 g). 1H NMR in CDCl3, δ(ppm): 1.21–1.30 (m, CH3), 1.43 (s, CH3), 1.51–1.88 (m, CH2), 1.88–2.14 (m, CH2), 2.14–2.63 (m, O
CCH2), 2.95–3.47 (m, NCH2), 3.49–4.09 (m, NCH), 4.59–4.88 (m, OCH2),7.02–7.54 (m, Ar–H).
Critical micelle concentration (CMC)
The CMC was determined using pyrene as a fluorescence probe according to a procedure as described previously.42
Encapsulation of drug or dye in polymer micelles
PNVP-Phe-Lys-b-Gn (n = 1–3) (10 mg) and Nile red were dissolved in acetone. PBS buffer was added dropwise to the solution under vigorous stirring. Then acetone was thoroughly removed by evaporation at room temperature. The precipitate of un-encapsulated Nile red was removed by centrifugation at 3000 rpm for 10 min. Fluorescence spectra of Nile red were recorded on a luminescence spectrometer. The emission spectra were recorded ranging from 570 to 800 nm with an excitation wavelength of 550 nm.
PNVP-Phe-Lys-b-G3 and PTX were dissolved in ethanol, then deionized water was added slowly after complete dissolution. Ethanol was removed and the residual solution was centrifuged at high speed for 30 minutes. The drug-loaded micelles were prepared by freeze-drying the supernatant. The freeze-dried drug-loaded micelles were diluted with ethanol, their UV absorbance was determined at 227 nm, and the content of PTX in the drug-loaded micelles was calculated. The entrapping efficiency and drug-loading capacity were calculated according to the following formulas:
Transmission electron microscopy (TEM)
A drop of micellar solution (1 mg mL−1, or Nile red-loaded micelles, or dealt with trypsin) was placed on a copper grid coated with a carbon film and dried before TEM measurement.
In vitro release of PTX from PNVP-Phe-Lys-b-G3 micelles
Trypsin solution was added to 15 mL drug-loaded micelle solution (1.0 mg mL−1) or PTX solution, and the final trypsin concentration was 0, 25 and 75 μM, respectively. The solution was placed into pre-swelled dialysis bag (3 kDa) that was immersed into 150 mL PBS solution (pH 7.4, containing 0.5% Tween 80) or acetate buffer solution (pH 5.0, containing 0.5% Tween 80) at 37 °C. At regular intervals of time, 2 mL of aliquots of the solutions were taken out and the amount of released PTX was determined by UV analysis.
Cytotoxicity tests
In vitro cytotoxicity of PNVP-Phe-Lys-b-G2 and PNVP-Phe-Lys-b-G3 micelles against human lung epithelial cells (BEAS-2B) and human liver cancer cells (SMMC-7721) were determined by MTS assay as described previously.17
Results and discussion
Synthesis and characterization of PNVP-Phe-Lys-b-Gn
Three generations of new enzyme and pH responsive LDBCs with a phenylalanyl–lysine (Phe–Lys) dipeptide linking hydrophilic linear poly(N-vinylpyrrolidone) (PNVP) block and a hydrophobic acid-labile acetonide-terminated dendron derived from bis-MPA were synthesized via interchange of xanthates/reversible addition–fragmentation chain transfer polymerization (MADIX/RAFT) of NVP and “chain-first” strategy (Scheme 2). The successful synthesis of the desired LDBCs was confirmed by 1H NMR spectra and GPC. The 1HNMR spectra of PNVP-Phe-Lys-b-Gn (Fig. 1a, S1 and S2, ESI†) displayed characteristic signals attributed to linear PNVP chain (peaks d, e, f, g and h at 1.51–1.88 ppm, 3.49–4.09 ppm, 2.95–3.47 ppm, 1.88–2.14 ppm and 2.14–2.63 ppm, respectively) and acetonide-terminated bis-MPA dendrons (peaks b′, a′ and c′ at 3.49–4.09 ppm, 1.43 ppm, respectively). All copolymers showed unimodal symmetrical GPC curves (Fig. 2) with narrow molecular weight distributions (PDI ≤ 1.25, Table 1) and their Mn,GPC increase with increased generation of the dendron which indicated the synthesis of three generations of LDBCs.
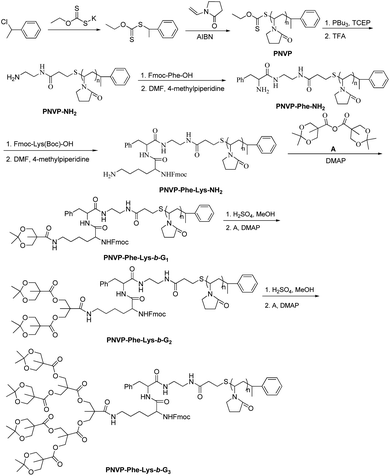 |
| Scheme 2 Synthesis of PNVP-Phe-Lys-b-Gn (n = 1–3). | |
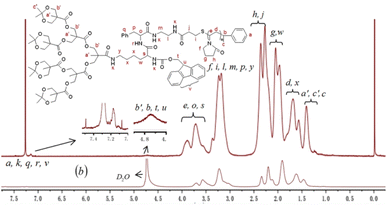 |
| Fig. 1 1H NMR spectra of PNVP-Phe-Lys-b-G3 in CDCl3 (a) and D2O (b). | |
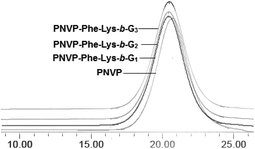 |
| Fig. 2 GPC traces of PNVP-Phe-Lys-b-Gn (n = 1–3). | |
Table 1 Characterizations of PNVP-Phe-Lys-b-Gn (n = 1–3)
Sample |
Mn,th (kDa) |
Mn,GPCc (kDa) |
Đc = Mw/Mn |
Conversion (%) |
The theoretic molecular weight was calculated by the formula: Mn,th = Mmonomer × ([monomer]/[initiator]) × conversion% + Minitiator. The theoretic molecular weight was calculated by the formula: Mn,th = Mn,th of PNVP + Mth of Phe–Lys and Gn (n = 1–3). Mn and Đ were determined by GPC. |
PNVP |
11.9a |
21.2 |
1.24 |
77 |
PNVP-Phe-Lys-b-G1 |
12.7b |
21.4 |
1.25 |
— |
PNVP-Phe-Lys-b-G2 |
13.0b |
21.6 |
1.24 |
— |
PNVP-Phe-Lys-b-G3 |
13.5b |
21.8 |
1.24 |
— |
Self-assembly behavior
PNVP-Phe-Lys-b-Gn (n = 1–3) are amphiphilic and can therefore self-assemble into micelles in aqueous media. The fluorescence probe technique, 1H NMR spectra, transmission electron microscopy (TEM) and particle size analysis were used to examine the self-organization of the amphiphilic LDBCs in water. The critical micelle concentration (CMC) values of PNVP-Phe-Lys-b-Gn (n = 1–3) was evaluated by fluorescence techniques using pyrene as a probe. As shown in Fig. 3, they are 0.056, 0.0316, 0.0158 mg mL−1, respectively, which decrease with the increase of the acetonide-terminated bis-MPA dendrons, indicating that the copolymer is more likely to form stable micelles as the generation of the LDBCs increases.43,44 The self-assembly behavior of the copolymers was further investigated by 1H NMR spectroscopy. When the 1H NMR experiments of the PNVP-Phe-Lys-b-G3 were performed in D2O, the methyl proton peaks of at the end of the acetonide-terminated bis-MPA dendrons were highly suppressed (Fig. 1b), indicating the formation of core–shell micellar structure in the aqueous environment, thereby restricting the motion of methyl protons within the hydrophobic core.45,46 Average hydrodynamic diameters of PNVP-Phe-Lys-b-Gn (n = 1–3) micelles determined by particle sizing measurements ranged from 110 nm to 270 nm (Fig. 4), which increases with increasing the generation of the LDBCs. TEM images showed that PNVP-Phe-Lys-b-G3 was able to self-assemble into spherical micelles (Fig. 5a).
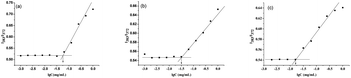 |
| Fig. 3 Plots of I383/I372 of pyrene emission spectra versus logarithm of concentration for PNVP-Phe-Lys-b-G1 (a), PNVP-Phe-Lys-b-G2 (b), PNVP-Phe-Lys-b-G3 (c). | |
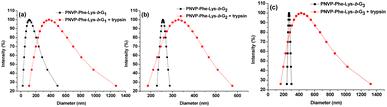 |
| Fig. 4 The particle size of PNVP-Phe-Lys-b-G1 (a), PNVP-Phe-Lys-b-G2 (b), PNVP-Phe-Lys-b-G3 (c) micelles and after the incubation with trypsin (75 μM). | |
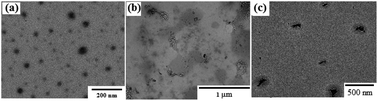 |
| Fig. 5 TEM images of the formed micelles by PNVP-Phe-Lys-b-G3 before (a) and after (b) the enzyme treatment at pH 7.4, at pH 5.0 (c). | |
Enzymatic responsive properties
Trypsin can catalyze the hydrolysis of proteins and has a high degree of specificity for the bond between the carboxyl group of basic amino acids (arginine, lysine) and other amino groups. Its high specificity is expressed as the choice of one end of the basic amino acid.47–50 An enzymatically cleavable Phe–Lys dipeptide was incorporated between a hydrophobic dendron derived from bis-MPA and hydrophilic PNVP of the amphiphilic LDBCs (PNVP-Phe-Lys-b-Gn, n = 1–3). This dipeptide is a substrate for trypsin that is known to be overexpressed in pathological conditions such as cancer and inflammation.39,51 Enzymatic cleavage at the Phe–Lys dipeptide will trigger disassembly of the LDBC micelles. To gain an insight into enzyme responsive disassembly of the micelles, PNVP-Phe-Lys-b-G3 micelle solution was incubated with trypsin solution (75 μM) at 37 °C for 48 h. We observed that a large amount of white flocculation appeared (Fig. S3, ESI†), indicating that the Phe–Lys dipeptide between hydrophilic PNVP and a hydrophobic dendron derived from bis-MPA was disrupted by trypsin to trigger disintegration of the LDBC micelles, thus resulting in precipitation of the hydrophobic portion from solution to produce some white flocculation.
Nile red dye as a model payload was further employed to investigate enzyme responsive disassembly behavior of PNVP-Phe-Lys-b-Gn (n = 1–3) micelles. The fluorescence intensity of Nile red for all three generation of LDBCs showed no significant change in the absence of trypsin (Fig. 6), indicating that Nile red loaded micelles are stable. However, upon the addition of solutions of trypsin (25 μM or 75 μM) for 48 h, we observed that the purple-red dye-loaded micellar solutions of the LDBCs gradually became lighter in color and generated precipitates (Fig. S4, ESI†). In addition, the color of the micellar solution became lighter with increasing enzyme concentration. After 25 μM solution of trypsin was added, all three generation of LDBCs showed that the fluorescence intensity of Nile red decreased (Fig. 6), indicating that trypsin cleaves the peptide bond, which causes the copolymer to decompose into a hydrophilic part and hydrophobic part, and the micelles disassemble. The hydrophobic Nile red contained in the micelles is released, and the environment in which it is changed causes the fluorescence intensity to decrease, and the phenomenon becomes more pronounced with the increase of time. At the same enzyme concentration, the micelle solution color becomes lighter as the polymer generation increases and the disassembly rates of micelles decrease upon increase of the LDBCs generation, because the micelles becomes more stable with increasing hydrophobic peripheral ketal groups of the LDBCs, and trypsin is less accessible to the Phe–Lys dipeptide in the tightly packed higher generation dendrons. Similar phenomena were also observed in other amphiphilic dendrimer systems.52 At the high enzyme concentration of 75 μM, the fluorescence intensity of Nile red was similar to that of trypsin at 25 μM, except that the disassembly rate of micelles was faster as the concentration of trypsin increased. The size change of the LDBC micelles in response to trypsin hydrolysis was followed by particle sizing measurement. As shown in Fig. 4, the particle size of the micelles increased significantly after the addition of trypsin. When the different concentrations of trypsin were added, the particle size of the micelles increased with the increase of the enzyme concentration (Fig. S5, ESI†). This is because high concentrations of trypsin have a greater probability of contact with the micelles to lead to disassembly of the micelles. The enzyme triggered disassembly of the LDBC micelles was confirmed by TEM measurement. As shown in Fig. 5b, small pieces and large aggregates instead of spherical micelles in the presence of trypsin were observed in the TEM images, further confirming enzymatic responsive degradation of the copolymers.
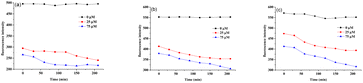 |
| Fig. 6 Comparison of the disassembly rates (fluorescence assay) of micelles formed by PNVP-Phe-Lys-b-G1 (a), PNVP-Phe-Lys-b-G2 (b) and PNVP-Phe-Lys-b-G3 (c) after the incubation with different concentrations of trypsin (0 μM, 25 μM and 75 μM). | |
pH–responsive properties
The pH–responsive disassembly of LDBC micelles was first investigated by monitoring the change in the fluorescence of encapsulated Nile red dyes at pH 5.0 (tumor acidic environment53,54) and pH 7.4 (blood environment55). As plotted in Fig. 7, S6 and S7 (ESI†), no obvious change in the fluorescence intensities was observed throughout 48 h at pH 7.4, indicating that the micelles were stable under neutral conditions. But time dependent decreases in fluorescence intensity of Nile red were observed for all three generation of LDBCs at pH 5.0 (Fig. 8). The spherical nanostructures could no longer be found for PNVP-Phe-Lys-b-G3 in the TEM image of buffer solution at pH 5.0 (Fig. 5c), further confirming pH responsive degradation of the LDBCs. This could be attributed to the fact that the hydrophobic dendritic end groups of the LDBCs are composed of acid-sensitive ketal groups, which can be cleaved under mildly acidic conditions. Upon cleavage of the ketal groups, the LDBCs were converted from amphiphilic to hydrophilic, thereby destroying the assembly of micelles, triggering the release of Nile red and the decrease of fluorescence intensity. Furthermore, we observed that disassembly rates of the LDBC micelles decreased with increase in the generation of bis-MPA dendrons at pH 5.0. This effect of the generation on the disassembly rate is similar to that observed in enzyme triggered disintegration of the LDBC micelles,9 which is probably because the protons in buffer solution could not penetrate into the hydrophobic core of the micelle and instead the hydrolysis of peripheral ketal groups occur at the non-assembled LDBC molecules in the solution, which are in equilibrium with the micellar assemblies.
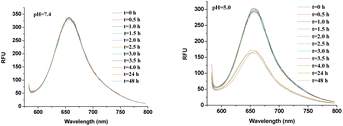 |
| Fig. 7 Fluorescence spectra of Nile red in PNVP-Phe-Lys-b-G3 micelles at pH 7.4 and pH 5.0. | |
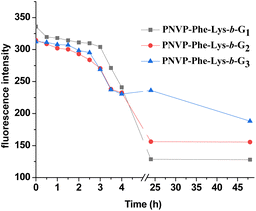 |
| Fig. 8 Comparison of the disassembly rates (fluorescence assay) of micelles formed by PNVP-Phe-Lys-b-G1, PNVP-Phe-Lys-b-G2 and PNVP-Phe-Lys-b-G3 at pH 5.0. | |
Enzyme and pH dual responsive properties
To study enzyme and pH dual responsive disassembly behavior of the LDBCs micelles, the Nile red loaded LDBC solution was subjected to trypsin (75 μM) and acetate buffer of pH 5.0 and fluorescence intensity was monitored with the progress of time. The decrease in fluorescence intensity upon the addition of trypsin at pH 5.0 was much faster than either in the presence of enzyme or buffer solution of pH 5.0 over the same time period (Fig. 9), which corroborates the fact that disassembly rate of the micelles at pH 5.0 can be accelerated by adding trypsin solution. The reason was that simultaneous cleavage of the dipeptide Phe–Lys linkage as well as the peripheral ketal groups of the LDBCs in the presence of pH and enzyme dual stimuli caused a rapid disassembly of the micelles, thus providing an enhancement in the release kinetics of the loaded Nile red.
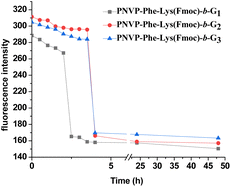 |
| Fig. 9 Comparison of the disassembly rates (fluorescence assay) of micelles formed by PNVP-Phe-Lys-b-G1, PNVP-Phe-Lys-b-G2 and PNVP-Phe-Lys-b-G3 after the incubation with trypsin (75 μM) at pH 5.0. | |
In vitro drug release from PTX-loaded micelles
Paclitaxel (PTX) was used as a model drug to assess the suitability of PNVP-Phe-Lys-b-G3 micelles as anti-cancer drug delivery carriers. The drug loading content and encapsulation efficiency were determined by a UV spectrometer. The results exhibited that PTX was successfully incorporated into LDBC micelles, and the entrapment efficiency (EE) and drug loading content (DLC) were 81.36% and 5.5%, respectively. In comparison with the blank micelles, the size of the PTX-loaded micelles increased to 381 nm (Fig. S8, ESI†). These PTX-loaded LDBC micelles have been found to be very stable, showing no significant changes in average particle size over 30 days (Fig. S8, ESI†). The release of PTX from PNVP-Phe-Lys-b-G3 micelles was investigated in buffer solution (pH 7.4 or pH 5.0) and in presence or absence of 25 μM or 75 μM trypsin at 37 °C (Fig. 10). It could be observed that the release rates of PTX from the micelles were markedly influenced by trypsin. In presence of 25 μM or 75 μM trypsin, the drug loaded micelles showed a much faster drug release rate and 28–42% of the loaded PTX was released within 10 h, while only a small amount of PTX are released within 48 h in absence of trypsin at pH 7.4. Compared with the release at this relatively low trypsin concentration (25 μM), PTX-release from micelles at the high trypsin concentration of 75 μM is much faster. The rate and amount of drug release was found to be much faster at pH 5.0 than that at pH 7.4, exhibiting a stronger dependence of the release rate on pH values. Interestingly, the release rate of PTX from the LDBC micelles in buffer solution at pH 5.0 is faster than those upon the addition of the activating enzyme. This could be attributed to the fact that the protons in buffer solution are more accessible to peripheral ketal groups of the LDBCs while the enzymatic substrate Phe–Lys dipeptide is at the junction point between linear chain and dendritic block of LDBCs, which is less accessible to the enzyme. This result further supported the equilibrium-based activation mechanism, which was described previously for enzyme responsive assemblies9,55 and above mentioned for pH responsive assemblies. More importantly, the fastest PTX release rate was observed in both pH and enzyme stimuli and the accumulative PTX release (83% of PTX released after 48 h) was higher than that in a single stimulus, suggesting that combination of two stimuli could significantly accelerate and more complete release of the loaded drug.
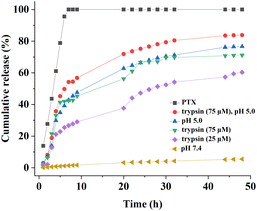 |
| Fig. 10 In vitro PTX release profiles of the PTX-loaded PNVP-Phe-Lys-b-G3 micelles at 37 °C under different stimuli conditions. | |
In vitro cytotoxicity study
Cytotoxic effects of PNVP-Phe-Lys-b-G2 and PNVP-Phe-Lys-b-G3 were investigated using the MTS assay against cultured human lung epithelial cells (BEAS-2B) and human liver cancer cells (SMMC-7721). As shown in Fig. 11, the LDBCs exhibited more than 85% cell viability over a concentration range of 0.05–800 μg mL−1 regardless of cell type after 48 h of culture. In addition, cell viability was not significantly influenced by the concentration of the LDBCs. These results showed that LDBCs have a good biocompatibility within limit concentration and were suitable for drug delivery.
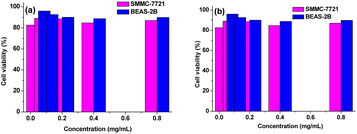 |
| Fig. 11 Cell viability of BEAS-2B and SMMC-7721 cells following 48 h of incubation with PNVP-Phe-Lys-b-G2 (a) and PNVP-Phe-Lys-b-G3 (b) micelles. | |
Conclusions
In summary, a dipeptide Phe–Lys linkage was introduced, as an artificial enzyme active site, at amphiphilic linear-dendritic block copolymers (LDBCs) junction and the LDBCs were decorated with peripheral ketal groups, which endowed their enzyme and pH dual responsiveness. Three generations of well-defined amphiphilic LDBCs, PNVP-Phe-Lys-b-Gn (n = 1–3) were synthesized by a combination of MADIX/RAFT polymerization and “chain-first” strategy. They could self-assemble into spherical nanomicelles and disassembly to release loaded drug in various microenvironment which typically exist in cancer cells. In particular, release of the loaded drug could be tuned by changing the generation of the LDBCs. Additionally, the results of cell viability assays indicated that the LDBCs were nontoxic up to a tested concentration (800 μg mL−1). This study not only enriches the structure types of LDBCs, but also provides novel drug carriers for smart drug delivery systems.
Author contributions
Y. Wang and W. Song: synthesis, investigation, writing-original draft. L. Bao: investigation. J. Wei and Y. Qian: investigation. Y. Bi: conceptualization, supervision, methodology, writing-review & editing, funding acquisition.
Conflicts of interest
There are no conflicts to declare.
Acknowledgements
The authors gratefully acknowledge the support for this study from the National Natural Science Foundation of China (21564017).
Notes and references
- E. Blasco, M. Piñol and L. Oriol, Responsive linear-dendritic block copolymers, Macromol. Rapid Commun., 2014, 35, 1090–1115 CrossRef CAS PubMed.
- G. Liu, W. Liu and C. M. Dong, UV- and NIR-responsive polymeric nanomedicines for on-demand drug delivery, Polym. Chem., 2013, 4, 3431–3443 RSC.
- X. Liu, F. M. Yavitt and I. Gitsov, Supramolecular linear-dendritic nanoreactors: Synthesis and catalytic activity in “green” Suzuki-Miyaura reactions, Polymers, 2023, 15, 1671 CrossRef CAS PubMed.
- L. Zhu, G. Zhu, M. Li, E. Wang, R. Zhu and X. Qi, Thermosensitive aggregates self-assembled by an asymmetric block copolymer of dendritic polyether and poly(N-isopropylacrylamide), Eur. Polym. J., 2002, 38, 2503–2506 CrossRef CAS.
- E. R. Gillies, T. B. Jonsson and J. M. J. Fréchet, Stimuli-responsive supramolecular assemblies of linear-dendritic copolymers, J. Am. Chem. Soc., 2004, 126, 11936–11943 CrossRef CAS PubMed.
- V. Percec, D. Sahoo and J. Adamson, Stimuli-responsive principles of supramolecular organizations emerging from self-assembling and self-organizable dendrons, dendrimers, and dendronized polymers, Polymers, 2023, 15, 1832 CrossRef CAS.
- S. Han, P. Xin, Q. Guo, Z. Cao, H. Huang and J. Wu, Oral delivery of protein drugs via lysine polymer-based nanoparticle platforms, Adv. Healthcare Mater., 2023, 2300311 CrossRef.
- X. You, L. Wang, L. Wang and J. Wu, Rebirth of aspirin synthesis by-product: prickly poly (salicylic acid) nanoparticles as self-anticancer drug carrier, Adv. Funct. Mater., 2021, 31, 2100805 CrossRef CAS.
- A. J. Harnoy, I. Rosenbaum, E. Tirosh, Y. Ebenstein, R. Shaharabani, R. Beck and R. J. Amir, Enzyme-responsive amphiphilic PEG-dendron hybrids and their assembly into smart micellar nanocarriers, J. Am. Chem. Soc., 2014, 136, 7531–7534 CrossRef CAS PubMed.
- J. Hu, G. Zhang and S. Liu, Enzyme-responsive polymeric assemblies, nanoparticles and hydrogels, Chem. Soc. Rev., 2012, 41, 5933–5949 RSC.
- T. Kuang, Y. Liu, T. Gong, X. Peng, X. Hu and Z. Yu, Enzyme-responsive nanoparticles for anticancer drug delivery, Curr. Nanosci., 2016, 12, 38–46 CrossRef CAS.
- Y. Ding, Y. Kang and X. Zhang, Enzyme-responsive polymer assemblies constructed through covalent synthesis and supramolecular strategy, Chem. Commun., 2015, 51, 996–1003 RSC.
- M. Zelzer, S. J. Todd, A. R. Hirst, T. O. McDonald and R. V. Ulijn, Enzyme responsive materials: design strategies and future developments, Biomater. Sci., 2013, 1, 11–39 RSC.
- M. Segal, R. Avinery, M. Buzhor, R. Shaharabani, A. J. Harnoy, E. Tirosh, R. Beck and R. J. Amir, Molecular precision and enzymatic degradation: from readily to undegradable polymeric micelles by minor structural changes, J. Am. Chem. Soc., 2017, 139, 803–810 CrossRef CAS.
- A. J. Harnoy, M. Buzhor, E. Tirosh, R. Shaharabani, R. Beck and R. J. Amir, Modular synthetic approach for adjusting the disassembly rates of enzyme-responsive polymeric micelles, Biomacromolecules, 2017, 18, 1218–1228 CrossRef CAS PubMed.
- Y. Qian, D. You, F. Lin, J. Wei, Y. Wang and Y. Bi, Enzyme triggered disassembly of amphiphilic linear-dendritic block copolymer micelles based on poly[N-(2-hydroxyethyl-l-glutamine)], Polym. Chem., 2019, 10, 94–105 RSC.
- J. Wei, F. Lin, D. You, Y. Qian, Y. Wang and Y. Bi, Self-assembly and enzyme responsiveness of amphiphilic linear-dendritic block copolymers based on poly(N-vinylpyrrolidone) and dendritic phenylalanyl–lysine dipeptides, Polymers, 2019, 11, 1625 CrossRef CAS PubMed.
- E. R. Gillies and J. M. J. Fréchet, pH-responsive copolymer assemblies for controlled release of doxorubicin, Bioconjugate Chem., 2005, 16, 361–368 CrossRef CAS PubMed.
- L. Tian and P. T. Hammond, Comb-dendritic block copolymers as tree-shaped macromolecular amphiphiles for nanoparticle self-assembly, Chem. Mater., 2006, 18, 3976–3984 CrossRef CAS.
- J. C. M. van Hest, M. W. P. L. Baars, C. Elissen-Román, M. H. P. van Genderen and E. W. Meijer, Acid-functionalized amphiphiles, derived from polystyrene-poly (propylene imine) dendrimers, with a pH-dependent aggregation, Macromolecules, 1995, 28, 6689–6691 CrossRef CAS.
- S. Khoee and K. Hemati, Synthesis of magnetite/polyamino-ester dendrimer based on PCL/PEG amphiphilic copolymers via convergent approach for targeted diagnosis and therapy, Polymer, 2013, 54, 5574–5585 CrossRef CAS.
- L. Chen, T. Chen, W. Fang, Y. Wen, S. Lin, J. Lin and C. Cai, Synthesis and pH-responsive “schizophrenic” aggregation of a linear-dendron-like polyampholyte based on oppositely charged polypeptides, Biomacromolecules, 2013, 14, 4320–4330 CrossRef CAS PubMed.
- D. Huang, Y. Wang, F. Yang, H. Shen, Z. Weng and D. Wu, Charge-reversible and pH-responsive biodegradable micelles and vesicles from linear-dendritic supramolecular amphiphiles for anticancer drug delivery, Polym. Chem., 2017, 8, 6675–6687 RSC.
- H. E. Rogers, P. Chambon, S. E. Auty, F. Y. Hern, A. Owen and S. P. Rannard, Synthesis, nanoprecipitation and pH sensitivity of amphiphilic linear–dendritic hybrid polymers and hyperbranched-polydendrons containing tertiary amine functional dendrons, Soft Matter, 2015, 11, 7005–7015 RSC.
- H. Cai, P. Tan, X. Chen, M. Kopytynski, D. Pan, X. Zheng, L. Gu, Q. Gong, X. Tian, Z. Gu, H. Zhang, R. Chen and K. Luo, Stimuli-sensitive linear-dendritic block copolymer-drug prodrug as a nanoplatform for tumor combination therapy, Adv. Mater., 2022, 34, 2108049 CrossRef CAS.
- M. J. Heffernan and N. Murthy, Polyketal nanoparticles: a new pH-sensitive biodegradable drug delivery vehicle, Bioconjugate Chem., 2005, 16, 1340–1342 CrossRef CAS PubMed.
- D. Chen and H. Wang, Novel pH-sensitive biodegradable polymeric drug delivery systems based on ketal polymers, J. Nanosci. Nanotechnol., 2014, 14, 983–989 CrossRef CAS PubMed.
- Y. Qian, J. Wei, Y. Wang, D. You, F. Lin, W. Yue and Y. Bi, Thermal and enzymatic dual-stimuli responsive linear-dendritic block copolymers based on poly(N-vinylcaprolactam), Polym. Adv. Technol., 2020, 31, 2797–2805 CrossRef CAS.
- W. Song, J. Wei, L. Li, Y. Qian, Y. Wang and Y. Bi, Cathepsin B and thermal dual-stimuli responsive linear-dendritic block copolymer micelles for anticancer drug delivery, Polym. Int., 2022, 71, 317–327 CrossRef CAS.
- P. Schattling, F. D. Jochum and P. Theato, Multi-stimuli responsive polymers-the all-in-one talents, Polym. Chem., 2014, 5, 25–36 RSC.
- Z. Q. Cao and G. J. Wang, Multi-stimuli-responsive polymer materials: Particles, films, and bulk gels, Chem. Rec., 2016, 16, 1398–1435 CrossRef CAS PubMed.
- X. Fu, L. Hosta-Rigau, R. Chandrawati and J. Cui, Multi-stimuli-responsive polymer particles, films, and hydrogels for drug delivery, Chem, 2018, 4, 2084–2107 CAS.
- J. L. Wike-Hooley, J. Haveman and H. S. Reinhold, The relevance of tumour pH to the treatment of malignant disease, Radiother. Oncol., 1984, 2, 343–366 CrossRef CAS.
- L. E. Gerweck and K. Seetharaman, Cellular pH gradient in tumor versus normal tissue: potential exploitation for the treatment of cancer, Cancer Res., 1996, 56, 1194–1198 CAS.
- M. Kanamala, W. R. Wilson, M. Yang, B. D. Palmer and Z. Wu, Mechanisms and biomaterials in pH-responsive tumour targeted drug delivery: a review, Biomaterials, 2016, 85, 152–167 CrossRef CAS PubMed.
- K. Kessenbrock, V. Plaks and Z. Werb, Matrix metalloproteinases: regulators of the tumor microenvironment, Cell, 2010, 141, 52–67 CrossRef CAS PubMed.
- S. Ferber, H. Baabur-Cohen, R. Blau, Y. Epshtein, E. Kisin-Finfer, O. Redy, D. Shabat and R. Satchi-Fainar, Polymeric nanotheranostics for real-time non-invasive optical imaging of breast cancer progression and drug release, Cancer Lett., 2014, 352, 81–89 CrossRef CAS PubMed.
- J. Zhang, J. Gao, M. Chen and Z. Yang, Using phosphatases to generate self-assembled nanostructures and their applications, Antioxid. Redox Signaling, 2014, 20, 2179–2190 CrossRef CAS PubMed.
- K. Soreide, E. A. Janssen, H. Körner and J. P. A. Baak, Trypsin in colorectal cancer: molecular biological mechanisms of proliferation, invasion, and metastasis, J. Pathol., 2006, 209, 147–156 CrossRef CAS PubMed.
- E. R. Gillies and J. M. J Fréchet, Designing macromolecules for therapeutic applications: polyester dendrimers poly(ethylene oxide) “bow-tie” hybrids with tunable molecular weight and architecture, J. Am. Chem. Soc., 2002, 124, 14137–14146 CrossRef CAS PubMed.
- M. Malkoch, E. Malmström and A. Hult, Rapid and efficient synthesis of aliphatic ester dendrons and dendrimers, Macromolecules, 2002, 35, 8307–8314 CrossRef CAS.
- G. Tang, M. Hu, Y. Ma, D. You and Y. Bi, Synthesis and solution properties of novel thermo-and pH-responsive poly(N-vinylcaprolactam)-based linea-dendritic block copolymers, RSC Adv., 2016, 6, 42786–42793 RSC.
- J. C. M. van Hest, D. A. P. Delnoye, M. W. P. L. Baars, M. H. P. van Genderen and E. W. Meijer, Polystyrene-dendrimer amphiphilic block copolymers with a generation-dependent aggregation, Science, 1995, 268, 1592–1595 CrossRef CAS PubMed.
- X. Liu and I. Gitsov, Nonionic amphiphilic linear dendritic copolymers. Morphology tuning by solvent-induced self-assembly, Macromolecules, 2019, 52, 5563–5573 CrossRef CAS.
- Y. Li, K. Xiao, J. Luo, J. Lee, S. Pan and K. S. Lam, A novel size-tunable nanocarrier system for targeted anticancer drug delivery, J. Controlled Release, 2010, 144, 314–323 CrossRef CAS PubMed.
- Y. Li, K. Xiao, J. Luo, W. Xiao, J. S. Lee, A. M. Gonik, J. Kato, T. A. Dong and K. S. Lam, Well-defined, reversible disulfide cross-linked micelles for on-demand paclitaxel delivery, Biomaterials, 2011, 32, 6633–6645 CrossRef CAS PubMed.
- J. V. Olsen, S. E. Ong and M. Mann, Trypsin cleaves exclusively C-terminal to arginine and lysine residues, Mol. Cell. Proteomics, 2004, 3, 608–614 CrossRef CAS PubMed.
- K. Rose, R. Stöcklin, L. A. Savoy, P. O. Regamey, R. E. Offord, P. Vuagnat and J. Markussen, Reaction mechanism of trypsin-catalysed semisynthesis of human insulin studied by fast atom bombardment mass spectrometry, Protein Eng., Des. Sel., 1991, 4, 409–412 CrossRef CAS.
- P. Chanphai and H. A. Tajmirriahi, Trypsin and trypsin inhibitor bind PAMAM nanoparticles: Effect of hydrophobicity on protein–polymer conjugation, J. Colloid Interface Sci., 2016, 461, 419–424 CrossRef CAS PubMed.
- R. Månsson, G. Frenning and M. Malmsten, Factors affecting enzymatic degradation of microgel-bound peptides, Biomacromolecules, 2013, 14, 2317–2325 CrossRef.
- G. Ma, W. Lin, Z. Yuan, J. Wu, H. Qian, L. Xu and S. Chen, Development of ionic strength/pH/enzyme triple-responsive zwitterionic hydrogel of the mixed L-glutamic acid and L-lysine polypeptide for site-specific drug delivery, J. Mater. Chem. B, 2017, 5, 935–943 RSC.
- M. A. Azagarsamy, P. Sokkalingam and S. Thayumanavan, Enzyme-triggered disassembly of dendrimer-based amphiphilic nanocontainers, J. Am. Chem. Soc., 2009, 131, 14184–14185 CrossRef CAS.
- H. Wei, R. X. Zhuo and X. Z. Zhang, Design and development of polymeric micelles with cleavable links for intracellular drug delivery, Prog. Polym. Sci., 2013, 38, 503–535 CrossRef CAS.
- C. I. C. Crucho, Stimuli-responsive polymeric nanoparticles for nanomedicine, ChemMedChem, 2015, 10, 24–38 CrossRef CAS PubMed.
- A. J. Harnoy, G. Slor, E. Tirosh and R. J. Amir, The effect of photoisomerization on the enzymatic hydrolysis of polymeric micelles bearing photo-responsive azobenzene groups at their cores, Org. Biomol. Chem., 2016, 14, 5813–5819 RSC.
|
This journal is © The Royal Society of Chemistry 2023 |