DOI:
10.1039/D3RA03477A
(Review Article)
RSC Adv., 2023,
13, 21345-21364
Wound healing strategies based on nanoparticles incorporated in hydrogel wound patches†
Received
24th May 2023
, Accepted 7th July 2023
First published on 17th July 2023
Abstract
The intricate, tightly controlled mechanism of wound healing that is a vital physiological mechanism is essential to maintaining the skin's natural barrier function. Numerous studies have focused on wound healing as it is a massive burden on the healthcare system. Wound repair is a complicated process with various cell types and microenvironment conditions. In wound healing studies, novel therapeutic approaches have been proposed to deliver an effective treatment. Nanoparticle-based materials are preferred due to their antibacterial activity, biocompatibility, and increased mechanical strength in wound healing. They can be divided into six main groups: metal NPs, ceramic NPs, polymer NPs, self-assembled NPs, composite NPs, and nanoparticle-loaded hydrogels. Each group shows several advantages and disadvantages, and which material will be used depends on the type, depth, and area of the wound. Better wound care/healing techniques are now possible, thanks to the development of wound healing strategies based on these materials, which mimic the extracellular matrix (ECM) microenvironment of the wound. Bearing this in mind, here we reviewed current studies on which NPs have been used in wound healing and how this strategy has become a key biotechnological procedure to treat skin infections and wounds.
1. Introduction
Wound healing is a vital physiological mechanism for preserving tissue integrity, following the formation of an acute or chronic wound (ulcer) that leads to tissue damage.1 Long-term, and persistent wounds (chronic) produced due to the complications such as obesity, microbial infection, aging, or other factors may disrupt the tissue integrity and result in severe morbidity, and reduced quality of life.2 Effective wound healing strategies are challenging due to the requirement of complicated treatment strategies, along with increased medical expenses for clinicians and researchers.2,3 On the other hand, wound management has been identified by the World Health Organization (WHO) as a global public health concern.4 Wound repair is a complicated and staged process with known cell types (e.g., neutrophils, macrophages, fibroblasts, and keratinocytes) and microenvironment conditions (e.g., extracellular matrix (ECM) components, cytokines, and growth factors (GFs)).3,5,6 Routine wound healing consists of sequential phases, including hemostasis, inflammation, proliferation, and remodelling phase.1,2 Following a skin injury, platelet aggregation is activated, resulting in degranulation and the release of chemokines and GFs to form the clots. Subsequently, macrophages accumulate at the site of injury, promoting the phagocytosis of pathogenic microorganisms. The proliferative phase is distinguished by an increase in the number of cells (e.g., fibroblasts, keratinocytes, and endothelial cells) and abundant connective tissues (e.g., proteoglycans, hyaluronic acid, collagen, and elastin). The remodelling phase (final stage) includes both current cells' apoptosis and cell production.1
In wound healing studies, novel therapeutic approaches have been proposed with the aim to develop effective treatments that target different wound healing stages, or even the entire processes. Wounds demonstrate differences in terms of molecular and cellular mechanisms. Therefore, therapeutic strategies to treat these injuries are also diverse. Nano-therapeutic-based, stem cell-based, bioprinting-based, ECM-based, platelet-rich plasma-based, cold-atmospheric plasma-based, and microRNA-based therapies have come into prominence as recent options for wound healing.3 These strategies can be used to support the initiation/maintenance of the cells' functional expression, target collagen synthesis activation, angiogenesis activation, cytokine modulation, GF activation, antimicrobial effects, etc.7 Various types of nanomaterials, including metal NPs (e.g., silver, gold, copper, zinc oxide, titanium dioxide, etc.), ceramic NPs, synthetic polymeric NPs (e.g., PET, PLGA, PLLA, etc.), natural polymeric NPs (e.g., chitosan, gelatin, silk, fibrin, collagen, etc.),self-assembled NPs, and composite NPs have been used for acute or chronic wound healing. All these strategies are covered in this review article.
The improvement of these nanomaterials, which imitate the wound ECM microenvironment, has opened the path for better wound care/healing therapies. The most significant reasons for the preference of metal NPs are that they are less toxic and present intrinsic bacteriostatic and bactericidal activity.8 Ceramic nanofibers are generally used with the integration of synthetic or natural polymers in wound repair approaches because of their bioactivity and biodegradability characteristics.9 Polymers with low toxicity are often used in tissue engineering and drug delivery applications through further modifications.10 Additionally, the biocompatible self-assembled NPs have vast potential to closely mimic the natural ECM that can be functionally developed to increase their communication with other cells.8
Moreover, as the focus of this review, hydrogels-NPs composites possess many advantages, including antibacterial properties, and providing a moist environment.11 Recently, nanoparticle-loaded hydrogels for wound healing have attracted attention as a better option than standard dressing. Hydrogels also have natural healing qualities, including excellent water absorption, biocompatibility, and biodegradability properties. Furthermore, modern wound dressing systems prioritize natural polymer hydrogel dressings that can improve the wound microenvironment and promote healing at various stages.12
Considering all the above, this review is an update on current findings of wound healing from the perspective of nanoparticle strategies that are incorporated in hydrogel wound patches, aiming at better understanding the wound healing mechanisms for the development of more effective treatment strategies to maintain a regulated and coordinated inflammatory response at the injury site.
2. Wound healing and chronic wound physiology
Wounds are categorized into two groups: acute and chronic, which is determined by the healing time.13 Generally, if the healing time exceeds 12 weeks, the wound can be considered as chronic. However, in case the healing process reach 30 days, it can be categorized as a wound that does not show any signs of healing.14–16 The rate of healing depends on several internal and external factors. The lack of extracellular matrix (ECM) proteins, increased reactive oxygen species (ROS) production, worsening neovascularization, hypoxia, decreased collagen formation, and the attenuation of angiogenic growth factors can be considered as several reasons of reduced healing rate.17 Diabetic foot ulcers, pressure ulcers and vascular ulcers are examples of chronic wounds.14 The protective epithelium layer in the vicinity of the wound is injured, rendering it ineffective.18
The wound healing process consists of a linear set of stages. Delays in this flow cause an inflammation cycle, and the wound becomes chronic as a result.19,20 An increase in the number of M1 macrophages and neutrophils at the chronic wound site is generally caused by increased ROS, chemokines, cytokines, and other inflammation-related substances. The ROS and matrix metalloproteinase (MMPs) molecules released by these cells also contribute towards inflammation. As a result, scar tissues are subjected to unremitting cycle of inflammation (Fig. 1). On the other hand, wound healing in acute wounds, consists of four stages: hemostasis, inflammation, proliferation, and remodelling.14,21 Nevertheless, it is worth noting that hemostasis is not considered as a different stage in several articles.16,22 At the hemostasis stage, clot formation is observed to stop bleeding and prevent blood loss via platelets at the wound site, which is no longer protected with an epithelial layer against external pathogens. It is a complex process involving many factors, including the conversion of fibrinogen to fibrin and the eventual formation of a fibrin clot that enables cell migration to the affected area.23–25 From that wound vicinity, calcium, ROS, microbe-associated molecular patterns (MAMPs), pro-inflammatory cytokines (mostly interleukin-1β (IL-1β), IL-6, and tumor necrosis factor α (TNF-α), and damage-associated molecular patterns (DAMPs) such as ATP, DNA, or uric acid are released either directly or indirectly to stimulate migration to these regions.13–16,26,27 For instance, in response to these factors, innate macrophages release hydrogen peroxide (H2O2), which is a ROS that promotes inflammation (e.g., via nuclear factor κB (NF-κB))26 and, subsequently, causes migration.28 Consequently, pro-inflammatory responses are activated, leading to the second stage of wound healing (inflammation).24 To break down the damaged ECM, to offer defense against external pathogens, and to clear debris, several cell types such as neutrophils and monocytes are recruited to the affected area.27,29,30 These inflammatory cell types are localized in certain regions by tracking elevated pro-inflammatory factors.26,27,31 Neutrophils are a type of migrating cell that secrete and/or are activated by cytokines, including leukotriene B4 and chemokines like CXC motif chemokine ligand 8, IL-1β, IL-6, IL-10, IL-17, and TNF-α via various pathways (e.g., NF-κB, phosphoinositide-3-kinase, extracellular signal-regulated kinase, RAC).27,32–34 Additionally, they use various strategies, including phagocytosis, ROS or granules release, and the construction of neutrophil extracellular traps that have antimicrobial properties and are capable of secreting proteases.19,26,27,33–38 By contrast, MMPs digest damaged ECM.28 While innate macrophages are present at the site of the wound, monocytes, which represent another class of migratory cells, predominantly transform into pro-inflammatory (M1) macrophages.29,35 M1 macrophages secrete MMP-2, MMP-9, and cytokines, including IL-1, IL-6, and TNF-α.26,36 Pro-inflammatory cells migrating to the affected area through inflammatory mediators enhance the inflammatory response, leading to increased inflammation. For example, macrophages secrete MMPs and cleave the ECM, increasing the number of DAMPs molecules in the wound area, whereas neutrophils release ROS.36,39,40 When the wound area lacks unnatural substances, the pro-inflammatory cells must be cleared, so that the wound can recover properly and completely.34,39
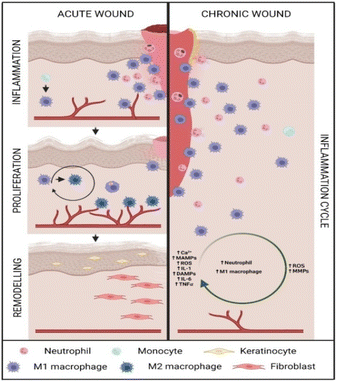 |
| Fig. 1 Phases of acute and chronic wound healing processes. | |
There is more than one way to clear neutrophils: either by re-entering the bloodstream or by macrophages phagocytosis after neutrophils have gone through the necrosis and/or apoptosis pathways.34,41 By contrast, M1 macrophages can transform into anti-inflammatory (M2) macrophages and participate in the following proliferation stage in accordance with the normal healing timeline.19,29,42,43 These systems are vulnerable to disruption.19 It is mentioned that macrophages' ability to phagocytize neutrophils may be reduced, or the transition between types of macrophages can be inhibited.29,44–46 For example, in the regular wound healing process, SETDB2, which silences pro-inflammatory cytokine genes by methylating the NF-κB binding sites of those gene promoters, increases their expression after four days. As demonstrated by Gallagher and colleagues, this pathway is induced by high amounts of interferon (IFN)-β and causes M1 to transform into M2.46 However, in diabetic patients, the impairment starting from that up-regulation of IFN-β causes an increase in pro-inflammatory cytokines, along with an increase in uric acid production.46,47 Sustained inflammation is caused by disruptions in pro-inflammatory mechanisms, including neutrophil clearance or the transition between M1 and M2 macrophages, as might be seen in chronic wounds.19,20,48,49 In chronic wounds, the oxygen pressure can be as low as 10 mm Hg, which is lower than in normally healing wounds. The hypoxia is caused by a defective angiogenesis mechanism.50,51
Proliferation phase is composed of four steps: re-epithelisation, angiogenesis, granulation of tissue formation, and collagen deposition.38 A provisional matrix is formed by fibrin and fibronectin, and then keratinocytes and epithelial cells multiply move across ECM to begin the re-epithelization process.52,53 Angiogenesis is required to supply enough oxygen and nutrients to the wound area.53,54 Macrophages aid angiogenesis by secreting TGF-β (transforming growth factor) and VEGF (vascular endothelial growth factor).55 Also, collagen I induces angiogenesis by attracting endothelial cells.56 Fibroblasts degrade provisional matrix and create granulation tissue, which helps the wound space closure by secreting collagen, fibronectin and hyaluronic acid.30,57
During the granulation of tissue formation, macrophages, fibroblasts, and blood vessels are recruited.58–60 Collagen fibers that are produced by fibroblasts to form granulation tissue are irregular. They are first disintegrated using enzymes produced by neutrophils, macrophages, and fibroblasts. These fibers then adopt a more structured appearance. The arranged fibers begin to deposit at the end of the proliferation phase, starting the remodeling phase.24,38
The remodeling stage is the final stage of wound healing, which can take years.61 The granulation tissue turns into scar tissue by transformation of fibroblasts into myofibroblasts. Myofibroblasts secrete α-SMA (α-smooth muscle actin), which leads to elevated mechanical strength.62 Also, type III collagen converts to type I collagen, which has a higher tensile strength; however, the scar tissue recovers about 50–80% of their tensile strength.61,63 In chronic wounds, ECM cannot be regenerated properly due to excessive MMPs, an unbalanced ratio of protease inhibitors that cause a persistent inflammatory environment.19 The inflammatory environment enhances ROS production that induces cell damage.39,64 Thus, many senescent cells are localized in chronic wounds.19 By contrast, microbial contamination that causes biofilm formation, which is known to be drug resistant, is present in the chronic wound environment. The most common microorganisms are Enterococcus faecium, Staphylococcus aureus, Klebsiella pneumoniae, Acinetobacter baumannii, Pseudomonas aeruginosa and Enterobacter sp., known as the “ESKAPE” group of pathogens.65–67 Even fungal biofilms exist in chronic wounds, although their mechanisms and biological roles have not yet been fully understood.68
3. Nanoparticles in wound healing
3.1. Metal nanoparticles
The exploitation of metals as antibacterial agents is traced back to early civilizations. During the past ten years, the use of metals and metal NPs to fight infection has increased. Numerous metal NPs are now easily accessible on the market and utilized more regularly in hospitals for dressings.69 Metal NPs like silver, gold, and zinc are the main options for wound dressing's development because of their antibacterial capabilities and low toxicity.70
3.1.1. Silver nanoparticles. In vitro antimicrobial activity studies on silver NPs (AgNPs) are comprehensive. AgNPs have remarkable antimicrobial properties and specific physicochemical characteristics (high surface area to mass ratio), which provide them with a significant advantage in the evolution of different options against multidrug-resistant microorganisms.71 Silver NPs may be combined with antibiotics at low quantities to boost effectiveness, while minimizing adverse effects. In a recent report, it was shown that using AgNPs and tetracycline together greatly reduced the load of microorganisms in a mouse model's surface and deeper tissue regions, thus accelerating the healing process.71 The antibacterial characteristic of silver is well recognized for being regulated via the blocking of respiratory enzyme pathways and modifying the microbial DNA and cell walls.72 Moreover, AgNPs increase wound contractibility by stimulating the segregation of myofibroblasts from normal fibroblasts and speeding up the recovery process.73The antibacterial effect of Ag+ ions also comes from their interaction with sulphur and phosphorus groups within the structure of bacterial cell walls and plasma membrane proteins, which causes these proteins to malfunction.74 By contrast, silver ions directly bond to the cell membranes, and the deposition of silver upon negatively charged regions of the cell surface causes membrane rupture, intracellular content leakage, and cell death.75 The activity of the bacteria's electron transport chain may then be hampered by the existence of silver ions inside the cell. Moreover, silver ions bond to the bacterial DNA and RNA and block cell division.76
Nanobiocomposite (NBC's) hydrogels were developed by R. Singla et al.77 using bamboo cellulose nanocrystals combined with AgNPs. As a result, this hydrogel enhanced the recovery process in just 18 days.77 Also, several clinical experiments involving wound healing, particularly burns and chronic wounds such as diabetic ulcers, used silver NPs.78
3.1.2. Gold nanoparticles. Gold NPs (AuNPs) are suitable materials for treating wounds because of their simple synthesis, chemical stability, and unique optical properties.79 The antibacterial and antioxidant activities of AuNPs can drastically accelerate wound healing and repair damaged collagen tissues.80 This anti-inflammatory and anti-angiogenic effect of AuNPs stimulates the release of proteins that are essential for wound healing.81 AuNPs are useful in biomedical activities, as different biomolecules should be integrated into them. Among the benefits to biomedical application, we can cite that fact that different biomolecules can be integrated into these structures. The inclusion of the gold surface does not cause a change in the structure of the collagen–collagen crosslink, which means that cooperating with polysaccharides, growth factors, peptides and molecules will provide benefits in terms of adhesion to cells. These modified AuNPs exhibit characteristics including biocompatibility and biodegradability, making them useful in various applications involving wound healing. Comparable to collagen, chitosan, and gelatin, these substances may also be added to AuNPs, showing good results in accelerating wound healing.82,83Regarding their bacteriostatic and bactericidal effects, AuNPs can either target interfere with the bacterial cell wall integrity, or they can bind to the double-helix of bacterial DNA and stop it from unfolding throughout transcription or replication. Therefore, they can inhibit bacteria that are multidrug resistant, including Escherichia coli, S. aureus and P. aeruginosa. Besides, AuNPs also act as antioxidants by preventing the development of ROS and accelerating curing.84 Also, according to some studies, AuNPs had a significant impact on the healing of wounds by successfully scavenging free extremist like DPPH (2,2-diphenyl-picrylhydrazyl), OH (hydroxyl), H2O2 (hydrogen peroxide), and NO (nitric oxide).85–87
In the context of bacterial infections, vancomycin-combined gold NPs (Au-Vanc-NP) massively improved vancomycin's effectiveness over E. coli.88 For photothermal treatment89 or photodynamic therapy (PDT),90 AuNPs can be conjugated with pathogen-specific antibodies or photosensitizing compounds.
3.1.3. Copper nanoparticles. Copper is a necessary component in many wound-healing processes.91 Copper enhances healing through raising the levels of ECM particles such as fibrinogen, collagen, and integrins, which are the principal mediators of cell attachment to the ECM.92 The copper-induced activity of vascular endothelial growth factor (VEGF) is recognized to support angiogenesis in wounds.93 Copper NPs (Cu NPs) can also promote angiogenesis via influencing the expression of hypoxia-inducible factor (HIF-1a) and the control of VEGF.94CuNPs have been produced using a variety of methods, including sonochemical methods, metal vapour deposition, electrochemical reduction, radiolytic reduction, thermal decomposition, mechanical attrition, laser ablation, microemulsion techniques, and chemical reduction.95 The chemical reduction method is one of the most universal for fabricating metal NPs due to its low cost and convenience.95
Generally, copper-containing nanomaterials show excellent activity in wound dressings and can be potentially used in biomedical applications.96 Alizadeh and his colleague observed that 21 days exposure of CuNPs (80 nm/1 mM), could efficiently enhance the wound healing process within the treated rats than the rats in control set.94 Cu has the ability to speed up the skin healing process in mice by increasing VEGF expression at the wound margin.97 Copper-containing biologically active egg shell membrane nanocomposites were found to increase in vivo angiogenesis rate and generate neo-epidermis, which are beneficial to wound healing.98
3.2. Ceramic nanoparticles
Several advanced nanocomposite hydrogel systems can be obtained by integrating natural or synthetic polymers with ceramic NPs that have a high variety of options such as silica, hydroxyapatite and metal oxides.99,100 These nanocomposites will be discussed in detail in the following topics.
3.2.1. Silicon derived ceramic nanoparticles. Silicon and its derivatives are preferred in bone tissue applications due to their ability to promote osteogenic differentiation in human stem cells and collagen type I synthesis.101 Silicon dioxide is also known to support bone regeneration. For instance, silicon dioxide derived bioglass materials have shown to promote regeneration by forming apatite bonds on surface layer.102Silica is a type of mineral that is obtained from silicon dioxide, which is known to be non-toxic and highly biodegradable.103 When integrated with hydrogel systems, they have shown to promote wound healing with different aspects. For instance, silica-collagen type-I nanocomposite hydrogels have been formed by encapsulating two types of antibiotics into silica NPs (SiNP). These nanocomposites have been immobilized at high silica dosage in concentrated collagen hydrogels without changing their fibrillar structure or affecting their rheological behavior. Additionally, it was observed that their proteolytic stability was increased.104 By contrast, Öri and colleagues designed SiNP-PVP nanocomposites that fastened re-epithelization and promoted the wound healing property of silica-based nanomaterials.105
Another research area related to nanocomposites is adjoining cut wounds and different tissue adhesives. A simple aqueous solution of SiNPs was developed by Rose et al.,106 which was efficiently used as a tissue adhesive for exvivo bonding of natural bovine tissue. The same group has further refined it as a nanobridging technology for fast and powerful closure and healing of deep wounds in the skin and liver of mice.106 In addition, another study found that SiNPs helped skin fibroblast cells proliferate and spread to the injured area, released silicic acid, and improved topical wound repair in vitro.104 The very rapid release of loaded drugs in some polymeric structures (e.g., collagen) has a serious limiting role in areas such as wound dressing. Due to this limitation, silica NP integrated systems have been developed to enhance the controlled drug release.
Mesoporous silica NPs (MSNPs) are acknowledged as ideal candidates to drug carriers and promote wound healing due to their high surface area-to-volume ratios. It has been shown that basic fibroblast growth factor (bFGF) encapsulation into MSNPs is possible, revealing high loading capacity and a highly controlled release capacity. Reportedly, bFGF-loaded MSNPs were capable of increasing bone regeneration and revealed a promising system for wound healing.107
Silicates are mainly salts containing anions of silicon and oxygen. Wang et al.108 reported that the acceleration of diabetic wound healing has been demonstrated through the release of the silicon ions from silicate-based bioceramics during their degradation. The basic form of silicate-based biomaterials mainly includes silicate bioactive glass and bioceramic powders. Typical silicate biomaterials for wound healing are silicate-based bioglass or bioceramic powders combined with natural or synthetic polymers to produce hydrogels.108,109 They can also be loaded with clotting factors and due to their charged interactions; they can induce blood clotting and, therefore, can be used as homeostatic agents.110
Studies have reported an alendronate (ALN)-Ca2+/Mg2+-doped supramolecular (CMS) hydrogel that improved the hydrogel's mechanical properties and osteogenic activity. Moreover, encapsulated bioglass particles in oxidized sodium alginate (OSA) cross-linked with adipic acid dihydrazide (ADH) modified γ-polyglutamic acid, and bioglass NPs creating an alkaline micro-environment providing the hydrogel stickiness to different medical implants.111,112 Recent studies have shown that silicate-based biomaterials actively promote wound healing by regulating the function of various skin-related cells. It has been proven that such bioactivities are attributed to bioactive ions released from wound dressings.113,114
Chitosan-based injectable systems containing bioactive glass NPs are silicate derivatives that induce the formation of bone tissue that integrates well with the hydrogel structure that have played an important role in stimulating cell proliferation, angiogenesis and wound healing.115 Some other studies have shown that bioactive glass NPs can bind to soft tissue through the apatite layers formed on their surface when they come into contact with cells.116 Mao et al.117 investigated the effect of bioglass on cultures of human umbilical vein endothelial effects (HUVECs). As a result, the bioglasses stimulated HUVEC proliferation, accelerated cell migration, and up-regulated maintenance of endothelial NO synthase.118
Nanoclays are composed of NPs of layered mineral silicates with exceptional mechanical properties containing the minerals found in bone tissue. These nanomaterials can successfully interact and disperse within networks of polymeric hydrogels, due to their interaction capacity.108 They have been researched on traumatic wounds that require fast homeostasis and it was shown that injectable nanosilicate-gelatin hydrogels decrease in vitro blood clotting 77% and it formed stable clot-gel systems under in vivo conditions.119 In addition, the integration of nanosilicate to collagen stabilizes the nanocomposite network due to strong physical interactions.
Nanocomposite hydrogels have also been shown to promote tubule formation of cultured endothelial cells.118 Synthetic silicate nanoplatelets such as LAPONITE® (Lap), which is a commercial name for synthetic nanoclay, are highly charged NPs that have been shown to induce blood coagulation. It has been proven that Lap can support bone mineralization without the help of external growth factors (GFs). Due to the presence of both positive and negative charges on the nanosilicate surface, they interact strongly with proteins and the presence of these strong ionic interactions mean that chemical conjugation is not required. It has been shown that the incorporation of vascular endothelial growth factor (VEGF) into Lap-collagen gel enhances angiogenesis in vivo. The ability of the nanocomposite gel to increase angiogenesis can be used to promote wound healing.118 Therefore; Lap-coupled hydrogels have emerged as promising options for applications such as tissue engineering and wound healing without the need for GFs. Moreover, it has been reported that the integration of Lap and polyethylene glycol (PEG) stimulated cytoskeletal arrangement and cell binding.120 It was shown that Lap is a potential antibacterial agent and assists in homeostatic processes. Moreover, it can also be used to encapsulate drug-like molecules.121 Studies have also shown that mafenide (Maf) can be added between layers of nanosized Lap to be used as an antibiotic elution gel for healing burn wounds. The film showed a water absorption capacity higher than alginate alone.119
45S5 is one of the most representative bioglasses. It has been used for bone tissue regeneration osteogenesis stimulation, and angiogenesis promotion at a high rate.122 This system is useful for acute wounds,123 chronic wounds,124 infected wounds,125 and burns.126 Mesoporous bioglasses (MBGs) have also been used extensively to repair bone tissues, as they form a direct and firm bond with living tissues. To repair large bone defects, porous structures with proper vascularization that can allow migration and proliferation of osteoblasts are critical. Studies have shown that (PEGSH)4 homopolymer MBGs form an injectable and self-healing dynamic hydrogel for bone regeneration. It has also been observed that the dimensions, surface areas, surface chemistry and other properties of MBGs will significantly affect the biocompatibility and mechanical properties of the prepared bioink.127
3.2.2. Calcium and phosphate salts. Calcium phosphate NPs can be easily combined with different polymers to form a hydrogel mesh. With this hydrogel complex, bone regeneration can be achieved with promoted angiogenesis and osteogenesis. Also, they have shown to enhance drug release and antibacterial effect. It has been shown that bicalcium phosphate (BCP) NPs combined with chitosan/gelatin hydrogels form bone scaffolds for bone tissue engineering.128 In that study, physicochemical and biological properties were investigated by preparing chitosan/gelatin/BCP NPs in different weight ratios.128 It was observed that the porosity, swelling rate and mechanical strength of 3D printed bone scaffolds were mainly affected by the dosage of BCP NPs. In addition, in the same study, it was shown that BCP NPs can improve the hMSC proliferation of scaffolds and, therefore, have a great potential in bone tissue engineering both in vitro and in vivo.128Tricalcium phosphate (TCP) can be cited as another example. There are two main types of TCP: α-TCP and β-TCP. Because β-TCP is more stable than α-TCP, it is more commonly used as a bone scaffold. β-TCP NPs can promote the mechanical functioning of bone structures and proliferation of osteoblasts. These qualities predominate β-TCP in bone scaffolds.129 In active and rapid chemical regeneration, the support is obvious as they are the main minerals in the bone structure and, therefore, β-TCP NPs have been integrated into the PEGylated specific membrane.130 In that study, the membrane exhibited a controllable degradation rate and enhanced mechanical properties based on different β-TCP dosages. It is believed to be capable of promoting the adhesion and proliferation of rat bone marrow-derived mesenchymal stem cells (rBMSCs).130
Hydroxyapatite is a natural form of calcium apatite that is found in the nature. It is known to support homeostasis, prevent inflammation and promote proliferation and re-epithelization.131 It also provides high mechanical strength.111 Due to their high physiological stability, HA structures are preferred as injectable fillers in orthopaedic applications. Moreover, considering its highly elastomeric structure, it provides control on tissue viscoelasticity.132
3.2.3. Metal oxides. Metal oxides are one of the most important and widely characterized classes of ceramics. Some of the most used types of metal oxides for wound healing applications are zinc oxide (ZnO), titanium dioxide (TiO2) and zirconium dioxide (ZrO2).Due to their high mineral content and combinability, magnesium oxides (MgO) and aluminium oxides (Al2O3) are promising candidates for tissue healing applications.108 Furthermore, metal oxides such as copper oxide (CuO) and NO are known to act as antibacterial agents and promote wound healing.133
3.2.3.1 Zinc oxide nanoparticles. Zinc oxide NPs (ZnO NPs) are used in numerous hydrogel-based wound treatments due to their exceptional antibacterial characteristics.130 ZnO is known to regulate homeostasis131 and high antibacterial potential.119,134 The antibacterial efficacy of ZnO NPs with diameters of 20 nm, 70 nm, and 100 nm was quantified against Gram-negative (E. coli) and Gram-positive (S. aureus and B. subtilis) bacterial strains.135 ZnO NPs are far more effective against Gram-positive bacteria, most probably due to its interaction mechanisms with bacterial cell walls that lack lipopolysaccharides (LPS).135 The utilization of ZnO NPs is beneficial because of its significant activity against harmful bacteria even at low concentrations. Moreover, they are long-lasting, with high selectivity and temperature resistance.136 It is also antineoplastic, promoting keratinocyte mobility, angiogenesis and wound healing.134,137 Due to these characteristics, it is mainly used for the control of inflammation during wound healing.138 Studies have shown that ZnONPs exhibit therapeutic activities against bacterial infection and inflammation, and showed potential for wound healing applications.139Zinc is one of the most important trace elements that regulate burns and slow-healing wounds through regulation of DNA and RNA polymerases, ribonuclease and thymidine kinase. It has been reported that it has low toxicity but preserving its permeability in engineered chitosan–ZnONP hydrogels. These dressings have been reported to have excellent biocompatibility, inhibit bacterial growth, and improve wound closure in vitro and in vivo.140,141
3.2.3.2 Titanium dioxide nanoparticles. Titanium dioxide NPs (TiO2NPs) are appropriate for the recovery of wounds materials due to their bactericidal and anti-inflammatory activities, higher bump, hydrophilicity, and biocompatibility. Bacterial cellulose (BC) and TiO2 were conjugated by Khalid et al.142 to create nanoconjugates that may be used as bandages for wounds.142 In that work, the authors noted that BC was highly water-retentive, porous, and biocompatible, making it an ideal material for wound care applications. The same research group also demonstrated that incorporating TiO2NPs into BC improved its potency as a material for wound treatment. The biocompatibility and antibacterial activity of these newly created nanocomposites were increased. Similarly, a synthetic skin-equivalent nanocomposite from chitosan and TiO2 was produced. Such synthetic skin substitutes show low density, good thickness, moderate biodegradability, and adequate water absorption capabilities. Also, it was seen that the titanium increased the strength and showed excellent antimicrobial effects on pathogenic bacteria whilst increasing wound healing in albino rat models.143Additionally, Sivaranjani et al.144 produced TiO2NPs from Moringa oleifera leaves under optimum conditions. Upon analysis, it was discovered that the NPs were 100 nm in size. TiO2NPs have improved wound healing and acted as an antibacterial agent against Gram-positive and Gram-negative bacteria because of their superior mechanical properties.144 Future research will benefit from these findings, and wound care products may use TiO2NPs.
ZrO2 and TiO2 are also known to promote bone regeneration.145 This combination has displayed improved antibacterial properties and increased mechanical strength through ROS production. In this context, it has been stated that it accelerates the healing of open excision wounds.146 It has been shown that TiO2NPs promote wound healing through the production and secretion of critical molecules for the process.147 Moreover, wound dressings made out of TiO2NPs showed an improvement in structural, textural, thermal, optical, mechanical, vapour barrier properties and their biodegradability in a dose-dependent manner.148
3.2.3.3 Magnesium oxide nanoparticles. Investigations on the role of magnesium oxide nanoparticles (MgO NPs) on wound healing were intensified due to their biocompatibility, low toxicity, thermal stability and antibacterial properties. In a study, carboxymethyl cellulose hydrogels containing magnesium hydroxide nanoparticles (Mg(OH)2 NPs) were produced as a scaffold owing to increased wound healing as well as antibacterial activity.149 In a separate study, Qu et al.150 designed a hybrid nanocomposite hydrogel composed of MgO NPs and chitosan. They observed excellent mechanical properties, high biocompatibility, and improved antibacterial activity. Their results showed that the integration of MgO NPs significantly enhances in vivo wound healing process in a skin defect rat model.
3.2.3.4 Aluminium oxide nanoparticles. Aluminium oxide nanoparticles (Al2O3 NPs) are one of the metal oxides that have antibacterial activity.151 Tanveer and colleagues152 designed a biodegradable superabsorbent hydrogel derived from acrylic acid and Al NPs exhibited antibacterial activity. Rahmanpour et al. reported that the potential of TiO2/Al2O3/Chitosan nanocomposites as effective and multi-functional dressings for the treatment of infected wounds, exhibiting antibacterial activity, reduced bacterial count, decreased expression of inflammatory genes, and increased expression of proliferative genes, fibroblast, and collagen, thus highlighting their promising healing capabilities and potential as a competitive alternative to commercial ointments.153 There are studies in which tubular aluminium oxide particles, grown in a contiguous manner using an anodizing system, are employed as molds for the production of nanopatterned polymer surfaces, presenting a novel perspective. For example, Altuntas et al. worked on multiple studies regarding ossteointegration and wound healing have demonstrated that chitosan:gelatin nanorods, which take the form of the Al2O3nanotubes, play a beneficial role in these processes.154,155
3.2.3.5 Copper oxide nanoparticles. Copper ions promote wound healing due to their promotion of angiogenesis. During the applications, one possible outcome for the patient is the potentially toxic levels due to the exposure to copper ions. Due to this risk and to develop a potential system that may eliminate this toxicity, researchers have designed a novel antioxidant copper metal–organic framework-thermo responsive polydiolcitrate hydrogel composite that accelerates chronic wound healing with reduced toxicity and apoptosis in a splinted excisional wound healing diabetic mouse model.156 Another study, Li et al.157 designed a hydrogel system including CuO NPs that increased antibacterial effects and skin tissue regeneration due to the ions release from the hydrogel stimulating proliferation and angiogenesis.
3.2.3.6 Cerium oxide nanoparticles. Non-healing wounds in patients with diabetic complications are a major issue that frequently leads to amputations and death. Cerium nanoparticles, in this connection, have exhibited potential results in several experimental studies.158–161 Diabetic wounds are distinguished by impaired wound healing as a result of elevated oxidative stress, anomalies in its defence mechanism and prolonged inflammation.Notably, biodegradable and highly porous gelatin methacryloyl hydrogel patches combined with cerium oxide NPs have showed promising exudate uptake capability, ROS scavenging activity, in vitro cellular proliferation (i.e. keratinocyte, fibroblast etc.), and in vivo mechanisms of diabetic wound healing.159 Cerium NPs-miR146a composite raises wound collagen, improves angiogenesis, and reduces inflammation as well as oxidative stress, eventually encouraging faster wound closure in diabetic wounds.160 Furthermore, chitosan/PVA hydrogels combined with Cerium NPs could be a competing candidate as a strong wound dressing material that effectively reduces infection without the usage of antibiotics.161
3.3. Polymer nanoparticles
Polymer NPs have gained significant attention over the last few years because of its size-related properties.162 Polymer NPs are in the family of nanocarriers (nanoparticle-based drug carriers). Nanocarriers also include lipid-based nanocarrier (liposome or micelle), gold (Au) NPs (nanoshell or nanocage), magnetic nanoparticle, carbon nanoparticle (nanotube), quantum dot, dendrimer etc.163,164 Nanocarriers are used for drug delivery applications. Herein, the polymer NPs provide safeguarding and managing controlled release of drugs, as well as increase the bioavailability and the therapeutic index.165 They are advantageous over other nanocarriers because of easy fabrication, cost-effectiveness, biocompatibility, biodegradability, non-immunogenicity and water solubility. In comparison to cationic lipids, cationic polymer generates a more stable complex which aids the cellular migration.163
Polymeric NPs can be used in the form of either nanocapsules or nanospheres, and also in the form of carrier system with diameter less than 1 μm.166 Nanocapsules are based on a lipid core enclosed within a polymeric shell. The drugs are typically dissolved within the lipid core and the shell regulates controlled drug release from the core.167 The lipid core composed of vegetable oil or fatty acids acts as lipophilic drug-dissolving medium, facilitates the encapsulation of lipophilic chemical compounds and act as an alternative therapeutic. It is also possible to create polymeric nanocapsules with an aqueous core that will serve as a platform for the controlled delivery of hydrophilic compounds.167 Nanospheres are comprised of matricial network of polymeric chains where the drug may adhere to the surface or be preserved within.165,168 Polymer NPs have a size distribution ranging from 10 nm to 100 nm, a positive or negative surface charge, a significant drug carrying capacity and diverse component matrices.169 Its component matrices can be either synthetic (e.g., poly-caprolactone) or natural (e.g., gelatin or chitosan). They can be synthesized from nonbiodegradable materials (e.g., cyanoacrylate or poly(lactic-co-glycolic acid)) or biodegradable materials (e.g., polyurethane).169 These nanostructures demonstrate significant drug loading efficiency for a wide range of drugs. One of the most widely used polymers for generating NPs is poly(lactic-co-glycolic acid), as it presents beneficial properties, including biocompatibility, biodegradability, and non-toxicity. Moreover, surface PEGylation is frequently employed to extend their in vivo half-life. As a result of surface-tuning of these NPs with PEG chains, the immunogenicity is significantly decreased as well as the stability and longevity is increased. Also, the aqueous solubility is improved, and opsonization is minimized.169
Several biological applications, including anti-inflammatory therapy, anti-cancer therapy, and immunotherapy, use nanocapsules or nanospheres as delivery vehicles for preserving and encapsulating metabolites, peptides, enzymes, hormones, genes, and other pharmaceuticals.164–169 Polymeric NPs also involve organ targeted drug delivery and genetic drug delivery to alter the central dogma of the cell along with bioimaging, biosensing and theragnostic applications in clinical settings.170–172
3.4. Self-assembled nanoparticles
The mechanism of self-assembly is the organization of the nano-sized molecules such as polymers, peptides, nucleic acids, and other NPs.173 Various processes lead to the synthesis of self-assembling in ordered structures for gaining fully function of the molecules in living cells, causing several biological changes. The main processes comprise the enzyme instructed self-assembly such as alkaline phosphatase induction, polymerization-induced self-assembly that comprises another intracellular alteration, host–guest interaction as non-covalent interactions, and pH-changes.174 The self-assembly processes can also be stimulated artificially to control biological mechanisms in living cells.175 Consequently, nanotechnological advances around self-assembled systems present features of structural adjustability, biocompatibility and functionality that are of great significance.176,177 They focus on improving treatment strategies,178 vaccination,179,180 wound healing,177,181 DNA origami-nanorobot for drug nanodelivery system,182,183 disease detection applications,172 neural tissue repair,184 and other nanoscale therapeutic delivery systems.185 Self-assembled NPs represent the most potential cost-efficient and high-throughput practical technique to nano-production.175
Self-assembled NPs especially used for wound healing strategies can be classified according to its material types, including metal, peptide amphiphiles, peptide-based self-assembled NPs, and elastin-like polypeptides. The fabrication methods can be divided into three major mechanisms: spontaneous (non-covalent) self-assembly, enzyme-catalysed self-assembly and chemical/physical cross-linked self-assembly.177
Metal-based self-assembled NPs are the prominent biomaterials in wound healing studies, primarily attributable to their antibacterial effects. In 2015, Chen et al. developed 2.5 nm hybrid nanodots via immobilization of self-assembled antimicrobial lipopeptides (surfactin, SFT) with 1-dodecanethiol (DT) anchored gold NPs (Au). The hybrid nanodots demonstrate excellent antibacterial properties for multi- and non-multi-drug resistant bacteria. Moreover, it is worth highlighting the impact of SFT and DT/Au-nanodots synergistically. The underlying mechanism of this is through bacterial membranes damage. In vivo experiments with SFT/Dt-Au nanodots revealed that bacterially infected wounds healed quicker and constituted improved epithelization, and it was productive in terms of collagen fiber formation.186
In another study, an antibacterial hybrid silver nanoparticle was developed as an alternative therapeutic strategy for wound healing. In vitro and in vivo studies demonstrated that this hybrid material which is formed by the incorporation of magnetic carboxymethyl cellulose-ε-polylysine hybrids (FCE) and silver nanoparticles (Ag NPs), has superantibacterial and wound healing promoting effects.187 Moreover, in a study by Perez-Rafael and co-workers, silver-incorporated NPs were developed for chronic wound healing. The developed nanomaterial is an injectable self-assembly of hyaluronic acid (HA) modified with 2-iminothiolane, mixed with bioactive silver-lignin NPs in physiological conditions, as shown in Fig. 2A. Because of the silver-lignin NPs in its construction, this combined multifunctional hydrogel demonstrated high antioxidant and antimicrobial characteristics. Moreover, these biocompatible hydrogels block myeloperoxidase and matrix metalloproteinases. Furthermore, in in vivo experiments with a diabetic mouse model skin integrity restoration and remodelling were observed.188
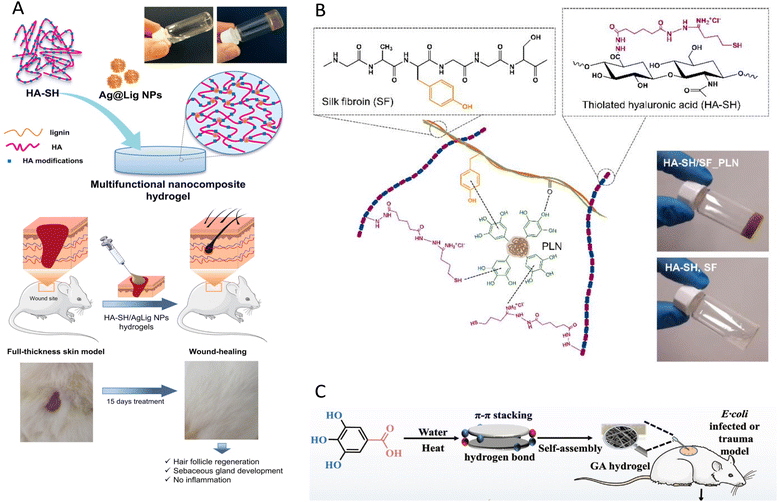 |
| Fig. 2 Wound healing strategies developed by self-assembling NPs. (A) Schematic diagram showing multifunctional hydrogel production and images of the wound healing process on mouse skin exposed to the modified HA-silver-lignin NPs has been reproduced from Perez-Rafael et al.,188 with permission from Elsevier, copyright 2023. (B) Representative illustration of the interactions between thiolated-HA, silk fibroin, and phenolated-lignin. The illustration shows that the mixture has turned into a gel, successfully has been reproduced from Morena et al.,189 available under CC-BY 4.0 license. (C) Schematic representation of GA hydrogel self-assembled via hydrogen bond and p–p stacking that enhances the wound healing has been adapted from Huang et al.,192 with permission from Wiley-VCH, copyright 2023. | |
By contrast, a chimeric non-metallic nanocomposite composed of phenolated-lignin, thiolated-HA, and silk fibril (Fig. 2B) showed around 52% of disruption of myeloperoxidase and matrix metalloproteinases enzymatic event, which is associated with the wound chronicity. Indeed, this self-assembled hydrogel showed high S. aureus and P. aeruginosa load reduction. Additionally, cell viability assays conducted with BJ5ta fibroblasts and HaCaT keratinocytes cell lines revealed approximately 93% of cell viability. Therefore, this mechanically and functionally tunable dressing material has a high potential for wound treatment.189
The tripeptide glycyl-L-histidyl-L-lysine (GHK)-Cu NPs has been shown to promote wound healing and repair DNA damage. At the same time, its instability in physiological condition limits its applications. The physical structure of GHK-Cu NPs has gained fluorescence characteristics. The research on fluorescent GHK-Cu peptides has a potential to create novel fluorescent peptides class to advance healing strategies.190 Additionally, growth factors have role in chronic wound healing process, and have also been combined with self-assembly peptides.191 The RADA 16-I nano hydrogel, which includes PDGF-BB incorporated ionic self-complementary peptide (RADARADARADARADA), is one of the examples. This self-assembled hydrogel showed capability for angiogenesis and chronic wound healing in cell culture and animal studies, offering a high potential strategy for wound treatment.191 Naturally small molecules such as gallic acid, a natural polyphenol having anti-inflammatory and antibacterial properties was used as a self-assembled NPs to accelerate wound healing processes (Fig. 2C).192 One example of antibacterial self-assembled wound dressing studies is a synthetic polymeric hydrogel (oxadiazole-group-decorated quaternary ammonium salts (QAS)-conjugated poly(ε-caprolactone)-poly(ethylene glycol)-poly(ε-capro-lactone; PCEC-QAS) created by Liu et al., especially for healing wounds infected with Gram-negative and Gram-positive bacteria. Methicillin-resistant S. aureus-infected skin wound on mouse was recovered successfully 12 days after hydrogel sealing of wound with PCEC-QAS.193
3.5. Composite nanoparticles
Researchers have shown that a healthy wound healing can be achieved with a proper and multifunctional wound dressing. Although there are some certain criteria for a hydrogel wound patch, for different stages of wound healing process, different properties are required for a healthy healing.194 To achieve a multifunction or a time-dependent functionality, the advantages of different materials can be used. Also, for more serious skin injuries like skin ulcers and skin burns, more effective wound dressings are needed. The advanced functions can be achieved by composite NPs incorporated in hydrogels as the hydrogels are typically used as a physical isolation and provide a moist environment.11 These functional modifications are performed to gain the patches certain properties that can be listed but not limited to antibacterial property, adhesion, mechanical strength, hemostasis, antioxidant/anti-inflammatory, controlled delivery, self-healing, stimulus response, and conductivity.
In the previous sections, the effect of incorporating different types of NPs in hydrogels as wound dressings were discussed and the effect of each type of nanoparticle incorporation to a hydrogel wound patch system were presented. Here, we will also discuss the use of composite NPs in hydrogels that are developed for wound patches. The use of composite NPs can enhance the effect and/or has multifunction for serious skin damages or fastened healing without any damage or abnormality on the skin tissue.
Avoiding the bacterial inflammation, causing an improper deposition of collagen and delaying the wound repair is one of the most crucial aspects for developing a wound patch.195 Especially for antibiotic resistant bacteria, different antibacterial strategies must be followed. The use of inorganic metals such as silver, gold and zinc in composite NPs has been widely studied for its contribution to create antibacterial hydrogels. Among the inorganic metals, Ag NPs are the most widely used.196 Aggregation is a challenge for such particles, thus hydrogels with composite NPs containing Ag are favourable.197,198 Another strategy is to use ceramic NPs such as ZnO,199 and graphene oxide200 for antibacterial patches. Multifunctional antibacterial hydrogel wound patches can be fabricated by using composite NPs composed of oxides or inorganic metal NPs.201 In a study by Fan and his coworkers,202 Ag and graphene nanocomposite in polyacrylic acid and N,N′-methylene bisacrylamide hydrogels were fabricated to obtain antibacterial and high mechanical strength patches with accelerated wound healing.202 They reported a high swelling ratio despite its enhanced tensile strength and elongation at break of hydrogel due to the use of graphene and antibacterial effect against both Gram-positive and Gram-negative bacteria and a full-thickness skin wound healing was achieved. In another study, zinc-doped bioactive glass NPs incorporated in succinyl chitosan/oxidized alginate hydrogel was developed as an antibacterial wound dressing, which promotes the enrichment of fibroblasts, production of vascular endothelial growth factor and fibroblast growth factor 2.203 Also, the incorporation of zinc-doped bioactive glass NPs has significantly enhanced the release of angiogenic factors from the cells at the wound area of rats, which fulfils the multifunction purpose of the composite nanoparticle embedded hydrogel.
Another strategy for achieving antibacterial wound dressings is to use light-assisted antibacterial therapy that is locally raising the temperature to achieve the mortality of bacteria, which is also effective for drug resisted bacteria and produce less side effects.204,205 This technique can also be used to fabricate hydrogels containing composite NPs that can show photothermal effect. It has been shown that polyvinyl alcohol hydrogel was incorporated with reduced graphene oxide/MoS2/Ag3PO4 nanocomposite particles to obtain efficient sterilization of the wound area under light irradiation.206 The antibacterial effect of Ag+ was enhanced with the ability to raise the local temperature with NIR illumination with the use of graphene oxide-based nanomaterials and MoS2. Reduced graphene oxide further enhanced the mechanical property and swelling ratio of the hydrogel. The efficiency of in vitro and in vivo antibacterial activity of the reduced graphene oxide/MoS2/Ag3PO4 PVA hydrogel was reported with no abnormalities in major organs in rats during the wound healing process.
Apart from achieving multifunctioning wound dressings, broad spectrum of antibacterial performance can also be achieved by incorporating composite NPs into hydrogel wound dressings. It has been reported that multiple targeting approaches were employed to achieve efficient antibacterial activity with a reduced risk of resistance.207 ZnO impregnated mesoporous silica NPs were used in carboxymethyl cellulose hydrogel and dual antibacterial effect of a broad-spectrum antibiotic tetracycline, which was loaded in mesoporous silica NPs and Zn2+, was achieved. The results proved that this composite nanoparticle structure effectively enhanced the antibacterial property with a wider inhibition zone against E. coli. This study also shows that composite NPs can also be used to efficiently develop a hydrogel wound patch with a drug release system.
Another strategy to overcome the increase of the drug-resistant microorganisms due to the over usage of antibiotics is to mimic the property of natural enzymes to generate ROS for disinfection by constructing artificial nanozymes.208,209 This artificial nanozymes can catalyze a low concentration of H2O2 into ·OH, which destroys the bacterial membrane via oxidation.210,211 Iron oxide,212 V2O5,213 and graphene quantum dots214 can be used as artificial enzyme catalysts to have antibacterial performance. However, the low efficiency of the nanozymes is still a challenge for effective antibacterial application. The use of composite NPs can enhance the efficiency of the nanozymes, as well as the multifunctional nanocomposites can be more beneficial. For example, in a study, Au nanoparticle with its superb glucose oxidase-like activity was combined with Pt NPs that can convert H2O2 into O2 was used to obtain a synergistic effect in an oxidized hyaluronic acid/carboxymethyl chitosan hydrogel.215 The results showed that the developed wound dressing was antibacterial, reduced blood glucose, alleviated the oxidative stress and the supply of oxygen due to the glucose oxidase-like and catalase-like activity of Au/Pt alloy nanoparticle, also accelerating the wound healing in vivo. In another study tannic acid-chelated Fe-decorated MoS2 nanosheets were incorporated in polyvinylalcohol, dextran and borax hydrogel and due to the peroxidase-like activity of MoS2 and catalase-like activity tannic acid-chelated Fe, high and affective disinfection, and supply of O2 was achieved.216 Also, photothermal therapy was also integrated to the healing via tannic acid-chelated Fe. As a result, antibacterial, anti-inflammatory, adhesive, self-healing multifunctional hydrogel was developed for wound healing applications.
Recently, injectable and in situ forming hydrogels have emerged as a promising candidate for wound patches due to its advantage for fabricating different-shaped patches depending on the wound area.217 It has been shown that an irreversible gelation of poly(N-isopropylacrylamide-co-n-butyl acrylate)-poly(ethylene glycol)-poly(N-isopropylacrylamide-co-n-butyl acrylate) copolymer in situ forming hydrogel containing silver NPs-decorated reduced graphene oxide nanosheets (Ag@rGO) was developed as a potential wound dressing. It was reported that the incorporation of Ag@rGO improved the stability and provides the irreversible gelation as well as significantly enhance the wound healing of methicillin-resistant S. aureus-infected wound.
4. Nanoparticle-loaded hydrogels for wound healing
Skin, being a 1st-line of defence of the human body, plays a vital role in regulating body temperature, shielding the human body from external injuries or pathogens, and is most likely to receive injuries. The skin wound is one of the most common clinical cases; hence it is of utmost necessity to develop an effective wound dressing. Wound dressings cover up the peri-wound area to provide a suitable wound-healing environment and prevent tissue dehydration. Conventional dressing systems are cheaper and easy to use but cannot provide a suitable wound-healing environment. Ideal dressing platforms should be biocompatible and have the ability to retain moisture with better mechanical strength.218 A new wound-dressing approach has emerged in the current hunt for effective wound dressings, using both drugs and various innovative biomaterials to optimize and promote wound healing.219 Researchers suggested that nanoparticle-loaded hydrogels for wound healing can be a better alternative to traditional dressing (Table 1). Hydrogels are three-dimensional with vigorous hydrophilic networks and can mimic the skin's ECM structure. Along with this, hydrogels have some inherent properties like better absorption and retention of water, biocompatible, and biodegradability to promote the healing process. Modern-day, wound dressing systems focuses on the natural polymer (e.g., chitosan, gelatin, collagen, cellulose, hyaluronic acid, etc.) hydrogel dressings over synthetic polymers because of their excellent biodegradability and biocompatibility and the ability to improve the microenvironment of the wound and promote healing at different stages.220 Nanomaterials in hydrogels have significantly enhanced mechanical strength by forming electrostatic interactions, hydrophobic interactions, hydrogen bonds, covalent bonds, etc.221 NPs, mainly metal-based NPs (e.g., silver, gold, copper), metal oxide NPs (e.g., zinc oxide, titanium oxide, cerium oxide), polymer (e.g., polyurethane, poly(lactic-glycolic acid), etc.) and glass-ceramic nanoparticles are exploited for wound healing. A study of nanogel based wound healing, Zhao and co-workers developed a genetically engineered polypeptide nanogel loaded with silver NPs as a wound healing as well as an antibacterial agent.222 An earlier study suggested that cerium-doped biotype Linde type a zeolite-based NPs loaded chitosan hydrogel have the ability to healing the diabetic wound by eliminated mitochondrial ROS and promoted the angiogenesis.223 Similarly, advanced bioceramics and polymer based hydrogel play an integral role in wound healing process. Additionally, these nanoparticles showed antibacterial and antitumoral activity along with the enhanced osteogenesis and angiogenesis.224,225 Sharifi and co-workers developed a skin substitute in the form of silver-doped bioactive glass-ceramic nanoparticles-based embryonic fibroblast cell loaded hydrogel. This substitute could enhance the cell attachment, proliferation and angiogenesis.226
Table 1 Various nanoparticle loaded hydrogel materials used for wound healing
Types of hydrogels |
Nanomaterial used |
Dosages/exposure to wound area |
Advantages |
Applications |
References |
Guar gum hydrogels |
Silver NPs |
16 days |
Boost up the proliferation, migration, and collagen production of human dermal fibroblasts |
Antibacterial agent |
220 |
Calcium alginate hydrogel |
Chitosan NPs |
MBC of 100 μg mL−1 |
Induced skin wound healing and re-epithelialization by promoting the secretion of IL-6 in vascular endothelial cell |
Antibacterial agent against E. coli and S. aureus |
227 |
Poly ethylene glycol and cationic poly allyl amine hydrochloride hydrogel |
Gold NPs |
21 days |
Enhanced skin re-epithelization and collagen deposition |
Antibacterial agent against S. aureus and P. aeruginosa |
228 |
Polycaperlactone and gelatin scaffold |
Chitosan NPs |
14 days |
Promoted cell attachment and proliferation |
Suitable scaffold for epidermis engineering and regenerative medicine |
229 |
Carboxymethylcellulose hydrogel |
Silver NPs |
50 ppm |
Maintains moist wound environment and facilitate early wound healing |
Suitable for the treatment of deep infected wounds and antibacterial agent against methicillin resistant S. aureus |
230 |
Cellulose hydrogel |
Silver NPs |
— |
High cytocompatibility and better antioxidant properties |
Suitable for moist wound dressing, antimicrobial agent against S. aureus, P. aeruginosa, and C. auris |
231 |
Pullulan microneedle patch |
Chitosan/fucoidan NPs |
7 days |
Helps to restore the collagen deposition by accelerated cell proliferation, granulation, and reduced pro-inflammatory cytokines |
Smart and combined therapy for high-quality wound healing |
232 |
Chitosan hydrogel |
Silver NPs |
250 ppm |
Biodegradable porous hydrogels showed fast wound healing with no scar marks |
Effective for diabetic patients and chronic burn wounds and antimicrobial agent against S. aureus, and E. coli |
233 |
Gelatine hydrogel |
Silver NPs |
MIC of 63 μg mL−1 |
Suitable as a vehicle for wound drug delivery due to its better physical properties |
Antibacterial and anti-biofilm agent against S. aureus, B. subtilis, P. aeruginosa and E. coli |
234 |
Collagen/chitosan scaffolds |
Silver NPs |
10 ppm |
Modulate local inflammatory responses and promote fibroblast migration by interacting with macrophages and fibroblasts |
Ideal dermal substitute for wound regeneration |
235 |
Poloxamer 407-based polymeric hydrogel |
Polyurethane NPs |
10 days |
Boost up the drug retention time in the tumor tissue |
Effective against glioblastoma multiforme |
236 |
Polymeric hydrogel |
Simvastatin polymeric nanoparticles |
11 days |
Induced epithelialization, collagen fiber formation, and growth of hair follicle |
Suitable for the topical wound healing |
237 |
Cell loaded nanofibrous hydrogel |
Glass–ceramic nanoparticles |
21 days |
Promoted cell attachment and proliferation, angiogenesis, collagen formation, regeneration of the sebaceous glands and hair follicles |
Antibacterial agent |
226 |
Gelatin collagen bioactive glass nanocomposite |
Bioactive glass nanoparticles |
14 days |
Triggers expression of VEGF and angiogenesis |
Suitable for the myocardial tissue engineering |
238 |
Thiolated hyaluronic acid/silk fibroin dual-network hydrogel |
Bioglass nanoparticles |
14 days |
Stimulate the migration of both fibroblasts and human umbilical vein endothelial cells |
Suitable for the wound healing |
239 |
Chitosan hydrogel |
Nano bioglass |
— |
Cytocompatible and induce rapid blood clotting |
Bleeding controlling agent |
240 |
Chitosan/silk fibroin/glycerophosphate hydrogel |
Bioactive glass nanoparticles |
8 weeks |
Induces osteogenesis and angiogenesis |
Suitable for bone regeneration |
241 |
Polymeric hydrogel |
Eudragit nanoparticles |
13 days |
Acts as an efficient free radical scavenger |
Promising for diabetic wound dressing |
242 |
Gelatin based hydrogel |
Asiaticoside polymeric nanoparticles |
— |
Improved collagen biosynthesis |
Promising wound dressing |
243 |
Polyvinyl alcohol nanofiber based hydrogel |
Chitosan nanoparticles |
— |
Cytocompatible and shows continuous and slow release of drugs |
Antibacterial agent |
244 |
Dextran based hydrogel |
Cerium oxide nanoparticle |
— |
Prolonged drug release, accelerated cell migration, in vivo anti-inflammatory activity |
Ideal wound dressing |
245 |
Poly(N-isopropylacrylamide) (PNIPAM) hydrogel |
Silk fibroin-sodium alginate nanoparticles |
20 days |
Induces proliferation and growth of the fibroblast cells |
Promising wound dressing |
246 |
Silica-collagen type I nanocomposite hydrogel |
Gentamicin and rifamicin, encapsulate silica nanoparticles |
37 mg mL−1 |
Shows prolonged antibacterial activity against Pseudomonas aeruginosa and Staphylococcus aureus |
Anti-bacterial wound dressing |
104 |
Chitosan/gelatin hydrogel |
Calcium phosphate nanoparticles |
— |
Modulates the porosity, swelling rate and mechanical strength as well as hMSC proliferation of bone scaffolds |
Bone tissue engineering |
128 |
Gelatin hydrogel |
Titanium dioxide and zinc oxide nanoparticles |
— |
Exhibits adhesive effects between hydrogels, hydrogel/polymer, and tissues promoting wound closure and healing |
Effective antimicrobial tissue adhesive |
141 |
Carboxymethyl cellulose hydrogels |
Magnesium hydroxide nanoparticles |
— |
Shows biocompatibility, hemocompatibility and the high antibacterial activity against P. aeruginosa biofilm formation |
Potential wound dressing |
149 |
Chitosan/sodium alginate/polyacrylamide hydrogel |
Magnesium hydroxide nanoparticles |
— |
Shows mechanical properties, high biocompatibility, improved broad-spectrum antibacterial activity and enhances in vivo wound healing process in a skin defect rat model |
Next-generation wound dressing |
150 |
Chitosan hydrogel |
Titanium dioxide/aluminum oxide nanoparticles |
— |
Decreases total bacterial count, the expression of inflammatory genes, and increases the expression of proliferative genes, fibroblast, and collagen |
Promising wound-healing material |
153 |
Poly-(polyethyleneglycol citrate-co-N-isopropylacrylamide) hydrogel |
Copper metal organic framework nanoparticles |
— |
Enhances dermal cell migration in vitro and wound closure rates along with angiogenesis, collagen deposition, and re-epithelialization, in vivo |
Promising innovative dressing for the treatment of chronic wounds |
156 |
3-(Trimethoxysilyl)propyl methacrylate hydrogel |
Mesoporous silica (mSiO2) modified CuS nanoparticles |
— |
Stimulates fibroblast proliferation, angiogenesis and skin tissue regeneration as well as antibacterial effect |
Skin tissue engineering and antimicrobial wound dressing |
157 |
Gelatin methacryloyl hydrogel |
Cerium oxide nanoparticles |
— |
Shows free radical scavenging activity, in vitro cell proliferation, and in vivo diabetic wound healing activity |
Promising wound dressing for treating diabetic wounds |
159 |
Polyvinyl alcohol/chitosan hydrogel |
Cerium oxide nanoparticle |
5 days |
Shows antibacterial activity and healthy human dermal fibroblast viability |
Robust wound dressing agent |
161 |
5. Ethical aspects
The emergence of the concept of nanomedicine is nearly 35 years ago and the technological developments in this area raised ethical issues concerning the use of nanomaterials due to the concept of “nanotoxicity”. As the nanomaterials exhibit different properties compared to their bulk counterparts, new knowledge, and research are needed for the use of each nanoparticle, especially in medicine. Especially due to the increase in the surface area to volume ratio, nanomaterials have enhanced reactivity both in vitro and in vivo which brings the necessity to a serious tracing.247,248 Despite the various advantages of using NPs as a part of therapy/healing, there is an increased risk of cyto- and/or genotoxicity. This risk is also dependent on the size, shape, and concentration of the NPs.249,250 As the material type, size, and route of exposure change, the risks can also be changed. Therefore, one must consider every situation and material separately.251 Also, the accumulation of nanomaterials in the body can have its own potential toxic effects.252 While investigating the benefits and research areas of NPs, it is an ethical response to point out the safety issues regarding nanomedicine products before being used in clinical trials.253 It is stated that the ethical issues about using NPs in medicine, including patient's rights, safety, and clinical trials, are also valid for other medical science and technologies. Knowing that every medication can have the risk of causing a side effect, deciding whether a technology is proven to be effective and safe can have some uncertainties. Also, it is a matter of fact how much risk can be taken by the patients to accept new technology.254 If the potential risks of human exposure to nanomaterials can be stated certainly, scientists can work on risk minimization more effectively.255
6. Conclusions
Four overlapping and interdependent phases make up the well-organized process of wound healing where intricate cellular and molecular mechanisms regulate these phases. However, these stages occur naturally, but distinctive dressing strategies may improve and speed it up. Dressings have evolved in recent years with new options, such as polymer blends and nanotechnology tools, to produce better materials while ensuring an ideal climate for wound healing. The current review has covered the various wound healing techniques in great detail and shown both the benefits and pitfalls of using nanomaterials in hydrogel wound patches. In addition to strengthening the mechanical characteristics, water solubility, and cell adhesion, nanotechnology can also load different particles with specialized biological functions or antibacterial activities in the hydrogels to accomplish long-term and slow drug delivery. Moreover, it shows a more effective curative impact than traditional topical medications. Additionally, a lesser amount of medications can function better due to the huge surface area of the NPs, which also somewhat reduces its toxicity. Nanomaterial-enhanced custom wound dressings have enormous promise for customised wound treatment. To achieve minimal resistance and rapid wound healing, one should be aware of the major difficulties linked to nanomaterial wound wraps and how they interact with the wound. To comply with international health norms, manufacturing expenses should be decreased overall. As a result, the potential applications of hydrogels-NPs composites amalgamation are endless and should be further investigated in future studies.
The commercialization of nanoparticle-loaded hydrogels for wound healing has emerged as a promising field with significant potential for clinical applications. Silver, zinc oxide, or gold nanoparticles have been incorporated into these advanced hydrogel formulations, which have demonstrated impressive therapeutic properties facilitating healing of wounds. For instance, commercially available products like Nanomedic's SpinCare®,256 Systagenix's Acticoat™,257 and Mölnlycke's Mepilex® Ag258 utilize nanoparticle-decorated wound patches with antimicrobial activity, accelerated wound closure, and enhanced tissue regeneration. Commercial products containing nanoparticles have earned the attention of researchers, clinicians, and industry stakeholders, which has further accelerated the commercialization of nanoparticle-loaded hydrogels.259 The successful outcomes reported in studies evaluating the efficacy of silver nanoparticle-loaded hydrogels in treating chronic wounds256 and the accelerated wound healing capabilities demonstrated by zinc oxide nanoparticle-loaded hydrogels259 provide strong evidence for their commercial viability. Moreover, the biocompatibility and ease of synthesis of these hydrogel systems contribute towards their attractiveness for commercialization. The availability of commercial products and their prospects are poised to increase as ongoing research continues to reveal new insights and refine the properties of nanoparticle-loaded hydrogels, ultimately benefiting patients and contributing to the advancement of wound healing therapies in the future.
Author contributions
PD, MHC, SA and AKM: conceptualization. PD, RM, AKM, MHC and OLF: funding acquisition. SA, OLF and AKM: investigation. AKM: project administration. SA, AKM: supervision. MLRM, OLF and MHC: visualization. PD, MC, MU, SS, CS, EAK, SK, ENK, ST, FB, RM and PR: writing – original draft. MHC, OLF, SA and AKM: writing – review & editing.
Conflicts of interest
There are no conflicts to declare.
Acknowledgements
P. D. was provided with an independent PhD fellowship for financial assistance (UGC-JRF; NTA Ref. No. 201610181190). R. M. was provided DST-INSPIRE PhD Fellowship (DST-INSPIRE-SRF; INSPIRE Code-IF190457). A. K. M. would like to acknowledge DST-SERB (E. E. Q./2021/000058) for financial assistance. This work was supported by grants from Conselho Nacional de Desenvolvimento e Tecnológico (CNPq), Coordenação de Aperfeiçoamento de Pessoal de Nível Superior (CAPES), Universidade Federal de Mato Grosso do Sul (UFMS), Fundação de Apoio à Pesquisa do Distrito Federal (FAPDF) and Fundação de Apoio ao Desenvolvimento do Ensino, Ciência e Tecnologia do Estado de Mato Grosso do Sul (FUNDECT), Brazil.
Notes and references
- P. H. Wang, B.-S. Huang, H.-C. Horng, C.-C. Yeh and Y.-J. Chen, J. Chin. Med. Assoc., 2018, 2, 94–101 CrossRef PubMed.
- A. O. Ijaola, D. O. Akamo, F. Damiri, C. J. Akisin, E. A. Bamidele, E. G. Ajiboye, M. Berrada, V. O. Onyenokwe, S. Y. Yang, E. Asmatulu and J. Biomater, Sci. Polym. Ed., 2022, 33, 1998–2050 CAS.
- V. Junnuthula, P. Kolimi, D. Nyavanandi, S. Sampathi, L. K. Vora and S. Dyawanapelly, Int. J. Pharm., 2022, 14, 1860 CrossRef CAS PubMed.
- C. Heerschap, A. Nicholas and M. Whitehead, Int. Wound J., 2019, 16(1), 233–242 CrossRef PubMed.
- D. R. Childs and A. S. Murthy, Surg. Clin. North Am., 2017, 97, 189–207 CrossRef PubMed.
- C. Tyavambiza, M. Meyer and S. Meyer, Bioeng, 2022, 9, 712 CAS.
- R. Tiwari and K. Pathak, Pharmaceutics, 2023, 15, 634 CrossRef CAS PubMed.
- A. L. Kushwaha and B. S. Kim, Nanomater, 2022, 12, 618 CrossRef CAS.
- D. dos Santos Gomes, R. de Sousa Victor, B. V. de Sousa, G. de Araújo Neves, L. N. de Lima Santana and R. R. Menezes, J. Mater., 2022, 15, 3909 CrossRef CAS.
- D. Nunes, S. Andrade, M. J. Ramalho, J. A. Loureiro and M. C. Pereira, Polym. J., 2022, 14, 1010 CAS.
- H. Hu and F. J. Xu, Biomater. Sci., 2020, 8, 2084–2101 RSC.
- S. Bhubhanil, C. Talodthaisong, M. Khongkow, K. Namdee, P. Wongchitrat, W. Yingmema, J. A. Hutchison, S. Lapmanee and S. Kulchat, Sci. Rep., 2021, 11, 1–4 CrossRef.
- M. M. Arif, S. M. Khan, N. Gull, T. A. Tabish, S. Zia, R. U. Khan, S. M. Awais and M. A. Butt, Int. J. Pharmacol., 2021, 598, 120270 CrossRef.
- C. T. D. S. Shih and A. Khachemoune, J. Dermatol. Treat., 2020, 31, 639–648 CrossRef.
- Y. Z. Wang, U. Armato and J. Wu, Front. Bioeng. Biotechnol., 2020, 8, 14 CrossRef.
- H. S. Kim, Y. Sun, J. H. Lee, H. W. Kim, X. B. Fu and K. W. Leong, Adv. Drug Deliv. Rev., 2019, 146, 209–239 CrossRef CAS PubMed.
- I. Suntar, S. Cetinkaya, E. Panieri, S. Saha, B. Buttari, E. Profumo and L. Saso, Molecules, 2021, 26, 21 CrossRef.
- B. Enyedi and P. Niethammer, Trends Cell Biol., 2015, 25, 398–407 CrossRef CAS PubMed.
- V. Falanga, R. R. Isseroff, A. M. Soulika, M. Romanelli, D. Margolis, S. Kapp, M. Granick and K. Harding, Nat. Rev. Dis. Prim., 2022, 8, 21 CrossRef PubMed.
- B. Blanco-Fernandez, O. Castano, M. A. Mateos-Timoneda and E. E. S. Perez-Amodio, Adv. Wound Care, 2021, 10, 234–256 CrossRef PubMed.
- L. E. Lindley, O. Stojadinovic, I. Pastar and M. Tomic-Canic, Plast. Reconst. Surg., 2016, 138, 18S–28S CrossRef CAS PubMed.
- G. Han and R. Ceilley, Adv. Ther., 2017, 34, 599–610 CrossRef.
- S. Pourshahrestani, E. Zeimaran, N. A. Kadri, N. Mutlu and A. R. Boccaccini, Adv. Healthc. Mater., 2020, 9, 52 Search PubMed.
- N. X. Landen, D. Q. Li and M. Stahle, Cell. Mol. Life Sci., 2016, 73, 3861–3885 CrossRef CAS PubMed.
- S. Nandi, E. P. Sproul, K. Nellenbach, M. Erb, L. Gaffney, D. O. Freytes and A. C. Brown, Biomater. Sci., 2019, 7, 669–682 RSC.
- F. J. Martinez-Navarro, F. J. Martinez-Morcillo, S. de Oliveira, S. Candel, I. Cabas, A. Garcia-Ayala, T. Martinez-Menchon, R. Corbalan-Velez, P. Mesa-del-Castillo, M. L. Cayuela, A. B. Perez-Oliva, D. Garcia-Moreno and V. Mulero, Dev. Comp. Immunol., 2020, 105, 8 CrossRef PubMed.
- A. Kovtun, D. A. C. Messerer, K. Scharffetter-Kochanek, M. Huber-Lang and A. Ignatius, J. Immunol. Res., 2018, 2018, 12 Search PubMed.
- C. M. Minutti, J. A. Knipper, J. E. Allen and D. M. W. Zaiss, Semin. Cell Dev. Biol., 2017, 61, 3–11 CrossRef CAS PubMed.
- P. Krzyszczyk, R. Schloss, A. Palmer and F. Berthiaume, Front. Physiol., 2018, 9, 22 Search PubMed.
- R. B. Diller and A. J. Tabor, Biomimetics, 2022, 7(3), 87 CrossRef CAS PubMed.
- T. Maheswary, A. A. Nurul and M. B. Fauzi, Pharmaceutics, 2021, 13(7), 981 CrossRef CAS PubMed.
- M. B. Serra, W. A. Barroso, C. Rocha, P. G. R. Furtado, A. C. R. Borges, S. N. Silva, M. M. P. Tangerina, J. R. do Nascimento, W. Vilegas, A. C. Alves, D. F. Barbeiro, H. P. de Souza, I. C. Abreu and M. O. R. Borges, Evid. Based Complementary Altern. Med., 2020, 2020, 13 Search PubMed.
- G. Christoffersson and M. Phillipson, Cell Tissue Res., 2018, 371, 415–423 CrossRef PubMed.
- S. de Oliveira, E. E. Rosowski and A. Huttenlocher, Nat. Rev. Immunol., 2016, 16, 378–391 CrossRef CAS PubMed.
- M. Rodrigues, N. Kosaric, C. A. Bonham and G. C. Gurtner, Physiological Rev., 2019, 99, 665–706 CrossRef CAS.
- M. Phillipson and P. Kubes, Trends Immunol., 2019, 40, 635–647 CrossRef CAS PubMed.
- V. Papayannopoulos, Nat. Rev. Immunol., 2018, 18, 134–147 CrossRef CAS PubMed.
- M. B. Serra, W. A. Barroso, N. N. da Silva, S. D. Silva, A. C. R. Borges, I. C. Abreu and M. O. D. Borges, Int. J. Inflamm., 2017, 2017, 17 Search PubMed.
- K. Raziyeva, Y. Kim, Z. Zharkinbekov, K. Kassymbek, S. Jimi and A. Saparov, Biomolecules, 2021, 11(5), 700 CrossRef CAS PubMed.
- H. N. Wilkinson and M. J. Hardman, Open Biol., 2020, 10, 200223 CrossRef CAS.
- Q. Xu, W. Zhao, M. Yan and H. Mei, J. Inflamm., 2022, 19, 22 CrossRef CAS PubMed.
- W. Liu, M. Yu, D. Xie, L. Wang, C. Ye, Q. Zhu, F. Liu and L. Yang, Stem Cell Res. Ther., 2020, 11, 259 CrossRef CAS PubMed.
- G. J. Kotwal and S. Chien, Results Probl. Cell Differ., 2017, 62, 353–364 CrossRef CAS PubMed.
- A. E. Louiselle, S. M. Niemiec, C. Zgheib and K. W. Liechty, Transl. Res., 2021, 236, 109–116 CrossRef CAS PubMed.
- S. J. Wolf, W. J. Melvin and K. Gallagher, Semin. Cell Dev. Biol., 2021, 119, 111–118 CrossRef CAS PubMed.
- A. S. Kimball, F. M. Davis, A. DenDekker, A. D. Joshi, M. A. Schaller, J. Bermick, X. Y. Xing, C. F. Burant, A. T. Obi, D. Nysz, S. Robinson, R. Allen, N. W. Lukacs, P. K. Henke, J. E. Gudjonsson, B. B. Moore, S. L. Kunkel and K. A. Gallagher, Immunity, 2019, 51, 258 CrossRef CAS PubMed.
- I. Visan, Nat. Immunol., 2019, 20, 1089 Search PubMed.
- M. Li, Q. Hou, L. Zhong, Y. Zhao and X. Fu, Front. Immunol., 2021, 16, 681710 CrossRef.
- K. Jung, S. Covington, C. K. Sen, M. Januszyk, R. S. Kirsner, G. C. Gurtner and N. H. Shah, Wound Repair Regen., 2016, 24, 181–188 CrossRef PubMed.
- R. G. Frykberg, Medicina, 2021, 57, 917 CrossRef PubMed.
- E. Shaabani, M. Sharifiaghdam, R. Faridi-Majidi, S. C. De Smedt, K. Braeckmans and J. C. Fraire, Mol. Ther. Nucleic Acids, 2022, 29, 871–899 CrossRef CAS PubMed.
- S. Das, P. Bose, E. Lejeune, D. Reich, C. Chen and J. Eyckmans, Tissue Eng., 2021, 27, 1447–1457 CrossRef CAS PubMed.
- R. L. Zhao, H. L. N. Liang, E. Clarke, C. Jackson and M. L. Xue, Int. J. Mol. Sci., 2016, 17, 14 Search PubMed.
- L. A. DiPietro, J. Leukoc. Biol., 2016, 100, 979–984 CrossRef CAS PubMed.
- M. Chang and T. T. Nguyen, Acc. Chem. Res., 2021, 54, 1080–1093 CrossRef CAS PubMed.
- A. Kisling, R. M. Lust and L. C. Katwa, Life Sci., 2019, 228, 30–34 CrossRef CAS PubMed.
- L. Moretti, J. Stalfort, T. H. Barker and D. Abebayehu, J. Biol. Chem., 2022, 298, 101530 CrossRef CAS PubMed.
- T. A. Wynn and K. M. Vannella, Immunity, 2016, 44, 450–546 CrossRef CAS PubMed.
- R. C. de Oliveira and S. E. Wilson, Opthalmol. Vis. Sci., 2020, 61, 28 CrossRef CAS PubMed.
- H. Sorg, D. J. Tilkorn, S. Hager, J. Hauser and U. Mirastschijski, Eur. Surg. Res., 2017, 58, 81–94 CrossRef PubMed.
- S. S. Mathew-Steiner, S. Roy and C. K. Sen, Bioengineering, 2021, 8, 15 CrossRef.
- B. Hinz, Exp. Eye Res., 2016, 142, 56–70 CrossRef CAS PubMed.
- R. T. Li, K. Liu, X. Huang, D. Li, J. X. Ding, B. Liu and X. S. Chen, Adv. Sci., 2022, 9, 22 Search PubMed.
- G. Wang, F. Yang, W. Zhou, N. Xiao, M. Luo and Z. Tang, Biomed. Pharmacother., 2023, 157, 114004 CrossRef.
- B. A. R. N. Durand, C. Pouget, C. Magnan, V. Molle, J. P. Lavigne and C. Dunyach-Remy, Microorganisms, 2022, 10, 1500 CrossRef CAS PubMed.
- G. H. R. Vale de Macedo, G. D. E. Costa, E. R. Oliveira, G. V. Damasceno, J. S. P Mendonça, L. D. S. Silva, V. L. Chagas, J. M. N. Bazán, A. S. D. S. Aliança, R. C. M. Miranda, A. Zagmignan, A. S. Monteiro and L. C. Nascimento da Silva, Pathogens, 2021, 10, 148 CrossRef CAS PubMed.
- G. de Macedo, G. D. E. Costa, E. R. Oliveira, G. V. Damasceno, J. S. P. Mendonca, L. D. Silva, V. L. Chagas, J. M. N. Bazan, A. S. D. Alianca, R. D. M. de Miranda, A. Zagmignan, A. D. Monteiro and L. C. N. da Silva, Pathogens, 2021, 10, 34 CrossRef.
- B. Short, A. Bakri, A. Baz, C. Williams, J. Brown and G. Ramage, Curr. Clin. Microbiol. Rep., 2023, 10, 9–16 CrossRef.
- S. T. Khan, J. Musarrat and A. A. Al-Khedhairy, Colloids Surf., B, 2016, 146, 70–83 CrossRef CAS.
- I. Negut, V. Grumezescu and A. M. Grumezescu, Molecules, 2018, 23, 2392 CrossRef PubMed.
- M. Ahmadi and M. Adibhesami, Iran. J. Pharm. Sci., 2018, 16, 661 Search PubMed.
- S. H. I. Pastar, S. Drakulich, E. Dikici, M. Tomic-Canic, S. Deo and S. Daunert, ACS Cent. Sci., 2017, 3, 163–175 CrossRef.
- V. Vijayakumar, S. K. Samal, S. Mohanty and S. K. Nayak, Int. J. Biol. Macromol., 2019, 122, 137–148 CrossRef CAS PubMed.
- H. H. Lara, N. V. Ayala-Núnez, L. D. IxtepanTurrent and C. Rodríguez Padilla, World J. Microbiol. Biotechnol., 2010, 26, 615–621 CrossRef CAS.
- M. L. Knetsch and L. H. Koole, Polym. J., 2011, 3, 340–366 CAS.
- R. Y. Pelgrift and A. J. Friedman, Adv. Drug Deliv. Rev., 2013, 65, 1803–1815 CrossRef CAS PubMed.
- R. Singla, S. Soni, P. M. Kulurkar, A. Kumari, S. Mahesh, V. Patial, Y. S. Padwad and S. K. Yadav, Carbohydr. Polym., 2017, 155, 152–162 CrossRef CAS PubMed.
- Z. Boroumand, N. Golmakani and S. Boroumand, Nanomed. J., 2018, 5 CAS.
- K. Niska, E. Zielinska, M. W. Radomski and I. Inkielewicz-Stepniak, Chem. Biol. Interact., 2018, 295, 38–51 CrossRef CAS PubMed.
- N. Volkova, M. Yukhta, O. Pavlovich and A. Goltsev, Nano Res., 2016, 11, 1–6 CAS.
- V. Pivodová, J. Franková, A. Galandáková and J. Ulrichová, Nanomed, 2015, 2, 2–7 Search PubMed.
- O. Akturk, K. Kismet, A. C. Yasti, S. Kuru, M. E. Duymus, F. Kaya, M. Caydere, S. Hucumenoglu and D. Keskin, J. Biomater. Appl., 2016, 2, 283–301 CrossRef PubMed.
- R. Jayakumar, M. Prabaharan, P. S. Kumar, S. V. Nair and H. Tamura, Biotechnol. Adv., 2011, 29, 322–337 CrossRef CAS PubMed.
- M. M. Mihai, M. B. Dima, B. Dima and A. M. Holban, Mater, 2019, 12, 2176 CrossRef CAS.
- J. G. Leu, S. A. Chen, H. M. Chen, C. F. Wu, W. M. Hung, Y. D. Yao, C. S. Tu and Y. J. Liang, Nanomed, 2012, 8, 767–775 CrossRef CAS PubMed.
- S. Medhe, P. Bansal and M. M. Srivastava, Appl. Nanosci., 2014, 4, 153–161 CrossRef CAS.
- N. O. Yakimovich, A. A. Ezhevskii, D. V. Guseinov, L. A. Smirnova, T. A. Gracheva and K. S. Klychkov, Russ. Chem. Bull., 2008, 57, 520–523 CrossRef CAS.
- H. Gu, L. Ho, E. Tong, L. Wang and B. Xu, Nano Lett., 2003, 3, 1261–1263 CrossRef CAS.
- R. S. Norman, J. W. Stone, A. Gole, C. J. Murphy and T. L. Sabo-Attwood, Nano Lett., 2008, 1, 302–306 CrossRef PubMed.
- J. Gil-Tomás, S. Tubby, I. P. Parkin, N. Narband, L. Dekker, S. P. Nair, M. Wilson and C. Street, J. Mater. Chem., 2007, 17, 3739–3746 RSC.
- G. Borkow, J. Gabbay and R. C. Zatcoff, Med. Hypotheses, 2008, 70, 610–613 CrossRef CAS.
- J. Salvo and C. Sandoval, Burn. Trauma, 2022, 1, 10 Search PubMed.
- M. Tiwari, K. Narayanan, M. B. Thakar, H. V. Jagani and J. Venkata Rao, IET Nanobiotechnol., 2014, 8, 230–237 CrossRef.
- S. Alizadeh, B. Seyedalipour, S. Shafieyan, A. Kheime, P. Mohammadi and N. Aghdami, Biochem. Biophys. Res. Commun., 2019, 517, 684–690 CrossRef CAS.
- T. Kruk, K. Szczepanowicz, J. Stefańska, R. P. Socha and P. Warszyński, Colloids Surf., B, 2015, 128, 17–22 CrossRef CAS PubMed.
- S. K. Nethi, S. Das, C. R. Patra and S. Mukherjee, Biomater. Sci., 2019, 7, 2652–2674 RSC.
- C. Sen, C. K. Khanna, S. Venojarvi, M. Trikha, P. Ellison, E. C. Hunt and S. Roy, J. Physiol. Heart Circ. Physiol., 2002, 282, 1821–1827 CrossRef.
- J. Li, D. Zhai, F. Lv, Q. Yu, H. Ma, J. Yin and C. Wu, Acta Biomater., 2016, 36, 254–266 CrossRef CAS.
- F. Buyukserin, S. Altuntas and B. Aslım, RSC Adv., 2014, 4, 23535–23539 RSC.
- G. H. Gwak, A. J. Choi, Y. S. Bae, H. J. Choi and J. M. Oh, Biomater. Res., 2016, 20, 1–8 CrossRef PubMed.
- N. Shadjou and M. Hasanzadeh, J. Biomed. Mater. Res. A, 2016, 104, 1250–1275 CrossRef CAS PubMed.
- J. A. Killion, S. Kehoe, L. M. Geever, D. M. Devine, E. Sheehan, D. Boyd and C. L. Higginbotham, Mater. Sci. Eng. C, 2013, 33, 4203–4212 CrossRef CAS PubMed.
- N. Wang, S. T. Dheen, J. Y. Fuh and A. S. Kumar, Int. J. Bioprinting, 2021, 23, 00146 Search PubMed.
- G. S. Alvarez, C. Hélary, A. M. Mebert, X. Wang, T. Coradin and M. F. Desimone, J. Mater. Chem. B., 2014, 29, 4660–4670 RSC.
- F. Öri, R. Dietrich, C. Ganz, M. Dau, D. Wolter, A. Kasten, T. Gerber and B. Frerich, J. Craniomaxillofac. Surg., 2017, 45, 99–107 CrossRef.
- S. Rose, A. Prevoteau, P. Elzière, D. Hourdet, A. Marcellan and L. Leibler, Nature, 2014, 505, 382–385 CrossRef CAS PubMed.
- M. Shen, L. Wang, L. Feng, Y. Gao, S. Li, Y. Wu and G. Pei, Int. J. Nanomed., 2022, 17, 2593–2608 CrossRef.
- X. Wang, J. Chang and C. Wu, Appl. Mater. Today, 2018, 11, 308–319 CrossRef.
- T. Mehrabi, A. S. Mesgar and Z. Mohammadi, ACS Biomater. Sci. Eng., 2020, 6, 5399–5430 CrossRef CAS PubMed.
- A. M. Villalba-Rodríguez, S. Martínez-González, J. E. Sosa-Hernández, R. Parra-Saldívar, M. Bilal and H. M. Iqbal, Gels, 2021, 7, 1–13 CrossRef PubMed.
- T. Yu, Y. Hu, W. He, Y. Xu, A. Zhan, K. Chen and L. Jiang, Mater. Today Bio., 2023, 19, 100558 CrossRef CAS.
- L. Gao, Y. Zhou, J. Peng, C. Xu, Q. Xu, M. Xing and J. Chang, NPG Asia Mater., 2019, 11, 66 CrossRef CAS.
- B. Li, H. Tang, X. Bian, K. Ma, J. Chang, X. Fu and C. Zhang, Burn. Trauma, 2021, 9, 29 Search PubMed.
- P. Shen, Y. Chen, S. Luo, Z. Fan, J. Wang, J. Chang and J. Deng, Acta Biomater., 2021, 126, 31–44 CrossRef CAS PubMed.
- M. Prabaharan, and P. R. Sivashankari, Book: Chitin and Chitosan for Regenerative Medicine, 2016, pp. 41–59 Search PubMed.
- P. Nezhad-Mokhtari, M. Akrami-Hasan-Kohal and M. Ghorbani, Int. J. Biol. Macromol., 2020, 154, 198–205 CrossRef CAS PubMed.
- C. Mao, X. Chen, G. Miao and C. Lin, J. Biomed. Mater., 2015, 10, 025005 CrossRef.
- H. Li, J. He, H. Yu, C. R. Green and J. Chang, Biomater, 2016, 84, 64–75 CrossRef CAS.
- M. Parani, G. Lokhande, A. Singh and A. K. Gaharwar, ACS Appl. Mater. Interfaces, 2016, 8, 10049–10069 CrossRef CAS.
- T. Maeda, M. Kitagawa, A. Hotta and S. Koizumi, Polym. J., 2019, 11, 250 Search PubMed.
- N. Rajab, M. Kharaziha, R. Emadi, A. Zarrabi, H. Mokhtari and S. Salehi, J. Colloid Interface Sci., 2020, 564, 155–169 CrossRef.
- J. Hum and A. R. Boccaccini, Int. J. Mol. Sci., 2018, 19, 1–21 Search PubMed.
- C. Yang, R. Zheng, M. R. Younis, J. Shao, L. H. Fu, D. Y. Zhang, J. Lin, Z. Li and P. Huang, Chem. Eng. J., 2021, 419, 129437 CrossRef CAS.
- L. Kong, Z. Wu, H. Zhao, H. Cui, J. Shen, J. Chang, H. Li and Y. He, ACS Appl. Mater. Interfaces, 2018, 10, 30103–30114 CrossRef CAS PubMed.
- Q. Xu, F. Jiang, G. Guo, E. Wang, M. R. Younis, Z. Zhang, F. Zhang, Z. Huan, C. Fan, C. Yang, H. Shen and J. Chang, Nano Today, 2021, 41, 101330 CrossRef CAS.
- L. Ma, Y. Zhou, Z. Zhang, Y. Liu, D. Zhai, H. Zhuang, Q. Li, J. Yuye, C. Wu and J. Chang, Sci. Adv., 2020, 6, 1311 CrossRef.
- A. Gantar, N. Drnovšek, P. Casuso, A. Pérez-San Vicente, J. Rodriguez, D. Dupin, S. Novak and I. Loinaz, RSC Adv., 2016, 6, 69156–69166 RSC.
- L. Nie, Q. Wu, H. Long, K. Hu, P. Li, C. Wang, M. Sun, J. Dong, X. Wei, J. Suo and D. Hua, J. Biomater. Sci. Polym. Ed., 2019, 30, 1636–1657 CrossRef CAS PubMed.
- E. Ryan and S. Yin, Ceram. Int., 2022, 48, 15516–15524 CrossRef CAS.
- S. Hamdan, I. Pastar, S. Drakulich, E. Dikici, M. Tomic-Canic, S. Deo and S. Daunert, ACS Cent. Sci., 2017, 3, 163–175 CrossRef CAS PubMed.
- K. Shanmugapriya and H. W. Kang, ACS Cent. Sci., 2019, 105, 110110 CAS.
- M. Biondi, A. Borzacchiello, L. Mayol and L. Ambrosio, Gels, 2015, 1, 162–178 CrossRef PubMed.
- M. Berthet, Y. Gauthier, C. Lacroix, B. Verrier and C. Monge, Trends Biotechnol., 2017, 35, 770–784 CrossRef CAS PubMed.
- M. Kharaziha, A. Baidya and N. Annabi, Adv. Mater., 2021, 33, 2100176 CrossRef CAS.
- B. Echalar, D. Dostalova, K. Palacka, E. Javorkova, B. Hermankova, T. Cervena, A. Zajicova, V. Holan and P. Rossner, Toxicol. Vitro, 2023, 87, 105536 CrossRef CAS.
- M. Kaushik, R. Niranjan, R. Thangam, B. Madhan, V. Pandiyarasan, C. Ramachandran and G. D. Venkatasubbu, Appl. Surf. Sci., 2019, 479, 1169–1177 CrossRef CAS.
- M. Alavi and A. Nokhodchi, Carbohydr. Polym., 2020, 227, 115349 CrossRef CAS PubMed.
- V. K. H. Bui, D. Park and Y. C. Lee, Polymers, 2017, 9, 1–24 CrossRef PubMed.
- S. E. Jin and H. E. Jin, Int. J. Pharm., 2019, 11, 1–26 Search PubMed.
- P. C. Balaure, A. M. Holban, A. M. Grumezescu, G. D. Mogoşanu, T. A. Bălşeanu, M. S. Stan, A. Dinischiotu, A. Volceanov and L. Mogoantă, Int. J. Pharm., 2019, 557, 199–207 CrossRef CAS.
- Y. Gao, Y. Han Y, M. Cui, H. L. Tey, L. Wang and C. Xu, J. Mater. Chem. B., 2017, 5, 4535–4541 RSC.
- A. Khalid, H. Ullah, M. Ul-Islam, R. Khan, S. Khan, F. Ahmad, T. Khan and F. Wahid, RSC Adv., 2017, 7, 47662–47668 RSC.
- Z. A. Khan, S. Jamil, A. Akhtar, M. M. Bashir and M. Yar, Int. J. Polym. Mater. Polym. Biomater., 2019, 69, 419–436 CrossRef.
- V. Sivaranjani and P. J. Philominathan, Wound Med., 2016, 12, 1–5 CrossRef.
- D. Bhattacharya, B. Ghosh and M. Mukhopadhyay, IET Nanobiotechnol., 2019, 13, 778–785 CrossRef PubMed.
- G. A. Seisenbaeva, V. G. Kessler, K. Fromell, B. Nilsson, K. N. Ekdahl, V. V. Vinogradov, A. N. Terekhov and A. V. Pakhomov, Sci. Rep., 2018, 8, 4416 CrossRef PubMed.
- G. A. Seisenbaeva, K. Fromell, V. V. Vinogradov, A. N. Terekhov, A. V Pakhomov, B. Nilsson, K. N. Ekdahl, V. V. Vinogradov and V. G. Kessler, Sci. Rep., 2017, 7, 15448 CrossRef.
- L. M. Anaya-Esparza, J. M. Ruvalcaba-Gómez, C. I. González-Silva, N. Romero-Toledo, R. Aguilera-Aguirre and E. Montalvo-González, Materials, 2020, 13, 1–27 CrossRef PubMed.
- R. Eivazzadeh-Keihan, F. Khalili, N. Khosropour, H. A. M. Aliabadi, F. Radinekiyan, S. Sukhtezari and S. Lanceros-Mendez, ACS Appl. Mater. Interfaces, 2021, 13, 33840–33849 CrossRef CAS PubMed.
- J. Qu, J. Li, W. Zhu, Y. Xu, S. Yang and X. Qian, Polymer, 2022, 251, 124902 CrossRef CAS.
- V. Vijayakumar, S. K. Samal, S. Mohanty and S. K. Nayak, Int. J. Biol. Macromol., 2019, 122, 137–148 CrossRef CAS PubMed.
- M. Tanveer, A. Farooq, S. Ata, I. Bibi, M. Sultan, M. Iqbal and S. H. Al-Mijalli, Surface. Interfac., 2021, 25, 101285 CrossRef CAS.
- A. Rahmanpour, M. R. Farahpour, R. Shapouri, S. Jafarirad and P. Rahimi, Colloids Surf. A Physicochem. Eng. Asp., 2022, 644, 128839 CrossRef CAS.
- S. Altuntas, H. K. Dhaliwal, A. E. Radwan, M. Amiji and F. Buyukserin, RSC Biomater. Sci., 2023, 11, 181–194 RSC.
- S. Altuntas, H. K. Dhaliwal, N. J. Bassous, A. E. Radwan, P. Alpaslan, T. Webster, F. Buyukserin and M. Amiji, ACS Biomater. Sci. Eng., 2021, 5, 4311–4322 CrossRef PubMed.
- J. Xiao, S. Chen, J. Yi, H. F. Zhang and G. A. Ameer, Adv. Funct. Mater., 2017, 27, 1604872 CrossRef PubMed.
- M. Li, X. Liu, L. Tan, Z. Cui, X. Yang, Z. Li and S. Wu, Biomater. Sci., 2018, 6, 2110–2121 RSC.
- S. Chigurupati, M. R. Mughal, E. Okun, S. Das, A. Kumar, M. McCaffery and M. P. Mattson, Biomater, 2013, 33, 2194–2201 CrossRef PubMed.
- R. Augustine, A. A. Zahid, A. Hasan, Y. B. Dalvi and J. Jacob, ACS Biomater. Sci. Eng., 2020, 7, 279–290 CrossRef PubMed.
- L. C. Dewberry, S. M. Niemiec, S. A. Hilton, A. E. Louiselle, S. Singh, T. S. Sakthivel and C. Zgheib, Nanomed.: Nanotechnol. Biol. Med., 2022, 40, 102483 CrossRef CAS PubMed.
- K. Kalantari, E. Mostafavi, B. Saleh, P. Soltantabar and T. J. Webster, Eur. Polym. J., 2020, 134, 109853 CrossRef CAS.
- C. I. Crucho and M. T. Barros, Mater. Sci. Eng. C, 2017, 80, 771–784 CrossRef CAS PubMed.
- A. Bolhassani, S. Javanzad, T. Saleh, M. Hashemi, M. R. Aghasadeghi and S. M. Sadat, Hum. Vaccines Immunother., 2014, 10, 321–332 CrossRef CAS PubMed.
- Z. Edis, J. Wang, M. K. Waqas, M. Ijaz and M. Ijaz, Int. J. Nanomed., 2021, 16, 1313 CrossRef.
- A. Zielińska, F. Carreiró, A. M. Oliveira, A. Neves, B. Pires, D. N. Venkatesh, A. Durazzo, M. Lucarini, P. Eder, A. M. Silva and A. Santini, Molecules, 2020, 25, 3731 CrossRef PubMed.
- S. S. Guterres, M. P. Alves and A. R. Pohlmann, Drug Target Insights, 2007, 2, 117739280700200002 CrossRef.
- S. Deng, M. R. Gigliobianco, R. Censi and P. Di Martino, Nanomaterials, 2020, 10, 847 CrossRef CAS PubMed.
- K. S. Soppimath, T. M. Aminabhavi, A. R. Kulkarni and W. E. Rudzinski, J. Control Release, 2001, 70, 1–20 CrossRef CAS.
- A. Cano, M. Ettcheto, J. H. Chang, E. Barroso, M. Espina, B. A. Kühne, M. Barenys, C. Auladell, J. Folch, E. B. Souto and A. Camins, J. Control Release, 2019, 301, 62–75 CrossRef CAS.
- S. A. Dilliard and D. J. Siegwart, Nat. Mater., 2023, 19, 1–9 Search PubMed.
- X. Chen, S. Hussain, A. Abbas, Y. Hao, A. H. Malik, X. Tian, H. Song and R. Gao, Mikrochim. Acta, 2022, 183, 83 CrossRef PubMed.
- R. Rai, S. Alwani and I. Badea, Polym. J., 2019, 11, 745 CAS.
- M. Varga, Self-assembly of nanobiomaterials, William Andrew, Publishing, 2016, ch. 31, pp. 57−90 Search PubMed.
- M. Dergham, S. Lin and J. Geng, Angew. Chem., Int. Ed. Engl., 2022, 61, e202114267 CrossRef CAS PubMed.
- X. Sun, Y. Dong, Y. Liu, N. Song, F. Li and D. Yang, Sci. China Chem., 2021, 65, 31–47 CrossRef.
- Nanochemistry: Synthesis, Characterization and Applications,ed. A. Sharma, and G. Oza, CRC Press, 1st edn, 2023 Search PubMed.
- H. J. Kang, N. Chen, B. C. Dash, H. C. Hsia and F. Berthiaume, Adv. Wound Care, 2021, 10, 221–233 CrossRef.
- L. B. Vong, Y. Sato, P. Chonpathompikunlert, S. Tanasawet, P. Hutamekalin and Y. Nagasaki, Acta Biomater., 2020, 109, 220–228 CrossRef CAS PubMed.
- C. Pan, J. Wu, S. Qing, X. Zhang, L. Zhang, H. Yue, M. Zeng, B. Wang, Z. Yuan, Y. Qiu, H. Ye, D. Wang, X. Liu, P. Sun, B. Liu, E. Feng, X. Gao, L. Zhu, W. Wei, G. Ma and H. Wang, Adv. Mater., 2020, 32, e2002940 CrossRef.
- M. Melo, E. Porter, Y. Zhang, M. Silva, N. Li, B. Dobosh, A. Liguori, P. Skog, E. Landais, S. Menis, D. Sok, D. Nemazee, W. R. Schief, R. Weiss and D. J. Irvine, Mol. Ther., 2019, 27, 2080–2090 CrossRef CAS PubMed.
- P. Kolimi, S. Narala, D. Nyavanandi, A. A. A. Youssef and N. Dudhipala, Cells, 2022, 11 Search PubMed.
- V. Linko, A. Ora and M. A. Kostiainen, Trends Biotechnol., 2015, 33, 586–594 CrossRef CAS PubMed.
- D. Muraoka, N. Harada, H. Shiku and K. Akiyoshi, J. Control Release, 2022, 347, 175–182 CrossRef CAS PubMed.
- S. Peressotti, G. E. Koehl, J. A. Goding and R. A. Green, ACS Biomater. Sci. Eng., 2021, 7, 4136–4163 CrossRef CAS PubMed.
- S. Yadav, A. K. Sharma and P. Kumar, Front. Bioeng. Biotechnol., 2020, 8, 127 CrossRef.
- W.-Y. Chen, H.-Y. Chang, J.-K. Lu, Y.-C. Huang, S. G. Harroun, Y.-T. Tseng, Y.-J. Li, C.-C. Huang and H.-T. Chang, Adv. Funct. Mater., 2015, 25, 7189–7199 CrossRef CAS.
- H. Jia, X. Zeng, S. Fan, R. Cai, Z. Wang, Y. Yuan and T. Yue, Int. J. Biol. Macromol., 2022, 210, 703–715 CrossRef CAS.
- S. Perez-Rafael, K. Ivanova, I. Stefanov, J. Puiggali, L. J. Del Valle, K. Todorova, P. Dimitrov, D. Hinojosa-Caballero and T. Tzanov, Acta Biomater., 2021, 134, 131–143 CrossRef CAS PubMed.
- A. G. Morena, S. Perez-Rafael and T. Tzanov, Pharmaceutics, 2022, 14, 1–20 CrossRef PubMed.
- L. Sun, A. Li, Y. Hu, Y. Li, L. Shang and L. Zhang, Part. Part. Syst. Char., 2019, 36, 1800420 CrossRef.
- E. Santhini, R. Parthasarathy, M. Shalini, S. Dhivya, L. A. Mary and V. V. Padma, Int. J. Biol. Macromol., 2022, 197, 77–87 CrossRef CAS PubMed.
- H. Huang, W. Gong, X. Wang, W. He, Y. Hou and J. Hu, Adv. Health Mater., 2022, 11, e2102476 CrossRef PubMed.
- W. Liu, W. Ou-Yang, C. Zhang, Q. Wang, X. Pan, P. Huang, C. Zhang, Y. Li, D. Kong and W. Wang, ACS Nano, 2020, 14, 12905–12917 CrossRef CAS.
- J. Zhu, H. Zhou, E. M. Gerhard, S. Zhang, F. I. Rodríguez, T. Pan, H. Yang, Y. Lin, J. Yang and H. Cheng, Bioact. Mater., 2023, 19, 360–375 CrossRef PubMed.
- G. Shi, W. Chen, Y. Zhang, X. Dai, X. Zhang and Z. Wu, Langmuir, 2018, 35, 1837–1845 CrossRef PubMed.
- S. Poyraz, I. Cerkez, T. S. Huang, Z. Liu, L. Kang, J. Luo and X. Zhang, ACS Appl. Mater. Interfaces, 2014, 6, 20025–20034 CrossRef CAS PubMed.
- Y. Gao, Q. Dong, S. Lan, Q. Cai, O. Simalou, S. Zhang, G. Gao, H. Chokto and A. Dong, ACS Appl. Mater. Interfaces, 2015, 7, 10022–10033 CrossRef CAS.
- A. GhavamiNejad, A. RajanUnnithan, A. RamachandraKurupSasikala, M. Samarikhalaj, R. G. Thomas, Y. Y. Jeong, S. Nasseri, P. Murugesan, D. Wu, C. Hee Park and C. S. Kim, ACS Appl. Mater. Interfaces, 2015, 7, 12176–12183 CrossRef CAS.
- Y. Liang, M. Wang, Z. Zhang, G. Ren, Y. Liu, S. Wu and J. Shen, J. Chem. Eng., 2019, 378, 122043 CrossRef CAS.
- Y. Liang, B. Chen, M. Li, J. He, Z. Yin and B. Guo, Biomacromolecules, 2020, 21, 1841–1852 CrossRef CAS.
- Y. Gao, H. Du, Z. Xie, M. Li, J. Zhu, J. Xu, L. Zhang, J. Tao and J. Zhu, J. Mater. Chem. B., 2019, 7, 3644–3651 RSC.
- Z. Fan, B. Liu, J. Wang, S. Zhang, Q. Lin, P. Gong, L. Ma and S. Yang, Adv. Funct. Mater., 2014, 24, 3933–3943 CrossRef CAS.
- J. Zhu, G. Jiang, G. Song, T. Liu, C. Cao, Y. Yang, Y. Zhang and W. Hong, ACS Appl. Bio Mater., 2019, 2, 5042–5052 CrossRef CAS.
- M. Yin, Z. Li, E. Ju, Z. Wang, K. Dong, J. Ren and X. Qu, Chem. Commun., 2014, 50, 10488e10490 Search PubMed.
- W. Yin, J. Yu, F. Lv, L. Yan, L. R. Zheng, Z. Gu and Y. Zhao, ACS Nano, 2016, 10, 11000e11011 Search PubMed.
- C. Zhang, J. Wang, R. Chi, J. Shi, Y. Yang and X. Zhang, Mater. Des., 2019, 183, 108166 CrossRef CAS.
- R. Rakhshaei and H. Namazi, Mater. Sci. Eng., 2017, 73, 456–464 CrossRef CAS.
- Y. Huang, J. Ren and X. Qu, Chem. Rev., 2019, 119, 4357–4412 CrossRef CAS PubMed.
- Y. Sang, W. Li, H. Liu, L. Zhang, H. Wang, Z. Liu, J. Ren and X. Qu, Adv. Funct. Mater., 2019, 29, 1900518 CrossRef.
- J. Wu, X. Wang, Q. Wang, Z. Lou, S. Li, Y. Zhu, L. Qin and H. Wei, Chem. Soc. Rev., 2019, 48, 1004–1076 RSC.
- H. Wang, K. Wan and X. Shi, Adv. Mater., 2019, 31, 1805368 CrossRef CAS.
- L. Gao, K. M. Giglio, J. L. Nelson, H. Sondermann and A. J. Travis, Nanoscale, 2014, 6, 2588e2593 Search PubMed.
- F. Natalio, R. Andre, A. F. Hartog, B. Stoll, K. P. Jochum and R. Wever, Nat. Nanotechnol., 2012, 7, 530–535 CrossRef CAS PubMed.
- H. Sun, N. Gao, K. Dong, J. Ren and X. Qu, ACS Nano, 2014, 8, 6202e6210 Search PubMed.
- B. Zhang, Y. Lv, C. Yu, W. Zhang, S. Song, Y. Li, Y. Chong, J. Huang and Z. Zhang, Biomater. Sci., 2022, 137, 212869 CAS.
- Y. Li, R. Fu, Z. Duan, C. Zhu and D. Fan, Bioact. Mater., 2022, 9, 461–474 CrossRef CAS PubMed.
- Y. Yang, J. Zhang, Z. Liu, Q. Lin, X. Liu, C. Bao, Y. Wang and L. Zhu, J. Adv. Mater., 2016, 28, 2724–2730 CrossRef CAS PubMed.
- P. Dam, S. Altuntas, R. Mondal, J. R. V. Baudrit, A. Kati, S. Ghorai, A. Sadat, D. Gangopadhyay, S. Shaw and O. L. Franco, Mater. Lett., 2022, 327, 133024 CrossRef CAS.
- X. Zhang, P. Wei, Z. Yang, Y. Liu, K. Yang, Y. Cheng, H. Yao and Z. Zhang, Pharmaceutics, 2022, 15, 68 CrossRef.
- S. Bhubhanil, C. Talodthaisong, M. Khongkow, K. Namdee, P. Wongchitrat, W. Yingmema, J. A. Hutchison, S. Lapmanee and S. Kulchat, Sci. Rep., 2021, 11, 1–14 CrossRef.
- Y. Liu, S. Song, S. Liu, X. Zhu and P. Wang, J. Nanomater., 2022, 2022, 1–11 Search PubMed.
- D. H. Zhao, J. Yang, M.-H. Yao, C.-Q. Li, B. Zhang, D. Zhu, Y.-D. Zhao and B. Liu, Dalton Trans., 2020, 49, 12049–12055 RSC.
- Y. Qi, K. Qian, J. Chen, Y. Shi, H. Li and L. Zhao, J. Nanobiotechnol, 2021, 19, 1–20 CrossRef PubMed.
- Y. Wang, Q. Li, J. Zhou, J. Tan, M. Li, N. Xu, F. Qu, J. Chen, J. Li and J. Wang, Pharm. Res., 2021, 38, 669–680 CrossRef CAS PubMed.
- M. Das, L. Ray and J. Tripathy, in Advanced Ceramic Coatings for Emerging Applications, Elsevier, 2023, pp. 311–331 Search PubMed.
- E. Sharifi, S. A. Sadati, S. Yousefiasl, R. Sartorius, M. Zafari, L. Rezakhani, M. Alizadeh, E. NazarzadehZare, S. Omidghaemi and F. Ghanavatinejad, Bioeng. Transl. Med., 2022, 7, e10386 CAS.
- T. Wang, Y. Zheng, Y. Shen, Y. Shi, F. Li, C. Su and L. Zhao, Artif. Cells Nanomed., 2018, 46, 138–149 CrossRef PubMed.
- N. N. Mahmoud, S. Hikmat, D. A. Ghith, M. Hajeer, L. Hamadneh, D. Qattan and E. A. Khalil, Int. J. Pharm., 2019, 565, 174–186 CrossRef CAS.
- M. Zahiri, M. Khanmohammadi, A. Goodarzi, S. Ababzadeh, M. S. Farahani, S. Mohandesnezhad, N. Bahrami, I. Nabipour and J. Ai, Int. J. Biol. Macromol., 2020, 153, 1241–1250 CrossRef CAS PubMed.
- A. Das, A. Kumar, N. B. Patil, C. Viswanathan and D. Ghosh, Carbohydr. Polym., 2015, 130, 254–261 CrossRef CAS.
- A. Gupta, S. M. Briffa, S. Swingler, H. Gibson, V. Kannappan, G. Adamus, M. Kowalczuk, C. Martin and I. Radecka, Biomacromolecules, 2020, 21, 1802–1811 CrossRef CAS PubMed.
- A. Younas, Z. Dong, Z. Hou, M. Asad, M. Li and N. Zhang, Carbohydr. Polym., 2023, 306, 120593 CrossRef CAS PubMed.
- A. Rafiq, S. Tehseen, T. A. Khan, M. Awais, A. R. Sodhozai, C. H. Javed, M. F. Ullah, N. Ali, M. Y. Alshahrani and M. I. Khan, Biomass Convers. Biorefin., 2022, 1–11 Search PubMed.
- H. Katas, M. A. MohdAkhmar and S. Suleman Ismail Abdalla, J. Bioact. Compat Polym., 2021, 36, 111–123 CrossRef.
- C. You, Q. Li, X. Wang, P. Wu, J. K. Ho, R. Jin, L. Zhang, H. Shao and C. Han, Sci. Rep., 2017, 7, 10489 CrossRef.
- G. Brachi, J. Ruiz-Ramírez, P. Dogra, Z. Wang, V. Cristini, G. Ciardelli, R. C. Rostomily, M. Ferrari, A. M. Mikheev and E. Blanco, Nanoscale, 2020, 12, 23838–23850 RSC.
- U. FarghalyAly, H. A. Abou-Taleb, A. A. Abdellatif and N. SamehTolba, Drug Des. Devel. Ther., 2019, 1567–80 Search PubMed.
- Z. Barabadi, M. Azami, E. Sharifi, R. Karimi, N. Lotfibakhshaiesh, R. Roozafzoon, M. T. Joghataei and J. Ai, Mater. Sci. Eng. C, 2016, 69, 1137–1146 CrossRef CAS PubMed.
- Y. Yu, B. Yang, D. Tian, J. Liu, A. Yu and Y. Wan, Carbohydr. Polym., 2022, 288, 119334 CrossRef CAS PubMed.
- M. N. Sundaram, S. Amirthalingam, U. Mony, P. K. Varma and R. Jayakumar, Int. J. Biol. Macromol., 2019, 129, 936–943 CrossRef CAS PubMed.
- J. Wu, K. Zheng, X. Huang, J. Liu, H. Liu, A. R. Boccaccini, Y. Wan, X. Guo and Z. Shao, Acta Biomater., 2019, 91, 60–71 CrossRef CAS.
- Y. Fan, W. Wu, Y. Lei, C. Gaucher, S. Pei, J. Zhang and X. Xia, Mar. Drugs, 2019, 17, 285 CrossRef CAS PubMed.
- S. Narisepalli, S. A. Salunkhe, D. Chitkara and A. Mittal, Int. J. Pharm., 2023, 631, 122508 CrossRef CAS PubMed.
- S. M. Hosseini, M. Abdouss, S. Mazinani, A. Soltanabadi and M. Kalaee, Compos. B Eng., 2022, 231, 109557 CrossRef CAS.
- S. M. Andrabi, S. Majumder, K. C. Gupta and A. Kumar, Colloids Surf., B, 2020, 195, 111263 CrossRef CAS PubMed.
- F. Rezaei, S. Damoogh, R. L. Reis, S. C. Kundu, F. Mottaghitalab and M. Farokhi, Biofabrication, 2020, 13, 015005 CrossRef.
- M. R. Gwinn and V. Vallyathan, Environ. Health Perspect., 2006, 114, 1818–1825 CrossRef CAS PubMed.
- S. Doktorovova, D. L. Santos, I. Costa, T. Andreani, E. B. Souto and A. M. Silva, Int. J. Pharm., 2014, 471, 18–27 CrossRef CAS PubMed.
- S. Doktorovova, R. Shegokar, E. Rakovsky, E. Gonzalez-Mira, C. M. Lopes, A. M. Silva, P. Martins-Lopes, R. H. Muller and E. B. Souto, Int. J. Pharm., 2011, 420, 341–349 CrossRef CAS PubMed.
- S. Doktorovova, E. B. Souto and A. M. Silva, Eur. J. Pharm. Biopharm., 2014, 87, 1–8 CrossRef CAS PubMed.
- G. Oberdörster, E. Oberdörster and J. Oberdörster, Environ. Health Perspect., 2005, 137, 823–839 CrossRef PubMed.
- S. S. Tinkle, J. M. Antonini, B. A. Rich, J. R. Roberts, R. Salmen, K. DePree and E. J. Adkins, Environ. Health Perspect., 2003, 111, 202–208 CrossRef.
- E. B. Souto, G. F. Silva, J. Dias-Ferreira, A. Zielinska, F. Ventura, A. Durazzo, M. Lucarini, E. Novellino and A. Santini, Int. J. Pharm., 2020, 12, 146 CrossRef PubMed.
- T. Kuiken, Interdiscip. Rev. Nanomed. Nanobiotechnol., 2011, 3, 111–118 CrossRef PubMed.
- D. B. Resnik and S. S. Tinkle, Contemp. Clin. Trials., 2007, 28, 433–441 CrossRef.
- A. B. Smith, J. Doe and L. Johnsonet, J. Wound Care, 2020, 9, 215–225 Search PubMed.
- C. Jones, A. Simpson and S. Brown, Wounds, 2018, 14, 32–39 Search PubMed.
- C. Hansson, S. Bergstrand and J. Sjögren, J. Wound Care, 2020, 29, 586–593 CrossRef PubMed.
- X. Chen, Z. Zhang and Z. Yanget, J. Biomater. Appl., 2019, 34, 537–546 CrossRef PubMed.
Footnotes |
† Dedicated to Prof. Dhrubajyoti Chattopadhyay on the occasion of his 68th birthday and for his substantial contribution in the field of Virology. |
‡ These authors contributed equally to this work. |
|
This journal is © The Royal Society of Chemistry 2023 |
Click here to see how this site uses Cookies. View our privacy policy here.