DOI:
10.1039/D3RA02969G
(Review Article)
RSC Adv., 2023,
13, 21365-21382
Nanoparticle-based drug delivery systems targeting cancer cell surfaces
Received
5th May 2023
, Accepted 11th July 2023
First published on 17th July 2023
Abstract
Traditional cancer chemotherapy easily produces serious toxic and side effects due to the lack of specific selection of tumor cells, which restricts its curative effect. Targeted delivery can increase the concentration of drugs in the target site and reduce their toxic and side effects on normal tissues and cells. Biocompatible and surface-modifiable nanocarriers are novel drug delivery systems, which are used to specifically target tumor sites in a controllable way. One of the effective ways to design effective targeting nanocarriers is to decorate with functional ligands, which can bind to specific receptors overexpressed on the surfaces of cancer cells. Various functional ligands, including transferrin, folic acid, polypeptide and hyaluronic acid, have been widely explored to develop tumor-selective drug delivery systems. This review focuses on the research progress of various receptors overexpressed on the surfaces of cancer cells and different nano-delivery systems of anticancer drugs targeted on the surfaces of cancer cells. We believe that through continuous research and development, actively targeted cancer nano-drugs will make a breakthrough and become an indispensable platform for accurate cancer treatment.
1. Introduction
Malignant tumors are a common public health problem faced by human beings all over the world, which seriously endanger the life, health and quality of life of patients. According to the latest statistics of the World Health Organization (WHO), it is estimated that 19.3 million new cancer cases and 10 million cancer deaths occurred worldwide in 2020.1,2 Traditional cancer treatment methods include surgery, chemotherapy and radiotherapy.3,4 Chemotherapy is the most commonly used cancer treatment strategy and can also be used in combination with other methods. Although these conventional methods have achieved clinical success to some extent, many chemotherapy drugs will lead to serious side effects because of their short half-life and lack of targeting ability.5,6 In addition, tumor cells will also be resistant to drugs, which limits the clinical application of some chemotherapy drugs.7 Therefore, it is an urgent and important problem to explore and develop more effective methods to selectively deliver drugs to tumor sites. With the development of nanotechnology, nano-medicine has shown a good application prospect in improving cancer treatment.8 Compared with individual drug delivery, nano-delivery system can prolong drug half-life to reduce side effects, and improve drug accumulation in tumor through passive or active targeting, which has greater advantages in cancer treatment.8–10 It is crucial to develop effective nano-drug carriers to deliver drugs in nano-delivery system. Nano-drug delivery carrier, as a nano-sized drug carrier system, can improve the safety of chemotherapy drugs used for antitumor and improve the therapeutic effect.7,11 Common nano-drug carriers mainly include polymer nanocarriers, liposomes and inorganic nanocarriers (such as gold nanocarriers, silica nanocarriers and magnetic nanocarriers, etc.)12–15
Compared with normal tissues, tumor tissues have abundant blood vessels, irregular blood vessel wall cells, and nanoparticles are easy to ooze from tumor blood vessels (Fig. 1). In addition, the increase in the number of collagen fibers, fibroblasts, and macrophages in tumor tissue, as well as the dysfunction of the lymphatic drainage system, lead to the inability to effectively remove nanocarriers entering the tumor and remain in the tumor for a long time. This passive phenomenon is called “enhanced permeability and retention (EPR) effect” of tumor.16–19 The exudation strength of nanoparticles depends on the gap size of endothelial cells and the size of cross-endothelial channels.20,21 Generally, the smaller the particles, the easier it is to reach the tumor site through EPR effect.22 However, the nanoparticles should not be too small, otherwise they will be easily removed by the kidney or invaded into capillaries, and at the same time, they should not be too large to escape the phagocytosis of reticuloendothelial system (RES) and the clearance of immune system.23,24 Considering all these factors, the effective nanocarriers should be in the diameter range of 10–150 nm.25 Although EPR effect alleviates the dilemma that chemotherapy drugs are easy to be eliminated in vivo and have no targeting ability, it is difficult to obtain high enough drug concentration in tumor tissue through passive targeting, so the curative effect is not significant.
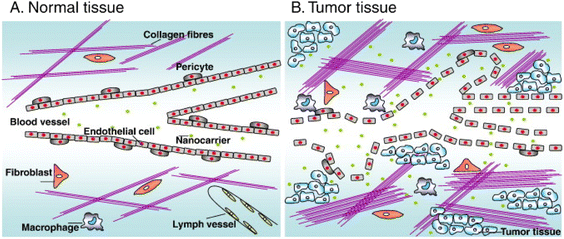 |
| Fig. 1 (A) Normal tissues maintain vascular integrity by peripheral cells and lymphatic vessels exist. (B) The tumor tissue contains defective blood vessels, many cystic structures and perforations, and lymphatic vessels are lacking. Reprinted with permission from ref. 16. Copyright @2010 Elsevier. | |
Due to the defects of passive targeting, it is necessary to explore the active targeting strategy of nanocarriers.26,27 Active targeting strategy mainly depends on the difference of pathophysiology and microenvironment between tumor site and normal tissue. Compared with normal cells, many receptors on the surface of cancer cells or tumor vascular endothelial cells are overexpressed, such as folate receptor (FR), transferrin receptor (TfR), epidermal growth factor receptor (EGFR) and integrin receptor (shown in Table 1).28–30 It is the overexpression of these special receptors that can couple different types of ligands, such as antibodies, peptides, protein, nucleic acids and polysaccharides, to the surface of nanocarriers, so as to increase the specific recognition between the carriers and cancer cells.23 When the nanocarriers bind to the cell surface receptors, they internalize into cancer cells through receptor-mediated endocytosis to maximize drug accumulation.31 The delivery ability of nanoparticles is directly related to the structure and composition of nanoparticles. The main challenge of developing actively targeted nanoparticles is that the required nanoparticles must be able to reach the vicinity of the target and interact with it. Therefore, based on the overexpression of specific receptors on tumor cells, the development of active targeting nano-drugs targeting the surface of cancer cells has attracted great attention of researchers. In this review, some receptors overexpressed on the surface of cancer cells are introduced, and the research progress of anti-cancer drugs delivery through targeted receptors on the surface of cancer cells actively targeting nanomaterials is reviewed.
Table 1 Various receptors overexpressed on different cancer cells along with their targeting moieties
Receptor |
Targeting moieties |
Cancer type |
Transferrin |
Transferrin receptor ligand |
Pancreatic cancer, colon cancer, bladder cancer |
Transferrin |
Folate |
Folic acid |
Ovarian cancer, lung cancer, breast cancer, cervical cancer, renal cancer, brain cancer |
EGFR |
Anti-EGFR |
Colon cancer, breast cancer, head, neck cancer, ovarian cancer |
CD 44 |
Hyaluronic acid |
Liver cancer, breast cancer, colon cancer, lymphoma |
Integrin |
RGD peptide |
Glioma, melanoma, lung cancer, breast cancer |
2. Cell membrane surface receptor
2.1. Transferrin receptor
Transferrin (Tf) is a transmembrane glycoprotein, which is used to transport and regulate the distribution of iron in human cells.32,33 It binds to transferrin receptor (TfR) and mediates the intracellular transport of iron, which is an important way for cells to obtain iron. Usually, Tf combines with iron in serum to transport iron to liver and other cells, and then combines with TfR on the cell surface to form Tf–TfR complex, which is then absorbed into cells through internalization.34 Due to iron being an essential protein cofactor and participating in basic life processes such as cell proliferation and growth, many tumor cells have stable and highly expressed TfR on their surface.35 The expression of transferrin receptor in common tumors such as pancreatic cancer, colon cancer and bladder cancer are up-regulated, which is higher than that in normal cells.33 Moreover, the expression of TfR is related to the malignant level of tumor, and TfR is more expressed in breast cancer, glioma, lung adenocarcinoma, chronic lymphocytic leukemia and liver cancer with high malignant degree and easy metastasis.36 This has attracted the attention of researchers, making it an active targeting strategy to deliver drugs to tumor cells. TfR can be used either to target the delivery of drugs into cancerous cells or to prevent the normal function of the receptor leading to cancer cell death. Up to now, transferrin receptor has become the most widely studied cell target in anticancer.
2.2. Folate receptor
Folic acid (FA) also known as vitamin B9, plays an essential role in DNA synthesis and replication, cell division, growth and survival.37 Folic acid is usually combined with folate receptor (FR) and transported across the membrane through endocytosis. As shown in Fig. 2, after FA binds to FR, it forms vesicles and enters cells through endocytosis.38 After the fusion of intracellular vesicles and lysosomes, acidification leads to the release of FA into cells, and FR is transferred to the surface of cell membrane again.39,40 FR, also known as folic acid binding protein, is a glycosyl phosphatidylinositol ankyrin with four subtypes: FRα, FRβ, FRγ and FRδ.41 FRα, FRβ and FRδ are membrane proteins fixed by glycosyl phosphatidylinositol,42 while FRγ is a secretory protein of lymphoid cells.43 Among them, FRα has a high affinity for FA.44 The expression level of FRα in normal tissues is very low,45 and most of FRα is expressed at the apical (luminal) surface of epithelial cells, which will not directly interact with FA or molecules targeted by FR in the circulatory system.46,47 However, FR is highly expressed in some epithelial tumors, including ovarian cancer, lung cancer, breast cancer, endometrial cancer, cervical cancer, renal cancer, bladder cancer and brain cancer,48 in order to meet high folate demand of rapidly dividing cells.49 Cancer cells can make FRα approach FA by changing its transmembrane region, transcription level and translation level,50 and can also promote the growth and proliferation of tumor cells through signal transduction and transcription factors.45 Therefore, FRα has become an attractive therapeutic receptor for specific drug delivery.
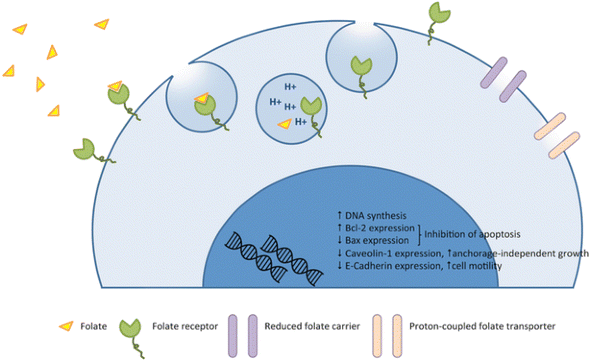 |
| Fig. 2 Mechanism of action of folate and the folate receptor against the tumor. Reprinted with permission from ref. 45. Copyright @2015 Spring Nature. | |
2.3. Epidermal growth factor receptor
Epidermal growth factor receptor (EGFR), also called HER1 (human epidermal receptor 1) or ErbB1 (receptor tyrosine-protein kinase erbB-1), is a transmembrane glycoprotein belonging to tyrosine kinase receptor family.51 The EGFR family also includes three other family members with similar structures: HER2, HER3 and HER4, all of which contain the extracellular ligand-binding domain, the intracellular tyrosine kinase domain (except HER3) and the intermediate transmembrane structure.52 EGFR is distributed on various cell surfaces and plays an important role in cell proliferation, differentiation and other life activities.53 When endogenous ligands such as epidermal growth factor (EGF) bind to EGFR, kinases will dimerize,54 γ-phosphate will be transferred from adenosine triphosphate (ATP) to kinase domain,55 and phosphorylated EGFR can activate downstream signal pathways (such as MAPK and P13K pathways),51 thus regulating cell proliferation and differentiation and other life activities. Overexpression or mutation of EGFR family will lead to kinase dysfunction, which will lead to abnormal cell life activities and eventually tumor. Therefore, EGFR is a key participant in the development of many cancers (such as colon cancer, non-small cell lung cancer, breast cancer, head and neck cancer and ovarian cancer)56 and has become an important drug target.57 At present, targeted drugs for EGFR mainly include small tyrosine kinase inhibitors (TKIs) and monoclonal antibodies (mAb), which have been developed and successfully used in clinic.52 The mechanism of tyrosine kinase inhibitors and anti-EGFR monoclonal antibodies in cancer cells is shown in Fig. 3. TKIs inhibits tyrosine kinase phosphorylation by interacting with intracellular domains, thus inhibiting the activation of EGFR signaling pathway.58 Common TKIs include the first generation gefitinib, erlotinib, the second generation afatinib, dacomitinib and the third generation osimertinib.59 However, mAb specifically binds to the extracellular domain of EGFR, blocking the binding of ligands, thus cutting off the EGFR signaling pathway.60 Cetuximab (Erbitux) and panitumumab (Vectibix) are both monoclonal antibodies approved by FDA.61 Therefore, EGFR is a mature and attractive receptor for targeted therapy of specific drugs on the surface of cancer cells.
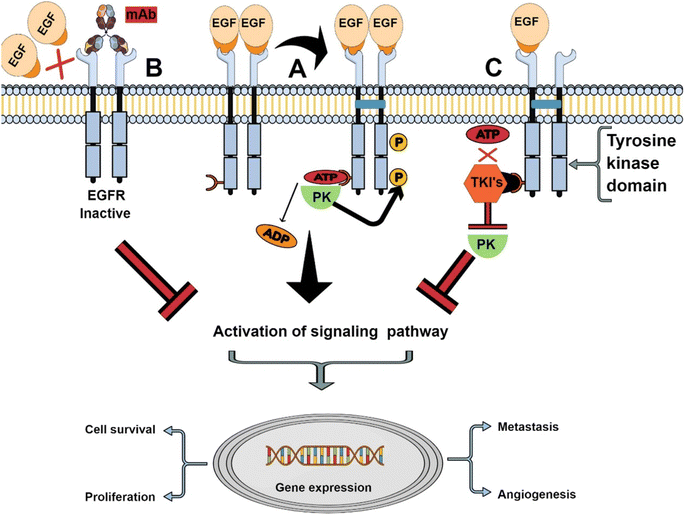 |
| Fig. 3 The mechanism of tyrosine kinase inhibitors and anti-EGFR monoclonal antibodies. Reprinted with permission from ref. 61. Copyright @2021 Elsevier. | |
2.4. Cluster of differentiation 44
Cluster of differentiation 44 (CD44) is a single-chain transmembrane adhesion glycoprotein, which is involved in many signal pathways in physiological and pathological processes, especially the occurrence, progress and metastasis of tumors.62 CD44 is overexpressed on the surface of many tumors, including liver cancer, breast cancer, colon cancer, lymphoma, etc.63 It is considered as a marker of many cancer stem cells (CSCs),64 so it can be a cell surface target for active drug targeting and treatment. CD44 can interact with various cytokines, growth factors and extracellular matrix (ECM) secreted by cells in tumor microenvironment.65 The common ligands of CD44 include hyaluronic acid (HA), osteopontin (OPN), chondroitin and so on.66 Among them, HA is the main component of extracellular matrix,67 and its binding with CD44 is cell-specific. In addition, HA is a natural polymer which is easy to biodegrade and has good biocompatibility. Usually, HA-modified nanocarriers are used to specifically bind CD44, and at the same time, the binding between endogenous HA and CD44 is disturbed, which weakens the intracellular signaling pathway and eventually leads to cell apoptosis.68
2.5. Integrin receptor
Integrin is a transmembrane glycoprotein receptor, which is expressed on various cell surfaces. It is involved in a variety of cell signal transduction pathways and is closely related to the proliferation, invasion and migration of cancer cells.69 Integrin is composed of an α subunit and a β subunit through non-covalent bond to form a heterodimer structure.70 Up to now, it has been found that there are 18 α subunits and 8 β subunits, which together form 24 integrin receptors.71 Each integrin can recognize and bind to ECM ligands, cell surface ligands or soluble protein ligands, thus widely regulating cell functions.72 Arginine–glycine–aspartic acid (RGD) sequence in ECM is a common binding motif of integrins, and it has the strongest binding ability with ανβ3 and ανβ5 integrins that are not expressed in normal tissues.73 ανβ3 integrin is overexpressed in tumor blood vessels and can specifically recognize RGD on ECM. Therefore, integrin has become one of the important targets of drug development.
3. Nanoparticles targeting cancer cells
Next, some nanoparticle-based targeted formulations that have shown positive results in recent studies are presented (Table 2).
Table 2 Some formulations of nanoparticles with positive results in the recent investigations
Nanoparticle |
Anticancer drug |
Targeting agent |
Reference |
Pluronic P123 |
Paclitaxel (PTX) |
Transferrin |
74 |
PEG |
4-Methylumbelliferone |
Folic acid, HA |
75 |
DSPE–PEG |
Doxorubicin (DOX) |
RGD peptide |
76 |
DSPE–PEG |
Doxycycline, docetaxel |
Folate receptor beta (FRβ) |
77 |
Poly(amidoamine) dendrimers |
Doxorubicin (DOX) |
EGFR-binding peptide 1 |
78 |
Gold nanorods |
Paclitaxel (PTX), curcumin (CUR) |
cRGD peptide |
79 |
Gold nanoparticle |
p53DNA |
Antibody EGFR (C225) |
80 |
Gold-polyvinylpyrrolidone |
Curcumin (CUR) |
Folic acid |
81 |
Chitosan-coated mesoporous silica |
Gemcitabine |
Transferrin |
82 |
Chitosan-coated mesoporous silica |
Doxorubicin (DOX) |
EGFR/HER2 aptamer |
83 |
Superparamagnetic iron oxides (SPIO) |
Doxorubicin (DOX) |
Folic acid |
84 |
3.1. Polymer delivery system
In the past few decades, polymer delivery system has been widely explored as a drug delivery system for biomedical applications. Polymer micelles synthesized from the polymer materials have significant applications in cancer diagnosis and treatment because of its unique advantages. Polymer micelles is usually a “core–shell” structure formed by self-assembly of amphiphilic block copolymer in aqueous solution. Its hydrophobic core can carry hydrophobic drugs, thus improving the solubility and stability of drugs; hydrophilic shell forms a protective barrier by hydration, which reduces the absorption of protein and the recognition and clearance of reticuloendothelial system (RES) during blood circulation, and prolongs the half-life of drugs.85 The hydrophilic shell of polymer is easy to be chemically modified to achieve active targeting. In particular, polymer-based nano micelles such as polyethylene glycol (PEG), polylactic acid (PLGA) and chitosan (CS) modified by cancer cell surface-related ligands have been designed to treat breast cancer, glioma, prostate cancer, lung cancer and so on.
Ge et al. co-encapsulated paclitaxel (PTX) and photosensitizer (5-ALA) into nanoparticles (Tf-5-AlA-PTX-NCs) with magnetic carrier modified by Tf-coupled copolymer Pluronic P123, as a new strategy of combined chemotherapy and photodynamic therapy.74 They proved that Tf-5-ALA-PTX-NCs modified with transferrin ligand showed stronger fluorescence signal in MCF-7 cell xenotransplantation tumor compared with 5-ALA-PTX-NCs treatment, thus enhancing the antitumor effect. Hu et al. developed a hyaluronic acid–cysteamine–polylactic acid–glycolic acid (HA–SS–PLGA) polymer nano-delivery system to deliver doxorubicin (DOX) and cyclopamine to breast cancer cells with CD44 overexpression.67 They found that the intake of PLGA–DOX and HA–SS–PLGA–DOX into MCF-7 cells was similar. However, HA–SS–PLGA–DOX particles are easier to enter MDA-MB-231 cells with CD44+ overexpression than PLGA–DOX, and MDA-MB-231 is more cytotoxic to MDA-MB-231. They proved that the modification of HA can enhance the targeting ability of nano-drug delivery system to CD44 overexpressed cancer cells. Pan et al. constructed two polymer micelles based on pentaerythritol polycaprolactone-b-poly(N-isopropylacrylamide) and pentaerythritol polycaprolactone-b-poly(N-vinylcaprolactam) to deliver DOX for targeted treatment of glioma.86 The cellular uptake study showed that FA receptor promoted the accumulation of folate-coupled polymer micelles in C6 glioma cell lines. In addition, compared with normal cells (HaCaT), the nano-system has a selective killing effect on cancer cells, thus reducing the side effects of drug-loaded carriers. Recently, Ling et al. used DSPE–PEG5K–COOH to wrap NIR-II dye SQ890 to form nanoparticles, and modified EGFR targeting peptide (GE890) on its surface, which can accurately target to the tumor site, so as to realize photoacoustic (FA)/NIR-II fluorescence dual-mode imaging to guide PTT to treat oral cancer (Fig. 4A).87 As shown in Fig. 4B, compared with L-02 cells with low EGFR expression, the nanoparticles modified by targeted peptides have more uptake in CAL 27 cells with high EGFR expression. Finally, SQ890 NPs-Pep showed better imaging ability of NIR PA/NIR-II fluorescence imaging and higher PTT effect in mice (Fig. 4C and D). All these results reveal the EGFR targeting effect of cetuximab against immune micelles. Amit S. Yadav et al. developed an RGD peptide functionalized chitosan nano-delivery system (RGD-CHNPs) to deliver raloxifene (Rlx).88 Compared with non-targeting CHNPs, RGD-CHNPs showed higher tumor accumulation. Critically, Rlx–RGD-CHNPs have no toxicity to healthy tissues, but show significant antitumor effect on breast tumors expressing αvβ3 integrin due to the active targeting of RGD peptide. Marwa Labib Essa et al. prepared CD44 and folate receptor dual-targeted nanoparticles (4-MU@FA–PEG–HA NPs) based on folic acid–polyethylene glycol–hyaluronic (FA–PEG–HA) to improve the therapeutic efficacy of 4-methylumbelliferone (4-MU) on lung cancer.75 The cytotoxicity analysis showed that the IC50 of 4-MU was reduced from 224.5 to 64.75 μg ml−1 in the case of 4-MU@FA–PEG–HA NPs.
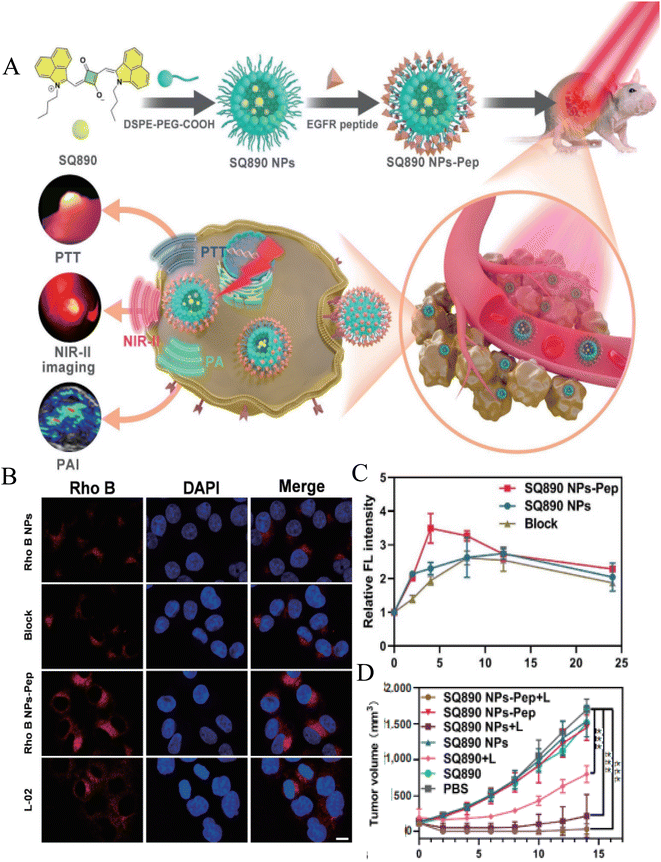 |
| Fig. 4 (A) Schematic illustration of the EGFR-targeting nanoparticle SQ90 NPs-Pep for photoacoustic NIR-II fluorescence dual-modality imaging-guided PTT of oral cancer; (B) cellular uptake (CAL 27 and L-02 cells) of Rho B-labeled NPs observed by CSLM; (C) semiquantitative analysis of relative fluorescent intensity at different time points after various treatments; (D) tumor volume growth of mice with CAL 27 tumors in each group. Reprinted with permission from ref. 87. Copyright @2022 Springer Nature. | |
Due to their good biocompatibility and biodegradability, many polymer materials have been used to develop nano-drug delivery systems for targeted delivery of cancer cells. However, there are still some problems in its clinical application and transformation potential, such as poor micelle stability and premature drug release. It is still urgent to develop a stable, multifunctional and on-demand intelligent polymer delivery system.
3.2. Liposome delivery system
Liposome delivery systems are the most commonly used research nanocarriers for tumor targeted drug delivery. In the 1960s, Bangham first reported the structure with liposomes.89 Liposomes are tiny vesicles composed of natural phospholipids and cholesterol, including a phospholipid bilayer and a hydrophilic inner compartment. Generally, hydrophilic drugs are wrapped in hydrophilic inner compartments, while hydrophobic drugs are embedded in hydrophobic double-layer membranes.90 This unique encapsulation ability makes it have the advantages of good biocompatibility, high drug loading rate, no immunogenicity and easy surface modification.76 In 1995, PEGylated liposome (Doxil) coated with anticancer drug DOX was approved by FDA for clinical cancer treatment, and became the first nano-drug on the market.91 Since then, many liposome-based nano-drugs have been researched and developed, and many liposome drugs such as DepoCyt, DepoDur, Exparel, Marqibo and DaunoXome have been approved for clinical research.92,93 Liposome is the most successful nano-drug delivery carrier in clinical application so far. PEG modification can endow liposomes with “stealth” function and prolong the circulation time in vivo.94 However, only relying on the EPR effect of liposomes, the enrichment rate of drugs in tumor sites is still low. In order to achieve selective delivery, many drugs with different structures can be modified on the surface of liposomes, such as some targeting ligands, to target the specific receptors of tumor cells, thus improving the therapeutic effect.
Arabi et al. functionalized Doxil with CD44 monoclonal antibody (mAb) and compared its antitumor activity with Doxil.95 In vitro results showed that the uptake of CD44-targeting (mAb)-modified Doxi in CD44 mouse colon cancer cells was significantly improved compared with Doxil (Fig. 5). Furthermore, CD44-Doxil exhibited higher doxorubicin concentrations within tumor cells as well as excellent antitumor efficacy and improved therapeutic effect compared with Doxil-treated C-26 colon cancer mice. Amin et al. designed liposomes containing doxorubicin modified with two ligands: RGD and type 1 transactivator of transcription (TAT) peptides.76 They found that the presence of two active ligands together increased the targeting area and improved the binding of liposomes to cells, showing enhanced therapeutic effect on the B16 tumor model. Yong Il Park et al. constructed a FRβ-targeted pH-sensitive liposome (FRβ-pH lipo) to deliver doxycycline and docetaxel to better inhibit tumor growth in non-small cell lung cancer (NSCLC).77 It was observed by laser confocal that in FRβ overexpressed A549 cells, the fluorescence signal of folate-modified FRβ-pH lipo-Cy5.5 was 7.23 times stronger than that of NH2-pH lipo-Cy5.5. Song et al. prepared biodegradable liposome nanoparticles (LPN) coupled with epidermal growth factor receptor to co-deliver docetaxel (DTX) and resveratrol (RSV) for the treatment of advanced non-small cell lung cancer.96 Sonali et al. studied a therapeutic diagnostic liposome conjugated with transferrin (DTX-QD-TPGS-Tf) for co-delivery of docetaxel (DTX) and quantum dots (QD) to brain cancer cells.97 Compared with Docel, the liposome modified by transferrin receptor shows significantly higher delivery of DTX and QD to brain cancer sites. After 4 hours, the drug accumulation efficiency of targeted liposomes was 10.56 times that of Docel.
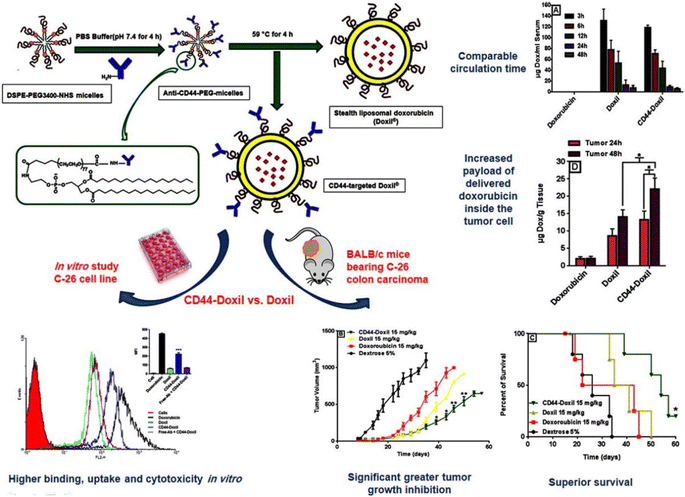 |
| Fig. 5 Preparation method of CD44-Doxil and therapeutic effect in vivo and in vitro. Reprinted with permission from ref. 95. Copyright @2015 Elsevier. | |
Generally, the structure of liposome endows it with unique characteristics and functions, making liposome an ideal carrier for various therapeutic drugs and clinical applications. Liposomes modified by targeting ligands increase the target accumulation of drugs and greatly reduce the off-target toxicity of various drugs. However, the complexity of functionalized liposomes increases, which will hinder the transfer to large-scale production and clinic. Therefore, the development of functional liposomes from the bench to the bedside is still the focus of researchers.
3.3. Dendrimer nanocarriers
Dendrimers are highly branched macromolecules assembled from monomeric structures through covalent bonds. Its 3D branched structure with multiple functional groups on the surface makes it multifunctional and biocompatible.98 Due to its good water solubility, biodegradability, low polydispersity, controllable molecular size, and high adaptability of surface chemistry, it has become an excellent carrier candidate for biological and drug delivery systems.99 Among various nanocarriers, dendrimers have received great attention from researchers. Therefore, dendrimers can be used as a very promising drug delivery system, which is expected to achieve drug targeting to specific cells. Gong et al. designed a hydrophilic dendritic copolymer as a platinum-based drug nanocarrier targeting ovarian cancer, composed of poly(amidoamine)-b-poly(aspartic acid)-b-poly(ethylene glycol) conjugated to cRGD peptide and anthocyanin 5 (Cy5) fluorescent dye.100 Cell uptake experiments demonstrated that cRGD conjugation can effectively enhance the uptake of nanoparticles in cancer cells through integrin-mediated endocytosis. Importantly, carboplatin-complexed cRGD-conjugated nanoparticles were more cytotoxic to ovarian cancer cells than non-targeting nanoparticles.
Poly(amidoamine) (PAMAM) dendrimers have high-density amino groups on the surface, which can be easily modified and functionalized by chemical coupling with various functional molecules.101 PAMAM dendrimers have been developed to the fifth generation (G5) at present, and are uniform spherical shapes with small size.102 Hong et al. synthesized acetamide-terminated G5 dendrimers (G5-Ac-AF488-FAx) functionalized with 2–14 folic acid molecules and Alexa Fluor 488 dye (AF488).103 They found that the binding affinity of G5-Ac-AF488-FAx with folate-binding protein (FBP) was ∼2500 to ∼170
000-fold stronger than free FA and increased the residence time of the nanocomplexes on cells. Wang et al. covalently coupled fluorescein isothiocyanate (FI) and FA to G5 PAMAM dendrimers (G5.NH2) with acetyl terminal groups, and the anticancer drug 2-methoxyestradiol (2-ME) targeted delivery to cancer cells overexpressing FAR.102 The G5.NHAc–FI–FA/2-ME complex could specifically target human epithelial carcinoma (KB) cells with high levels of FAR and showed more toxicity. Liu et al. established a bifunctional drug delivery system based on PAMAM dendrimers coupled with EGFR-binding peptide 1 (EBP-1) and cell-penetrating peptide trans-activating transcriptional activator (TAT), using for encapsulating doxorubicin (DOX) (Fig. 6).78 This bifunctional dendritic polymer drug carrier significantly improves the drug accumulation at the tumor site, improves the inhibitory effect of DOX on human breast cancer, and reduces the systemic toxicity of DOX in vivo.
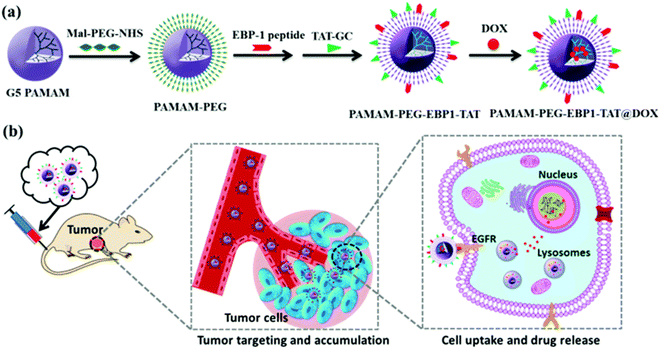 |
| Fig. 6 (a) Construction of the dual-functional dendrimer-based drug carrier and (b) schematic illustration of tumor targeting therapy using the dual-functional drug delivery system. Reprinted with permission from ref. 78. Copyright @2019 The Royal Society of Chemistry. | |
In a word, targeted nano-delivery systems based on dendrimers have been widely studied because of their promising characteristics. However, due to the complexity of multi-component 3D structure, careful design is needed to ensure repeatable formula in mass manufacturing process.
3.4. Inorganic nanocarriers for drug delivery
Inorganic delivery systems are widely used in biomedicine and other fields because of their diverse structures and functions, such as adjustable size, shape, high specific surface area, unique optics and magnetism, and easy surface modification. The most important thing is that it is easy to modify, and different ligands can be used to functionalize nanocarriers, thus improving their attractiveness to targets. Therefore, inorganic nanocarriers are excellent prospects in targeted drug delivery, imaging diagnosis and drug synergistic therapy. The main inorganic nanocarriers include: gold nanocarriers, mesoporous silica nanocarriers, magnetic nanocarriers, etc.
3.4.1. Gold nanocarriers. Gold nanocarriers are widely used in the biological field due to their unique size, controlled release of drugs, and lower toxic side effects than other metals. The surface of gold nanocarriers is easy to modify, and some organic compounds (e.g., DNA and peptides) can be functionalized through Au–S bonds or Au–thiol bonds, which greatly improves its bioavailability,104 making it useful in tumor imaging, diagnosis and treatment.Kim et al. synthesized a radioactive iodine-labeled, cyclic RGD-PEGylated gold nanoparticle probes (125I-cRGD-AuNP) for tumor site imaging.104 Experiments showed that the binding affinity of 125I-cRGD-AuNP probes to αvβ3 integrin was 150-fold higher than that of unmodified free cRGD. In vivo single-photon emission computed tomography (SPECT/CT) imaging results showed that the 125I-cRGD-AuNP probes could rapidly and efficiently target αvβ3 integrin overexpressing tumor sites within 10 minutes after injection. Due to the unique optical properties of gold, functionalized gold nanocarriers are not only used for in vivo imaging, but also used in the targeted therapy of tumors. Zhu et al. utilized gold nanorods, cRGD peptide, paclitaxel (PTX), and curcumin (CUR) to construct tumor-targeting and multi-stimuli-responsive nanocarriers (PTX/CUR/Au NRs@cRGD) for chemo-photothermal synergistic therapy.79 Through the analysis of in vivo behavior, DiR/PEG/Au NRs did not observe fluorescence signal in A549 tumor, while DiR/PEG/Au NRs@cRGD showed obvious accumulation of fluorescence signal in the tumor site, and presented an ideal tumor ablation effect. Rajesh Kotcherlakota et al. designed a cationic gold nanoparticle functionalized with monoclonal antibody EGFR (C225) and wild type p53 plasmid DNA (p53DNA) for targeted gene delivery to ovarian cancer (Fig. 7A).80 The intracellular uptake of Au-C225-p53DNA was observed in SKOV-3 cells (human ovarian cancer) and CHO cells (china hamster ovary), respectively. As shown in Fig. 7B, the fluorescence intensity of Au-C225-p53DNA in EGFR overexpressing SKOV-3 cells was much more obvious than that in CHO cells (Fig. 7B). In nude mice bearing SKOV-3 xenotransplantation model, Au-C225-p53DNA treatment group showed effective tumor targeting and significant tumor regression. Sneha Mahalunkar et al. designed gold–polyvinylpyrrolidone nanoparticles (FA–CurAu–PVP NPs) modified with folic acid and curcumin for targeted delivery in a breast cancer model system.81 In vitro experiments demonstrated that folic acid-modified FA–CurAu–PVP NPs had a higher potential in inhibiting the proliferation and migration of breast cancer cells. FA–CurAu–PVP had higher anticancer activity in triple-negative breast cancer cells with higher folate receptor expression than other breast cancer cells. Liu et al. employed CD44-specific targeting of hyaluronic acid (HA) and cationic bovine serum albumin-protected gold nanoclusters to construct a size-reducible nanoplatform (AuNC@CBSA@HA).105 AuNC@CBSA@HA was further loaded with paclitaxel (PTX) and indocyanine green (ICG) for chemical photothermal therapy. Among them, HA not only endows nanoparticles with active targeting properties, but also degrades at the tumor site to reduce the size of nanoparticles and enhance the penetration depth of drugs in tumors. The Au nanoclusters finally inhibited 95.3% of orthotopic tumor growth and 88.4% of lung metastatic growth in 4T1 tumor-bearing mice.
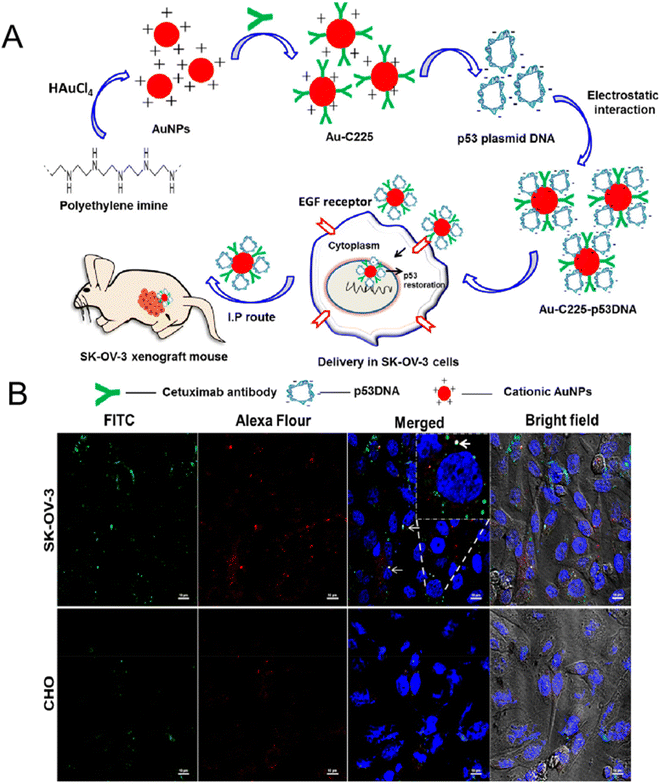 |
| Fig. 7 (A) Preparation and mechanism diagram of Au-C225-p53DNA; (B) confocal uptake of Au-C225-p53DNA in SK-OV-3 cells (top) and CHO cells (bottom). Reprinted with permission from ref. 80. Copyright @2019 American Chemical Society. | |
In order to develop more effective targeting gold nanocarriers, some researchers consider the introduction of multiple targeting molecules at the same time to enhance the tumor targeting ability and therapeutic effect of gold nanocarriers. Xu et al. functionalized HA through Au–S bonds to gold nanorods (GNR), and then coupled 5-aminolevulinic acid (ALA), Cy7.5 and anti-HER2 antibody onto HA moiety for photodynamic therapy (PDT), fluorescence imaging and active targeting, respectively (Fig. 8).106 Experiments showed that the dual targeting strategy of HER2 and CD44 could significantly enhance the endocytosis of GNR–HA–ALA/Cy7.5-HER2 in human breast cancer cell MCF-7. In vivo studies showed that tumors in mice were completely eliminated without significant side effects.
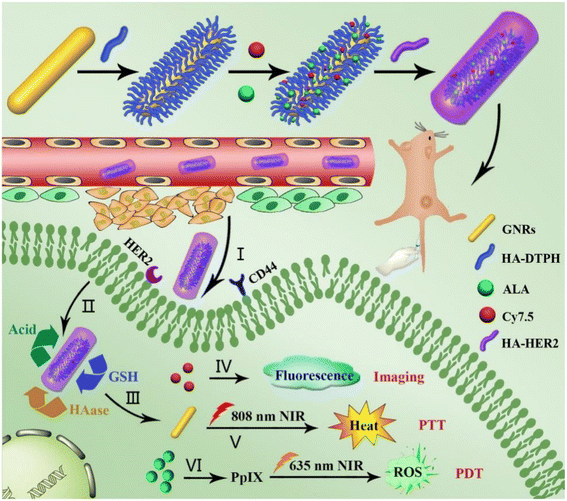 |
| Fig. 8 Schematic diagram of the preparation of GNR–HA–ALA/Cy7.5-HER2, and its application in HER2/CD44 dual-targeting and fluorescence imaging-guided PDT/PTT combination therapy for breast cancer. Reprinted with permission from ref. 106. Copyright @2019 Elsevier. | |
3.4.2. Silica nanocarriers. Silica nanocarriers mainly include silica nanoparticles, mesoporous silica (MSN), hollow mesoporous silica, and silica nanotubes. Among them, MSNs are porous structures with high drug loading capacity due to large specific surface area and high pore volume.107 In addition, the surface of MSNs can be easily modified with functional groups such as amino groups and sulfhydryl groups to further modify targeting molecules, making it a multifunctional and efficient delivery platform for various anticancer drugs. An ideal MSN drug-loading system not only accurately target the tumor site, but also have no drug leakage during blood delivery, and be able to control the release of local high-concentration drugs at the tumor site. Therefore, the surface of MSNs can be modified with gatekeepers that can respond to certain external stimuli (such as pH, oxygen, enzymes, redox reagents, and temperature, etc.) to achieve controlled and on-demand drug release.108Due to the acidic environment of tumors, which is different from the physiological pH value of 7.4,108 this difference can enable the specific release of encapsulated drugs into tumor tissues, and pH-responsive MSNs are becoming more and more popular. Cheng et al. coated pH-sensitive polydopamine (PDA) on the surface of mesoporous silica nanoparticles (MSNs), and then introduced FR-targeting molecule PEG–FA to load doxorubicin (DOX) to form drug delivery system (MSNs–DOX@PDA–PEG–FA, Fig. 9).109 In vitro drug release experiments showed that the release of DOX was pH-dependent, reducing the toxic and side effects on normal tissues. They also observed that MSNs–DOX@PDA–PEG–FA had significantly higher targeting efficiency and antitumor efficacy compared with free DOX and DOX-loaded NPs without FA-targeting ligands. Saini and Bandyopadhyaya designed transferrin (Tf)-conjugated, chitosan-coated mesoporous silica nanoparticles (MSNs) loaded with the anticancer drug gemcitabine.82 They demonstrated that the nanoparticles release the drug at a very low rate in a neutral environment, but in a large amount in the tumor environment. The Tf-bound MSNs had better uptake on MIA PaCa-2 cells due to Tf–TfR interaction. Most importantly, the modification of Tf increased its killing efficiency against cancer cells to 75%. Lohiya and Katti developed EGFR/HER2 aptamer-conjugated, DOX-loaded, chitosan-coated mesoporous silica nanoparticles (MSNs) for active targeting of EGFR- and HER2-overexpressing breast cancer cells.83 The developed targeted MSNs exhibited higher uptake and cytotoxicity against triple-negative and HER2-positive breast cancer cells compared to non-targeted MSNs. Chitosan coating endows MSNs with pH responsiveness and endo/lysosomal escape ability, resulting in precise control of drug release.
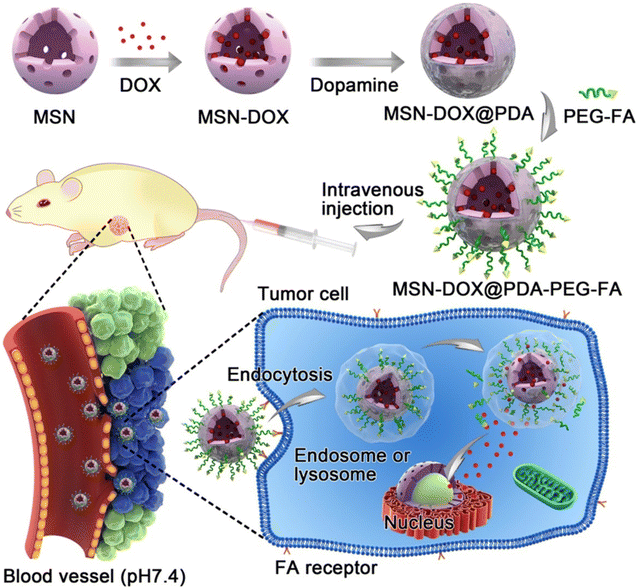 |
| Fig. 9 Schematic illustration of DOX-loaded MSN–DOX@PDA–PEG–FA. Reprinted with permission from ref. 109. Copyright @2017 American Chemical Society. | |
Glutathione (GSH) is a reducing agent in organisms, and the concentration of GSH in tumor cells is about 100–1000 times higher than that in normal tissues.110 This marked difference in GSH levels allows GSH to be used as a selective intracellular stimulant for the development of drug delivery systems that can be degraded by GSH. Disulfide bonds (SS) can maintain stability under normal physiological conditions, blocking the contact of encapsulated drugs with the external environment, but can be reduced by GSH to cause bond breaks and drug release.111 Based on this, many researchers have introduced disulfide bonds into nanocarriers to prepare drug-controlled release systems with reduction-sensitive stimuli responsiveness. Zhao et al. developed a redox/enzyme dual-stimuli-responsive targeted delivery system.112 They grafted HA onto the surface of MSN through a disulfide bond, which not only acts as a gatekeeper, but also can actively target the CD44 receptor on cancer cells. They demonstrated that DOX release was accelerated in the presence of glutathione (GSH) and hyaluronidase (HAase) (Fig. 10). MSN-SS-HA exhibited higher uptake efficiency in HCT-116 cells through CD44 receptor-mediated endocytosis. Venkatesan et al. covalently linked the mesoporous shell of mesoporous silica nanoparticles (MSNP) to cancer-targeting Tf via disulfide bonds for GSH-controlled DOX drug release in tumor cells.113 They demonstrated that Tf on the surface of MSN selectively recognizes TfR on the surface of cancer cells, enabling MSNP-SS-Tf@PEG to be internalized into HT-29 and MCF-7 cells. Then, DOX is released into tumor cells triggered by high GSH concentration.
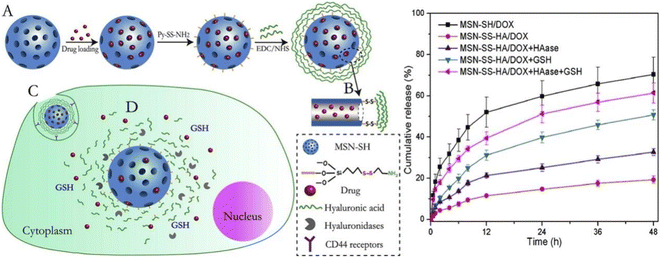 |
| Fig. 10 Schematic structure of MSN-SS-HA/DOX drug delivery and cumulative release profiles of MSN-SS-HA/DOX and MSN-SH/DOX in pH 5.0 PBS under different conditions. Reprinted with permission from ref. 112. Copyright @2015 Elsevier. | |
3.4.3. Magnetic nanocarriers. Magnetic nanoparticles (MNPs) refer to magnetic materials with particle sizes ranging from 0 to 100 nm. Magnetic nanoparticles include hematite, maghemite, nano ferrite, and magnetite.114 In particular, iron and iron oxide particles, such as magnetite and its oxidized form maghemite, have unique magnetic properties and biocompatibility, which have been widely used in the fields of drug delivery, tumor hyperthermia, and magnetic resonance imaging (MRI), etc. However, due to poor water solubility and easy agglomeration of naked MNPs, plasma proteins are easily adsorbed on the surface and cleared by RES, which poses a certain obstacle to the field of biomedicine. In order to prepare well-dispersed water-soluble MNPs, surface modification and functional group modification are required.The polymer coating adds a protective film to the MNPs, preventing them from being cleared out of the body by the RES due to aggregation. Huang et al. designed a nano-delivery system (FA-SPIONs) based on superparamagnetic iron oxides (SPIO) coated with FA-modified polymers (PEI and PEG).84 DOX was loaded onto FA-SPIONs through electrostatic adsorption and hydrogen bonding (DOX@FA-SPIONs) (Fig. 11). They found that DOX@FA-SPIONs were actively targeted to MCF-7 cells by FA receptor-mediated endocytosis. DOX@FA-SPIONs achieved MRI monitoring and more effectively inhibited the growth of MCF-7 cells and their xenograft tumors under the action of a magnetic field. Wang et al. functionalized the surface of PEGylated SPIO nanoparticles with an anti-EGFR monoclonal antibody (cetuximab) for targeted delivery to EGFR-overexpressing lung cancers.115 Compared with non-targeting PEGylated SPIO NPs, anti-EGFR-PEG-SPIO NPs exhibited better targeting ability to H460 tumor cells and enhanced targeted tissue thermogenesis by magnetic resonance-guided focused ultrasound surgery (MRgFUS) therapy. Ghadiri et al. developed a dextran spermine-coated iron oxide nanoparticles coupled with Tf to obtain a targeted drug delivery system (TDS-NPs) to specifically target the brain and deliver capecitabine across the blood–brain barrier (BBB).116 In vivo biodistribution and histological studies showed a significant increase in iron concentrations in the brain transported by targeted nanoparticles.
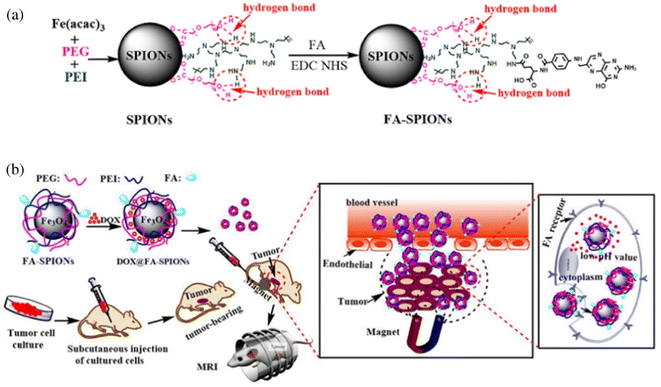 |
| Fig. 11 (a) The synthesis and surface coating of SPIONs and FA-SPIONs. (b) The DOX loaded FA-SPIONs for FA-mediated and magnetically targeted drug delivery to the tumor and the MR imaging. Reprinted with permission from ref. 84. Copyright @2017 Elsevier. | |
Coating silicon dioxide on the surface of magnetic nanoparticles can obtain magnetic nanocarriers with a core–shell structure. The silica shell can protect MNPs from chemical degradation in the body, avoid the leakage of harmful substances in the nucleus, and reduce the toxic and side effects on normal tissues.117 Tabasi et al. developed CD44-modified superparamagnetic Fe3O4/mesoporous silica nanocarriers (NCs-OXA) for the delivery of oxaliplatin (OXA) to HCT-116 colon cancer cells.118 They found that OXA was more lethal to HCT-116 cells, which was due to the interaction of CD44 receptors with NH2-groups to increase the intracellular uptake of NCs-OXA. Avedian et al. designed an iron oxide (Fe3O4) core coated with mesoporous silica, and then conjugated with FA through PEI to construct a pH-sensitive targeting nanocarrier for loading erlotinib anticancer agent.119 The results showed that folic acid-labeled nanoparticles exhibited high toxicity to HeLa cells. Therefore, targeted drug delivery systems can reduce the side effects of anticancer drugs.
Generally, inorganic nanoparticles are more stable and easier in the manufacturing process than liposomes and polymer nanoparticles, which enables them to circulate in the body for a long time. However, it is precisely this point that makes them difficult to degrade in the body and has potential toxicity. Therefore, the research and development of biodegradable inorganic nanoparticles is the direction of inorganic delivery system in the future.
4. Conclusion
With the development of medical diagnosis and treatment technology, people's understanding of cancer is deepening, and nanoparticles of various materials have been developed as drug carriers for cancer cell membrane-targeted drug delivery systems. Here, we focus on some recent progress of nanocarriers as an innovative platform for effective delivery and controlled drug release. In order to improve the intratumor delivery and active targeting of drugs, especially for drugs targeting cancer cells, the surface of nanocarriers can be modified with various receptor-targeting ligands (such as, FR, TfR, HA, etc.) to specifically bind to the receptors on the surface membrane of cancer cells. Among them, nano-materials containing biocompatible polymers, liposomes or inorganic materials combined with targeting parts show great scope in carrying effective drugs to target sites to enhance therapeutic purposes.
Although the research on targeted nano-delivery system has been invested heavily at present, its clinical application still faces many problems, such as biological distribution, pharmacokinetics, nano-biological interface interaction, and lack of mass production technology. In addition, when used in patients, the potential toxicity of nanoparticles seriously hinders clinical transformation. There is still a lack of accurate in vitro and in vivo models to evaluate the real patient treatment, which leads to the difference between clinical and preclinical results. Therefore, when designing, optimizing and developing nanoparticles in preclinical stage, there is an urgent need for more evaluation in these aspects. It is worth mentioning that through the joint efforts of scientists and clinicians, more encouraging news can be brought to the active targeting field of anticancer drug nanomaterials in the future.
Author contributions
The manuscript was written through contributions of all authors. All authors have given approval to the final version of the manuscript.
Conflicts of interest
There are no conflicts to declare.
Acknowledgements
This review was supported by the Zhejiang Provincial Natural Science Foundation of China (grant numbers LZ23B040001 and LY23E030011), the National Natural Science Foundation of China (grant numbers 21971049 and 51903070), and “Ten-thousand Talents Plan” of Zhejiang Province (grant number 2019R52040).
References
- H. Sung, J. Ferlay, R. L. Siegel, M. Laversanne, I. Soerjomataram, A. Jemal and F. Bray, Global Cancer Statistics 2020: GLOBOCAN Estimates of Incidence and Mortality Worldwide for 36 Cancers in 185 Countries, Ca-Cancer J. Clin., 2021, 71, 209–249 CrossRef PubMed.
- J. Verma, C. Warsame, R. K. Seenivasagam, N. K. Katiyar, E. Aleem and S. Goel, Nanoparticle-mediated cancer cell therapy: basic science to clinical applications, Cancer Metastasis Rev., 2023 DOI:10.1007/s10555-023-10086-2.
- E. Atlihan-Gundogdu, D. Ilem-Ozdemir, M. Ekinci, E. Ozgenc, E. S. Demir, B. Sánchez-Dengra and I. González-Alvárez, Recent developments in cancer therapy and diagnosis, J. Pharm. Invest., 2020, 50, 349–361 CrossRef.
- M. Chen, Y.-X. Ren, Y. Xie and W.-L. Lu, Gene regulations and delivery vectors for treatment of cancer, J. Pharm. Invest., 2020, 50, 309–326 CrossRef.
- M. T. Manzari, Y. Shamay, H. Kiguchi, N. Rosen, M. Scaltriti and D. A. Heller, Targeted drug delivery strategies for precision medicines, Nat. Rev. Mater., 2021, 6, 351–370 CrossRef CAS PubMed.
- S. Bajpai, S. K. Tiwary, M. Sonker, A. Joshi, V. Gupta, Y. Kumar, N. Shreyash and S. Biswas, Recent Advances in Nanoparticle-Based Cancer Treatment: A Review, ACS Appl. Nano Mater., 2021, 4, 6441–6470 CrossRef CAS.
- Z. Wei, Y. Zhou, R. Wang, J. Wang and Z. Chen, Aptamers as Smart Ligands for Targeted Drug Delivery in Cancer Therapy, Pharmaceutics, 2022, 14, 2561 CrossRef CAS.
- X. Yu, Z. Weng, Z. Zhao, J. Xu, Z. Qi and J. Liu, Assembly of Protein Cages for Drug Delivery, Pharmaceutics, 2022, 14, 2609 CrossRef CAS.
- M. Chamundeeswari, J. Jeslin and M. L. Verma, Nanocarriers for drug delivery applications, Environ. Chem. Lett., 2019, 17, 849–865 CrossRef CAS.
- L. Meylina, M. Muchtaridi, I. M. Joni, A. F. A. Mohammed and N. Wathoni, Nanoformulations of α-Mangostin for Cancer Drug Delivery System, Pharmaceutics, 2021, 13, 1993 CrossRef CAS PubMed.
- K. Nishida, Recent Advances in Lipid-Based Drug Delivery, Pharmaceutics, 2021, 13, 926 CrossRef PubMed.
- K. Liu, X. Jiang and P. Hunziker, Carbohydrate-based amphiphilic nano delivery systems for cancer therapy, Nanoscale, 2016, 8, 16091–16156 RSC.
- L. Ding, C. Yao, X. Yin, C. Li, Y. Huang, M. Wu, B. Wang, X. Guo, Y. Wang and M. Wu, Size, Shape, and Protein Corona Determine Cellular Uptake and Removal Mechanisms of Gold Nanoparticles, Small, 2018, 14, 1801451 CrossRef.
- A. A. H. Abdellatif, H. A. Mohammed, R. A. Khan, V. Singh, A. Bouazzaoui, M. Yusuf, N. Akhtar, M. Khan, A. Al-Subaiyel, S. A. A. Mohammed and M. S. Al-Omar, Nano-scale delivery: A comprehensive review of nano-structured devices, preparative techniques, site-specificity designs, biomedical applications, commercial products, and references to safety, cellular uptake, and organ toxicity, Nanotechnol. Rev., 2021, 10, 1493–1559 CrossRef CAS.
- M. J. Mitchell, M. M. Billingsley, R. M. Haley, M. E. Wechsler, N. A. Peppas and R. Langer, Engineering precision nanoparticles for drug delivery, Nat. Rev. Drug Discovery, 2021, 20, 101–124 CrossRef CAS PubMed.
- F. Danhier, O. Feron and V. Préat, To exploit the tumor microenvironment: Passive and active tumor targeting of nanocarriers for anti-cancer drug delivery, J. Control. Release, 2010, 148, 135–146 CrossRef CAS.
- V. Torchilin, Tumor delivery of macromolecular drugs based on the EPR effect, Adv. Drug Delivery Rev., 2011, 63, 131–135 CrossRef CAS.
- H. Maeda, J. Wu, T. Sawa, Y. Matsumura and K. Hori, Tumor vascular permeability and the EPR effect in macromolecular therapeutics: a review, J. Control. Release, 2000, 65, 271–284 CrossRef CAS PubMed.
- Y. Yao, Y. Zhou, L. Liu, Y. Xu, Q. Chen, Y. Wang, S. Wu, Y. Deng, J. Zhang and A. Shao, Nanoparticle-Based Drug Delivery in Cancer Therapy and Its Role in Overcoming Drug Resistance, Front. Mol. Biosci., 2020, 7, 193–206 CrossRef CAS PubMed.
- M. I. Setyawati, C. Y. Tay, B. H. Bay and D. T. Leong, Gold Nanoparticles Induced Endothelial Leakiness Depends on Particle Size and Endothelial Cell Origin, ACS Nano, 2017, 11, 5020–5030 CrossRef CAS PubMed.
- H. Maeda, T. Sawa and T. Konno, Mechanism of tumor-targeted delivery of macromolecular drugs, including the EPR effect in solid tumor and clinical overview of the prototype polymeric drug SMANCS, J. Control. Release, 2001, 74, 47–61 CrossRef CAS PubMed.
- D. Manzanares and V. Ceña, Endocytosis: The Nanoparticle and Submicron Nanocompounds Gateway into the Cell, Pharmaceutics, 2020, 12, 371 CrossRef CAS PubMed.
- S. Raj, S. Khurana, R. Choudhari, K. K. Kesari, M. A. Kamal, N. Garg, J. Ruokolainen, B. C. Das and D. Kumar, Specific targeting cancer cells with nanoparticles and drug delivery in cancer therapy, Semin. Cancer Biol., 2021, 69, 166–177 CrossRef CAS PubMed.
- M. J. Nirmala, U. Kizhuveetil, A. Johnson, G. Balaji, R. Nagarajan and V. Muthuvijayan, Cancer nanomedicine: a review of nano-therapeutics and challenges ahead, RSC Adv., 2023, 13, 8606–8629 RSC.
- T. Yang, J. Zhai, D. Hu, R. Yang, G. Wang, Y. Li and G. Liang, “Targeting Design” of Nanoparticles in Tumor Therapy, Pharmaceutics, 2022, 14, 1919 CrossRef CAS PubMed.
- H. Yu, Z. Yang, F. Li, L. Xu and Y. Sun, Cell-mediated targeting drugs delivery systems, Drug Delivery, 2020, 27, 1425–1437 CrossRef CAS.
- T. Nakamura, H. Akita, Y. Yamada, H. Hatakeyama and H. Harashima, A Multifunctional Envelope-type Nanodevice for Use in Nanomedicine: Concept and Applications, Acc. Chem. Res., 2012, 45, 1113–1121 CrossRef CAS PubMed.
- S. Biswas and V. P. Torchilin, Nanopreparations for organelle-specific delivery in cancer, Adv. Drug Delivery Rev., 2014, 66, 26–41 CrossRef CAS PubMed.
- K. Cho, X. Wang, S. Nie, Z. Chen and D. M. Shin, Therapeutic Nanoparticles for Drug Delivery in Cancer, Clin. Cancer Res., 2008, 14, 1310–1316 CrossRef CAS.
- K. B. Sutradhar and M. L. Amin, Nanotechnology in Cancer Drug Delivery and Selective Targeting, ISRN Nanotechnol., 2014, 2014, 939378 Search PubMed.
- Y. Zhu, J. Feijen and Z. Zhong, Dual-targeted nanomedicines for enhanced tumor treatment, Nano Today, 2018, 18, 65–85 CrossRef CAS.
- H. O. Habashy, D. G. Powe, C. M. Staka, E. A. Rakha, G. Ball, A. R. Green, M. Aleskandarany, E. C. Paish, R. Douglas Macmillan, R. I. Nicholson, I. O. Ellis and J. M. W. Gee, Transferrin receptor (CD71) is a marker of poor prognosis in breast cancer and can predict response to tamoxifen, Breast Cancer Res. Treat., 2010, 119, 283–293 CrossRef CAS.
- T. R. Daniels, T. Delgado, J. A. Rodriguez, G. Helguera and M. L. Penichet, The transferrin receptor part I: Biology and targeting with cytotoxic antibodies for the treatment of cancer, Clin. Immunol., 2006, 121, 144–158 CrossRef CAS PubMed.
- X.-N. Wu, D. Su, L. Wang and F.-L. Yu, Roles of the hepcidin–ferroportin axis and iron in cancer, Eur. J. Cancer Prev., 2014, 23, 122–133 CrossRef CAS PubMed.
- R. Sutherland, D. Delia, C. Schneider, R. Newman, J. Kemshead and M. Greaves, Ubiquitous cell-surface glycoprotein on tumor cells is proliferation-associated receptor for transferrin, Proc. Natl. Acad. Sci. U. S. A., 1981, 78, 4515–4519 CrossRef CAS.
- P. Carbognani, M. Rusca, A. Romani, L. Spaggiari, L. Cattelani, P. Solli and P. Bobbio, Transferrin receptor expression in nonsmall cell lung cancer: Histopathologic and clinical correlates, Cancer, 1996, 78, 178–179 CrossRef CAS.
- Z. Wang, F. Meng and Z. Zhong, Emerging targeted drug delivery strategies toward ovarian cancer, Adv. Drug Delivery Rev., 2021, 178, 113969 CrossRef CAS PubMed.
- T. E. Ritter, O. Fajardo, H. Matsue, R. G. Anderson and S. W. Lacey, Folate receptors targeted to clathrin-coated pits cannot regulate vitamin uptake, Proc. Natl. Acad. Sci. U. S. A., 1995, 92, 3824–3828 CrossRef CAS PubMed.
- K. G. Rothberg, Y. S. Ying, J. F. Kolhouse, B. A. Kamen and R. G. Anderson, The glycophospholipid-linked folate receptor internalizes folate without entering the clathrin-coated pit endocytic pathway, J. Cell Biol., 1990, 110, 637–649 CrossRef CAS PubMed.
- V. Boshnjaku, K.-W. Shim, T. Tsurubuchi, S. Ichi, E. V. Szany, G. Xi, B. Mania-Farnell, D. G. McLone, T. Tomita and C. S. Mayanil, Nuclear localization of folate receptor alpha: a new role as a transcription factor, Sci. Rep., 2012, 2, 980 CrossRef PubMed.
- O. Spiegelstein, J. D. Eudy and R. H. Finnell, Identification of two putative novel folate receptor genes in humans and mouse, Gene, 2000, 258, 117–125 CrossRef CAS PubMed.
- H. Elnakat and M. Ratnam, Distribution, functionality and gene regulation of folate receptor isoforms: implications in targeted therapy, Adv. Drug Delivery Rev., 2004, 56, 1067–1084 CrossRef CAS PubMed.
- R. Bajracharya, J. G. Song, B. R. Patil, S. H. Lee, H.-M. Noh, D.-H. Kim, G.-L. Kim, S.-H. Seo, J.-W. Park, S. H. Jeong, C. H. Lee and H.-K. Han, Functional ligands for improving anticancer drug therapy: current status and applications to drug delivery systems, Drug Delivery, 2022, 29, 1959–1970 CrossRef CAS PubMed.
- L. E. Kelemen, The role of folate receptor α in cancer development, progression and treatment: Cause, consequence or innocent bystander?, Int. J. Cancer, 2006, 119, 243–250 CrossRef CAS PubMed.
- I. B. Vergote, C. Marth and R. L. Coleman, Role of the folate receptor in ovarian cancer treatment: evidence, mechanism, and clinical implications, Cancer Metastasis Rev., 2015, 34, 41–52 CrossRef CAS PubMed.
- J. F. Ross, P. K. Chaudhuri and M. Ratnam, Differential regulation of folate receptor isoforms in normal and malignant tissues in vivo and in established cell lines. Physiologic and clinical implications, Cancer, 1994, 73, 2432–2443 CrossRef CAS PubMed.
- N. Parker, M. J. Turk, E. Westrick, J. D. Lewis, P. S. Low and C. P. Leamon, Folate receptor expression in carcinomas and normal tissues determined by a quantitative radioligand binding assay, Anal. Biochem., 2005, 338, 284–293 CrossRef CAS PubMed.
- A. Cheung, H. J. Bax, D. H. Josephs, K. M. Ilieva, G. Pellizzari, J. Opzoomer, J. Bloomfield, M. Fittall, A. Grigoriadis, M. Figini, S. Canevari, J. F. Spicer, A. N. Tutt and S. N. Karagiannis, Targeting folate receptor alpha for cancer treatment, Oncotarget, 2016, 7, 52553–52574 CrossRef PubMed.
- C. Chen, J. Ke, X.
E. Zhou, W. Yi, J. S. Brunzelle, J. Li, E.-L. Yong, H. E. Xu and K. Melcher, Structural basis for molecular recognition of folic acid by folate receptors, Nature, 2013, 500, 486–489 CrossRef CAS.
- L. Xu, Q. Bai, X. Zhang and H. Yang, Folate-mediated chemotherapy and diagnostics: An updated review and outlook, J. Control. Release, 2017, 252, 73–82 CrossRef CAS PubMed.
- M. London and E. Gallo, Epidermal growth factor receptor (EGFR) involvement in epithelial-derived cancers and its current antibody-based immunotherapies, Cell Biol. Int., 2020, 44, 1267–1282 CrossRef CAS.
- S. Kumagai, S. Koyama and H. Nishikawa, Antitumour immunity regulated by aberrant ERBB family signalling, Nat. Rev. Cancer, 2021, 21, 181–197 CrossRef CAS PubMed.
- E. R. Purba, E.-i. Saita and I. N. Maruyama, Activation of the EGF Receptor by Ligand Binding and Oncogenic Mutations: The “Rotation Model”, Cells, 2017, 6, 13 CrossRef PubMed.
- M. A. Lemmon and J. Schlessinger, Cell Signaling by Receptor Tyrosine Kinases, Cell, 2010, 141, 1117–1134 CrossRef CAS PubMed.
- W. Pao and J. Chmielecki, Rational, biologically based treatment of EGFR-mutant non-small-cell lung cancer, Nat. Rev. Cancer, 2010, 10, 760–774 CrossRef CAS.
- S. Kamath and J. K. Buolamwini, Targeting EGFR and HER-2 receptor tyrosine kinases for cancer drug discovery and development, Med. Res. Rev., 2006, 26, 569–594 CrossRef CAS PubMed.
- S. N. Milik, D. S. Lasheen, R. A. T. Serya and K. A. M. Abouzid, How to train your inhibitor: Design strategies to overcome resistance to Epidermal Growth Factor Receptor inhibitors, Eur. J. Med. Chem., 2017, 142, 131–151 CrossRef CAS PubMed.
- C. Yewale, D. Baradia, I. Vhora, S. Patil and A. Misra, Epidermal growth factor receptor targeting in cancer: A review of trends and strategies, Biomaterials, 2013, 34, 8690–8707 CrossRef CAS PubMed.
- R. Roskoski, The ErbB/HER family of protein-tyrosine kinases and cancer, Pharmacol. Res., 2014, 79, 34–74 CrossRef CAS PubMed.
- H. Bier, T. Hoffmann, I. Haas and A. van Lierop, Anti-(epidermal growth factor) receptor monoclonal antibodies for the induction of antibody-dependent cell-mediated cytotoxicity against squamous cell carcinoma lines of the head and neck, Cancer Immunol., Immunother., 1998, 46, 167–173 CrossRef CAS.
- E. d. S. Santos, K. A. B. Nogueira, L. C. C. Fernandes, J. R. P. Martins, A. V. F. Reis, J. d. B. V. Neto, I. J. d. S. Júnior, C. Pessoa, R. Petrilli and J. O. Eloy, EGFR targeting for cancer therapy: Pharmacology and immunoconjugates with drugs and nanoparticles, Int. J. Pharm., 2021, 592, 120082 CrossRef CAS PubMed.
- M. Mishra, V. Chandavarkar, R. Sharma and D. Bhargava, Structure, function and role of CD44 in neoplasia, J. Oral Maxillofac. Pathol., 2019, 23, 267–272 CrossRef PubMed.
- M. Hassn Mesrati, S. E. Syafruddin, M. A. Mohtar and A. Syahir, CD44: A Multifunctional Mediator of Cancer Progression, Biomolecules, 2021, 11, 1850 CrossRef CAS PubMed.
- C. Hou, Y. Ishi, H. Motegi, M. Okamoto, Y. Ou, J. Chen and S. Yamaguchi, Overexpression of CD44 is associated with a poor prognosis in grade II/III gliomas, J. Neuro-Oncol., 2019, 145, 201–210 CrossRef CAS PubMed.
- H. Xu, M. Niu, X. Yuan, K. Wu and A. Liu, CD44 as a tumor biomarker and therapeutic target, Exp. Hematol. Oncol., 2020, 9, 36 CrossRef CAS PubMed.
- I. Morath, T. N. Hartmann and V. Orian-Rousseau, CD44: More than a mere stem cell marker, Int. J. Biochem. Cell Biol., 2016, 81, 166–173 CrossRef CAS.
- K. Hu, H. Zhou, Y. Liu, Z. Liu, J. Liu, J. Tang, J. Li, J. Zhang, W. Sheng, Y. Zhao, Y. Wu and C. Chen, Hyaluronic acid functional amphipathic and redox-responsive polymer particles for the co-delivery of doxorubicin and cyclopamine to eradicate breast cancer cells and cancer stem cells, Nanoscale, 2015, 7, 8607–8618 RSC.
- J. Ni, P. J. Cozzi, J. L. Hao, J. Beretov, L. Chang, W. Duan, S. Shigdar, W. J. Delprado, P. H. Graham, J. Bucci, J. H. Kearsley and Y. Li, CD44 variant 6 is associated with prostate cancer metastasis and chemo-/radioresistance, Prostate, 2014, 74, 602–617 CrossRef CAS PubMed.
- J. S. Desgrosellier and D. A. Cheresh, Integrins in cancer: biological implications and therapeutic opportunities, Nat. Rev. Cancer, 2010, 10, 9–22 CrossRef CAS PubMed.
- D. A. Calderwood, Integrin activation, J. Cell Sci., 2004, 117, 657–666 CrossRef CAS PubMed.
- J. Xiong, L. Yan, C. Zou, K. Wang, M. Chen, B. Xu, Z. Zhou and D. Zhang, Integrins regulate stemness in solid tumor: an emerging therapeutic target, J. Hematol. Oncol., 2021, 14, 177 CrossRef CAS.
- H. Hamidi and J. Ivaska, Every step of the way: integrins in cancer progression and metastasis, Nat. Rev. Cancer, 2018, 18, 533–548 CrossRef CAS PubMed.
- Y.-S. Zhu, K. Tang and J. Lv, Peptide–drug conjugate-based novel molecular drug delivery system in cancer, Trends Pharmacol. Sci., 2021, 42, 857–869 CrossRef CAS PubMed.
- P. Ge, Y. Liu, Q. Chen, Z. Su, Y. Du, S. Luo, X. Zhao, X. Cao, H. Song and X. Zhu, Transferrin receptors/magnetic resonance dual-targeted nanoplatform for precise chemo-photodynamic synergistic cancer therapy, Nanomed. Nanotechnol., 2022, 39, 102467 CrossRef CAS.
- M. L. Essa, A. A. Elashkar, N. A. N. Hanafy, E. M. Saied and M. El-Kemary, Dual targeting nanoparticles based on hyaluronic and folic acids as a promising delivery system of the encapsulated 4-Methylumbelliferone (4-MU) against invasiveness of lung cancer in vivo and in vitro, Int. J. Biol. Macromol., 2022, 206, 467–480 CrossRef CAS.
- M. Amin, M. Mansourian, P. C. Burgers, B. Amin, M. R. Jaafari and T. L. M. ten Hagen, Increased Targeting Area in Tumors by Dual-Ligand Modification of Liposomes with RGD and TAT Peptides, Pharmaceutics, 2022, 14, 458 CrossRef CAS PubMed.
- Y. I. Park, S.-H. Kwon, G. Lee, K. Motoyama, M. W. Kim, M. Lin, T. Niidome, J. H. Choi and R. Lee, pH-sensitive multi-drug liposomes targeting folate receptor β for efficient treatment of non-small cell lung cancer, J. Control. Release, 2021, 330, 1–14 CrossRef CAS PubMed.
- C. Liu, H. Gao, Z. Zhao, I. Rostami, C. Wang, L. Zhu and Y. Yang, Improved tumor targeting and penetration by a dual-functional poly(amidoamine) dendrimer for the therapy of triple-negative breast cancer, J. Mater. Chem. B, 2019, 7, 3724–3736 RSC.
- F. Zhu, G. Tan, Y. Zhong, Y. Jiang, L. Cai, Z. Yu, S. Liu and F. Ren, Smart nanoplatform for sequential drug release and enhanced chemo-thermal effect of dual drug loaded gold nanorod vesicles for cancer therapy, J. Nanobiotechnol., 2019, 17, 44 CrossRef PubMed.
- R. Kotcherlakota, K. Vydiam, D. Jeyalakshmi Srinivasan, S. Mukherjee, A. Roy, M. Kuncha, T. N. Rao, R. Sistla, V. Gopal and C. R. Patra, Restoration of p53 Function in Ovarian Cancer Mediated by Gold Nanoparticle-Based EGFR Targeted Gene Delivery System, ACS Biomater. Sci. Eng., 2019, 5, 3631–3644 CrossRef CAS PubMed.
- S. Mahalunkar, A. S. Yadav, M. Gorain, V. Pawar, R. Braathen, S. Weiss, B. Bogen, S. W. Gosavi and G. C. Kundu, Functional design of pH-responsive folate-targeted polymer-coated gold nanoparticles for drug delivery and in vivo therapy in breast cancer, Int. J. Nanomed., 2019, 14, 8285–8302 CrossRef CAS.
- K. Saini and R. Bandyopadhyaya, Transferrin-Conjugated Polymer-Coated Mesoporous Silica Nanoparticles Loaded with Gemcitabine for Killing Pancreatic Cancer Cells, ACS Appl. Nano Mater., 2020, 3, 229–240 CrossRef CAS.
- G. Lohiya and D. S. Katti, Carboxylated chitosan-mediated improved efficacy of mesoporous silica nanoparticle-based targeted drug delivery system for breast cancer therapy, Carbohydr. Polym., 2022, 277, 118822 CrossRef CAS PubMed.
- Y. Huang, K. Mao, B. Zhang and Y. Zhao, Superparamagnetic iron oxide nanoparticles conjugated with folic acid for dual target-specific drug delivery and MRI in cancer theranostics, Mater. Sci. Eng., C, 2017, 70, 763–771 CrossRef CAS PubMed.
- D. Hwang, J. D. Ramsey and A. V. Kabanov, Polymeric micelles for the delivery of poorly soluble drugs: From nanoformulation to clinical approval, Adv. Drug Delivery Rev., 2020, 156, 80–118 CrossRef CAS PubMed.
- S. Panja, G. Dey, R. Bharti, K. Kumari, T. K. Maiti, M. Mandal and S. Chattopadhyay, Tailor-Made Temperature-Sensitive Micelle for Targeted and On-Demand Release of Anticancer Drugs, ACS Appl. Mater. Interfaces, 2016, 8, 12063–12074 CrossRef CAS.
- M. Ling, R. Sun, G. Li, M. Z. Syeda, W. Ma, Z. Mai, L. Shao, L. Tang and Z. Yu, NIR-II emissive dye based polymer nanoparticle targeting EGFR for oral cancer theranostics, Nano Res., 2022, 15, 6288–6296 CrossRef CAS.
- A. S. Yadav, N. N. V. Radharani, M. Gorain, A. Bulbule, D. Shetti, G. Roy, T. Baby and G. C. Kundu, RGD functionalized chitosan nanoparticle mediated targeted delivery of raloxifene selectively suppresses angiogenesis and tumor growth in breast cancer, Nanoscale, 2020, 12, 10664–10684 RSC.
- A. D. Bangham, M. M. Standish and J. C. Watkins, Diffusion of univalent ions across the lamellae of swollen phospholipids, J. Mol. Biol., 1965, 13, 238–252 CrossRef CAS PubMed.
- B. S. Pattni, V. V. Chupin and V. P. Torchilin, New Developments in Liposomal Drug Delivery, Chem. Rev., 2015, 115, 10938–10966 CrossRef CAS PubMed.
- S. G. Klochkov, M. E. Neganova, V. N. Nikolenko, K. Chen, S. G. Somasundaram, C. E. Kirkland and G. Aliev, Implications of nanotechnology for the treatment of cancer: Recent advances, Semin. Cancer Biol., 2021, 69, 190–199 CrossRef CAS.
- X. Zhu, X. Chi, J. Chen, L. Wang, X. Wang, Z. Chen and J. Gao, Real-Time Monitoring In Vivo Behaviors of Theranostic Nanoparticles by Contrast-Enhanced T1 Imaging, Anal. Chem., 2015, 87, 8941–8948 CrossRef CAS.
- D. E. Large, R. G. Abdelmessih, E. A. Fink and D. T. Auguste, Liposome composition in drug delivery design, synthesis, characterization, and clinical application, Adv. Drug Delivery Rev., 2021, 176, 113851 CrossRef CAS PubMed.
- G. Bozzuto and A. Molinari, Liposomes as nanomedical devices, Int. J. Nanomed., 2015, 10, 975–999 CrossRef CAS.
- L. Arabi, A. Badiee, F. Mosaffa and M. R. Jaafari, Targeting CD44 expressing cancer cells with anti-CD44 monoclonal antibody improves cellular uptake and antitumor efficacy of liposomal doxorubicin, J. Control. Release, 2015, 220, 275–286 CrossRef CAS PubMed.
- Z. Song, Y. Shi, Q. Han and G. Dai, Endothelial growth factor receptor-targeted and reactive oxygen species-responsive lung cancer therapy by docetaxel and resveratrol encapsulated lipid-polymer hybrid nanoparticles, Biomed. Pharmacother., 2018, 105, 18–26 CrossRef CAS PubMed.
- Sonali, R. P. Singh, N. Singh, G. Sharma, M. R. Vijayakumar, B. Koch, S. Singh, U. Singh, D. Dash, B. L. Pandey and M. S. Muthu, Transferrin liposomes of docetaxel for brain-targeted cancer applications: formulation and brain theranostics, Drug Delivery, 2016, 23, 1261–1271 CrossRef CAS PubMed.
- A. P. Sherje, M. Jadhav, B. R. Dravyakar and D. Kadam, Dendrimers: A versatile nanocarrier for drug delivery and targeting, Int. J. Pharm., 2018, 548, 707–720 CrossRef CAS PubMed.
- M. Nikzamir, Y. Hanifehpour, A. Akbarzadeh and Y. Panahi, Applications of Dendrimers in Nanomedicine and Drug Delivery: A Review, J. Inorg. Organomet. Polym. Mater., 2021, 31, 2246–2261 CrossRef CAS.
- Y. Wang, L. Wang, G. Chen and S. Gong, Carboplatin-Complexed and cRGD-Conjugated Unimolecular Nanoparticles for Targeted Ovarian Cancer Therapy, Macromol. Biosci., 2017, 17, 1600292 CrossRef.
- S. H. Medina and M. E. H. El-Sayed, Dendrimers as Carriers for Delivery of Chemotherapeutic Agents, Chem. Rev., 2009, 109, 3141–3157 CrossRef CAS.
- Y. Wang, R. Guo, X. Cao, M. Shen and X. Shi, Encapsulation of 2-methoxyestradiol within multifunctional poly(amidoamine) dendrimers for targeted cancer therapy, Biomaterials, 2011, 32, 3322–3329 CrossRef CAS.
- S. Hong, P. R. Leroueil, I. J. Majoros, B. G. Orr, J. R. Baker and M. M. Banaszak Holl, The Binding Avidity of a Nanoparticle-Based Multivalent Targeted Drug Delivery Platform, Chem. Biol., 2007, 14, 107–115 CrossRef CAS.
- Y.-H. Kim, J. Jeon, S. H. Hong, W.-K. Rhim, Y.-S. Lee, H. Youn, J.-K. Chung, M. C. Lee, D. S. Lee, K. W. Kang and J.-M. Nam, Tumor Targeting and Imaging Using Cyclic RGD-PEGylated Gold Nanoparticle Probes with Directly Conjugated Iodine-125, Small, 2011, 7, 2052–2060 CrossRef CAS PubMed.
- R. Liu, W. Xiao, C. Hu, R. Xie and H. Gao, Theranostic size-reducible and no donor conjugated gold nanocluster fabricated hyaluronic acid nanoparticle with optimal size for combinational treatment of breast cancer and lung metastasis, J. Control. Release, 2018, 278, 127–139 CrossRef CAS PubMed.
- W. Xu, J. Qian, G. Hou, Y. Wang, J. Wang, T. Sun, L. Ji, A. Suo and Y. Yao, A dual-targeted hyaluronic acid-gold nanorod platform with triple-stimuli responsiveness for photodynamic/photothermal therapy of breast cancer, Acta Biomater., 2019, 83, 400–413 CrossRef CAS PubMed.
- C. Giménez, C. de la Torre, M. Gorbe, E. Aznar, F. Sancenón, J. R. Murguía, R. Martínez-Máñez, M. D. Marcos and P. Amorós, Gated Mesoporous Silica Nanoparticles for the Controlled Delivery of Drugs in Cancer Cells, Langmuir, 2015, 31, 3753–3762 CrossRef.
- A. Bakhshian Nik, H. Zare, S. Razavi, H. Mohammadi, P. Torab Ahmadi, N. Yazdani, M. Bayandori, N. Rabiee and J. Izadi Mobarakeh, Smart drug delivery: Capping strategies for mesoporous silica nanoparticles, Microporous Mesoporous Mater., 2020, 299, 110115 CrossRef CAS.
- W. Cheng, J. Nie, L. Xu, C. Liang, Y. Peng, G. Liu, T. Wang, L. Mei, L. Huang and X. Zeng, pH-Sensitive Delivery Vehicle Based on Folic Acid-Conjugated Polydopamine-Modified Mesoporous Silica Nanoparticles for Targeted Cancer Therapy, ACS Appl. Mater. Interfaces, 2017, 9, 18462–18473 CrossRef CAS PubMed.
- P. Pereira, A. C. Serra and J. F. J. Coelho, Vinyl Polymer-based technologies towards the efficient delivery of chemotherapeutic drugs, Prog. Polym. Sci., 2021, 121, 101432 CrossRef CAS.
- K. Thananukul, C. Kaewsaneha, P. Opaprakasit, N. Lebaz, A. Errachid and A. Elaissari, Smart gating porous particles as new carriers for drug delivery, Adv. Drug Delivery Rev., 2021, 174, 425–446 CrossRef CAS PubMed.
- Q. Zhao, J. Liu, W. Zhu, C. Sun, D. Di, Y. Zhang, P. Wang, Z. Wang and S. Wang, Dual-stimuli responsive hyaluronic acid-conjugated mesoporous silica for targeted delivery to CD44-overexpressing cancer cells, Acta Biomater., 2015, 23, 147–156 CrossRef CAS PubMed.
- P. Venkatesan, N. Thirumalaivasan, H.-P. Yu, P.-S. Lai and S.-P. Wu, Redox Stimuli Delivery Vehicle Based on Transferrin-Capped MSNPs for Targeted Drug Delivery in Cancer Therapy, ACS Appl. Bio Mater., 2019, 2, 1623–1633 CrossRef CAS PubMed.
- H. Sabit, M. Abdel-Hakeem, T. Shoala, S. Abdel-Ghany, M. M. Abdel-Latif, J. Almulhim and M. Mansy, Nanocarriers: A Reliable Tool for the Delivery of Anticancer Drugs, Pharmaceutics, 2022, 14, 1566–1586 CrossRef CAS PubMed.
- Z. Wang, R. Qiao, N. Tang, Z. Lu, H. Wang, Z. Zhang, X. Xue, Z. Huang, S. Zhang, G. Zhang and Y. Li, Active targeting theranostic iron oxide nanoparticles for MRI and magnetic resonance-guided focused ultrasound ablation of lung cancer, Biomaterials, 2017, 127, 25–35 CrossRef CAS PubMed.
- M. Ghadiri, E. Vasheghani-Farahani, F. Atyabi, F. Kobarfard, F. Mohamadyar-Toupkanlou and H. Hosseinkhani, Transferrin-conjugated magnetic dextran-spermine nanoparticles for targeted drug transport across blood-brain barrier, J. Biomed. Mater. Res., Part A, 2017, 105, 2851–2864 CrossRef CAS.
- Y. Chen, H. Chen, D. Zeng, Y. Tian, F. Chen, J. Feng and J. Shi, Core/Shell Structured Hollow Mesoporous Nanocapsules: A Potential Platform for Simultaneous Cell Imaging and Anticancer Drug Delivery, ACS Nano, 2010, 4, 6001–6013 CrossRef CAS.
- H. Tabasi, M. T. Hamed Mosavian, Z. Sabouri, M. Khazaei and M. Darroudi, pH-responsive and CD44-targeting by Fe3O4/MSNs-NH2 nanocarriers for Oxaliplatin loading and colon cancer treatment, Inorg. Chem. Commun., 2021, 125, 108430 CrossRef CAS.
- N. Avedian, F. Zaaeri, M. P. Daryasari, H. Akbari Javar and M. Khoobi, pH-sensitive biocompatible mesoporous magnetic nanoparticles labeled with folic acid as an efficient carrier for controlled anticancer drug delivery, J. Drug Delivery Sci. Technol., 2018, 44, 323–332 CrossRef CAS.
|
This journal is © The Royal Society of Chemistry 2023 |