DOI:
10.1039/D3RA03440B
(Review Article)
RSC Adv., 2023,
13, 22973-22997
Nanostructured wearable electrochemical and biosensor towards healthcare management: a review
Received
23rd May 2023
, Accepted 29th June 2023
First published on 31st July 2023
Abstract
In recent years, there has been a rapid increase in demand for wearable sensors, particularly these tracking the surroundings, fitness, and health of people. Thus, selective detection in human body fluid is a demand for a smart lifestyle by quick monitoring of electrolytes, drugs, toxins, metabolites and biomolecules, proteins, and the immune system. In this review, these parameters along with the main features of the latest and mostly cited research work on nanostructured wearable electrochemical and biosensors are surveyed. This study aims to help researchers and engineers choose the most suitable selective and sensitive sensor. Wearable sensors have broad and effective sensing platforms, such as contact lenses, Google Glass, skin-patch, mouth gourds, smartwatches, underwear, wristbands, and others. For increasing sensor reliability, additional advancements in electrochemical and biosensor precision, stability in uncontrolled environments, and reproducible sample conveyance are necessary. In addition, the optimistic future of wearable electrochemical sensors in fields, such as remote and customized healthcare and well-being is discussed. Overall, wearable electrochemical and biosensing technologies hold great promise for improving personal healthcare and monitoring performance with the potential to have a significant impact on daily lives. These technologies enable real-time body sensing and the communication of comprehensive physiological information.
1. Introduction
A wearable electrochemical biosensor (WEB) is an effective point-of-care (POC) diagnostic tool with excellent electrical signal response in normal physiological conditions. A wide range of platforms containing the properties of flexible, convenient, and light weighted materials that are sensitive to detect the target biomolecules have been reported for WEB applications.1,2 WEB as a POC diagnostic tool has a great demand for its comfort physiological signal sensing of electrolytes, drugs, toxins, metabolites and biomolecules, protein, and immune assay.3,4 In addition, wearable biosensor platforms can be easily attached to the body by using daily wearable items, such as glasses, clothes, shoes, gloves, and watches.5–8 Most wearable sensors can be easily controlled using bluetooth-driving smartphones or remote servers.9–16 Examples of the major wearable platforms are Google Glass, contact lenses, polyethylene terephthalate (PET) contact lenses, mouthguards, cotton underwear, cotton yarn, elastomeric stamps, and temporary tattoos.17,18 Currently, researchers are actively working on developing wearable biosensors to measure significant small molecules and biomarkers present in common body fluids.19 Up to date, skin, tears, and sweat-based electrochemical sensors and biosensors have been developed to monitor a few electrolytes and biomolecules with a selective and sensitive detection output.
Bio-receptors are utilized to modify the wearable sensing platforms depending on the physicochemical properties of the targeted analytes. In electrochemical analysis, the current is flown through the sensing platform by signaling for the oxidation or reduction of electroactive bio-nutrients. The modified working electrode is the key component, which is preferably prepared from poly(vinyl alcohol), silicones (e.g., PDMS, Ecoflex, and Solaris), or inert plastics (e.g., PET and PEN)20 to create a conductive base and then was decorated by using recognition agent to ensure better chemical responses. As an alternative to the flexible base, the metal-decorated conductive base becomes stretchable and bendable.21 To avoid this barrier, nanoparticles and graphene-processed conductive ink or tunable conductive polymers can be easily printed on a flexible base using conventional printing and binding methods.22–24 In the potentiometric and impedimetric sensors, no current is flown and further, the electrode is usually functionalized with commercial ionophores. Conversely, an enzymatic redox reaction is required to modify the working electrode, which is relatively complex and time-consuming. Besides, enzymes have high reduction potential and hierarchical structure, which is responsible for lower electron transfer from the enzyme pocket to the electrode. A mediator is required to reduce the working potential and increase electron mobility, such as Prussian blue (PB) and tetrathiafulvalene (TTF).25–27 Due to the toxicity of the mediator, alternatives, such as carbon-based materials, metal nanoparticles, and conductive polymers have been explored to mediate electron transfer in amperometric systems.28,29
Although enzymatic-recognized biosensors have a few limitations, they have wide applications in sensing metabolites in human sweat. In immune sensor studies, the bio-recognition elements have a strong affinity towards target analytes, but these bio receptors are not suitable for continuous monitoring in the human body for the lack of their irreversible nature. It is worth mentioning that, the surrounding is the reason for degradation and becoming unable to measure the target analyte.17 Self-life enzyme-modified electrodes were prepared by selected encapsulation technique and polymer-enzyme composites.30 Additionally, in wearable biosensors, molecularly imprinted polymers (MIPs) are intriguing replacements for enzymatic recognition elements because they are suitable ligand environments for specific analytes.31 Nanoparticles and organic frameworks have also been proposed as artificial receptors.32 In textiles and garments, modified wearable fibers can be used with a three-electrodes system to continuously measure electrochemical signals and avoid intermediate interactions.33 Furthermore, Wang et al. created a fiber-based working electrode by electrodepositing active materials onto carbon fibers and integrating these fibers into a sensing array.34 In another work, a thin gold fiber was prepared by dry-spinning gold nanowires for glucose sensing.35 Recently, fiber-based working electrodes and interconnected sensing systems have been reported through versatile research work on carbon fibers,36 stainless steel yarns,37 metallic fibers,38 and silver-coated nylon threads.39
This review surveys the modification of wearable electrochemical sensors and biosensors to detect ions, drugs, toxins, metabolite and biomolecules, proteins, and immune assay. The discussion about the previously reported research work along with the working principles, fabrication approaches, and flexible electrochemical biosensors based on nanoparticles and bio receptors to target analyte detection are presented. Furthermore, nanoparticles, MXene, composite materials, polymers, and biological receptors recently created extremely sensitive and selective electrochemical sensing devices and contributed to the modification of electrodes in electrochemical-based research work worldwide. The discussion and analysis in this work will be helpful to reach a concrete decision for the fabrication of wearable biosensor electrodes to detect a definite analyte. Furthermore, the insights are expected to become basic principles for upgrading next-generation wearable electrochemical bio-sensing devices.
2. Wearable sensor and biosensor platform
Body fluids are rich sources of important biomarkers including ions (e.g. Na+, K+, Ca+, NH4+, and Cl−), drugs and toxins (e.g. levodopa, caffeine, methyl xanthine, p-cresol), metabolites and biomolecules (e.g. glucose, lactate, uric acid, cortisol, ascorbic acid), protein and immune assay (e.g., AFP, CA125, CA153, ferritin, and E. coli), participate in many physiological diseases such as diabetes, gout, Parkinson's diseases, hepatitis, and myopia. The latest wearable electrochemical sensors and biosensors are designed according to their site of application. Joohee Kim et al. reported research on multifunctional contact lenses, which are applicable to both in vivo and in vitro analysis of live rabbits and bovine eyeballs.40,41 Contact lens would be an ideal vehicle for continuous tear glucose monitoring.42 Another wearable rapid diagnostic tool (RDT) is Google Glass, which is capable of both qualitative and quantitative analysis based on a hands-free voice-controlled interface and then digitally transmitted to a server for digital processing.41
The textile-based screen-printed carbon electrode cotton underwear offers voltammetric and chronoamperometric measurements of 0–3 mM ferrocyanide, 0–25 mM hydrogen peroxide, and 0–100 μM NADH.43 In sports and military applications, it brings great benefits to make digitalized human power. Another easily worn and replaceable biosensor is the mouthguard.43 This saliva monitoring biosensor can detect without any interruption or little occurrence. Additionally, conductive yarns can be prepared by simple dyeing with carbon nanotube ink. Afterwards, an ion-selective potentiostat was prepared by coating a polymeric membrane. This potentiometric yarn can easily sense pH, K+, and NH4+.44 Furthermore, conductive and insulating ink-mediated elastomeric stamps are well-suited for the formation of electrochemical sensors by following conventional screen-printed techniques.45 The elastomeric stamp preparation methods can be extended to epidermal electrochemical sensors. Temporary tattoos were used in several parts of the human body with individual platforms such as printed temporary transfer tattoos on the skin to monitor lactate in human perspiration.46 Windmiller et al. demonstrated the use of potentiometric ion-selective electrodes to monitor the pH level.47 Similarly, two research studies reported the detection of sodium47 and ammonium48 in sweat. In addition, a complete self-driven smartwatch can monitor glucose levels in a simple and easy way and further maintains safety and infection risk. It made a correlation between the sweat composition and human body dynamics.49
Jungil Choi et al. demonstrated the use of a pressure-induced skin-mounted microfluidic network for the quantification of lactate, sodium, and, potassium by chrono-sampling of device.50 Another soft microfluidic-based research work was performed using a microsystem designed with a biocompatible electronic-based electrochemical biosensor.51 Reflectance pulse oximetry is a miniature flexible device that can facilitate the mounting on the external (e.g. skin) and internal (e.g. heart and brain) of the human body. This flexible platform incorporated with optoelectronic functionality can be used for wireless capture and transmission of quantitative information on blood oxygenation, heart rate, and heart rate variability.52 The NFZ-GQDs@GOx platform was fabricated to construct a bioelectronic tongue to continuously monitor glucose with a detection limit of 14 μM53 (Scheme 1).
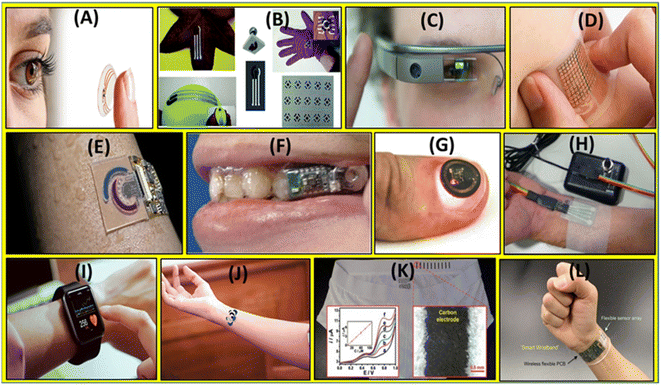 |
| Scheme 1 (A) Contact lens (https://xtalks.com/postech-researchers-develop-smart-contact-lenses-that-can-diagnose-and-treat-diabetes-2232/), (B) elastomeric stamp,45 (C) Google Glass (https://time.com/3669927/google-glass-explorer-program-ends/), (D) skin-patch (https://physicsworld.com/a/wearable-patch-could-predict-risk-of-stroke-and-heart-attacks/), (E) microfluidic device (https://innovationtoronto.org/index.php/2022/08/28/new-wearable-microfluidic-sensing-technology-can-provide-continuous-monitoring-for-many-health-conditions/), (F) mouthguard,54 (G) pulse-oximeter,52 (H) potentiostat,55 (I) smart watch,56 (J) temporary tattoo (https://www.wionews.com/science/tattoo-as-health-monitoring-device-south-korean-scientists-develop-unique-technology-502791), (K) underwear,43 and (L) wristband (https://www.medicaldesignandoutsourcing.com/wristband-detects-analyzes-real-time-changes-in-sweat-chemical-composition/). Figure is adopted from all reference sources with permission. | |
3. Nanostructured wearable biosensor
3.1 Biosensor for ions
Electrolytes play an important role in the body when they remain in body fluids as charged ions. They regulate the osmotic pressure in cells, help maintain the function of muscle and nerve cells, and transmit neural signals, body water content, and others. The main electrolytes include sodium, chlorine, potassium, calcium, and magnesium. A literature survey summarizing human body fluid concentration measurements is presented in Table 1. Gao et al. demonstrated research work for the detection of Na+ and K+ ions in human sweat samples using amperometric detection. A Na+ selective membrane cocktail mainly consisting of Na ionophore X (1% w/w), Na-TFPB (0.55% w/w), PVC (33% w/w), DOS (65.45% w/w), and tetrahydrofuran was used to disperse the cocktail for the purpose of drop casting. The drop casting electrode was denoted as Na+ selective electrode.68 On the other hand, the K+ selective membrane cocktail constructed from a mixture of valinomycin (2% w/w), Na-TPB (0.5%), PVC (32.7% w/w), DOS (64.7%), and cyclohexane was used to disperse and drop cast on a working electrode. Here, PVB modified electrode was used as a reference electrode.58 In both cases, poly(3,4-ethylenedioxythiophene) PEDOT:PSS polymerized film was first considered as an ion–electron transducer to minimize the potential drifts of the ISEs.59 Another study of Na+ selective membrane followed the same procedure with PVB reference electrode. The FeCl3 injected Ag/AgCl electrode was used as a Cl-selective electrode and PEDOT:PSS was chosen as the ion-selective transducer.60 Furthermore, AuNPs were electrodeposited on chips from 5 mM HAuCl4 and 0.5 M H2SO4 mixture at a constant potentiostatic voltage. Thereafter, Na+ ISE was also prepared from the same procedure reported by Bandodkar et al.57 An advanced-level research work detected the three electrolytes Na+, K+, and Ca+ using carbon nanotube-modified weaving fabric and was reported by Wang et al. In this fabric, Na+-ISE was prepared from a mixture containing Na-TFPB, high molecular weight PVC, DOS, sodium ionophore X in tetrahydrofuran, and Na+-ISE was prepared by replacing sodium ionophore X with potassium ionophore. The Ca+-ISE was also prepared following the same way as Na+ and K+-ISE, whereas, the ionophore was replaced with Ca ionophore II.34 The detection ranges of Na+, K+, and Ca+ ions were reported to be 10–160 × 10−3 M, 2–32 × 10−3 M, and 0.5–2.53 × 10−3 M, respectively. This study was conducted using sensing fiber weaving fabric in amperometric detection as represented in Fig. 1.
Table 1 A comparative electrolyte measurement study using wearable electrochemical sensorsa
Sensing material |
Analyte |
Detection range |
Detection limit |
Method of detection |
Bio-fluid |
Ref. |
Abbreviations: Amp-amperometric, Pote-potentiometric, Conduc-conduct metric, CV-cyclic voltammetry, ISE-ion selective electrode, CNT-carbon nanotube, rGO-reduced graphene oxide, PANI-polyaniline, PEDOT-poly(3,4-ethylenedioxythiophene), PSS-polystyrene sulfonate. |
ISE-K+ membrane |
K+ |
2–16 × 10−3 M |
N/A |
Amp |
Sweat |
68 |
ISE-Na+ membrane |
Na+ |
20–120 × 10−3 M |
N/A |
Amp |
Sweat |
68 |
ISE-Na+ membrane |
Na+ |
10–80 × 10−3 M |
N/A |
Amp |
Sweat |
60 |
ISE-Cl− membrane |
Cl− |
10–80 × 10−3 M |
N/A |
Amp |
Sweat |
60 |
AuND-ISE-Na+ membrane |
Na+ |
0–40 × 10−3 M |
0.8 × 10−6 M |
Poten |
Sweat |
61 |
Electrochemical fabric-CNT fabric substrate |
Na+ |
10–160 × 10−3 M |
N/A |
Amp |
Sweat |
34 |
Electrochemical fabric-CNT fabric substrate |
K+ |
2–32 × 10−3 M |
N/A |
Amp |
Sweat |
34 |
Electrochemical fabric-CNT fabric substrate |
Ca+ |
0.5–2.53 × 10−3 M |
N/A |
Amp |
Sweat |
34 |
Ag/AgCl |
Cl− |
N/A |
N/A |
Poten |
Sweat |
55 |
Bare gold |
Electrolytes |
N/A |
N/A |
Conduc |
Tears |
69 |
Graphene-doped Au mesh |
pH |
|
N/A |
Poten |
Sweat |
70 |
Carbon/rGO-Na+ membrane |
Na+ |
10–160 mM |
N/A |
Poten |
Sweat |
63 |
Carbon/rGO-K+ membrane |
K+ |
2–32 mM |
N/A |
Poten |
Sweat |
63 |
AuNP/PANI |
pH |
3–8 mM |
N/A |
Poten |
Sweat |
63 |
PEDOT:PSS/carbon fiber thread |
Na+ |
0.1–100 mM |
N/A |
Poten |
Sweat |
71 |
PEDOT:PSS/carbon fiber thread |
K+ |
0.1–100 mM |
N/A |
Poten |
Sweat |
72 |
CNT |
NH4+ |
N/A |
N/A |
Poten |
Sweat |
73 |
PANI conducting polymer |
pH |
N/A |
N/A |
Poten |
Wounds |
65 |
CNTs |
pH |
8.51–2.69 |
N/A |
Poten |
Sweat |
74 |
rGO-PANI |
pH |
75.09 nm pH−1 at pH 11.35 |
N/A |
Poten |
Sweat |
75 |
Graphite/Ag/AgCl |
pH |
pH range 6–9 |
N/A |
Poten |
Sweat |
76 |
Bi |
Cd+ |
<100 μg L−1 |
N/A |
CV |
Sweat and urine |
77 |
Bi, Au |
Pb+ |
<100 μg L−1 |
N/A |
CV |
Sweat and urine |
77 |
Au |
Cu2+ |
100–100 μg L−1 |
N/A |
CV |
Sweat and urine |
77 |
Au |
Hg+ |
<100 μg L−1 |
N/A |
CV |
Sweat and urine |
77 |
Ammonia ionophore |
NH4+ |
10−4 to 0.1 M |
N/A |
Poten |
Sweat |
65 |
Lanthanum fluoride |
Fluoride |
0.19–1.9 ppm |
N/A |
Poten |
Saliva |
78 |
ISE/fluorinated alkyl silane/GO |
K+ |
0–6.5 mM |
N/A |
Poten |
Sweat |
79 |
ISE/fluorinated alkyl silane/GO |
Na+ |
0–49.5 mM |
N/A |
Poten |
Sweat |
79 |
ISE/fluorinated alkyl silane/GO |
Cl− |
0–61.4 mM |
N/A |
Poten |
Sweat |
79 |
ISE/fluorinated alkyl silane/GO |
pH |
0–6.91 mM |
N/A |
Poten |
Sweat |
79 |
PEDOT |
Na+ |
1.89–2.97 mM |
N/A |
Amp |
Sweat |
2 |
PEDOT |
K+ |
3.31–7.25 mM |
N/A |
Amp |
Sweat |
2 |
PEDOT:PSS/Au |
Na+ |
10–160 mM |
N/A |
Poten |
Sweat |
68 |
PEDOT:PSS/Au |
K+ |
1–32 mM |
N/A |
Poten |
Sweat |
68 |
Polyaniline |
pH |
3–8 |
N/A |
Poten |
Sweat |
80 |
PEDOT:PSS |
Na+ |
45.8 mV dec−1 |
N/A |
Poten |
Sweat |
81 |
PEDOT:PSS |
K+ |
35.9 mV dec−1 |
N/A |
Poten |
Sweat |
81 |
PEDOT:PSS |
Ca+ |
52.3 mV dec−1 |
N/A |
Poten |
Sweat |
81 |
PANi/CNT fibre |
pH |
N/A |
N/A |
Poten |
Sweat |
81 |
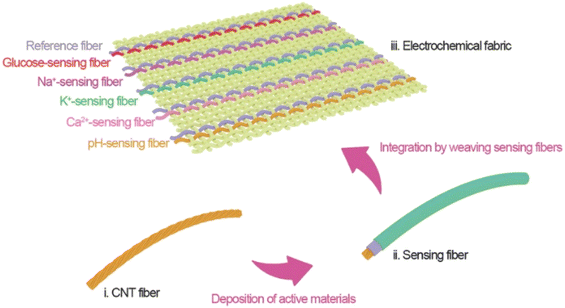 |
| Fig. 1 Weaving carbon nanotube fiber to smart electrochemical fabric. Adopted from ref. 35 with permission from American Chemical Society, Copyright© 2019. | |
Further detection of Na+ and K+ using all-solid-state ISEs was fabricated using reduced graphene oxide (rGO) as the ion-to-electron transducer between the ionophore and carbon working electrode.63 The rGO-based electrode showed high stability at low temperature without considering humidity. This sensor fabrication involves a low-cost method.64 The pH was measured by de-protonation from the surface of polyaniline (PANI).63 Similarly, pH was also measured from wounds and sweat in the human body.65 Additionally, Guinovart et al. reported a research work to quantify ammonium ions in sweat using a flower shaped potentiometric sensor similar to the tattoo sensor, and the construction is represented in Fig. 2.65 To make this temporary tattoo, a transparent insulator was printed on the tattoo paper. Thereafter, Ag/AgCl layer with a longer right petal, a carbon layer a let petal, and a surrounding blue insulator were printed. After the tattoo was printed and cured, the reference and ion selective membranes were incorporated via drop casting and drying methods.
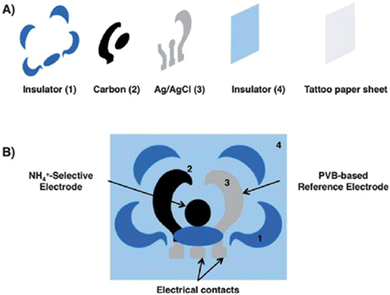 |
| Fig. 2 Stepwise potentiometric tattoo sensor fabrication. (A). Release the fabrication layer by the insulator, carbon, Ag/AgCl, and insulator. (B). Ion-selective and reference electrodes deposited onto the suitable area [adopted from ref. 65 with permission from Wiley, Copyright© 2014]. | |
Among the large classes of Na+, K+, and Cl− ion detection methods paper-based ISEs are one of the reported methods. In this study, high-quality graphene was dispersed in ethanol and sprayed onto the modified C10F paper through a stainless-steel mask. Then, Na+, K+, Cl−, and reference electrode cocktails were dropped on the graphene-modified electrode. After graphene modification, the electrode gained high charge carrier immobility, chemical stability, large surface area, increased toughness, and stretchability.66,67 From the above literature survey, it can be concluded that ISE-based ion detection can play a vital role in the next generation rapid diagnostic tools.
3.2 Biosensor for drugs and toxins
Drug monitoring plays an important role in doping control, at the right dose, for the right patient, and at the right time.82,83 It helps physicians to obtain information about drug dosages, compliance with prescriptions, and understanding the complex pharmacokinetics of drugs for optimal benefits.82 Normally, blood is a conventional biofluid source to monitor drugs, and further urine, transdermal, and sweat source are reported alternative sources for wearable electrochemical biosensing platforms.82 A literature survey for the comparison is summarized in Table 2. A wearable instant kit can also prevent the illegal accident of uptaking drug-related crimes, violence, sexual assaults, infectious diseases, etc.84 The ongoing expectation is that the continuous monitoring of the drug is a mechanism of selective detection in body fluidic samples.
Table 2 A comparative drug and toxin measurement study using wearable electrochemical and biosensorsa
Sensing material |
Analyte |
Detection range |
Detection limit |
Method of detection |
Bio-fluid |
Ref. |
Abbreviations: Amp-amperometric, SWV-square wave voltammetry, EIS-electrochemical impedance spectroscopy, DPV-differential pulse voltammetry, CNT-carbon nanotube, MOF-a metal–organic framework, BSA-bovine serum albumin, AOx-alcohol oxidase. |
Au |
Levodopa |
<10 μM |
N/A |
Amp |
Sweat |
85 |
Carbon paste/tyrosinase enzyme |
Levodopa |
0.5–3 μM |
0.5 μM |
SWV |
Artificial-ISF |
86 |
Carbon ink/CNT/Nafion |
Caffeine |
<40 μM |
N/A |
Amp |
Sweat |
82 |
Carbon ink/CNT/Nafion |
Methyl xanthine |
0–40 × 10−6 M |
3 × 10−6 M |
DPV |
Sweat |
82 |
Cu-MOF |
L-Histidine |
N/A |
5.3 μM |
|
Living cell |
87 |
Carbon ink/chitosan/BSA/AOx/PB |
Alcohol |
0–36 × 10−3 M |
N/A |
Amp |
Sweat |
89 |
Au–ZnO/AOx |
Alcohol |
2.17 × 10−6 to 43.4 × 10−3 M |
2.17 × 10−6 M |
EIS |
Sweat |
88 |
Pt wire/Chitosan/AOx |
Alcohol |
0–80 × 10−3 M |
N/A |
Amp |
Transdermal |
12 |
Au-electro needle |
p-Cresol |
1 × 10−6 to 1 × 10−3 M |
1.8 × 10−6 M |
N/A |
Transdermal |
90 |
Tai et al. reported research work on the fabrication of a PET substrate with Cr and Au by photolithography and evaporation method to detect levodopa, a medication to treat Parkinson's disease.85 In this study, Au nano-dendrites were grown on the electrode surface via a square wave of Gamry electrochemical potentiostat and chloroauric acidic medium. The lowest detected concentration of levodopa after 6000 cycles of Au deposition for 120 seconds was found to be 10 μM. Furthermore, a 1 × 3 pyramid-shaped hollow microneedle of 1500 μm height and 425 μm diameter was fabricated to detect levodopa in artificial ISF using SWV. Two of these electrodes, WE1 and WE2 were modified by carbon paste and the remaining electrode, Ag/AgCl was used as a reference electrode. WE2, the working electrode was further modified by tyrosinase mushroom enzyme.86 The schematic representation for the detection of levodopa is shown in Fig. 3.
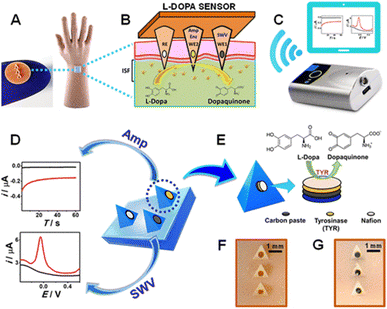 |
| Fig. 3 Schematic representation of levodopa (L-Dopa) detection, (A) hand-wearing mannequin microneedle sensor, (B) ISF levodopa monitoring, (C) wireless electroanalayser, (D) microneedle sensor platform for levodopa sensing using SWV and amperometry, (E) cross-sectional view of CP, tyrosinase and Nafion layer, (F) and, (G) optical image before and after CP packing of microneedles. Adopted from ref. 87 with permission from American Chemical Society, Copyright© 2019. | |
Another research work was performed by fabricating a PET substrate with silver, carbon, and nasion to quantitively measure methyl xanthine drugs and caffeine in sweat samples. In this study, Ag ink was mixed with 10% poly(vinyl butyral) in terpineol and printed at a constant temperature of 23 ± 2 °C and 35 ± 2%. Afterwards, carbon paste with 359 cP of viscosity was printed and dried at 150 °C for 5 seconds. Finally, the insulation was performed using polyethylene resin by annealing at 150 °C for 1 hour.82 L-Histidine is an essential amino acid and precursor of hormones and metabolites, as well as a drug for eczema. The detection was performed using MOF particles and chitosan-modified electrodes with a detection limit of L-histidine at 5.3 μM.87
Alcohol is a psychoactive and toxic substance depending on its producing properties. Ashlesha Bhide et al. published a research work on the detection of alcohol in sweat samples using alcohol oxidase modified electrode. The gold particle was deposited by Temescal e-beam and ZnO film by AJA Orion RF magnetron and finally with alcohol oxidase.88 Additionally, Ag/AgCl ink and PB conductive carbon were screen printed on working and counter electrodes by semi-automatic screen printing. A transparent insulator was screen-printed over the surface of the electrode pattern to confirm the electrodes and contact areas. For fabrication, the Ag/AgCl ink was cured at 90 °C for 10 minutes, and then the PB conductive carbon ink was cured at 80 °C for 10 minutes in a convection oven.89 The schematic representation is illustrated in Fig. 4. Furthermore, medical grade liquid crystal polymer (O-phenylene diamine) was poured onto the Pt wire micro-transducer followed by the immobilization of alcohol oxidase where chitosan was an intermediate layer.12 In this study, the alcohol detection linear range was 0–80 × 10−3 M. From an overall literature survey, it was found that levodopa detection with tyrosinase enzyme is suitable, and further, alcohol oxidase is suitable for the detection of alcohol.
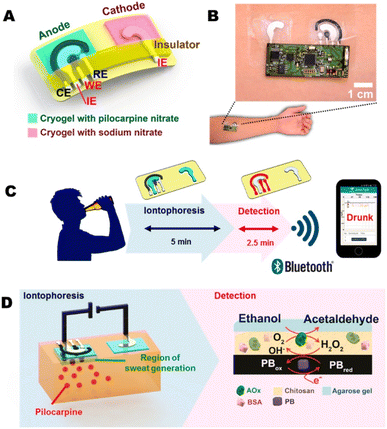 |
| Fig. 4 Transdermal alcohol sensor (A) iontophoretic tattoo electrode, (B) alcohol iontophoretic-sensing tattoo device, (C) diagram of iontophoresis and amperometric detection of alcohol, (D) diagram of iontophoresis system (left) and amperometric system (right). Adopted from ref. 13 with permission from Elsevier, Copyright© 2018. | |
3.3 Biosensor for metabolites and biomolecules
In metabolism, the intermediate and final products are the metabolites. These metabolites can be glucose, urea, uric acid, lactates, cholesterol, creatinine, hydrogen peroxide, ketone bodies, hypoxanthine, xanthine, etc. There have been some significant breakthroughs via electrochemical sensors in clinical applications concerning their measurement in a simple way, within the shortest possible time, with high accuracy, selectivity, and stability. The economic and sturdy nature of commercially available electrochemical glucometers is a strong indicator of their success. Here, we will discuss some of the significant developments of biosensors in different metabolites, as summarized in Table 3.
Table 3 A comparative metabolites and the biomolecules measurement study using wearable biosensorsa
Sensing material |
Analyte |
Detection range |
Detection limit |
Method of detection |
Sample |
Ref. |
Abbreviations: Amp-amperometric, Poten-potentiometric, DPV-differential pulse voltammetry, CV-cyclic voltammetry, ECL – electrochemiluminescence, Chrono amp-chrono amperometry, Colo-colorimetric, UA-uric acid, AA-ascorbic acid, DA-dopamine, AD-Adrenaline, PEDOT-poly(3,4-ethylenedioxythiophene), PSS-polystyrene sulfonate, GA-graphene, SWCNT-single-walled carbon nanotube, MWCNT-multiwalled carbon nanotube, CNT-carbon nanotube, GOx-glucose oxidase, UOx-uricase oxidase, PB-Prussian blue, BSA-bovine serum albumin, PVA-polyvinyl acetate, PANI-polyaniline, NW-nanowire, rGO-reduced graphene oxide, CP-carbon paste, PEI-polyethyleneimine, LOx-lactate oxidase, PEG-polyethylene glycol, OECT-organic electrochemical transistors. |
PEDOT-MeNH2/CLC/GCE |
UA |
5−400 μM |
0.255 μM |
DPV |
Blood |
102 |
GA/uricase/chitosan/SWCNT/Pt |
UA |
100–1000 μM |
1.0 μM |
CV |
Serum samples |
161 |
PEDOT-MeSH/CLC/GCE |
UA |
0.4–650 μM |
0.085 μM |
DPV |
Blood |
102 |
UOx/Pt NPs/PANI/MEA |
UA |
0.1–1.2 × 10−3 M |
4 μM |
Amp |
Standard sample |
162 |
CoO/N-CS-rGO |
UA |
1–125 μM |
0.22 μM |
CV |
Standard and human serum |
126 |
Urate oxidase/PLGA/MoS2-hydrogel system |
UA |
100–500 μmol L−1 |
20 μmol L−1 |
ECL |
Serum |
163 |
GF/NiCo2O4 |
UA |
10–26 μM |
0.2 μM |
CV and DPV |
Serum and urine |
93 |
Pt/LSG |
UA |
1–63 μM |
0.22 μM |
CV and DPV |
Urine |
94 |
Uricase/ZnONW |
UA |
0.024–0.101 × 10−3 M |
10 μM |
Piezo |
Sweat |
137 |
LEG-CS |
UA |
N/A |
0.74 μM |
DPV |
Sweat |
95 |
Uricase |
UA |
O.5–50 μM |
N/A |
CV and DPV |
Wound fluid |
164 |
Nafion/uricase/ZnO/Ag/Si |
UA |
50–2000 μM |
0.019 μM |
CV |
Serum |
165 |
Uricase/tetrapod-shaped ZnO |
UA |
0.8–3490 μM |
0.8 μM |
CV and amp |
Standard sample |
166 |
Nafion/uricase/ferrocene/GCE |
UA |
0.5–60 μM |
0.23 μM |
Amp and DPV |
Blood |
167 |
AsOx/c-MWCNT/PANI/Au |
AA |
2–206 μM |
0.9 μM |
Amp |
Serum substances |
109 |
AuNPs@PANI/CS/GCE |
AA |
20–1600 μM |
8 μM |
CV, amp and DPV |
Standard and real sample |
110 |
Ni@poly-1,5 DAN/GC |
AA |
100–500 μM |
0.010 |
SWV |
Serum |
168 |
Pt/LSG |
AA |
10–890 μM |
6.1 μM |
CV and DPV |
Urine |
94 |
GO/NNO20 |
AA |
30–1100 |
11.3 μM |
CV, chrono amp |
Standard and synthetic sweat |
111 |
GO/NNO100 |
AA |
30–1100 |
3.8 μM |
CV, chrono amp |
Standard and synthetic sweat |
111 |
GF/NiCo2O4 |
AA |
200–750 μM |
50 μM |
CV and DPV |
Serum and urine |
93 |
Transfer tattoo |
AA |
10–50 μM |
N/A |
CV |
Sweat |
169 and 170 |
MNA-PLA/f-MWCNT |
AA |
0–1 × 10−2 M |
180 μM |
DPV |
Dermal |
171 |
Pyox/LSGE |
DA |
0.01–0.5 and 0.5–10 μM |
0.007 μM |
DPV |
Human serum and tap water |
129 |
PANI-WO3/GCE |
DA |
20–300 μM |
0.139 μmol L−1 |
CV, DPV, EIS |
Standard |
125 |
CoO/N-CS-rGO |
DA |
0.5–110 |
0.15 |
CV |
Standard and human serum |
126 |
PEDOT-G-TYR |
DA |
N/A |
101 × 10−9 M |
Amp |
Tear |
124 |
Graphene/PEDOT/TYR |
DA |
N/A |
101 × 10−9 M |
Amp |
Tear |
124 |
AuNPs@PANI/CS/GCE |
DA |
10–1700 μM |
5 μM |
CV, amp and DPV |
Standard and real sample |
110 |
Ni@poly-1,5 DAN/GC |
DA |
100–500 μM |
0.00011 |
SWV |
Serum |
168 |
Pt/rGO paper |
DA |
87 nM to 100 μM |
5 nM |
DPV |
Living cell |
172 |
rGO MEA |
DA |
N/A |
0.1 μM |
DPV |
Sweat |
131 |
GF/NiCo2O4 |
DA |
1–13 μM |
0.1 μM |
CV |
Urine |
93 |
Pt/LSG |
DA |
0.5–56 μM |
0.07 μM |
CV and DPV |
Urine |
94 |
PEDOT/LSG |
DA |
1–150 μM |
0.33 μM |
Amp |
Rat brain |
173 |
GF/NiCo2O4 |
DA |
1–13 μM |
0.1 |
CV and DPV |
Serum and urine |
93 |
Textile-OECT/PEDOT:PSS |
DA |
1–10 × 10−6 |
1 μM |
DPV |
Sweat |
173 |
Textile-OECT/PEDOT:PSS |
AD |
10–100 × 10−6 M |
10 μM |
FET |
Sweat |
173 |
Transfer tattoo |
AA |
10–50 μM |
N/A |
CV |
Sweat |
169 and 170 |
SPCE-PPy-urease |
Urea |
10 μM to 5mM |
8 μM |
Poten |
Sweat |
174 |
AuMNA-P(GMA-co-VFc) |
Urea |
50–2500 × 10−3 |
2.8 μM |
CV |
Transdermal |
174 |
rGO MEA |
Tyramine |
N/A |
3.7 μM |
DPV |
Sweat |
175 |
LEG-CS |
Tyramine |
N/A |
3.6 μM |
DPV |
Sweat |
95 |
E200acryl-CP/catechol-agar |
Tyrosinase |
0.1–0.5 mg mL−1 |
N/A |
Amp |
Transdermal |
176 |
Au/rGO/Au-Pt NP/GOx/Nafion |
Glucose |
0–2.4 × 10−3 M |
5 μM |
CV, amp |
Sweat |
141 |
GOx/Pt-graphite |
Glucose |
0–0.9 mM |
0.01 mM |
Chrono amp |
Human perspiration |
177 |
PANI/TEGO/PVA |
Glucose |
0.2 μM to 10 mM |
0.2 μM |
CV |
Sweat |
177 |
PB/Au-graphene/GOx |
Glucose |
10 μM to 0.7 mM |
10 μM |
CV |
Sweat |
178 |
Pt/Co/NPG/GO |
Glucose |
35 μM to 30 mM |
5 μM |
Amp |
Blood |
179 |
PtAu/rGO-CNT-IL/GP |
Glucose |
0.1–11.6 mM |
80 μM |
Amp |
Blood |
180 |
GO/PB/Gp-hybrid |
Glucose |
0.01–0.7 mM |
10 μM |
Poten |
Sweat |
178 |
Au/graphene/AuNps/GOD |
Glucose |
0–40 mg dL−1 |
0.3 mg dL−1 |
Amp |
ISF |
181 |
LIG/PtNPs |
Glucose |
300 nM to 2.1 mM |
300 nM |
Amp |
Blood |
182 |
rGO/AuPtNPs |
Glucose |
0–2.4 mM |
5 μM |
Amp |
Sweat |
141 |
GOx/Au/MoS2/Au-nanofilm |
Glucose |
500–100 nM |
10 nM |
Amp |
Human serum |
141 |
Uricase/ZnONW |
Glucose |
0.042–0.208 × 10−3 M |
20 μM |
Piezo |
Sweat |
137 |
D-Lactate assay kit |
Glucose |
0–6.3 × 10−3 M |
N/A |
Colo |
Sweat |
140 |
PB ink/chitosan/BSA/GOx |
Glucose |
10–100 × 10−6 M |
3 μM |
Amp |
ISF |
143 |
PB ink/agarose/chitosan/GOx |
Glucose |
0–160 × 10−6 M |
N/A |
Amp |
ISF |
22 |
Alcoxysilanes-PB-GOx |
Glucose |
1 μM-1 mM |
1 μM |
Amp |
Sweat |
183 |
C-PB ink-GOx |
Glucose |
2–10 × 10–3 M |
50 μM |
Amp |
Sweat |
142 |
rGO/PU-Au |
Glucose |
500 × 10−9 to 10 × 10−3 M |
500 × 10−9 M |
CV |
Sweat |
1 |
Au/Pt black-Nafion |
Glucose |
50 × 10−6 to 36 × 10−3 |
50 μM |
Amp |
ISF |
184 |
GOx/Pt NPs/PANI/MEA |
Glucose |
2–12 × 10−3 M |
260 μM |
Amp |
Standard sample |
162 |
GOD/CMC/Microneedles |
Glucose |
0–35 × 10−3 M |
40 μM |
Chrono-amp |
ISF |
185 |
GOx/PANI-PEO/Pt |
Glucose |
1–10 × 10−3 M |
820 μM |
CV |
Standard sample |
186 |
Nafion/GOx/AuNP-PVP-PANI |
Glucose |
0.05–2.25 × 10−3 M |
10 μM |
CV |
Serum |
187 |
GOD/Pt/MWNT-PANI/GCE |
Glucose |
0.003–8.2 M |
1 μM |
Chrono amp |
Standard sample |
188 |
Chitosan-PVA/GOx |
Glucose |
5–50 mg dL−1 |
N/A |
Poten |
Tears |
189 |
Chitosan-PVA/GOx |
Glucose |
0.1–0.6 mM |
N/A |
Poten |
Tears |
190 |
PEGDA |
Glucose |
0–4 × 10−3 M |
1 μM |
CV |
Transdermal |
191 |
Uricase/ZnONW |
Lactate |
0–20 × 10−3 M |
0.1 × 10−3 μM |
Piezo |
Sweat |
137 |
PEGDA |
Lactate |
0–1 × 10−3 M |
1 μM |
CV |
Transdermal |
191 |
GP-MoS2-Cu-LOD |
Lactate |
5–1775 μM |
500 nM |
Amp |
Sweat |
192 |
CNTs/Ti3C2Tx/PB/CFM |
Lactate |
10 μM × 10−3 M |
0.67 μM |
Amp |
Sweat |
193 |
BSA-Lox/SPEES/PES |
Lactate |
0–28 × 10−3 M |
N/A |
Amp |
Sweat |
194 |
AuMN/AuMWCNT/MB |
Lactate |
0.01–0.2 × 10−3 M |
N/A |
Amp |
Transdermal |
195 |
E200acryl-filled CP-PEI-LOx |
Lactate |
0–8 × 10−3 M |
0.42 M |
Amp |
Transdermal |
196 |
GTA/BSA/LOx |
Lactate |
0–1 mM |
N/A |
Amp |
ISF |
197 |
Lactate oxidase |
Lactate |
0.1–0.5 mM |
N/A |
Amp |
Saliva |
198 |
Cat-Fe3O4/rGO |
H2O2 |
3.30 μM to 5.56 mM |
110 nM |
Amp |
PBS |
199 |
GF/AuNS |
H2O2 |
9.4 μM to 13 mM |
1.62 μM |
Amp |
PBS |
200 |
IL-rGO |
H2O2 |
0.1–37.6 μM |
0.01 μ |
Amp |
PBS |
200 |
Au/MnO2/graphene-coated CP |
H2O2 |
0.05–14.2 mM |
2 μM |
Amp |
Cancer cell |
176 |
As the biosensors can be prepared by easy procedure with the benefits of low cost, they are efficient and further very easy-to-use with a variety of areas. Moreover, they are popular for the detection of metabolites and biomolecules. Recently, different kinds of biosensors were applied to detect food-born, clinical or environmental pathogens harmful not only to humans but also to animals.
Uric acid (UA). Uric acid (UA), C5H4N4O3, is usually a human body waste product, this planar, heterocyclic compound is usually produced while the metabolic breakdown of purine nucleotides occurs.91 Glucose oxidase-based NiCo2O4 nanomaterial shows high sensitivity to the glucose sample with a wide range of concentrations from 0.005 mM to 15 mM with a sensitivity of 91.34 mV per decade and also showed a fast response time.92 Later, graphene fibers (GFs) with NiCo2O4 nanowires GF/NiCo2O4 were also reported for UA detection with a liner range of 10–26 μM and LOD of 0.2 μM.93 This novel biosensor can be successfully applied directly as a working electrode for the detection of ascorbic acid, AA (liner range of 200–750 μM and LOD of 50 μM) and dopamine, DA (liner range of 1–13 μM and LOD of 0.1 μM). On DPV profiles at GF/NiCo2O4 electrodes these UA, AA, and DA can be detected at different voltage values.Laser-scribed graphene, LSG, or LSG with platinum94 nanomaterials also may be a good promising and excellent electrode for the detection of UA, AA, and DA compound swith a wide range of applications. The measurement of UA is not limited to urine samples only; various reports show that wearable sweat sensors have great potential for the continuous measurements of this sample.95 They reported a highly sensitive LEG-CS; a laser-engraved graphene-based chemical sensor for the detection of lower concentrations of UA and tyrosine (Tyr). This LEG-CS-based multi-inlet microfluidic module can be an alternative to a wearable microfluidic system, which is made from silicone elastomers and is also complicated and expensive.
For the analysis of uric acid, especially point-of-care (POC) monitoring, M. Yang et al. developed a 3D electrochemical biosensor based on super aligned single wall carbon nanotube SWCNT array immobilized with uricase by the means of a precipitation and crosslinking procedure.96 This biosensor possesses a higher enzyme density, a larger contact area, and showed excellent conductivity after modification with a sensitivity of 518.8 μA (mM cm2)−1, a wide linear range of 100–1000 μM, and a low limit of detection of 1 μM for uric acid in serum samples.
Here, uric acid is catalyzed by uricase on the working electrode and oxidized into allantoin, while producing carbon dioxide and hydrogen peroxide, this reaction was previously described by Numnuam et al.161 group.
|
 | (1) |
The produced H2O2 decomposes on the surface of the electrodes according to eqn (2) and thus the corresponding current is detected.
To date, the development of a biosensor for UA detection offers very high sensitivity and selectivity and is thus highly desirable in different fields of chemistry. Overlapping of the oxidation potentials of different molecules along with providing poor selectivity and reproducibility are major problems facing biosensing detection.97 To overcome these problems, metal oxides,98 noble metals,99 polymers,100 and carbon materials101 were also considered as a candidate for electrode modification.
H. Liu et al.102 showed that the polymer of cellulose (CLC), which is dissolved in [BMIM]Cl, and combined with different functional groups such as –NH2, –SH – grafted poly(3,4-ethylene dioxythiophene) (PEDOT), i.e., PEDOT-MeNH2/CLC and PEDOT-MeSH/CLC electrode might be very useful for the detection of guanine (G) and uric acid (UA), respectively, with good selectivity and detection limits.
However, the tendency of people to visit any clinic is decreasing, and currently, patients visit doctors only after noticeable symptoms. Therefore, treatment at home using a single device such as a wearable or portable device is becoming popular as they are easy to operate and can measure the specific sample very accurately.
X. Wei et al. reported wearable biosensors for non-invasive and real-time monitoring of sweat compositions with high sensitivity and selectively.62 Their developed method described the fabrication of wearable biosensors for the detection of uric acid in artificial sweat samples as depicted in Fig. 5. In this biosensor, a flexible and conductive CNF-worked electrode was used for the detection of the uric acid molecules in artificial sweat samples with good selectivity. It ensured a significant linear correlation between the current output and the concentration of uric acid.
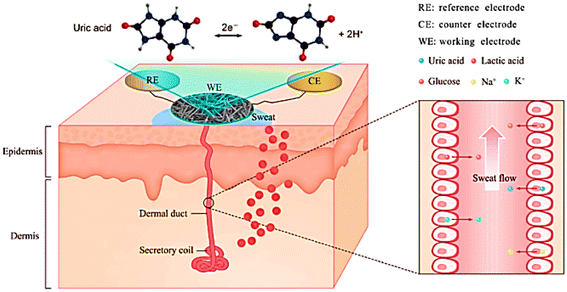 |
| Fig. 5 Schematic illustrations of sweat gland structure, biomarker secretion, and wearable biosensor for uric acid detection in sweat. Adopted from ref. 62 with permission from Elsevier, Copyright© 2021. | |
Ascorbic acid (AA). Ascorbic acid (AA), known as vitamin C, is a readily water-soluble micronutrient that is required for multiple physiological/biological functions in various living organisms. This acid is working as a reducing agent and antioxidant, thus, in cellular metabolism, it can protect cellular components from oxidative damage and various oxidizing free radicals or harmful oxygen-derived species, such as hydroxyl radicals, hydrogen peroxide, and singlet oxygen.103–106 So, the detection of this AA is very important for clinical application as well as to keep the human body fit.The literature revealed various reports for the detection of AA, such as electrochemical methods,107,108 but other molecules such as dopamine can interfere with this type of detection. To solve this problem and for the development of a selective and sensitive method for the determination of AA, conducting polymers107 is highly desirable for analytical and diagnostic applications. A polymer, polyaniline-based biosensor, and ascorbate oxidase (AsOx) immobilized covalently onto carboxylated multiwalled carbon nanotubes, was reported by Chauhan and his team.109 This AsOx/c-MWCNT/PANI/Au electrode was employed for the determination of AA in different samples, human serum, fruit juices, and vitamin C tablets without the interference of serum substances. The electrochemical reaction mechanism for the response is explained by the following equation:109
With this kind of polymer, another biosensor was proposed by L. Yang group.110 Their developed electrode, AuNPs@PANI/CS/GCE showed very excellent catalytic activity and selectivity to the electro-oxidation of DA and AA with peak currents in the range of 10–1700 and 20–1600 μM and detection limits of 5 and 8 μM, respectively.
In a very recent study, Rossato et al. reported111 that, GO/NNO biosensors might be a suitable candidate for the development of flexible and wearable electrochemical devices to use in AA detection with a very simple assembly, fast response, ultra-sensitivity, and low cost. They showed that a laser-induced graphene (GO) electrode with two different NaNiO3 (NNO) nanotubes, distinctive with their external diameter of 20 nm (NNO20) and 100 nm (NNO100) is a very promising candidate for the detection of AA in synthetic sweat. Both electrodes can detect AA in the linear range of 30–1100 μM−1, while the sensitivity of GO/NNO100 (0.031 μA μM−1 cm−2) is significantly better than GO/NNO20 (0.023 μA μM−1 cm−2). According to the sensitivity results, the diameters of NTs seem to affect the sensitivity due to their impact on Ni oxidation, thus affecting the biosensors performance.
Another study by Junlin Ma et al.,112 reported on the development of wearable self-powered textile smart sensors and their effectiveness in smart sensing systems for the portable detection of nutrition with a high sensitivity of 96.6 μA mM−1 cm−2 and a low LOD of 30 μM. They proposed a novel pH-assisted O/W (oil/water) self-assembly system of the bi-functional PANI/reduced graphene oxide (RGO) composite film. On the O/W interface, the composite film (PANI/RGO) exhibited both good capacitive performance and high-performance biosensing properties for AA detection, enabling it to act as a power source for wearable biosensors.
Vitamin C (ascorbic acid-AA) is a known nutrient in the human body needed for the formation of blood vessels, cartilage, muscle, and collagen in bones. It is always important to keep track of the nutrients level in the body. Sweat contains rich chemical information, and further is an attractive bio-fluid for routine assessment of nutrient levels of the human body. For nutritional screening and dietary intervention, a wearable sensor that can selectively measure AA concentration in bio-fluids, including sweat, urine, and blood was developed by Zhao et al.113 On the oral intake of vitamin C, they monitored its concentration increases (compared without intake) in sweat along with urine and blood. They used a conductive polymer, poly (3,4-ethylene dioxythiophene) doped with lithium perchlorate (PEDOT:LiClO4) on the Au surface, encapsulated with Nafion that was characterized by measuring amperometric responses with LOD of ≈4 μm. It showed high selectivity against glucose, lactate, and uric acid in the same measurement. The developed sensor showed a linear response within 0 to 5000 μm with a sensitivity of 1.2 and 2.0 nA μm−1 for bare Au and nano-textured electrodes, respectively.
An epidermal noninvasive bioelectronics wearable biosensor was developed114 for the detection of vitamin C in sweat (AA), realized by immobilizing the enzyme ascorbate oxidase (AAOx) on printable tattoo electrodes. Here, the AAOx enzyme catalyzes the oxidation of AA to dehydroascorbic acid by oxygen. Furthermore, this amount of oxygen consumed by the reaction is directly proportional to the concentration of AA, and the reduction current of the oxygen.115,116 Co-substrate expresses the changes in the AA concentration level by an amperometric method after the intake of vitamin pills and fruit juices, and, the related chemical reaction already discussed earlier, reported by Chauhan et al.109
For the real-time and multiplex sweat analysis such as glucose, lactate, ascorbic acid, uric acid, Na+, and K+ simultaneously, an integrated sensor was developed by Wenya He and his group.117 They used silk fabric derived intrinsically from nitrogen (N) doped carbon (graphitic) textile (SilkNCT), as illustrated in Fig. 6. For high selectivity glucose and lactate sensors, the working electrode was fabricated by drop-casting of glucose oxidase/chitosan and lactate oxidase/chitosan solution onto the Pt/SilkNCT electrode. The good electrical conductivity (electrochemical activity for redox reactions of the molecules) and rich active sites ensured high sensitivity of the AA and UA sensors made of pristine SilkNCT. The reason for this sensitivity can be attributed to N-doping and the hierarchical structure of SilkNCT. While measuring AA and UA, the sensor showed a linear range of 20 to 300 μM and 2.5 to 115 μM, LOD of 1 and 0.1 μM, and a sensitivity of 22.7 and 196.6 nA μM−1, respectively.
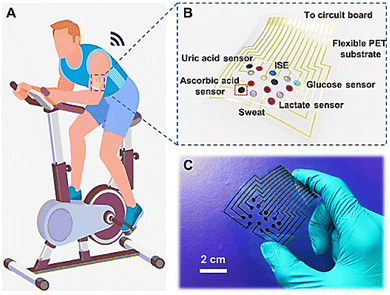 |
| Fig. 6 Wearable sweat analysis patch based on SilkNCT. (A) and (B) Schematic illustration of wearable sweat analysis patch mounted on human skin (A) and the multiplex electrochemical sensor array integrated into the patch (B). (C) Photograph of the wearable sweat analysis patch. Adopted from ref. 117 with permission from the American Association for the Advancement of Science (AAAS), Copyright© 2019. | |
For the measurements of glucose in real time it is always important to measure the accurate amount of glucose. Successive reports were also published reporting different substrates and electrode materials.
Dopamine (DA). Dopamine (DA) is a biomolecule and a key substance, which regulates the body's metabolism, and central neurological systems including mental activity in the human brain and body. If there is any deficiency or excess of DA, it causes various serious disorders and illnesses, including Parkinson's disease, senile, dementia, Harrington's, epilepsy, and schizophrenia.118–123 There are several methods for the detection of DA in the human body.W. Zhang and his group124 showed that the content of DA can be detected by a wearable corneal biosensor. The sensor is prepared by enzyme tyrosinase, and poly (3,4-ethylenedioxythiophene) functionalized with sulfur-doped graphene (PEDOT-G) on a self-designed corneal microelectrode. Here, the sulfur-doped π–π conjugated graphene contributed to a high electroactivity in the resulting microelectrode of PEDOT-G with excellent selectivity and high sensitivity of 12.9 μA × 10−3 m−1 cm−2 and a good LOD of 101 × 10−9 M.
A very recently developed sensor, PANI-WO3/GCE125 proved an excellent sensor for the detection of DA with a good linearity of concentration range of 20–300 μM and a detection limit of 0.139 μmol L−1 and further showed good selectivity.
Clinically applicable diagnostic graphene-based sensor CoO/N-CS-rGO/GCE was reported126 to monitor two analytes, dopamine and uric acid (UA) in human serum with high accuracy. It is an ultrasensitive electrochemical sensor, for DA and UA with a sensitivity and linear range of 1378 and 1393 μA mM−1, and 0.5–110 and 1–125 μM, respectively, with satisfactory stability, and a fast sensing process without pretreatment. The possible oxidation reaction can be expressed as follows127,128
CoO + H2O → CoOOH + H+ + e− |
CoOOH + C8H11NO2 (DA) → CoO + C8H9NO2 (dopamine-o-quinone) |
CoOOH + C5H2N4O3 (UA) → CoO + C5H2N4O3 (dehydrourate) |
Polypyrrole (PPyox) modified laser scribed graphene electrode (LSGE) for DA sensing was successfully developed,129 showing a great potential to be applied in flexible wearable biosensors. This modified electrode is very selectivity to DA in the presence of AA with a lower limit of detection of 7 nM and linearity of 0.5–10 μM, which can be employed for human blood serum and tap water samples, with satisfactory recovery values.
Glucose sensor. A glucose sensor based on a platinum wire and perfluorosulfonic acid polymer-coated enzyme electrodes was developed by Harrison et al. for blood sample.130 Their device showed a linear response from 10 nA to 2 μA with lower LOD <2 nA. For the detection and quantification of blood glucose samples, enzyme-based electrochemical sensors were also developed and some of them were based on invasive blood sampling techniques. Examples of these sensors are, screen-printed carbon (SPC) paste electrodes attached with a glucose oxidase immobilizer and hexamine ruthenium(III) chloride [Ru(NH3)6]3+ containing nitrocellulose electrode,131 thermal biosensor evaluated for the determination of glucose in whole blood by measuring the heat evolved when the glucose sample passed through in a small column with immobilized glucose oxidase with a directly measurable catalase around 1 μL,132 and amperometric glucose sensor on a Prussian blue layer developed based on glucose oxidase immobilized by chitosan for blood sample exhibiting an excellent sensitivity of 98 nA M−1 with a linear range of 0.1–6.0 mM.133 For human perspiration, previously used glucose-oxidase immobilized on Pt-decorated graphite exhibited a low LOD of 10 μM with a linear range between 0 μM and 0.9 mM.134Changes in glucose and norepinephrine levels can be monitored in tear fluid samples by an amperometric rolled thick-film biosensor. The glucose oxidase (GOx) with thick-film carbon working electrode showed a sensitivity range of 20–200 μM for glucose solution,135 while measuring the common electroactive interferences of ascorbic and uric acids can be effectively excluded using polytyramine.136 For the norepinephrine sample, the flow rolled microsensor of copper-clad polyimide contacted with screen-printed band electrodes displays sharp anodic peaks, i.e., the amperometric signals over the 300–900 ppb range, which reflect the rapid and sensitive response to the sample.135
The enzyme/ZnO nanoarray-based piezo biosensor electrode was proposed and named electronic-skin,137 where the surface of ZnO nanowires was modified with lactate oxidase, glucose oxidase, uricase, and urease. This developed electronic skin was a self-powered biosensor and could detect and monitor lactate, glucose, uric acid, and urea in the perspiration in real-time and continuously.137 It is noteworthy that selectivity is an important parameter for biosensors.138
H2O2 → 2H+ + O2 + 2e− (ref. 139) |
Modifier lactate oxidase (LOx), glucose oxidase (GOx), uricase, and urease showed high selectivity against lactate, glucose, uric acid, and urea in perspiration. Furthermore, piezo-based biosensing performance arises from the coupling effect among them. While lactate oxidase can only detect lactate, and the responses against glucose, uric acid, and urea are almost close to zero, which ensures the applications of electronic skin in real samples. The reaction between GOx and glucose can produce gluconic acid and H2O2,138 next H2O2 can increase the surface carrier density by producing H+ and e− (ref. 139) thus affecting the piezoelectric effect.
Another wearable microfluidic device was developed and is capable of monitoring human health conditions by the quantitative chemical analysis of sweat. Their microfluidic device can robustly bond to the skin surface (a small set of sweat glands such that perspiration spontaneously initiates routing of sweat) without mechanical and chemical irritation, which approaches based on a sporadic assessment of blood samples containing glucose or lactate.140
A wearable low-cost electrochemical glucose biosensor comprising a hybrid working electrode Au/rGO/AuPtNP/GOx/Nafion,141 was successfully developed for the determination of glucose levels/concentrations in human sweat by amperometric analysis. The Au and Pt nanoparticles modified on the Au/rGO surface contribute to the increase of the electroactive surface area of the electrode, resulting in the acceleration of electron transfer between the redox probe and the electrode. This sensor showed a good analytical sensitivity of 82 μA mM−1 cm−2 actively with a linear range of 0.1–2.3 mM, demonstrating a sufficient range for glucose sweat detection142 (Fig. 7).
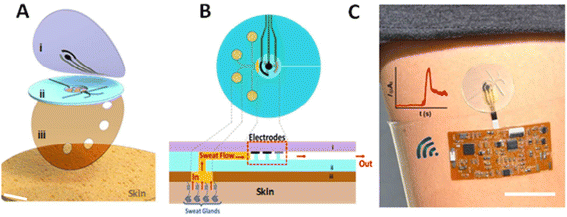 |
| Fig. 7 Microfluidic device design and operation. The soft epidermal microchip device conforms to the skin and routes the sampled sweat toward the electrochemical detector. (A) Schematic representation of layered microfluidic device configuration on skin composed of (i) top PDMS layer with incorporated sensor electrodes, (ii) PDMS microfluidic device, and (iii) adhesive layer on the skin. (B) Schematic representation of microfluidic device sweat collection and operation on the skin in top-down and cross-sectional views. (C) Photograph of microfluidic device integrated with wireless conformal electronics on skin with lithography-based gold current collectors and screen-printed silver–silver chloride (RE) and Prussian blue (WE and CE). Adopted from ref. 142 with permission from American Chemical Society, Copyright© 2019. | |
As such, the detection of glucose in sweat samples is becoming popular. Among these, interstitial fluid (ISF) recently received great attention in connection to the management of diabetes.143–147 For the first time, dual epidermal fluid sampling and detection methods integrated on a single conformal wearable platform22 based on PB ink/agarose/chitosan/GOx, (Prussian blue (PB)) were reported. Furthermore, it showed a wide range of concentration linearity around 0–160 × 10−6 M with 20 × 10−6 M increments and a very selective response to glucose sample concentrations against the electroactive interference compounds. Their developed concept and designed device are capable of noninvasive glucose and alcohol analysis in healthy humans and it further showed excellent correlation to commercial blood glucometer subjecting consumption of food and drink.
Several attempts were reported for the evaluation of glucose concentration detection with painless, convenient, and automated capabilities. For the glucose self-monitoring systems, proposed and developed methods include contact lenses, watches, tattoos, and patches, which collect their information from tears, interstitial fluid, or sweat. Patch-type wearable glucose sensor systems, which are wearable, can be mounted to the human body, and are able to determine the glucose levels in sweat or in ISF fluids with sensing continuously and non-invasively.
Biosensors can be used for the detection of female sex hormone named 17β-estradiol, which is known as a natural and bio-identical form of estrogen. A recent report by Bacchu et al.,148 showed that the biosensor, g-C3N4/APTES/SPE (g-C3N4 = graphitic carbon nitride, APTES = 3-aminopropyltriethoxysilane, SPE = screen-printed electrode) is very effective and can detect estradiol with a wide range of linearity from 1 × 10−6 to 1 × 10−18 mol L−1, LOD of 9.9 × 10−19 mol L−1, high selectivity, and stability. Other biosensors, such as aptamer-based label-free biosensors,149–153 near-infrared (NIR) phosphorescence aptasensor,154 split aptamer regulated CRISPR/Cas12a (CRISPR = Clustered Regularly Interspaced Short Palindromic Repeats, Cas12a = RNA-guided endonuclease) biosensor,155,156 Hydrogel optical waveguide spectroscopy a label-free biosensor,157 highly sensitive laccase-based biosensor, i.e., Lac/rGO-RhNP/GCE electrode,158 Lac/PLLY/CA-GR/GCE,159, (Lac = Laccase, CA-GR = critic acid@graphene, PLLY = poly l-lysine), Impedance-based E-screen cell biosensor160 were reported with excellent performance for different kinds of samples with 17β-estradiol.
From the survey of metabolites and biomolecules, it was found that uric acid detection is more priority on the uricase enzyme modified surface by amperometric technique, ascorbic acid on MWCNT modified surface by differential pulse voltammetric (DPV) technique, dopamine on graphene surface by DPV technique, glucose on glucose oxidase surface by chronoamperometric technique and lactate on lactate oxidase by amperometric technique. Thus, the modified surface materials or receptors and the detection techniques can be counted as selective detections of relative biomolecules.
3.4 Biosensor for protein and immune assay
The key to the innate immune response is the recognition of danger signals or damaged tissue by receptors modified sensor on the surface of the sensor.201 It is the first line of the host defense mechanism against pathogens and harmful substances.202 Recently, it was reported that wearable biosensor receptors can continuously monitor and patrol the host tissues for microbes, damage, and stress, searching for signals of danger and damage.201,203 A literature survey on protein and immune assay is summarized in Table 4.
Table 4 A comparative protein and immune assay study by using wearable electrochemical and biosensora
Sensing material |
Analyte |
Detection range |
Detection limit |
Method of detection |
Bio-fluid |
Ref. |
Abbreviations: Amp-amperometric, EIS-electrochemical impedance spectroscopy, CV-cyclic voltammetry, Conduc-conductometric, FET-field effect transistor, Poten-potentiometric, rGO-reduced graphene oxide, PANI-polyaniline, NRs-nanorods, SPE-screen printed electrode, BSA-bovine serum albumin, NW-nanowires, LBG-laser-burned graphene, PDMS-polydimethyl siloxane, IgG-immunoglobulin G, PASE-1-pyrenebutanoic acid succinimidyl ester, PMMA-polymethyl methacrylate, ssDNA-single-stranded DNA. |
Ti3C2Tx MXene/LBG/PDMS |
Cortisol |
0.01–100 nM |
88 pM |
EIS |
Sweat |
204 |
CCY-Fe2O3-anti-Cmab |
Cortisol |
2.75 × 10−15 to 2.75 × 10−6 M |
1.38 × 10−17 M |
CV |
Sweat |
36 |
ZnO SAM/DTSS/ssDNA |
Cortisol |
1–256 ng mL−1 |
N/A |
EIS |
Sweat |
206 |
Graphene/C-Mab |
Cortisol |
1–40 ng mL−1 |
10 pg mL−1 |
Conduc |
Tears |
207 |
Graphene-Nafion |
Cytokines (IFN-γ) |
0.015–250 nM |
740 fM |
FET |
Sweat |
209 |
Graphene/PMMA/PASE |
Cytokines (IFN-α, IFN-γ) |
N/A |
2.75 PM and 2.89 PM |
FET |
Artificial tears |
210 |
PANI paper based electrode |
IFN-γ |
500–20000 pg mL−1 |
106 pg mL−1 |
EIS |
Serum |
208 |
PANI/G-paper based electrode |
IFN-γ |
5–1000 pg mL−1 |
3.4 pg mL−1 |
EIS |
Serum |
208 |
GFET/PASE or Nafion |
IL-6, TNF-α, IFN-γ |
N/A |
IL-6: 6.11 fM; TNF-α: 6.08 fM., IFN-γ: 4.76 fM |
FET |
Sweat, tears, saliva, serum, urine |
209, 210, 218 and 219 |
AJP graphene IDE/PI |
IFN-γ and IL-10 |
0.1–5 ng mL−1 |
25 pg mL−1 |
EIS |
Serum |
211 |
|
|
0.1–2 ng mL−1 |
46 pg mL−1 |
|
|
|
Graphene/AgNWs/IgG |
MMP-19 |
N/A |
0.74 ng mL−1 |
FET |
Tears |
220 |
AJP graphene IDE/PI |
Histamine |
56.25 μM to 1.8 mM |
30.7 μM |
EIS |
PBS |
221 |
GO film |
Rotavirus |
103–105 |
103 |
CV |
PBS |
222 |
Ag/GOx |
Influenza A |
10 ng mL−1 to 10 μg mL−1 |
10 ng mL−1 |
Amp |
Sweat |
223 |
Au/MoS2/Au/PET |
Gp 120 |
0.1 pg mL−1 to 10 mg mL−1 |
0.066 pg mL−1 |
SWV |
PBS |
224 |
Anti-AFP/chitosan/rGO |
AFP |
0.001–100 ng mL−1 |
0.001 ng mL−1 |
DPV |
PBS |
213 |
Anti-CA125/chitosan/r-GO |
CA125 |
0.001–100 ng mL−1 |
0.001 ng mL−1 |
DPV |
PBS |
213 |
Anti-CA153/chitosan/r-GO |
CA153 |
0.005–100 ng mL−1 |
0.005 ng mL−1 |
DPV |
PBS |
213 |
rGO/Thi/Au NPzs |
CA125 |
0.1–200 UmL |
0.01 U mL |
DPV |
Serum |
213 |
Anti-CEA/chitosan/r-GO |
CEA |
0.005–100 ng mL−1 |
10 pg mL−1 |
DPV |
PBS |
213 |
(NH2-G)/thionine/Au NPs |
CEA standard |
50 pg mL−1 to 500 ng mL−1 |
10 pg mL−1 |
DPV |
PBS |
214 |
BSA/ANTI-FTH/GO/SPGE |
Ferritin |
1–1000 pg mL−1 |
0.19 ng mL−1 |
DPV |
Serum |
225 |
TR-GO |
Anti-IgG |
0.3–7 μg mL−1 |
10 μg mL−1 |
EIS |
PBS |
226 |
rGO/Au NPs |
E. coli |
1.5 × 102 to 1.5 × 107 cfu mL−1 |
1.5 × 102 cfu mL−1 |
EIS |
PBS |
227 |
Graphene/AMP |
E. coli |
N/A |
Single bacterium |
N/A |
Saliva |
228 |
SPE/DTSSP/antibodies |
IL-1β, IL-6, IL-8, IL-10, TNF-α, CRP |
0.2–200 pg mL−1 |
N/A |
EIS |
Sweat |
217 |
SPE/DSP/anti-NPY |
Neuropeptide Y |
10–500 pg mL−1 |
N/A |
EIS |
Sweat |
212 |
GFET/Pi stacked PBASE |
Neuropeptide Y |
1 pM to 10 μM |
N/A |
FET |
Sweat |
215 and 229 |
Au/TNF-α |
Peptides |
0.1 pM to 00.1 μM |
N/A |
Amp |
Human serum |
216 |
Textile/Zn NRs/PANI |
Pesticide |
N/A |
N/A |
Poten |
Body fluid |
217 |
Nah et al., developed a wearable immunosensing path with a microfluidic system for cortisol biomarker detection. They successfully incorporate Ti3C2Tx MXene nanosheets into the porous structure of laser-burned graphene.204 This wearable path system exhibited dynamic range and limit of detection of 0.01–100 nM and 88 pM, respectively. Additionally, conductive carbon yarn (CCY) and Fe2O3 materials were directly deposited onto the working electrode to improve flexible electrochemical biosensors. In this study, anti-Cmab was covalently bound onto the Fe2O3/CCY surface using EDC as a coupling agent and NHS as an activator. The fabricated BSA/anti-Cmab/Fe2O3/CCY electrode was used to measure cortisol concentration from 1 fg to 1 μg in PBS (pH 7.0) by the CV technique.36 Furthermore, highly specific single-stranded DNA (ssDNA) was immobilized onto the ZnO active region to construct a label-free electrochemical sensor for the real-time monitoring of cortisol levels. Here, cortisol is bound with the captured probe, changing the confirmation of the electrochemical signal.205,206 Another contact lens-controlled cortisol monitoring in tears was reported for the mobile-controlled electrochemical platform.207 Where monoclonal antibody was immobilized on the surface of graphene to construct a FET sensor. This process was able to measure low concentrations of cortisol. The schematic representation of the smart contact lens packaging is shown in Fig. 8.
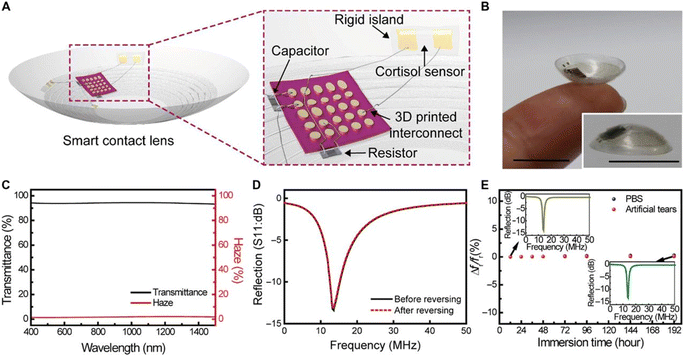 |
| Fig. 8 Wearable contact lens packaging, (A) smart contact lens integrated with three-dimensional interconnects, the sensor on the rigid island. A capacitor and resistor were interconnected for resonance frequency and reference resistance. (B) Fabricated smart contact lens, (C) optical transmittance and haziness of hybrid material, (D) after and before radiation characteristics of the stretchable antenna, (E) relative resonance frequency in PBS and artificial tears up to 192 hours (inset: radiation characteristics of antenna in artificial tears for 12 and 192 hours). Adopted from ref. 208 with permission from Elsevier, Copyright© 2019. | |
The aptamer-based field effect transistor was developed using graphene-Nafion composite film to detect the presence and quantification of cytokine levels by immune sensing human interferon-gamma (IFN-γ). This inflammatory cancer biomarker was collected by adhesive and disposable membrane capsule in sweat.209 Another report demonstrated aptamer functionalized graphene-based field effect transistor (GFET) for immune sensing IFN-α, and IFN-γ. In this study, a 2.5 μm Mylar film was deposited on a glass slide and then the drain, source, and gate electrodes were patterned by lithography process. Thereafter, a graphene sheet in polymethyl methacrylate (PMMA) was transferred onto the electrode, and after dissolving the PMMA layer the graphene was functionalized with the corresponding aptamer of IFN-α, and IFN-γ.210 Additionally, polyaniline was electrodeposited on graphene screen printed paper to increase surface area and immobilize the IFN-γ antibody. The remaining two electrodes were carbon ink and Ag/AgCl ink screened.208 The systematic representation is shown in Fig. 6. The other research work was done with an aerosol-jet-printed graphene-based immunosensor capable of monitoring two distinct cytokines enzymes, IFN-γ and IL-10 with heavy-ranging sensitivity. Here, the IFN-γ and IL-10 antibodies were covalently linked with graphene211 (Fig. 9).
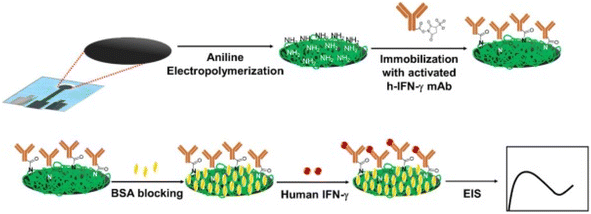 |
| Fig. 9 Fabrication of Human Interferon-gamma (IFN-γ) immunosensor for the detection in serum samples. The figure is adopted from 212 with permission from The Royal Society of Chemistry, Copyright© 2020. | |
Cancer biomarker detection using the paper-based differential pulse voltammetry method was a unique reported work in which the electrode was modified using the corresponding antibodies. In this study, GO was first modified by drop casting method, and then chitosan was dropped on GO film and finally incubated in glutaraldehyde solution to be ready for antibody immobilization. To immobilize the antibody, AFP, CEA, CA125, and CA153 captured antibodies were applied to a glutaraldehyde-modified electrode and after drying, they were ready to be applied in the corresponding antigen in PBS solution.213 Furthermore, the NH2-G/Thi/AuNPs nanocomposite was modified on a carbon working electrode and then anti-CEA was fabricated on an amine-functionalized modified electrode.214
Pancreatic polypeptide Neuropeptide Y (NPY) detection is certainly vital for immune sensing because it maintains essential biological processes in the human body. Kodjo et al. reported the detection of the neuropeptide Y using anti-neuropeptide Y-modified electrodes in sweat samples.212 The modified electrode showed an ultrasensitive linear range of 10–500 pg mL−1. Also, a graphene-based field effect transistor (GFET) was another electrode functionalized with anti-NPY and finally was tested in sweat and saliva samples.215 Additionally, the gold working electrode was carboxylate functionalized to immobilize the TNF-α antibody and thereafter trialed in PBS and sweat sample to measure TNF-α protein.216,217 In this literature survey, it was concluded that antigen detection showed an excellent response with the corresponding antibody-modified electrode.
4. Present challenges and future prospects
Over the last few decades, carbon nanomaterials, metal nanomaterials, polymer nanomaterials, and bio-recognized modified electrodes brought great attention to electrochemical sensors and biosensors in human body fluidic components. There are several challenges that need to be addressed before the realization of wearable flexible electronics, especially for the physical and chemical sensors.
Most recent wearable electrochemical and bio-sensing devices can measure a limited range of biomarkers. The multi-analyte measuring is essential for tracking health under several dynamic conditions, and up to date, all efforts are continued toward single and simultaneous monitoring. Additionally, the most accurate and reliable electrochemical responses are the desires of modern electroanalytical chemistry. Accuracy often hinders bio-fouling effects through non-specific binding, potential contamination from the surroundings, and signal drift (sensor calibration). Another common wireless electronic printed circuit board is used as a flexible wireless platform. Update is necessary and it can be performed by establishing a high level of integration with the biosensor platform. Furthermore, according to the market analysis report (report ID: GVR-2-68038-154-2), the global wearable sensor market value will reach $2.86 billion by 2025. This market price majorly depends on the wearable biosensor platform successfully integrated with the electrode modification material selection and finding out proper fabrication routes.
Proper installation of mobile devices and smartphone-based wireless platforms, including algorithm-based applications, is expected to facilitate the successful translation of wearable biosensors and proof-of-concept demonstration. Moreover, wearable biosensor technologies can improve human health and performance by monitoring and taking physiological treatment in human dynamic life. Furthermore, radiofrequency or bluetooth-based identification with wearable physical and chemical sensors will facilitate data transmission from users to cellphones/computers.230–232 The goal is to make a wearable flexible, decomposable, low-cost, high-performance, reliable, catalytic, highly conductive, and porous nanostructured working electrode with a suitable wireless installation that can address the demand of the next generation. Such future wearable electrochemical sensors and biosensors will non-invasively monitor a wide range of biomarkers including ions, drugs and toxins, metabolites and biomarkers, proteins and immune assays. These advances will reach a multidisciplinary collaboration in nano-engineering, bioengineering, electronics, and medical communications.
5. Conclusions
Wearable sensor technology is evolving in a remarkable way. The ability of these devices to extract quantitative and innovative information in real-time, especially selective detection with limited funds, enhanced the advancements in fields such as nanotechnology and internet-based point of care, revolutionizing user health, well-being, and safety practices. In electrochemical sensors, the production of these wearable platforms has drawn significant attention to wearable approaches. This is due to the fact that such techniques enable the production of fully integrated devices in a miniature, adaptable, and durable manner, enabling direct analysis of the human body and processing the wireless data transmission to a portable device. Although wearable sensors have largely been developed for health and fitness purposes, these technologies are crucial for many different scientific and industrial domains. From the perspective of practical applications and commercialization, little attention has been given to crucial and fundamental processes that will lead to the device's ultimate goal of leading smart life.
Conflicts of interest
There is no conflict of interest.
References
- P. T. Toi, T. Q. Trung, T. M. L. Dang, C. W. Bae and N.-E. Lee, Highly Electrocatalytic, Durable, and Stretchable Nanohybrid Fiber for On-Body Sweat Glucose Detection, ACS Appl. Mater. Interfaces, 2019, 11(11), 10707–10717, DOI:10.1021/acsami.8b20583.
- A. Furniturewalla, M. Chan, J. Sui, K. Ahuja and M. Javanmard, Fully Integrated Wearable Impedance Cytometry Platform on Fl Exible Circuit Board with Online Smartphone Readout, Microsyst. Nanoeng., 2018, 4(1), 20, DOI:10.1038/s41378-018-0019-0.
- J. Xu, Y. Fang and J. Chen, Wearable biosensors for non-invasive sweat diagnostics, Biosensors, 2021, 11(8), 245, DOI:10.3390/bios11080245.
- M. Mathew, S. Radhakrishnan, A. Vaidyanathan, B. Chakraborty and C. S. Rout, Flexible and wearable electrochemical biosensors based on two-dimensional materials: recent developments, Anal. Bioanal. Chem., 2021, 413, 727–762, DOI:10.1007/s00216-020-03002-y.
- Z. Zhou, K. Chen, X. Li, S. Zhang, Y. Wu, Y. Zhou, K. Meng, C. Sun, Q. He, W. Fan and E. Fan, Sign-to-speech translation using machine-learning-assisted stretchable sensor arrays, Nat. Electron., 2020, 3(9), 571–578, DOI:10.1038/s41928-020-0428-6.
- Y. Zou, A. Libanori, J. Xu, A. Nashalian and J. Chen, Triboelectric nanogenerator enabled smart shoes for wearable electricity generation, Research, 2020 DOI:10.34133/2020/7158953.
- M. A. P. Mahmud, A. Zolfagharian, S. Gharaie, A. Kaynak, S. H. Farjana, A. V. Ellis, J. Chen and A. Z. Kouzani, 3D-Printed Triboelectric Nanogenerators: State of the Art, Applications, and Challenges, Adv. Energy Sustainability Res.n.d., 2021, 99–102, DOI:10.1002/aesr.202000045.
- K. Meng, S. Zhao, Y. Zhou, Y. Wu, S. Zhang, Q. He, X. Wang, Z. Zhou, W. Fan, X. Tan, J. Yang and J. Chen, A wireless textile-based sensor system for self-powered personalized health care, Matter, 2020, 2(4), 896–907, DOI:10.1016/j.matt.2019.12.025.
- G. Chen, Y. Li, M. Bick and J. Chen, Smart Textiles for Electricity Generation, Chem. Rev., 2020, 120(8), 3668–3720, DOI:10.1021/acs.chemrev.9b00821.
- Z. Liu, H. Li, B. Shi, Y. Fan, Z. L. Wang and Z. Li, Wearable and Implantable Triboelectric Nanogenerators, Adv. Funct. Mater., 2019, 29(20), 1808820, DOI:10.1002/adfm.201808820.
- S. S. Kwak, H. Yoon and S. Kim, Textile-Based Triboelectric Nanogenerators for Self-Powered Wearable Electronics, Adv. Funct. Mater., 2018, 29(2), 1804533, DOI:10.1002/adfm.201804533.
- X. Chen, Y. Song, Z. Su, H. Chen, X. Cheng, J. Zhang, M. Han and H. Zhang, Flexible fiber-based hybrid nanogenerator for biomechanical energy harvesting and physiological monitoring, Nano Energy, 2017, 38, 43–50, DOI:10.1016/j.nanoen.2017.05.047.
- Y. Song, H. Wang, X. Cheng, G. Li, X. Chen, H. Chen, L. Miao, X. Zhang and H. Zhang, Traditional weaving craft for one-piece self-charging power textile for wearable electronics, Nano Energy, 2019, 55, 29–36, DOI:10.1016/j.nanoen.2018.10.045.
- P. Abiri, S. Duarte-Vogel, T. C. Chou, A. Abiri, V. Gudapati, A. Yousefi, M. Roustaei, C. C. Chang, Q. Cui, J. J. Hsu and M. Bersohn, In Vivo intravascular pacing using a wireless microscale stimulator, Ann. Biomed. Eng.n.d., 2021, 1–9, DOI:10.1007/s10439-021-02729-8.
- G. Chen, Y. Fang, X. Zhao, T. Tat and J. Chen, Textiles for Learning Tactile Interactions, Nat. Electron., 2021, 4, 175–176, DOI:10.1038/s41928-021-00560-6.
- E. Davoodi, H. Montazerian, R. Haghniaz, A. Rashidi, S. Ahadian, A. Sheikhi, J. Chen, A. Khademhosseini, A. S. Milani, M. Hoorfar and E. Toyserkani, 3D-printed ultra-robust surface-doped porous silicone sensors for wearable biomonitoring, ACS Nano, 2020, 14(2), 1520–1532, DOI:10.1021/acsnano.9b06283.
- S. Ye, S. Feng, L. Huang and S. Bian, Recent Progress in Wearable Biosensors: From Healthcare Monitoring to Sports Analytics, Biosensors, 2020, 205, DOI:10.3390/bios10120205.
- A. J. Bandodkar and J. Wang, Non-Invasive Wearable Electrochemical Sensors : A Review, Trends Biotechnol., 2014, 1–9, DOI:10.1016/j.tibtech.2014.04.005.
- R. Fan and T. L. Andrew, Perspective—Challenges in developing wearable electrochemical sensors for longitudinal health monitoring, J. Electrochem. Soc., 2020, 167 DOI:10.1149/1945-7111/ab67b0.
- Y. Yang and W. Gao, Wearable and flexible electronics for continuous molecular monitoring, Chem. Soc. Rev., 2019, 48(6), 1465–1491, 10.1039/C7CS00730B.
- B. Wang and A. Facchetti, Mechanically Flexible Conductors for Stretchable and Wearable E-Skin and E-Textile Devices, Adv. Mater., 2019, 31(28), 1901408, DOI:10.1002/adma.201901408.
- J. Kim, J. R. Sempionatto, S. Imani, M. C. Hartel, A. Barfidokht, G. Tang, A. S. Campbell, P. P. Mercier and J. Wang, Simultaneous monitoring of sweat and interstitial fluid using a single wearable biosensor platform, Adv. Sci., 2018, 5(10), 1800880, DOI:10.1002/advs.201800880.
- J. Kim, R. Kumar, A. J. Bandodkar and J. Wang, Advanced Materials for Printed Wearable Electrochemical Devices: A Review, Adv. Electron. Mater., 2017, 3(1), 1600260, DOI:10.1002/aelm.201600260.
- M. Gao, L. Li and Y. Song, Inkjet Printing Wearable Electronic Devices, J. Mater. Chem. C, 2017, 5(12), 2971–2993, 10.1039/C7TC00038C.
- H. Wang, F. Teng, L. Zhang, Q. Zhang, H. Zhang, T. Pei, S. Li and L. Xia, Meso-cellular silicate foam-modified reduced graphene oxide with a sandwich structure for enzymatic immobilization and bioelectrocatalysis, ACS Appl. Mater. Interfaces, 2019, 11(33), 29522–29535, DOI:10.1021/acsami.9b08569.
- M. Moyo, J. O. Okonkwo and N. M. Agyei, Recent advances in polymeric materials used as electron mediators and immobilizing matrices in developing enzyme electrodes, Sensors, 2012, 12(1), 923–953, DOI:10.3390/s120100923.
- T. Ghosh, H. J. Chung and J. Rieger, All-solid-state sodium-selective electrode with a solid contact of chitosan/prussian blue nanocomposite, Sensors, 2017, 17(11), 2536, DOI:10.3390/s17112536.
- E. Singh, M. Meyyappan and H. S. Nalwa, Flexible graphene-based wearable gas and chemical sensors, ACS Appl. Mater. Interfaces, 2017, 9(40), 34544–34586, DOI:10.1021/acsami.7b07063.
- D. Zhai, B. Liu, Y. Shi, L. Pan, Y. Wang, W. Li, R. Zhang and G. Yu, Highly sensitive glucose sensor based on Pt nanoparticle/polyaniline hydrogel heterostructures, ACS Nano, 2013, 7(4), 3540–3546 CrossRef CAS PubMed.
- J. Heikenfeld, A. Jajack, J. Rogers, P. Gutruf, L. Tian, T. Pan, R. Li, M. Khine, J. Kim and J. Wang, Wearable sensors: modalities, challenges, and prospects, Lab Chip, 2018, 18(2), 217–248, 10.1039/C7LC00914C.
- A. Herrera-chacón, X. Cetó and M. Del Valle, Molecularly Imprinted Polymers-towards Electrochemical Sensors and Electronic Tongues, Anal. Bioanal. Chem., 2021, 413, 6117–6140 CrossRef PubMed.
- A. N. Kozitsina, T. S. Svalova, N. N. Malysheva, A. V. Okhokhonin, M. B. Vidrevich and K. Z. Brainina, Sensors Based on Bio and Biomimetic Receptors in Medical Diagnostic, Environment, and Food Analysis, Biosensors, 2018, 8 DOI:10.3390/bios8020035.
- E. B. Bahadır and M. K. Sezgintürk, A review on impedimetric biosensors, Artif. Cells, Nanomed., Biotechnol., 2016, 44(1), 248–262, DOI:10.3109/21691401.2014.942456.
- L. Wang, L. Wang, Y. Zhang, J. Pan, S. Li, X. Sun, B. Zhang and H. Peng, Weaving Sensing Fibers into Electrochemical Fabric for Real-Time Health Monitoring, Adv. Funct. Mater., 2018, 28(42), 1804456, DOI:10.1002/adfm.201804456.
- Y. Zhao, Q. Zhai, D. Dong, T. An, S. Gong, Q. Shi and W. Cheng, Highly stretchable and strain-insensitive fiber-based wearable electrochemical biosensor to monitor glucose in the sweat, Anal. Chem., 2019, 91(10), 6569–6576, DOI:10.1021/acs.analchem.9b00152.
- M. Sekar, M. Pandiaraj, S. Bhansali, N. Ponpan and C. Viswanathan, Carbon Fiber Based Electrochemical Sensor for Sweat Cortisol Measurement, Sci. Rep., 2019, 9(1), 403, DOI:10.1038/s41598-018-37243-w.
- M. Cuartero, M. Parrilla and G. A. Crespo, Wearable potentiometric sensors for medical applications, Sensors, 2019, 19(2), 363, DOI:10.3390/s19020363.
- G. Acar, O. Ozturk, A. J. Golparvar, T. A. Elboshra, K. Böhringer and M. K. Yapici, Wearable and Flexible Textile Electrodes for Biopotential Signal Monitoring: A Review, Electronics, 2019, 8(5), 479 CrossRef CAS.
- A. Kiaghadi, S. Z. Homayounfar, J. Gummeson, T. Andrew and D. Ganesan, Phyjama: Physiological Sensing via Fiber-Enhanced Pyjamas, Proc. ACM Interact. Mob. Wearable Ubiquitous Technol., 2019, 3(3), 1–29 CrossRef.
- J. Kim, M. Kim, M. Lee, K. Kim, S. Ji, Y. Kim, J. Park, K. Na, K. Bae, H. K. Kim, F. Bien, C. Y. Lee and J. Park, Contact Lenses for Wireless Ocular Diagnostics, Nat. Commun., 2017, 1–8, DOI:10.1038/ncomms14997.
- S. Feng, R. Caire, B. Cortazar, M. Turan, A. Wong and A. Ozcan, Immunochromatographic Diagnostic Test Analysis Using Google Glass, ACS Nano, 2014, 8(3), 3069–3079 CrossRef CAS.
- H. Yao, A. J. Shum, M. Cowan, I. Lähdesmäki and B. A. Parviz, Biosensors and Bioelectronics A Contact Lens with Embedded Sensor for Monitoring Tear Glucose Level, Biosens. Bioelectron., 2011, 26(7), 3290–3296, DOI:10.1016/j.bios.2010.12.042.
- Y. L. Yang, M. C. Chuang, S. L. Lou and J. Wang, Thick-film textile-based amperometric sensors and biosensors, Analyst, 2010, 135(6), 1230–1234, 10.1039/b926339j.
- T. Guinovart, M. Parrilla, G. A. Crespo, F. X. Rius and F. J. Andrade, Potentiometric sensors using cotton yarns, carbon nanotubes and polymeric membranes, Analyst, 2013, 138(18), 5208–5215, 10.1039/c3an00710c.
- J. R. Windmiller, A. J. Bandodkar, S. Parkhomovsky and J. Wang, Stamp transfer electrodes for
electrochemical sensing on non-planar and oversized surfaces, Analyst, 2012, 137(7), 1570–1575, 10.1039/c2an35041f.
- W. Jia, A. J. Bandodkar, G. Valdés-Ramírez, J. R. Windmiller, Z. Yang, J. Ramírez, G. Chan and J. Wang, Electrochemical Tattoo Biosensors for Real-Time Noninvasive Lactate Monitoring in Human Perspiration, Anal. Chem., 2013, 85(14), 6553–6560, DOI:10.1021/ac401573r.
- J. R. Windmiller, A. G. Martinez, J. Ram, G. Chan, K. Kerman and J. Wang, Tattoo-Based Potentiometric Ion-Selective Sensors for Epidermal PH Monitoring, Analyst, 2013, 123–128, 10.1039/c2an36422k.
- T. Guinovart, A. J. Bandodkar, J. R. Windmiller, F. J. Andrade and J. Wang, A potentiometric tattoo sensor for monitoring ammonium in sweat, Analyst, 2013, 138(22), 7031–7038, 10.1039/c3an01672b.
- J. Zhao, Y. Lin, J. Wu, H. Y. Y. Nyein, M. Bariya, L. C. Tai, M. Chao, W. Ji, G. Zhang, Z. Fan and A. Javey, A fully integrated and self-powered smartwatch for continuous sweat glucose monitoring, ACS Sens., 2019, 4(7), 1925–1933, DOI:10.1021/acssensors.9b00891.
- J. Choi, D. Kang, S. Han, S. B. Kim and J. A. Rogers, Thin, Soft, Skin-Mounted Microfluidic Networks with Capillary Bursting Valves for Chrono-Sampling of Sweat, Adv. Healthcare Mater., 2017, 6(5), 1601355, DOI:10.1002/adhm.201601355.
- J. Choi, R. Ghaffari, L. B. Baker and J. A. Rogers, Skin-Interfaced Systems for Sweat Collection and Analytics, Sci. Adv., 2018, 4(2), 3921 CrossRef PubMed.
- J. Kim, P. Gutruf, A. M. Chiarelli, S. Y. Heo, K. Cho, Z. Xie, A. Banks, S. Han, K. I. Jang, J. W. Lee and K. T. Lee, Miniaturized Battery-Free Wireless Systems for Wearable Pulse Oximetry, Adv. Funct. Mater., 2017, 27(1), 1604373, DOI:10.1002/adfm.201604373.
- L. A. Mercante, R. S. Andre, M. H. M. Facure, L. Fugikawa-Santos and D. S. Correa, Design of a Bioelectronic Tongue for Glucose Monitoring Using Zinc Oxide Nanofibers and Graphene Derivatives, Sens. Actuators Rep., 2021, 3, 100050, DOI:10.1016/j.snr.2021.100050.
- T. Arakawa, K. Tomoto, H. Nitta, K. Toma, S. Takeuchi, T. Sekita, S. Minakuchi and K. Mitsubayashi, A wearable cellulose acetate-coated mouthguard biosensor for in vivo salivary glucose measurement, Anal. Chem., 2020, 92(18), 12201–12207, DOI:10.1021/acs.analchem.0c01201.
- J. Gonzalo-Ruiz, R. Mas, C. de Haro, E. Cabruja, R. Camero, M. A. Alonso-Lomillo and F. J. Munoz, Early determination of cystic fibrosis by electrochemical chloride quantification in sweat, Biosens. Bioelectron., 2009, 24(6), 1788–1791, DOI:10.1016/j.bios.2008.07.051.
- X. Kang, J. Zhang, Z. Shao, G. Wang, X. Geng, Y. Zhang and H. Zhang, A Wearable and Real-Time Pulse Wave Monitoring System Based on a Flexible Compound Sensor, Biosensors, 2022, 133 CrossRef CAS.
- A. J. Bandodkar, D. Molinnus, O. Mirza, T. Guinovart, J. R. Windmiller, G. Valdés-Ramírez, F. J. Andrade, M. J. Schöning and J. Wang, Epidermal Tattoo Potentiometric Sodium Sensors with Wireless Signal Transduction for Continuous Non-Invasive Sweat Monitoring, Biosens. Bioelectron., 2014, 54, 603–609, DOI:10.1016/j.bios.2013.11.039.
- T. Guinovart, G. A. Crespo, F. X. Rius and F. J. Andrade, A reference electrode based on polyvinyl butyral (PVB) polymer for decentralized chemical
measurements, Anal. Chim. Acta, 2014, 821, 72–80, DOI:10.1016/j.aca.2014.02.028.
- J. Bobacka, Potential Stability of All-Solid-State Ion-Selective Electrodes Using Conducting Polymers as Ion-to-Electron Transducers, Anal. Chem., 1999, 71(21), 4932–4937 CrossRef CAS PubMed.
- S. Emaminejad, W. Gao, E. Wu, Z. A. Davies, H. Yin Yin Nyein, S. Challa, S. P. Ryan, H. M. Fahad, K. Chen, Z. Shahpar and S. Talebi, Autonomous sweat extraction and analysis applied to cystic fibrosis and glucose monitoring using a fully integrated wearable platform, Proc. Natl. Acad. Sci. U. S. A., 2017, 114(18), 4625–4630, DOI:10.1073/pnas.1701740114.
- S. Wang, Y. Wu, Y. Gu, T. Li, H. Luo, L. Li, Y. Bai, L. Li, L. Liu, Y. Cao and H. Ding, Wearable sweatband sensor platform based on gold nanodendrite array as efficient solid contact of ion-selective electrode, Anal. Chem., 2017, 89(19), 10224–10231, DOI:10.1021/acs.analchem.7b01560.
- X. Wei, M. Zhu, J. Li, L. Liu, J. Yu, Z. Li and B. Ding, Nano Energy Wearable Biosensor for Sensitive Detection of Uric Acid in Artificial Sweat Enabled by a Fiber Structured Sensing Interface, Nano Energy, 2021, 85, 106031, DOI:10.1016/j.nanoen.2021.106031.
- G. Xu, C. Cheng, Z. Liu, W. Yuan, X. Wu, Y. Lu, S. S. Low, J. Liu, L. Zhu, D. Ji and S. Li, Battery-free and wireless epidermal electrochemical system with all-printed stretchable electrode array for multiplexed in situ sweat analysis, Adv. Mater. Technol., 2019, 4(7), 1800658, DOI:10.1002/admt.201800658.
- T. Raza, L. Qu, W. A. Khokhar, B. Andrews, A. Ali and M. Tian, Progress of Wearable and Flexible Electrochemical Biosensors With the Aid of Conductive Nanomaterials, Front. Bioeng. Biotechnol., 2021, 9, 761020, DOI:10.3389/fbioe.2021.76102.
- T. Guinovart, G. Valdés-Ramírez, J. R. Windmiller, F. J. Andrade and J. Wang, Bandage-based wearable potentiometric sensor for monitoring wound pH, Electroanalysis, 2014, 26(6), 1345–1353, DOI:10.1002/elan.201300558.
- C. Shan, H. Yang, J. Song, D. Han, A. Ivaska and L. Niu, Direct Electrochemistry of Glucose Oxidase and Biosensing for Glucose Based on Graphene, Anal. Chem., 2009, 81(6), 2378–2382, DOI:10.1021/ac802193c.
- C. Zhang, J. Ning, B. Wang, H. Guo, X. Feng, X. Shen, Y. Jia, J. Dong, D. Wang, J. Zhang and Y. Hao, Hybridized 1T/2H-MoS2/graphene fishnet tube for high-performance on-chip integrated micro-systems comprising supercapacitors and gas sensors, Nano Res., 2021, 14, 114–121 CrossRef CAS.
- W. Gao, S. Emaminejad, H. Yin, Y. Nyein, S. Challa, K. Chen, A. Peck, H. M. Fahad, H. Ota, H. Shiraki, D. Kiriya, D. Lien and G. A. Brooks, Multiplexed In Situ Perspiration Analysis, Nature, 2016, 529(7587), 509–514, DOI:10.1038/nature16521.
- K. Ogasawara, T. Tsuru, K. Mitsubayashi and I. Karube, Electrical conductivity of tear fluid in healthy persons and keratoconjunctivitis sicca patients measured by a flexible conductimetric sensor, Graefe’s Arch. Clin. Exp. Ophthalmol., 1996, 234, 542–546 CrossRef CAS PubMed.
- H. Lee, T. K. Choi, Y. B. Lee, H. R. Cho, R. Ghaffari, L. Wang, H. J. Choi, T. D. Chung, N. Lu, T. Hyeon and S. H. Choi, A graphene-based electrochemical device with thermoresponsive microneedles for diabetes monitoring and therapy, Nat. Nanotechnol., 2016, 11(6), 566–572, DOI:10.1038/nnano.2016.38.
- J. H. Yoon, S. M. Kim, Y. Eom, J. M. Koo, H. W. Cho, T. J. Lee, K. G. Lee, H. J. Park, Y. K. Kim, H. J. Yoo and S. Y. Hwang, Extremely fast self-healable bio-based supramolecular polymer for wearable real-time sweat-monitoring sensor, ACS Appl. Mater. Interfaces, 2019, 11(49), 46165–46175, DOI:10.1021/acsami.9b16829.
- P. K. Kannan, D. J. Late, H. Morgan and C. S. Rout, Recent Developments in 2D Layered Inorganic Nanomaterials for Sensing, Nanoscale, 2015, 7(32), 13293–13312, 10.1039/C5NR03633J.
- A. J. Bandodkar, I. Jeerapan, J. M. You, R. Nuñez-Flores and J. Wang, Highly Stretchable Fully-Printed CNT-Based Electrochemical Sensors and Biofuel Cells: Combining Intrinsic and Design-Induced Stretchability, Nano Lett., 2016, 16(1), 721–727, DOI:10.1021/acs.nanolett.5b04549.
- T. Guinovart, M. Parrilla, G. A. Crespo, F. X. Rius and F. J. Andrade, Potentiometric sensors using cotton yarns, carbon nanotubes and polymeric membranes, Analyst, 2013, 138(18), 5208–5215, 10.1039/c3an00710c.
- J. Tang, X. Liu, C. Yang, Z. Zhang, R. Sun, H. Li, C. Li and F. Wang, A Carbon-Rich Nanofiber Framework Based on a Conjugated Arylacetylene Polymer for Photocathodic Enzymatic Bioanalysis, RSC Adv., 2019, 9(72), 42533–42542, 10.1039/c9ra09157b.
- L. Manjakkal, W. Dang, N. Yogeswaran and R. Dahiya, Textile-Based Potentiometric Electrochemical pH Sensor for Wearable Applications, Biosensors, 2019, 9(1), 14 CrossRef CAS PubMed.
- W. Gao, H. Y. Nyein, Z. Shahpar, H. M. Fahad, K. Chen, S. Emaminejad, Y. Gao, L. C. Tai, H. Ota, E. Wu and J. Bullock, Wearable microsensor array for multiplexed heavy metal monitoring of body fluids, ACS Sens., 2016, 1(7), 866–874, DOI:10.1021/acssensors.6b00287.
- H. Graf and H. R. Mühlemann, Oral telemetry of fluoride ion activity, Arch. Oral Biol., 1969, 14(3), 259–263 CrossRef CAS PubMed.
- Q. An, S. Gan, J. Xu, Y. Bao, T. Wu, H. Kong and L. Zhong, Electrochemistry Communications Full Communication A Multichannel Electrochemical All-Solid-State Wearable Potentiometric Sensor for Real-Time Sweat Ion Monitoring, Electrochem. Commun., 2019, 107, 106553, DOI:10.1016/j.elecom.2019.106553.
- H. Y. Y. Nyein, W. Gao, Z. Shahpar, S. Emaminejad, S. Challa, K. Chen, H. M. Fahad, L. C. Tai, H. Ota, R. W. Davis and A. Javey, A wearable electrochemical platform for noninvasive simultaneous monitoring of Ca2+ and pH, ACS Nano, 2016, 10(7), 7216–7224, DOI:10.1021/acsnano.6b04005.
- T. Guinovart, A. J. Bandodkar, J. R. Windmiller, F. J. Andrade and J. Wang, A potentiometric tattoo sensor for monitoring ammonium in sweat, Analyst, 2013, 138(22), 7031–7038 RSC.
- L. C. Tai, W. Gao, M. Chao, M. Bariya, Q. P. Ngo, Z. Shahpar, H. Y. Nyein, H. Park, J. Sun, Y. Jung and E. Wu, Methylxanthine drug monitoring with wearable sweat sensors, Adv. Mater., 2018, 30(23), 1707442, DOI:10.1002/adma.201707442.
- S. Bian, B. Zhu, G. Rong and M. Sawan, Towards Wearable and Implantable Continuous Drug Monitoring: A Review, J. Pharm. Anal., 2021, 11(1), 1–14, DOI:10.1016/j.jpha.2020.08.001.
- B. Zanfrognini, L. Pigani and C. Zanardi, Recent Advances in the Direct Electrochemical Detection of Drugs of Abuse, J. Solid State Electrochem., 2020, 24, 2603–2616 CrossRef CAS.
- L. C. Tai, T. S. Liaw, Y. Lin, H. Y. Nyein, M. Bariya, W. Ji, M. Hettick, C. Zhao, J. Zhao, L. Hou and Z. Yuan, Wearable sweat band for noninvasive levodopa monitoring, Nano Lett., 2019, 19(9), 6346–6351, DOI:10.1021/acs.nanolett.9b02478.
- K. Y. Goud, C. Moonla, R. K. Mishra, C. Yu, R. Narayan, I. Litvan and J. Wang, Wearable Electrochemical Microneedle Sensor for Continuous Monitoring of Levodopa: Toward Parkinson Management, ACS Sens., 2019, 4(8), 2196–2204, DOI:10.1021/acssensors.9b01127.
- K. Y. Goud, C. Moonla, R. K. Mishra, C. Yu, R. Narayan, I. Litvan and J. Wang, Wearable electrochemical microneedle sensor for continuous monitoring of levodopa: toward Parkinson management, ACS Sens., 2019, 4(8), 2196–2204, DOI:10.1021/acssensors.9b01127.
- A. Bhide, S. Muthukumar, A. Saini and S. Prasad, Simultaneous Lancet-Free Monitoring of Alcohol and Glucose from Low-Volumes of Perspired Human Sweat, Sci. Rep., 2018, 1–11, DOI:10.1038/s41598-018-24543-4.
- J. Kim, I. Jeerapan, S. Imani, T. N. Cho, A. Bandodkar, S. Cinti, P. P. Mercier and J. Wang, Non-Invasive Alcohol Monitoring Using a Wearable Tattoo-Based Iontophoretic-Biosensing System, ACS Sens., 2016, 1(8), 1011–1019, DOI:10.1021/acssensors.6b00356.
- Y. C. Kim, J. H. Park and M. R. Prausnitz, Microneedles for drug and vaccine delivery, Adv. Drug Delivery Rev., 2012, 64(14), 1547–1568, DOI:10.1016/j.addr.2012.04.005.
- S. Pradhan, S. Pramanik, D. K. Das, R. Bhar, R. Bandyopadhyay, P. Millner and P. Pramanik, Nanosized Iron Telluride for Simultaneous Nanomolar Voltammetric Determination of Dopamine, Uric Acid, Guanine and Adenine, New J. Chem., 2019, 43(26), 10590–10600, 10.1039/C9NJ02329A.
- M. Hussain, Z. H. Ibupoto, M. A. Abbasi, X. Liu, O. Nur and M. Willander, Synthesis of Three Dimensional Nickel Cobalt Oxide Nanoneedles on Nickel Foam, Their Characterization and Glucose Sensing Application, Sensors, 2014, 5415–5425, DOI:10.3390/s140305415.
- W. Cai, J. Lai, T. Lai, H. Xie and J. Ye, Controlled Functionalization of Flexible Graphene Fibers for the Simultaneous Determination of Ascorbic Acid, Dopamine and Uric Acid, Sens. Actuators, B, 2016, 224, 225–232, DOI:10.1016/j.snb.2015.09.079.
- P. Nayak, N. Kurra, C. Xia and H. N. Alshareef, Highly Efficient Laser Scribed Graphene Electrodes for On-Chip Electrochemical Sensing Applications, Adv. Electron. Mater., 2016, 2(10), 1600185, DOI:10.1002/aelm.201600185.
- Y. Yang, Y. Song, X. Bo, J. Min, O. S. Pak, L. Zhu, M. Wang, J. Tu, A. Kogan, H. Zhang and T. K. Hsiai, A Laser-Engraved Wearable Sensor for Sensitive Detection of Uric Acid and Tyrosine in Sweat, Nat. Biotechnol., 2020, 38(2), 217–224, DOI:10.1038/s41587-019-0321-x.
- M. Yang, H. Wang, P. Liu and J. Cheng, A 3D Electrochemical Biosensor Based on Super-Aligned Carbon NanoTube Array for Point-of-Care Uric Acid Monitoring, Biosens. Bioelectron., 2021, 179, 113082, DOI:10.1016/j.bios.2021.113082.
- S. Kumar, G. Mahendra and S. Ponnazhagan, Determination of Osteoprogenitor-Specific Promoter Activity in Mouse Mesenchymal Stem Cells by Recombinant Adeno-Associated Virus Transduction, Biochim. Biophys. Acta, Gene Struct. Expression, 2005, 1731(2), 95–103, DOI:10.1016/j.bbaexp.2005.08.007.
- S. Biswas, S. Pradhan, H. Naskar, R. Bandyopadhyay and P. Pramanik, Sol-Gel Synthesis of Cubic Titanium Dioxide Nanoparticle Using Poly(Ethylene Glycol) as a Capping Agent: Voltammetric Simultaneous Determination of Uric Acid and Guanine, Microchim. Acta, 2018, 185(11), 513, DOI:10.1007/s00604-018-3042-9.
- C. T. Tracey, M. A. Torlopov, I. S. Martakov, E. A. Vdovichenko, M. Zhukov, P. V. Krivoshapkin, V. I. Mikhaylov and E. F. Krivoshapkina, Hybrid Cellulose Nanocrystal/Magnetite Glucose Biosensors, Carbohydr. Polym., 2020, 247, 116704, DOI:10.1016/j.carbpol.2020.116704.
- R. Zamora-Sequeira, I. Ardao, R. Starbird and C. A. García-González, Conductive Nanostructured Materials Based on Poly-(3,4-Ethylenedioxythiophene) (PEDOT) and Starch/κ-Carrageenan for Biomedical Applications, Carbohydr. Polym., 2018, 189, 304–312, DOI:10.1016/j.carbpol.2018.02.040.
- H. D. Jirimali, R. K. Nagarale, D. Saravanakumar, J. M. Lee and W. Shin, Hydroquinone Modified Chitosan/Carbon Film Electrode for the Selective Detection of Ascorbic Acid, Carbohydr. Polym., 2013, 92(1), 641–644, DOI:10.1016/j.carbpol.2012.09.024.
- H. Liu, R. Jamal, T. Abdiryim, R. Simayi, L. Liu and Y. Liu, Carboxylated Cellulose as a Soft Template Combined with PEDOT Derivatives in [BMIM]Cl: A Competent Biosensor for Detection of Guanine and Uric Acid in the Blood, ACS Sustainable Chem. Eng., 2021, 9(17), 5860–5871, DOI:10.1021/acssuschemeng.0c09259.
- B. M. Tolbert, M. Downing, R. W. Carlson, M. K. Knight and E. M. Baker, Chemistry and Metabolism of Ascorbic Acid and ascorbate sulfate, Ann. N. Y. Acad. Sci., 1975, 258(1), 48–69, DOI:10.1111/j.1749-6632.1975.tb29267.x.
- O. Arrigoni and M. C. De Tullio, Ascorbic Acid: Much More than Just an Antioxidant, Biochim. Biophys. Acta, 2002, 1569(1–3), 1–9, DOI:10.1016/s0304-4165(01)00235-5.
- B. Gassmann, Ascorbic Acid Metabolism and Uses. Herausgegeben von Tolbert. 604 Sei-Ten, 110 Abb., 119 Tab., Zahlreiche Formelschemata. Advances in Chemistry Series 200, American Chemical Society, Washington, D.C., 1982. Preis: 79.9% (Inland), 95,95 $ (Export), Mol. Nutr. Food Res., 1983, 27(10), 1028, DOI:10.1002/food.19830271036.
- D. Voet, J. G. Voet and C. W. Pratt, Fundamentals of Biochemistry (No. QD415 V63), Wiley, New York, 2002, DOI:10.1016/0307-4412(95)90658-4.
- Z. Gao and H. Huang, Simultaneous Determination of Dopamine Uric Acid and Ascorbic Acid at an Ultrathin Film Modified Gold Electrode, Chem. Commun., 1998, 2107–2108, 10.1039/A805915B.
- J. Huang, Y. Liu, H. Hou and T. You, Simultaneous Electrochemical Determination of Dopamine, Uric Acid and Ascorbic Acid Using Palladium Nanoparticle-Loaded Carbon Nanofibers Modified Electrode, Biosens. Bioelectron., 2008, 24(4), 632–637, DOI:10.1016/j.bios.2008.06.011.
- N. Chauhan, J. Narang and C. S. Pundir, Fabrication of Multiwalled Carbon Nanotubes/Polyaniline Modified Au Electrode for Ascorbic Acid Determination, Analyst, 2011, 136(9), 1938–1945, 10.1039/C0AN00218F.
- L. Yang, S. Liu, Q. Zhang and F. Li, Simultaneous Electrochemical Determination of Dopamine and Ascorbic Acid Using AuNPs @ Polyaniline Core – Shell Nanocomposites Modified Electrode, Talanta, 2012, 89, 136–141, DOI:10.1016/j.talanta.2011.12.002.
- J. H. Rossato, M. E. Oliveira, B. V. Lopes, B. B. Gallo, A. B. La Rosa, E. Piva, D. Barba, F. Rosei, N. L. Carreño and M. T. Escote, A Flexible Electrochemical Biosensor Based on NdNiO3 Nanotubes for Ascorbic Acid Detection, ACS Appl. Nano Mater., 2022, 5(3), 3394–3405, DOI:10.1021/acsanm.1c03992.
- J. Ma, L. Shen, Y. Jiang, H. Ma, F. Lv, J. Liu, Y. Su and N. Zhu, Wearable self-powered smart sensors for portable nutrition monitoring, Anal. Chem., 2022, 94(4), 2333–2340, DOI:10.1021/acs.analchem.1c05189.
- J. Zhao, H. Yin, Y. Nyein, L. Hou, Y. Lin, M. Bariya, C. H. Ahn, W. Ji, Z. Fan and A. Javey, A Wearable Nutrition Tracker, Adv. Mater., 2021, 33(1), 2006444, DOI:10.1002/adma.202006444.
- J. R. Sempionatto, A. A. Khorshed, A. Ahmed, A. N. De Loyola e Silva, A. Barfidokht, L. Yin, K. Y. Goud, M. A. Mohamed, E. Bailey, J. May and C. Aebischer, Epidermal Enzymatic Biosensors for Sweat Vitamin C: Toward Personalized Nutrition, ACS Sens., 2020, 5(6), 1804–1813, DOI:10.1021/acssensors.0c00604.
- O. Farver, S. Wherland and I. Pecht, Intramolecular Electron Transfer in Ascorbate Oxidase is Enhanced in the Presence of Oxygen, J. Biol. Chem., 1994, 269(37), 22933–22936 CrossRef CAS.
- G. Csiffáry, P. Fűtő, N. Adányi and A. Kiss, Ascorbate Oxidase-Based Amperometric Biosensor for l-Ascorbic Acid Determination in Beverages, Food Technol. Biotechnol., 2016, 54(1), 31–35, DOI:10.17113/ftb.54.01.16.4135.
- W. He, C. Wang, H. Wang, M. Jian, W. Lu and X. Liang, Integrated Textile Sensor Patch for Real-Time and Multiplex Sweat Analysis, Sci. Adv., 2019, 1–9 Search PubMed.
- P. Si, H. Chen, P. Kannan and D.-H. Kim, Selective and Sensitive Determination of Dopamine by Composites of Polypyrrole and Graphene Modified Electrodes, Analyst, 2011, 136(24), 5134–5138, 10.1039/C1AN15772H.
- B. Le Foll, A. Gallo, Y. Le Strat, L. Lu and P. Gorwood, Genetics of Dopamine Receptors and Drug Addiction: A Comprehensive Review, Behav. Pharmacol., 2009, 20(1), 1–17, DOI:10.1097/FBP.0b013e3283242f05.
- L. Smith, M. Watson, S. Gates, D. Ball and D. Foxcroft, Meta-Analysis of the Association of the Taq1A Polymorphism with the Risk of Alcohol Dependency: A HuGE Gene-Disease Association Review, Am. J. Epidemiol., 2008, 167(2), 125–138, DOI:10.1093/aje/kwm281.
- R. F. Tyndale, Genetics of Alcohol and Tobacco Use in Humans, Ann. Med., 2003, 35(2), 94–121, DOI:10.1080/07853890310010014.
- A. H. Wong, C. E. Buckle and H. H. Van Tol, Polymorphisms in Dopamine Receptors: What Do They Tell Us?, Eur. J. Pharmacol., 2000, 410(2–3), 183–203, DOI:10.1016/s0014-2999(00)00815-3.
- D. E. Comings and K. Blum, Reward Deficiency Syndrome: Genetic Aspects of Behavioral Disorders, Prog. Brain Res., 2000, 126, 325–341, DOI:10.1016/S0079-6123(00)26022-6.
- W. Zhang, G. Dong, H. Feng, S. Shan, L. Huang, F. Yuan, B. Bao, L. Yan, Z. Xia, T. Lawson, J. Chen, J. Qu and Y. Liu, Wearable Corneal Biosensors Fabricated from PEDOT Functionalized Sulfur-Doped Graphene for Use in the Early Detection of Myopia, Adv. Mater. Technol., 2020, 5(12), 2000682, DOI:10.1002/admt.202000682.
- C. Vanitha, A. Sanmugam, A. Yogananth, M. Rajasekar, P. G. Kuppusamy and G. Devasagayam, A Facile Synthesis of Polyaniline-WO3 Hybrid Nanocomposite for Enhanced Dopamine Detection, Mater. Lett., 2022, 328, 133149, DOI:10.1016/j.matlet.2022.133149.
- N. Lu, Y. Liu, X. Yan, Z. Xu, Y. Xing, Y. Song, P. Zhao, M. Liu, Y. Gu, Z. Zhang and S. Zhai, Bioinspired Surface Modification of Graphene-Based Hybrids as Nanozyme Sensors for Simultaneous Detection of Dopamine and Uric Acid, ACS Appl. Nano Mater., 2022, 5(8), 11361–11370, DOI:10.1021/acsanm.2c02446.
- A. Salimi and R. Hallaj, Cobalt Oxide Nanostructure-Modified Glassy Carbon Electrode as a Highly Sensitive Flow Injection Amperometric Sensor for the Picomolar Detection of Insulin, J. Solid State Electrochem., 2012, 16(3), 1239–1246, DOI:10.1007/s10008-011-1510-9.
- M. A. Zahed, S. C. Barman, R. M. Toyabur, M. Sharifuzzaman, X. Xuan, J. Nah and J. Y. Park, Ex Situ Hybridized Hexagonal Cobalt Oxide Nanosheets and RGO@MWCNT Based Nanocomposite for Ultra-Selective Electrochemical Detection of Ascorbic Acid, Dopamine, and Uric Acid, J. Electrochem. Soc., 2019, 166(6), B304–B311 CrossRef CAS.
- A. Berni, A. Ait, K. Nabil and A. Amine, 3D-Porous Laser-Scribed Graphene Decorated with Overoxidized Polypyrrole as an Electrochemical Sensing Platform for Dopamine, J. Electroanal. Chem., 2022, 919, 116529, DOI:10.1016/j.jelechem.2022.116529.
- D. J. Harrison, R. F. B. Turner and H. P. Baltes, Characterization of Perfluorosulfonic Acid Polymer Coated Enzyme Electrodes and a Miniaturized Integrated Potentiostat for Glucose Analysis in Whole Blood, Anal. Chem., 1988, 60(19), 2002–2007, DOI:10.1021/ac00170a003.
- G. Cui, J. H. Yoo, B. W. Woo, S. S. Kim, G. S. Cha and H. Nam, Disposable Amperometric Glucose Sensor Electrode with Enzyme-Immobilized Nitrocellulose Strip, Talanta, 2001, 54, 1105–1111 CrossRef CAS PubMed.
- U. Harborn, B. Xie, R. Venkatesh and B. Danielsson, Evaluation of a Miniaturized Thermal Biosensor for the Determination of Glucose in Whole Blood, Clin. Chim. Acta, 1997, 267(2), 225–237, DOI:10.1016/S0009-8981(97)00151-4.
- J. Zhu, Z. Zhu, Z. Lai, R. Wang, X. Guo, X. Wu, G. Zhang, Z. Zhang, Y. Wang and Z. Chen, Planar Amperometric Glucose Sensor Based on Glucose Oxidase Immobilized by Chitosan Film on Prussian Blue Layer, Sensors, 2002, 2(4), 127–136, DOI:10.3390/s20400127.
- A. Abellán-Llobregat, I. Jeerapan, A. Bandodkar, L. Vidal, A. Canals, J. Wang and E. Morallón, A Stretchable and Screen-Printed Electrochemical Sensor for Glucose Determination in Human Perspiration, Biosens. Bioelectron., 2017, 91, 885–891, DOI:10.1016/j.bios.2017.01.058.
- A. Kagie, D. K. Bishop, J. Burdick, J. T. La Belle, R. Dymond, R. Felder and J. Wang, Flexible Rolled Thick-Film Miniaturized Flow-Cell for Minimally Invasive Amperometric Sensing, Electroanalysis, 2008, 20(14), 1610–1614, DOI:10.1002/elan.200804253.
- S. A. Miscoria, G. D. Barrera and G. A. Rivas, Glucose Biosensors Based on the Immobilization of Glucose Oxidase and Polytyramine on Rodhinized Glassy Carbon and Screen Printed Electrodes, Sens. Actuators, B, 2006, 115(1), 205–211, DOI:10.1016/j.snb.2005.09.002.
- W. Han, H. He, L. Zhang, C. Dong, H. Zeng, Y. Dai, L. Xing, Y. Zhang and X. Xue, A Self-Powered Wearable Noninvasive Electronic-Skin for Perspiration Analysis Based on Piezo-Biosensing Unit Matrix of Enzyme/ZnO Nanoarrays, ACS Appl. Mater. Interfaces, 2017, 9(35), 29526–29537, DOI:10.1021/acsami.7b07990.
- Y. Lei, R. Sun, X. Zhang, X. Feng and L. Jiang, Oxygen-Rich Enzyme Biosensor Based on Superhydrophobic Electrode, Adv. Mater., 2016, 28(7), 1477–1481, DOI:10.1002/adma.201503520.
- M. Ahmad, C. Pan, Z. Luo and J. Zhu, A Single ZnO Nanofiber-Based Highly Sensitive Amperometric Glucose Biosensor, J. Phys. Chem. C, 2010, 114(20), 9308–9313, DOI:10.1021/jp102505g.
- A. Koh, D. Kang, Y. Xue, S. Lee, R. M. Pielak, J. Kim, T. Hwang, S. Min, A. Banks, P. Bastien, M. C. Manco, L. Wang, K. R. Ammann, K. I. Jang, P. Won, S. Han, R. Ghaffari, U. Paik, M. J. Slepian, G. Balooch, Y. Huang and J. A. Rogers, A Soft, Wearable Microfluidic Device for the Capture, Storage, and Colorimetric Sensing of Sweat, Sci. Transl. Med., 2016, 8(366), 1–14, DOI:10.1126/scitranslmed.aaf2593.
- X. Xuan, H. S. Yoon and J. Y. Park, A Wearable Electrochemical Glucose Sensor Based on Simple and Low-Cost Fabrication Supported Micro-Patterned Reduced Graphene Oxide Nanocomposite Electrode on Flexible Substrate, Biosens. Bioelectron., 2018, 109, 75–82, DOI:10.1016/j.bios.2018.02.054.
- A. Martin, J. Kim, J. F. Kurniawan, J. R. Sempionatto, J. R. Moreto, G. Tang, A. S. Campbell, A. Shin, M. Y. Lee, X. Liu and J. Wang, Epidermal Microfluidic Electrochemical Detection System: Enhanced Sweat Sampling and Metabolite Detection, ACS Sens., 2017, 2(12), 1860–1868, DOI:10.1021/acssensors.7b00729.
- A. J. Bandodkar, W. Jia, C. Yardımcı, X. Wang, J. Ramirez and J. Wang, Tattoo-Based Noninvasive Glucose Monitoring: A Proof-of-Concept Study, Anal. Chem., 2015, 87(1), 394–398, DOI:10.1021/ac504300n.
- M. J. Tierney, J. A. Tamada, R. O. Potts, L. Jovanovic and S. Garg, Clinical Evaluation of the GlucoWatch Biographer: A Continual, Non-Invasive Glucose Monitor for Patients with Diabetes, Biosens. Bioelectron., 2001, 16(9–12), 621–629, DOI:10.1016/s0956-5663(01)00189-0.
- J. Kim, A. S. Campbell and J. Wang, Wearable Non-Invasive Epidermal Glucose Sensors: A Review, Talanta, 2018, 177, 163–170, DOI:10.1016/j.talanta.2017.08.077.
- J. Moyer, D. Wilson, I. Finkelshtein, B. Wong and R. Potts, Correlation between Sweat Glucose and Blood Glucose in Subjects with Diabetes, Diabetes Technol. Ther., 2012, 14(5), 398–402, DOI:10.1089/dia.2011.0262.
- Y. Chen, S. Lu, S. Zhang, Y. Li, Z. Qu, Y. Chen, B. Lu, X. Wang and X. Feng, Skin-like Biosensor System via Electrochemical Channels for Noninvasive Blood Glucose Monitoring, Sci. Adv., 2017, 3(12), e1701629, DOI:10.1126/sciadv.1701629.
- M. S. Bacchu, M. R. Ali, M. N. Hasan, M. R. A. Mamun, M. I. Hossain and M. Z. H. Khan, Graphitic Carbon Nitride and APTES Modified Advanced Electrochemical Biosensor for Detection of 17β-Estradiol in Spiked Food Samples, RSC Adv., 2022, 12(26), 16581–16588, 10.1039/d2ra02315f.
- Z. Lin, L. Chen, G. Zhang, Q. Liu, B. Qiu, Z. Cai and G. Chen, Label-Free Aptamer-Based Electrochemical Impedance Biosensor for 17β-Estradiol, Analyst, 2012, 137(4), 819–822, 10.1039/C1AN15856B.
- K.-J. Huang, Y.-J. Liu, J.-Z. Zhang, J.-T. Cao and Y.-M. Liu, Aptamer/Au Nanoparticles/Cobalt Sulfide Nanosheets Biosensor for 17β-Estradiol Detection Using a Guanine-Rich Complementary DNA Sequence for Signal Amplification, Biosens. Bioelectron., 2015, 67, 184–191, DOI:10.1016/j.bios.2014.08.010.
- D. Zhang, W. Zhang, J. Ye, S. Zhan, B. Xia, J. Lv, H. Xu, G. Du and L. Wang, A Label-Free Colorimetric Biosensor for 17β-Estradiol Detection Using Nanoparticles Assembled by Aptamer and Cationic Polymer, Aust. J. Chem., 2016, 69(1), 12–19 CrossRef CAS.
- K.-J. Huang, Y.-J. Liu, J.-Z. Zhang and Y.-M. Liu, A Novel Aptamer Sensor Based on Layered Tungsten Disulfide Nanosheets and Au Nanoparticles Amplification for 17β-Estradiol Detection, Anal. Methods, 2014, 6(19), 8011–8017, 10.1039/C4AY01478B.
- Y. S. Kim, H. S. Jung, T. Matsuura, H. Y. Lee, T. Kawai and M. B. Gu, Electrochemical Detection of 17β-Estradiol Using DNA Aptamer Immobilized Gold Electrode Chip, Biosens. Bioelectron., 2007, 22(11), 2525–2531, DOI:10.1016/j.bios.2006.10.004.
- Y. Zhang, J. Zhou, X.-X. Zhang, W.-L. Wang, C. Yang, X. Shi, Y.-W. Feng and R. Abdurahman, NIR Persistent Luminescence Nanoparticles Based Turn-on Aptasensor for Autofluorescence-Free Determination of 17β-Estradiol in Milk, Food Chem., 2022, 373, 131432, DOI:10.1016/j.foodchem.2021.131432.
- Q. Li, X. Li, P. Zhou, R. Chen, R. Xiao and Y. Pang, Split Aptamer Regulated CRISPR/Cas12a Biosensor for 17β-Estradiol through a Gap-Enhanced Raman Tags Based Lateral FLow Strategy, Biosens. Bioelectron., 2022, 215, 114548, DOI:10.1016/j.bios.2022.114548.
- S. Qi, X. Dong, Y. Sun, Y. Zhang, N. Duan and Z. Wang, Split Aptamer Remodeling-Initiated Target-Self-Service 3D-DNA Walker for Ultrasensitive Detection of 17β-Estradiol, J. Hazard. Mater., 2022, 439, 129590, DOI:10.1016/j.jhazmat.2022.129590.
- Q. Zhang, Y. Wang, A. Mateescu, K. Sergelen, A. Kibrom, U. Jonas, T. Wei and J. Dostalek, Biosensor Based on Hydrogel Optical Waveguide Spectroscopy for the Detection of 17β-Estradiol, Talanta, 2013, 104, 149–154, DOI:10.1016/j.talanta.2012.11.017.
- E. Povedano, F. H. Cincotto, C. Parrado, P. Díez, A. Sánchez, T. C. Canevari, S. A. S. Machado, J. M. Pingarrón and R. Villalonga, Decoration of Reduced Graphene Oxide with Rhodium Nanoparticles for the Design of a Sensitive Electrochemical Enzyme Biosensor for 17β-Estradiol, Biosens. Bioelectron., 2017, 89, 343–351, DOI:10.1016/j.bios.2016.07.018.
- A. Wang, Y. Ding, L. Li, D. Duan, Q. Mei, Q. Zhuang, S. Cui and X. He, A Novel Electrochemical Enzyme Biosensor for Detection of 17β-Estradiol by Mediated Electron-Transfer System, Talanta, 2019, 192, 478–485, DOI:10.1016/j.talanta.2018.09.018.
- N. Pupinyo, C. D'Costa, A. Heiskanen, W. Laiwattanapaisal and J. Emnéus, Impedance-Based E-Screen Cell Biosensor for the Real-Time Screening of Xenoestrogenic Compounds, ACS ES&T Water, 2022, 2(3), 446–456, DOI:10.1021/acsestwater.1c00383.
- A. Numnuam, P. Thavarungkul and P. Kanatharana, An Amperometric Uric Acid Biosensor Based on Chitosan-Carbon Nanotubes Electrospun Nanofiber on Silver Nanoparticles, Anal. Bioanal. Chem., 2014, 406(15), 3763–3772, DOI:10.1007/s00216-014-7770-3.
- J. Gao, W. Huang, Z. Chen, C. Yi and L. Jiang, Simultaneous Detection of Glucose, Uric Acid and Cholesterol Using Flexible Microneedle Electrode Array-Based Biosensor and Multi-Channel Portable Electrochemical Analyzer, Sens. Actuators, B, 2019, 287, 102, DOI:10.1016/j.snb.2019.02.020.
- W. Liu, Y. Nie, M. Zhang, K. Yan, M. Wang, Y. Guo and Q. Ma, A Novel Nanosponge–Hydrogel System-Based Electrochemiluminescence Biosensor for Uric Acid Detection, Luminescence, 2022, 37(9), 1524–1531, DOI:10.1002/bio.4326.
- S. RoyChoudhury, Y. Umasankar, J. Jaller, I. Herskovitz, J. Mervis, E. Darwin, P. A. Hirt, L. J. Borda, H. A. Lev-Tov, R. Kirsner and S. Bhansali, Continuous Monitoring of Wound Healing Using a Wearable Enzymatic Uric Acid Biosensor, J. Electrochem. Soc., 2018, 165(8), B3168, DOI:10.1149/2.0231808jes.
- R. Ahmad, N. Tripathy, M. Ahn, K. S. Bhat, Y. Wang, J. Yoo, D. Kwon and H. Yang, Highly Efficient Non-Enzymatic Glucose Sensor Based on CuO Modified Vertically-Grown ZnO Nanorods on Electrode, Sci. Rep., 2017, 1–10, DOI:10.1038/s41598-017-06064-8.
- Y. Lei, X. Liu, X. Yan, Y. Song, Z. Kang, N. Luo and Y. Zhang, Multicenter Uric Acid Biosensor Based on Tetrapod-Shaped ZnO Nanostructures, J. Nanosci. Nanotechnol., 2012, 12(1), 513–518, DOI:10.1166/jnn.2012.5336.
- T. Ghosh, P. Sarkar and A. P. F. Turner, A Novel Third Generation Uric Acid Biosensor Using Uricase Electro-Activated with Ferrocene on a Nafion Coated Glassy Carbon Electrode, Bioelectrochemistry, 2015, 102, 1–9, DOI:10.1016/j.bioelechem.2014.11.001.
- A. A. Hathoot, U. S. Yousef and A. S. Shatla, Voltammetric Simultaneous Determination of Glucose, Ascorbic Acid and Dopamine on Glassy Carbon Electrode Modified ByNiNPs@Poly 1,5-diaminonaphthalene, Electrochim. Acta, 2012, 85, 531–537, DOI:10.1016/j.electacta.2012.08.063.
- J. R. Windmiller, A. J. Bandodkar, G. Valde, S. Parkhomovsky, A. G. Martinez and J. Wang, Electrochemical Sensing Based on Printable Temporary Transfer Tattoos, Chem. Commun., 2012, 48, 6794–6796, 10.1039/c2cc32839a.
- O. Mickelsen and A. Keys, The Composition of Sweat, with Special Reference to the Vitamins, J. Biol. Chem., 1943, 149(2), 479–490, DOI:10.1016/S0021-9258(18)72194-7.
- E. Skaria, B. A. Patel, M. S. Flint and K. W. Ng, Poly(Lactic) Acid/Carbon Nanotube Composite Microneedle Arrays for Dermal Biosensing, Anal. Chem., 2019, 91(7), 4436–4443, DOI:10.1021/acs.analchem.8b04980.
- X. Zan, H. Bai, C. Wang, F. Zhao and H. Duan, Graphene Paper Decorated with a 2D Array of Dendritic Platinum Nanoparticles for Ultrasensitive Electrochemical Detection of Dopamine Secreted by Live Cells, Chem.–Eur. J., 2016, 22(15), 5204–5210, DOI:10.1002/chem.201504454.
- G. Xu, Z. A. Jarjes, V. Desprez, P. A. Kilmartin and J. Travas-Sejdic, Sensitive, Selective, Disposable Electrochemical Dopamine Sensor Based on PEDOT-Modified Laser Scribed Graphene, Biosens. Bioelectron., 2018, 107, 184–191, DOI:10.1016/j.bios.2018.02.031.
- E. K. Varadharaj and N. Jampana, Non-Invasive Potentiometric Sensor for Measurement of Blood Urea in Human Subjects Using Reverse Iontophoresis, J. Electrochem. Soc., 2016, 163(7), 340–347, DOI:10.1149/2.0751607jes.
- M. H. Andrew, K. Nga, T. L. Chwee, Y. Hong and K. P. L. Lowa, Highly Sensitive Reduced Graphene Oxide Microelectrode Array Sensor, Biosens. Bioelectron., 2015, 65, 265–273, DOI:10.1016/j.bios.2014.10.048.
- A. A. M. Abdurhman, Y. Zhang, G. Zhang and S. Wang, Hierarchical Nanostructured Noble Metal/Metal Oxide/Graphene-Coated Carbon Fiber: In Situ Electrochemical Synthesis and Use as Microelectrode for Real-Time Molecular Detection of Cancer Cells, Anal. Bioanal. Chem., 2015, 407, 8129–8136, DOI:10.1007/s00216-015-8989-3.
- A. Abellán-Llobregat, I. Jeerapan, A. Bandodkar, L. Vidal, A. Canals, J. Wang and E. Morallon, A Stretchable and Screen-Printed Electrochemical Sensor for Glucose Determination in Human Perspiration, Biosens. Bioelectron., 2017, 91, 885–891, DOI:10.1016/j.bios.2017.01.058.
- H. Lee, T. K. Choi, Y. B. Lee, H. R. Cho, R. Ghaffari, L. Wang, H. J. Choi, T. D. Chung, N. Lu, T. Hyeon and S. H. Choi, A Graphene-Based Electrochemical Device with Thermoresponsive Microneedles for Diabetes Monitoring and Therapy, Nat. Nanotechnol., 2016, 11(6), 566–572, DOI:10.1038/nnano.2016.38.
- A. Zhao, Z. Zhang, P. Zhang, S. Xiao, L. Wang, Y. Dong, H. Yuan, P. Li, Y. Sun, X. Jiang and F. Xiao, 3D Nanoporous Gold Scaffold Supported on Graphene Paper : Freestanding and Fl Exible Electrode with High Loading of Ultra Fi Ne PtCo Alloy Nanoparticles for Electrochemical Glucose Sensing, Anal. Chim. Acta, 2016, 1–9, DOI:10.1016/j.aca.2016.08.013.
- W. He, Y. Sun, J. Xi, A. Alamer and M. Abdurhman, Printing Graphene-Carbon Nanotube-Ionic Liquid Gel on Graphene Paper: Towards Flexible Electrodes with Efficient Loading of PtAu Alloy Nanoparticles for Electrochemical Sensing of Blood Glucose, Anal. Chim. Acta, 2016, 903, 61–68, DOI:10.1016/j.aca.2015.11.019.
- Z. Pu, R. Wang, J. Wu, H. Yu, K. Xu and D. Li, A Flexible Electrochemical Glucose Sensor with Composite Nanostructured Surface of the Working Electrode, Sens. Actuators, B, 2016, 230, 801–809, DOI:10.1016/j.snb.2016.02.115.
- H. Yoon, J. Nah, H. Kim, S. Ko, S. C. Barman, X. Xuan, J. Kim and J. Y. Park, A Chemically Modified Laser-Induced Porous Graphene Based Flexible and Ultrasensitive Electrochemical Biosensor for Sweat Glucose Detection, Sens. Actuators, B, 2020, 127866, DOI:10.1016/j.snb.2020.127866.
- E. V. Karpova, E. V. Shcherbacheva, A. A. Galushin, D. V. Vokhmyanina, E. E. Karyakina and A. A. Karyakin, Non-Invasive Diabetes Monitoring through Continuous Analysis of Sweat Using Flow-through Glucose Biosensor, Anal. Chem., 2019, 91(6), 3778–3783, DOI:10.1021/acs.analchem.8b05928.
- S. J. Lee, H. S. Yoon, X. Xuan, J. Y. Park, S. J. Paik and M. G. Allen, A patch type non-enzymatic biosensor based on 3D SUS micro-needle electrode array for minimally invasive continuous glucose monitoring, Sens. Actuators, B, 2016, 222, 1144–1151, DOI:10.1016/j.snb.2015.08.013.
- L. M. Strambini, A. Longo, S. Scarano, T. Prescimone, I. Palchetti, M. Minunni, D. Giannessi and G. Barillaro, Self-Powered Microneedle-Based Biosensors for Pain-Free High-Accuracy Measurement of Glycaemia in Interstitial Fluid, Biosens. Bioelectron., 2015, 66, 162–168, DOI:10.1016/j.bios.2014.11.010.
- B. Hansen, M. A. Hocevar and C. A. Ferreira, A Facile and Simple Polyaniline-Poly (Ethylene Oxide) Based Glucose Biosensor, Synth. Met., 2016, 222, 224–226 CrossRef CAS.
- Z. Miao, P. Wang, A. Zhong, M. Yang, Q. Xu, S. Hao and X. Hu, Development of a Glucose Biosensor Based on Electrodeposited Gold Nanoparticles–Polyvinylpyrrolidone–Polyaniline Nanocomposite, J. Electroanal. Chem., 2015, 756, 153–160, DOI:10.1016/j.jelechem.2015.08.025.
- H. Zhong, R. Yuan, Y. Chai, W. Li, X. Zhong and Y. Zhang, In Situ Chemo-Synthesized Multi-Wall Carbon Nanotube-Conductive Polyaniline Nanocomposites: Characterization and Application for a Glucose Amperometric Biosensor, Talanta, 2011, 85(1), 104–111, DOI:10.1016/j.talanta.2011.03.040.
- D. H. Keum, S. K. Kim, J. Koo, G. H. Lee, C. Jeon, J. W. Mok, B. H. Mun, K. J. Lee, E. Kamrani, C. K. Joo and S. Shin, Wireless Smart Contact Lens for Diabetic Diagnosis and Therapy, Sci. Adv., 2020, 6(17), eaba3252 CrossRef CAS PubMed.
- H. Yao, Y. Liao, A. R. Lingley, A. Afanasiev, I. Lähdesmäki, B. P. Otis and B. A. Parviz, A Contact Lens with Integrated Telecommunication Circuit and Sensors for Wireless and Continuous Tear Glucose Monitoring, J. Micromech. Microeng., 2012, 22, 075007, DOI:10.1088/0960-1317/22/7/075007.
- A. Caliò, P. Dardano, V. Di Palma, M. F. Bevilacqua, A. Di Matteo, H. Iuele and L. De Stefano, Polymeric microneedles based enzymatic electrodes for electrochemical biosensing of glucose and lactic acid, Sens. Actuators, B, 2016, 236, 343–349, DOI:10.1016/j.snb.2016.05.156.
- Z. Wang, S. Dong, M. Gui, M. Asif, W. Wang, F. Wang and H. Liu, Graphene Paper Supported MoS2 Nanocrystals Monolayer with Cu Submicron-Buds: High-Performance Flexible Platform for Sensing in Sweat, Anal. Biochem., 2018, 543, 82–89, DOI:10.1016/j.ab.2017.12.010.
- Y. Lei, W. Zhao, Y. Zhang, Q. Jiang, J. He, A. J. Baeumner, O. S. Wolfbeis, Z. L. Wang, K. N. Salama and H. N. Alshareef, A MXene-Based Wearable Biosensor System for High-Performance In Vitro Perspiration Analysis, Small, 2019, 15(19), 1901190, DOI:10.1002/smll.201901190.
- S. Anastasova, B. Crewther, B. Rosa and G. Yang, A Wearable Multisensing Patch for Continuous Sweat Monitoring, Biosens. Bioelectron., 2016, 93, 139–145, DOI:10.1016/j.bios.2016.09.038.
- P. Bollella, S. Sharma, A. E. G. Cass and R. Antiochia, Microneedle-Based Biosensor for Minimally-Invasive Lactate Detection, Biosens. Bioelectron., 2019, 123, 152–159, DOI:10.1016/j.bios.2018.08.010.
- J. R. Windmiller, N. Zhou, M. Chuang, G. Vald, P. Santhosh, P. R. Miller, R. Narayan and J. Wang, Microneedle Array-Based Carbon Paste Amperometric Sensors and Biosensors, Analyst, 2011, 1846–1851, 10.1039/c1an00012h.
- N. Thomas, I. Lähdesmäki and B. A. Parviz, A Contact Lens with an Integrated Lactate Sensor, Sens. Actuators, B, 2012, 162(1), 128–134, DOI:10.1016/j.snb.2011.12.049.
- J. Kim, A. G. Martinez, J. Ram and J. Wang, Non-Invasive Mouthguard Biosensor for Continuous Salivary Monitoring of Metabolites, Analyst, 2014, 1632–1636, 10.1039/c3an02359a.
- K. Da, M. Aksoy and E. Topçu, Flexible and Freestanding Catalase-Fe3O4/Reduced Graphene Oxide Paper: Enzymatic Hydrogen Peroxide Sensor Applications, Mater. Res. Bull., 2018, 106, 57–65, DOI:10.1016/j.materresbull.2018.05.032.
- Y. Peng, D. Lin, J. J. Gooding, Y. Xue and L. Dai, Flexible Fiber-Shaped Non-Enzymatic Sensors with a Graphene-Metal Heterostructure Based on Graphene Fibres Decorated with Gold Nanosheets, Carbon, 2018, 136, 329–336, DOI:10.1016/j.carbon.2018.05.004.
- T. Stögerer and S. Stäger, Innate Immune Sensing by Cells of the Adaptive Immune System, Front. Immunol., 2020, 11, 1081, DOI:10.3389/fimmu.2020.01081.
- Y. Kasuga, Innate Immune Sensing of Coronavirus and Viral Evasion Strategies, Exp. Mol. Med., 2021, 723–736, DOI:10.1038/s12276-021-00602-1.
- J. Gallo, M. Raska, Y. T. Konttinen, C. Nich and S. B. Goodman, Innate immunity sensors participating in pathophysiology of joint diseases: a brief overview, J. Long-Term Eff. Med. Implants, 2014, 24, 297–317, DOI:10.1615/jlongtermeffmedimplants.2014010825.
- J. S. Nah, S. C. Barman, A. Zahed, H. Yoon, C. Park, S. Yoon, S. Zhang and J. Y. Park, A Wearable Microfluidics-Integrated Impedimetric Immunosensor Based on Ti3C2Tx MXene Incorporated Laser-Burned Graphene for Noninvasive Sweat Cortisol Detection, Sens. Actuators, B, 2021, 329, 129206, DOI:10.1016/j.snb.2020.129206.
- S. Upasham, K. Thai, R. Muthyala and S. Prasad, Flexible, low volume detection of chronobiology biomarkers from human sweat, Analyst, 2020, 145(3), 784–796, 10.1039/c9an01968e.
- A. Ganguly, K. C. Lin, S. Muthukumar and S. Prasad, Autonomous, Real-Time Monitoring Electrochemical Aptasensor for Circadian Tracking of Cortisol Hormone in Sub-Microliter Volumes of Passively Eluted Human Sweat, ACS Sens., 2020, 6(1), 63–72, DOI:10.1021/acssensors.0c01754.
- M. Ku, J. Kim, J. E. Won, W. Kang, Y. G. Park, J. Park, J. H. Lee, J. Cheon, H. H. Lee, J. U. Park and Smart, Smart, soft contact lens for wireless immunosensing of cortisol, Sci. Adv., 2020, 6(28), eabb2891 CrossRef CAS PubMed.
- N. Ruecha, K. Shin, O. Chailapakul and N. Rodthongkum, Label-Free Paper-Based Electrochemical Impedance Immunosensor for Human Interferon Gamma Detection, Sens. Actuators, B, 2019, 279, 298–304, DOI:10.1016/j.snb.2018.10.024.
- Z. Wang, Z. Hao, X. Wang, C. Huang, Q. Lin, X. Zhao and Y. Pan, A Flexible and Regenerative Aptameric Graphene–Nafion Biosensor for Cytokine Storm Biomarker Monitoring in Undiluted Biofluids toward Wearable Applications, Adv. Funct. Mater., 2021, 31(4), 2005958, DOI:10.1002/adfm.202005958.
- Z. Wang, Z. Hao, S. Yu, C. Huang, Y. Pan and X. Zhao, A Wearable and Deformable Graphene-Based Affinity Nanosensor for Monitoring of Cytokines in Biofluids, Nanomaterials, 2020, 10(8), 1503 CrossRef CAS PubMed.
- K. Parate, S. V. Rangnekar, D. Jing, D. L. Mendivelso-perez, S. Ding, E. B. Secor, E. A. Smith, J. M. Hostetter, M. C. Hersam and J. C. Claussen, Aerosol-Jet-Printed Graphene Immunosensor for Label-Free Cytokine Monitoring in Serum, ACS Appl. Mater. Interfaces, 2020, 12(7), 8592–8603, DOI:10.1021/acsami.9b22183.
- N. K. M. Churcher, S. Upasham, P. Rice, S. Bhadsavle and S. Prasad, Development of a flexible, sweat-based neuropeptide Y detection platform, RSC Adv., 2020, 10(39), 23173–23186, 10.1039/d0ra03729j.
- Y. Wu, P. Xue, Y. Kang and K. M. Hui, Paper-based microfluidic electrochemical immunodevice integrated with nanobioprobes onto graphene film for ultrasensitive multiplexed detection of cancer biomarkers, Anal. Chem., 2013, 85(18), 8661–8668, DOI:10.1021/ac401445a.
- Y. Wang, H. Xu, J. Luo, J. Liu, L. Wang, Y. Fan and S. Yan, A Novel Label-Free Micro Fl Uidic Paper-Based Immunosensor for Highly Sensitive Electrochemical Detection of Carcinoembryonic Antigen, Biosens. Bioelectron., 2016, 83, 319–326, DOI:10.1016/j.bios.2016.04.062.
- A. E. Islam, R. Martineau, C. M. Crasto, H. Kim, R. S. Rao, B. Maruyama, S. S. Kim and L. F. Drummy, Graphene-based electrolyte-gated field-effect transistors for potentiometrically sensing neuropeptide Y in physiologically relevant environments, ACS Appl. Nano Mater., 2020, 3(6), 5088–5097, DOI:10.1021/acsanm.0c00353.
- B. Kim, H. Lee and N. Lee, A Durable, Stretchable, and Disposable Electrochemical Biosensor on Three- Dimensional Micro-Patterned Stretchable Substrate, Sens. Actuators, B, 2019, 283, 312–320, DOI:10.1016/j.snb.2018.12.045.
- A. Hatamie, A. Khan, M. Golabi, A. P. Turner, V. Beni, W. C. Mak, A. Sadollahkhani, H. Alnoor, B. Zargar, S. Bano and O. Nur, Zinc oxide nanostructure-modified textile and its application to biosensing, photocatalysis, and as antibacterial material, Langmuir, 2015, 31(39), 10913–10921, DOI:10.1021/acs.langmuir.5b02341.
- Z. Hao, Z. Wang, Y. Li, Y. Zhu, X. Wang, C. G. De Moraes, Y. Pan, X. Zhao and Q. Lin, Measurement of Cytokine Biomarkers Using an Aptamer-Based Affinity Graphene Nanosensor on a Flexible Substrate toward Wearable Applications, Nanoscale, 2018, 10(46), 21681–21688, 10.1039/c8nr04315a.
- Y. Wang, A. Keinonen, S. Koskenmies, S. Pitkänen, N. Fyhrquist, M. Sadeghi, H. Mäkisalo, M. Söderlund-Venermo and K. Hedman, Occurrence of newly discovered human polyomaviruses in skin of liver transplant recipients and their relation with squamous cell carcinoma in situ and actinic keratosis–a single-center cohort study, Transplant Int., 2019, 32(5), 516–522, DOI:10.1111/tri.13397.
- J. Jang, J. Kim, H. Shin, Y. G. Park, B. J. Joo, H. Seo, J. E. Won, D. W. Kim, C. Y. Lee, H. K. Kim and J. U. Park, Smart Contact Lens and Transparent Heat Patch for Remote Monitoring and Therapy of Chronic Ocular Surface Inflammation Using Mobiles, Sci. Adv., 2021, 7(14), eabf7194 CrossRef.
- K. Parate, C. C. Pola, S. V. Rangnekar, D. L. Mendivelso-perez, E. A. Smith, M. C. Hersam, C. L. Gomes and J. C. Claussen, Aerosol-Jet-Printed Graphene Electrochemical Histamine Sensors for Food Safety Monitoring Aerosol-Jet-Printed Graphene Electrochemical Histamine Sensors for Food Safety Monitoring, 2D Mater., 2020, 7(3), 034002 CrossRef CAS.
- F. Liu, K. S. Choi, T. J. Park, S. Y. Lee and T. S. Seo, Graphene-Based Electrochemical Biosensor for Pathogenic Virus Detection, BioChip J., 2011, 5, 123–128, DOI:10.1007/s13206-011-5204-2.
- D. S. Kinnamon, S. Krishnan, S. Brosler, E. Sun and S. Prasad, Screen printed graphene oxide textile biosensor for applications in inexpensive and wearable point-of-exposure detection of influenza for at-risk populations, J. Electrochem. Soc., 2018, 165(8), B3084–B3090, DOI:10.1149/2.0131808jes.
- M. Shin, J. Yoon, C. Yi, T. Lee and J. W. Choi, Flexible HIV-1 Biosensor Based on the Au/MoS2 Nanoparticles/Au Nanolayer on the PET Substrate, Nanomaterials, 2019, 9(8), 1076 CrossRef CAS.
- S. Boonkaew, P. Teengam, S. Jampasa, S. Rengpipat, W. Siangproh and O. Chailapakul, Cost-effective paper-based electrochemical immunosensor using a label-free assay for sensitive detection of ferritin, Analyst, 2020, 145(14), 5019–5026, 10.1039/d0an00564a.
- J. Q. Hu, X. L. Ma, N. G. Shang, Z. Y. Xie, N. B. Wong, C. S. Lee and S. T. Lee, Large-Scale Rapid Oxidation Synthesis of SnO2 Nanoribbons, J. Phys. Chem. B, 2002, 106(15), 3823–3826, DOI:10.1021/jp0125552.
- Y. Wang, J. Ping, Z. Ye, J. Wu and Y. Ying, Impedimetric Immunosensor Based on Gold Nanoparticles Modi Fi Ed Graphene Paper for Label-Free Detection of Escherichia Coli O157:H7, Biosens. Bioelectron., 2013, 49, 492–498, DOI:10.1016/j.bios.2013.05.061.
- M. S. Mannoor, H. Tao, J. D. Clayton, A. Sengupta, D. L. Kaplan, R. R. Naik, N. Verma, F. G. Omenetto and M. C. Mcalpine, On Tooth Enamel, Nat. Commun., 2012, 3, 763–768, DOI:10.1038/ncomms1767.
- S. Kim, L. Xing, A. E. Islam, M. S. Hsiao, Y. Ngo, O. M. Pavlyuk, R. L. Martineau, C. M. Hampton, C. Crasto, J. Slocik and M. P. Kadakia, In operando observation of neuropeptide capture and release on graphene field-effect transistor biosensors with picomolar sensitivity, ACS Appl. Mater. Interfaces, 2019, 11(15), 13927–13934, DOI:10.1021/acsami.8b20498.
- M. Elsherif, M. U. Hassan, A. K. Yetisen and H. Butt, Wearable contact lens biosensors for continuous glucose monitoring using smartphones, ACS Nano, 2018, 12(6), 5452–5462, DOI:10.1021/acsnano.8b00829.
- Y. Sung, F. Campa and W. C. Shih, Open-source do-it-yourself multi-color fluorescence smartphone microscopy, Biomed. Opt. Express, 2017, 8(11), 5075–5086 CrossRef CAS PubMed.
- A. K. Yetisen, J. L. Martinez-hurtado, A. Garcia-melendrez, C. Vasconcellos and C. R. Lowe, A Smartphone Algorithm with Inter-Phone Repeatability for the Analysis of Colorimetric Tests, Sens. Actuators, B, 2014, 196, 156–160, DOI:10.1016/j.snb.2014.01.077.
|
This journal is © The Royal Society of Chemistry 2023 |
Click here to see how this site uses Cookies. View our privacy policy here.