DOI:
10.1039/D3RA01930F
(Paper)
RSC Adv., 2023,
13, 13556-13563
Remarkably and stable catalytic activity in reduction of 4-nitrophenol by sodium sesquicarbonate-supporting Fe2O3@Pt†
Received
24th March 2023
, Accepted 26th April 2023
First published on 3rd May 2023
Abstract
Reasonable design of bimetallic nanomaterials with support is beneficial to improve catalytic performance. This work reports a new kind of sodium sesquicarbonate-supporting Fe2O3@Pt via etching Fe3O4@Pt@SiO2, which exhibits highly efficient and stable catalytic reduction performance towards 4-NP. Sodium sesquicarbonate-supporting Fe2O3@Pt has an interconnected one-dimensional network structure that provides sufficient channels for mass transfer. At the same time, a large amount of Fe2O3@Pt is exposed on its surface, which hinders the aggregation of pt clusters and Fe2O3 nanoparticles, and facilitates the direct contact of Fe2O3@Pt reaction sites with reactant molecules, thus improving the catalytic rate of 4-NP reduction reaction. Moreover, the introduction of non-metallic Fe can not only reduce the consumption of precious metal Pt, but also improve the catalytic efficiency due to the synergistic effect. This study opens up a new avenue to develop robust catalysts for heterogeneous catalytic reactions.
Introduction
In recent decades, environmental pollution and the energy crisis are threatening human life, leading to a variety of deteriorative consequences. Nitrophenol and its derivatives are some of the most refractory pollutants that occur in industrial wastewater, synthetic dyes, pharmaceuticals and other industries.1,2 Among them, 4-nitrophenol (4-NP) is regarded by the U.S. government as one of the toxic and refractory priority pollutants endangering the human central nervous system.3–5 Nevertheless, aminophenol with low toxicity is an important chemical intermediate in the synthesis of drugs, dyes, pesticides and imaging agents.6 Therefore, it is of great environmental and energy significance to eliminate nitro groups in toxic phenolic compounds or convert them into amino groups. Additionally, the conversion of nitrophenol to aminophenol has considerable industrial relevance for aniline and paracetamol production.7
Among various methods, the reduction of 4-nitrophenol (4-NP) to 4-aminophenol (4-AP) using reducing agents (such as NaBH4) is one of the simplest and cost-effective methods.8,9 This process requires E0 (4-NP/4-AP) = −0.76 V, which is thermodynamically appropriate, but there is a high kinetic barrier among the 4-NP and BH4− ions. Therefore, it is particularly important to select a suitable catalyst for the catalytic reduction of 4-NP to 4-AP. Noble metal nanoparticles have attracted attention due to their distinctive physicochemical properties.10–14 The catalytic reduction of 4-NP by noble metal nanostructures has been extensively investigated, including Au,15,16 Pd,17,18 Ag,19,20 Pt,21,22 Ag-Pt,23 Au-Pt,24 etc. These catalysts exhibit good catalytic reduction effect toward 4-NP. However, noble metal nanoparticles have small size, large specific surface area and high specific surface energy, so there is a trend of aggregation, which will lead to the gradual decline of the catalytic activity. Therefore, insufficient catalyst utilization coefficient, low mass transfer rate and unstable deactivation of noble metal catalysts are still some common problems that limit their application.25
To maximize the catalytic activity of noble metal nanoparticles, it is necessary to choose appropriate carrier materials to disperse them. Some oxides are used as carriers to disperse noble metal nanoparticles, such as Fe2O3,26 Al2O3,27 SiO2,28 and CeO2.29 As the carrier of noble metal nanoparticles, two-dimensional membrane materials have more advantages, which can not only improve the catalytic activity, but also improve the stability of the catalyst. Zhang's group loaded Au–Pt alloy nanoparticles on α-Co(OH)2 nanosheets to improve the catalytic performance for the 4-NP reduction reaction.30 Han's group used Pd nanospheres decorated reduced graphene oxide for highly efficient catalytic reduction of the hazardous 4-nitrophenol pollutant.31 Our research group has also published a study on the high efficiency reduction of p-nitrophenol (4-NP) by Au atomic cluster decorated Fe(OH)3/Fe2O3 nanosheets.32 In addition, relevant literature reports that inorganic salt materials can also be used as carriers. Yang's group describe the synthesis of Au nanoparticles coated chicken eggshell composites (defined as Au/CaCO3 nanocomposite), which can also be used as a sensor and catalyst for the efficient detection and treatment of 4-NP.33
On the other hand, due to the high cost and scarcity of noble metals, their wide application has been severely hampered. Relevant literature reports that transition metals can also be used as catalysts for the catalytic reduction of 4-NP. CuFe2O4 magnetic nanoparticles prepared by Chen's group exhibited several advantages such as stability, monodispersity, low-cost, simplicity and rapid separation performance over other catalysts for the reduction of nitrophenol.34 Chiaki Terashima's group use nanoflakes-like NiCo2O4 samples as catalysts for rapid reduction of 4-nitrophenol.35 In addition, Cu/graphdiyne material prepared by Li's group also exhibits high catalytic ability for rapid reduction of 4-NP within 3 min.36 These literature show that the transition metal nanomaterials also have a good catalytic effect on the 4-NP reduction reaction.
Furthermore, the reasonable design of bimetallic catalysts, based on alloying of noble metal with other transition metal (such as Cu, Co), is not only crucial to reduce costs, but even more importantly, to improve the physical and chemical properties of alloys.37 Li's group reported a clean and composition-tunable PdCu and AuCu monolithic aerogels with 3D nanowire-based network for enhanced catalytic reduction of 4-nitrophenol, the introduction of nonprecious Cu not only drastically cuts down the cost but also attains the excellent catalytic activity due to the bimetallic intrinsic synergetic effect.38,39 However, there are few reports on the catalytic reduction of 4-NP by bimetallic catalysts based on the alloying of noble metals with other easily oxidized transition metals.
Herein, Fe3O4@Pt were prepared. Although the synergistic effect between Pt and Fe greatly improves the catalytic rate for 4-NP reduction reactions, Fe3O4/Pt is easily separated and aggregated during use or storage, resulting in a worsening catalytic rate. Therefore, Fe2O3@Pt was designed to be loaded onto the surface of sodium sesquicarbonate crystals. The prepared sodium sesquicarbonate structure can not only prevent the aggregation of Pt cluster and Fe2O3 nanoparticle, but also ensure the synergistic effect between Pt and Fe, and improve the catalytic activity for 4-NP reduction.
Experimental
Materials
Chloroplatinic acid hexahydrate (H2PtCl6·6H2O), 2-morpholinoethanesulfonic acid (MES), hexadecyl trimethyl ammonium bromide (CTAB), polyvinylpyrrolidone (PVP) and p-nitrophenol (4-NP) were obtained from Aladdin reagent (Shanghai) Co., Ltd. Tetraethyl orthosilicate (TEOS) were obtained from Shanghai Zhongqin Chemical Reagent Co., Ltd. Ethanol and aqueous ammonia were obtained from Beijing Chemical Reagent. FeSO4 and Na2CO3 were obtained from Sinopharm Chemical Reagent Co., Ltd. NaBH4 was obtained from Jinan Yuxin Chemical Co., Ltd. All reagents used in this study are analytically pure and have not been purified in any way except for special labels. All experiments used deionized water.
Synthesis of Fe3O4@Pt
First, 5 mL distilled water and 4 mg FeSO4 were added into a 50 mL beaker for ultrasonic dispersion. Then add 5 mL ethanol and 0.2 mL H2PtCl6 aqueous solution (1 g/100 mL). After fully stirring, 5 mg NaBH4 was added into the mixed system. Centrifuge after stirring for ten minutes, discard the supernatant, wash the sediment with distilled water before dispersing the sediment in 2 mL distilled water for standby.
Synthesis of Fe3O4@Pt@SiO2
First, 5 mL distilled water, 4 mg FeSO4 and 100 mg MES were added into a 50 mL beaker for ultrasonic dispersion. Then add 5 mL ethanol, 0.2 mL H2PtCl6 aqueous solution (1 g/100 mL) and 45 μL TEOS. After fully stirring, 5 mg NaBH4 and 0.7 mL ammonia water were added into the mixed system at the same time. Centrifuge after stirring for ten minutes, discard the supernatant and wash the sediment with distilled water. Mark the prepared sample as Fe3O4@Pt@SiO2.
The synthesis method of the Fe3O4@Pt@SiO2 with different experimental condition was the same as the above, except that without MES or with 100 mg MES and 100 mg PVP or with 100 mg MES and 100 mg CTAB.
Synthesis of sodium sesquicarbonate-supporting Fe2O3@Pt
Wheat spike shaped sodium sesquicarbonate-supporting Fe2O3@Pt could be obtained by etching the Fe3O4@Pt@SiO2. The etching method is as follows: Fe3O4@Pt@SiO2 obtained by the above method was ultrasonically dispersed in 10 mL distilled water. Then add 0.035 g CTAB and 1.06 g Na2CO3 successively, and stir in a constant temperature oil bath at 80 °C. 100 μL mixed solution was taken from the system every 3 hours to monitor the change of catalytic performance. Centrifuge after etching for 9 hours. The product was centrifuged at hot time, and washed with hot water five times, then dispersed in 2 mL distilled water for standby.
The catalytic performance test for 4-NP reduction reaction
The catalytic performance test was carried out at room temperature with a 4 mL quartz colorimetric dish as the reaction vessel. The absorbance performance during reaction was monitored by 722N visible spectrophotometer or UV-vis spectra. The specific operation steps are as follows: 3 mL H2O, 20 μL 4-NP (0.01 mol L−1), and 20 μL catalyst solution (Pt nanoparticles, Fe3O4 nanoparticles or sesquicarbonate-supporting Fe2O3@Pt solution take from 2 mL spare solution) were successively added into a 4 mL quartz colorimetric dish. After the solution was completely dispersed evenly, 100 μL fresh NaBH4 (0.2 mol L−1) aqueous solution is dropped into the above solution. Then continue to record the absorbance value at λ = 400 nm or scan the UV-vis spectrum in the range of 250–500 nm immediately.
Characterizations
The powder X-ray diffraction (XRD) patterns were performed on a Panaco X′ Pert PRO X-ray diffractometer with Cu Kα radiation (l = 0.15406 nm) with the operation voltage and current maintained at 45 kV and 40 mA. Scanning speed: 1° min−1, test range: 10° ≤ 2θ ≤ 70°. Fourier transform infrared spectroscopy (FT-IR) analysis was carried out on a PerkinElmer FT-IR spectrometer. The scanning electron microscope (SEM) images were determined by Zeiss MERLIN Compact SEM-EDX microanalysis. TEM images and elemental mapping analysis of the samples were obtained on a FEI Talos F200S transmission electron microscope operating at 200 kV. The chemical states of different elements were determined by Thermo ESCALAB 250XI X-ray photoelectron spectroscopy (XPS). The UV-vis absorption spectra of the samples were measured on Shimadzu UV-1780 UV-vis-NIR spectrophotometer. The absorbance of the solution during the reaction was monitored by a 722N visible spectrophotometer.
Results and discussions
The catalytic performance of Fe3O4@Pt for 4-NP reduction reaction
4-NP solution has an obvious absorption peak at 400 nm. The reduction of 4-NP to 4-AP using reducing agents NaBH4 is thermodynamically appropriate, but there is a high kinetic barrier among the 4-NP and BH4− ions. Therefore, precious metal nanoparticles are the first choice for catalytic reduction of 4-NP. Fig. S1† shows the reaction process and time-dependent absorption spectrum of the reaction mixture in the wavelength range of 250–500 nm for the catalytic reduction of 4-NP by Pt nanoparticles at room temperature. The decrease in the 400 nm peak indicates the disappearance of 4-NP, whereas the formation of a new peak at 300 nm indicates the formation of 4-AP. Therefore, the absorbance value A at 400 nm could represent the concentration C of 4-NP in the solution. Since the amount of NaBH4 is excessive compared with that of 4-NP, the reaction kinetics follows the pseudo-first-order law, the apparent kinetic rate constant kapp can be obtained according to eqn (1) |
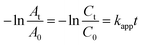 | (1) |
where t is the reaction time, At and A0 stand for the absorbance at time t and the initial absorbance of 4-NP, Ct and C0 is the concentration at time t and the initial concentration of 4-NP.
It can be seen from the Fig. 1a that the catalytic rate of Pt nanoparticles is not very ideal. In order to reduce the consumption of precious metals and solve the problems of insufficient utilization coefficient, low mass transfer rate and unstable deactivation, Fe3O4@Pt were designed and prepared. Fig. S2† shows the XRD result of Fe3O4@Pt, the diffraction peak at about 40° is attributed to Pt nanoparticles. The fine XPS spectrum of Fe element (as shown in Fig. S3†) indicates that Fe exists in the form of divalent and trivalent. The catalytic reduction effect of Fe3O4@Pt on 4-NP reduction reaction is much better than that of Pt, and Fe3O4 nanoparticles has no catalytic reduction effect (Fig. S4†). Therefore, it is speculated that the excellent catalytic performance of Fe3O4@Pt on the reduction of 4-NP was attributed to the synergistic effect between Pt and Fe. However, with the extension of the sample storage time, the catalytic performance of Fe3O4@Pt gradually weakened. When stored in aqueous solution for 3 hours, its catalytic rate decreases to half of the original one, and becomes worse after 6 hours.
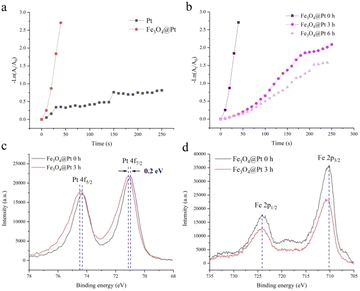 |
| Fig. 1 Plots of −ln(At/A0) versus the reaction time t for the reduction of 4-NP catalyzed by: (a) Pt and Fe3O4@Pt, (b) Fe3O4@Pt 0 h, 3 h, and 6 h, as well as the XPS fine spectra of (c) Pt 4f, (d) Fe 2p. | |
The reasons for the poor catalytic effect of Fe3O4@Pt were characterized by XPS and TEM. As shown in Fig. 1c, the high-resolution Pt 4f peaks near the binding energies of 71.0 and 74.5 eV are attributed to Pt 4f7/2 and 4f5/2, respectively. Notably, the Fe3O4@Pt (0 h) peak shows 0.2 eV negative shift compared to the Fe3O4@Pt (3 h), indicating the charge transfer from Fe to Pt in Fe3O4@Pt (0 h) and lead to the excellent catalytic effect of Fe3O4@Pt (0 h).41,42 In Fig. 1d, the peak at 710.0 and 724.2 eV were originated from Fe 2p3/2 and Fe 2p1/2, respectively. The TEM results of Fe3O4@Pt (0 h) and Fe3O4@Pt (3 h) were shown in Fig. 2. In Fe3O4@Pt (0 h), the dispersion and homogeneity of Pt and Fe3O4 are particularly good. Pt clusters of about 2 nm is evenly distributed on the surface of Fe3O4. After the sample is dispersed in aqueous solution for 3 h, Pt and Fe3O4 are separated from each other and agglomerated. Therefore, the interaction between Pt and Fe is reduced, and the catalytic reduction effect becomes worse. Combined with XPS and TEM results, Fe3O4@Pt (0 h) has a good catalytic effect on 4-NP reduction due to the synergistic effect between Pt and Fe.
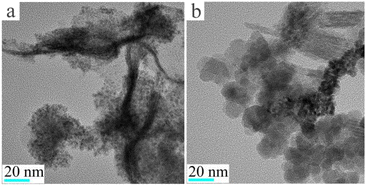 |
| Fig. 2 The TEM images of (a) Fe3O4@Pt 0 h and (b) Fe3O4@Pt 3 h at same magnifications. | |
Preparation and characterization of sodium sesquicarbonate-supporting Fe2O3@Pt
In order to prevent the separation of Pt and Fe3O4 and the agglomeration of Pt nanoparticles, newly prepared Fe3O4@Pt were encapsulated in SiO2 nanospheres (labeled as Fe3O4@Pt@SiO2), and then SiO2 was etched away by Na2CO3 to prepare sodium sesquicarbonate-supporting Fe2O3@Pt. As SEM results shown in Fig. 3, Fe3O4@Pt@SiO2 nanoparticles present irregular polyhedron and adhere to each other, which is attributed to the rapid polymerization of TEOS under alkaline conditions, and the Fe3O4@Pt nanoparticles are basically encapsulated in SiO2 polyhedron. After etching with Na2CO3 solution at 80 °C for 9 hours, irregular Fe3O4@Pt@SiO2 polyhedron are transformed into wheat ear like one-dimensional materials, as shown in Fig. 3c and d.
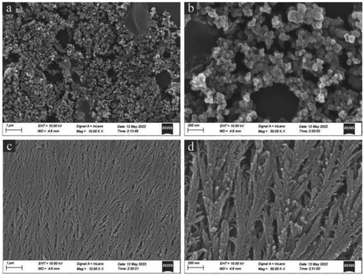 |
| Fig. 3 The scanning electron microscope (SEM) images of (a and b) Fe3O4@Pt@SiO2, (c and d) sodium sesquicarbonate-supporting Fe2O3@Pt at different magnifications. | |
XRD and FT-IR spectra verified the composition, crystal phase and surface characteristics of samples before and after Na2CO3 etching. According to X-ray diffraction results (Fig. 4), only a strong SiO2 (JCPDS#29-0085) diffraction peak at 20–30° is observed before Na2CO3 etching. However, a series of strong diffraction peaks were observed after Na2CO3 etching, the diffraction peaks at 18.17°, 27.90°, 29.12°, 29.21°, 33.91°, 36.83° and 40.09° correspond to (400), (111), (402), (
11), (
11), (800) and (204) crystal planes of sodium sesquicarbonate (JCPDS#72-1152), respectively. The FT-IR spectra also reflect the evolution of surface functional groups before and after etching. As shown in Fig. S5,† before etching, the strong and wide absorption band at 1077 cm−1 can be classified as Si–O–Si antisymmetric stretching vibration, and the wide peak at 3128 cm−1 is –OH antisymmetric stretching vibration on the SiO2 surface. After etching by Na2CO3, the above two characteristic peaks disappeared, and a new characteristic absorption peak appeared at 1457 cm−1, which was attributed to the stretching vibration of CO32−. The XRD and FT-IR results are consistent with SEM results. Before etching, Fe3O4@Pt were encapsulated in SiO2, only the diffraction peak of SiO2 and the absorption band of Si–O–Si antisymmetric stretching vibration could be detected. And after Na2CO3 etching, the SiO2 disappeared and there was a large amount of CO32− on the surface. Therefore, SEM, XRD and FT-IR spectra confirmed the formation of wheat spike shaped sodium sesquicarbonate crystals.
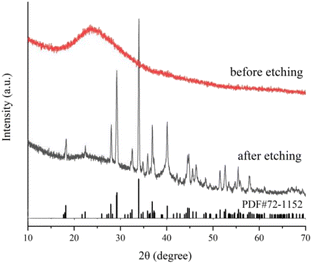 |
| Fig. 4 X-ray powder diffraction (XRD) patterns of Fe3O4@Pt@SiO2 (before etching) and wheat spike shaped sodium sesquicarbonate-supporting Fe2O3@Pt (after etching). | |
Due to the strong crystallinity of sodium sesquicarbonate crystals, the presence of Fe in the etched sample cannot be determined from XRD results. Therefore, the XPS fine spectrum of Fe was characterized. As shown in Fig. 5, the Fe 2p fine spectrum showed a significant shift after etching, and Fe exists in a trivalent form. Therefore, we speculate that Fe3O4 has transformed into Fe2O3.
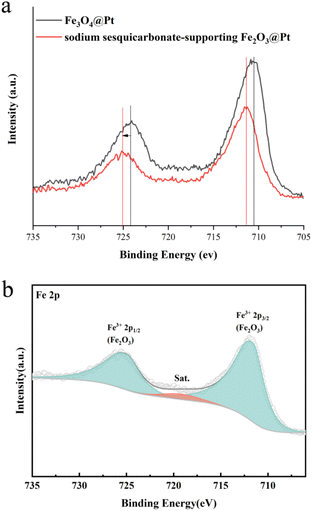 |
| Fig. 5 The Fe 2p XPS fine spectra of (a) Fe3O4@Pt and sodium sesquicarbonate-supporting Fe2O3@Pt, (b) sodium sesquicarbonate-supporting Fe2O3@Pt. | |
In order to further verify the composition of sodium sesquicarbonate crystal and the distribution of Fe2O3@Pt, the TEM images and the EDX elemental mapping patterns were characterized. As can be seen from Fig. 6, the residual SiO2 after etching is mixed with Fe2O3@Pt and uniformly adheres to the surface of sodium sesquicarbonate crystal (marked as sodium sesquicarbonate-supporting Fe2O3@Pt). Pt nanoparticles are uniform in size, with an average diameter of 2–3 nm. Due to natural self-assembly, they are in a disordered state. The HRTEM images of Pt nanoparticles presented all-pervading lattice fringes with a spacing of 0.23 nm, which corresponding to (111) interplane distance of cubic Pt (Fig. 6c and d). The chemical composition of sodium sesquicarbonate-supporting Fe2O3@Pt was also revealed by energy-dispersive X-ray (EDX) analysis. The EDX spectrum shown in Fig. S6† verified that the as-prepared sodium sesquicarbonate supporting Fe2O3@Pt is indeed doped with some Si. The EDX elemental mapping patterns further demonstrated the detailed composition distribution of sodium sesquicarbonate-supporting Fe2O3@Pt, indicating the homogeneous distribution of Pt and Fe elements (Fig. 6e–l).
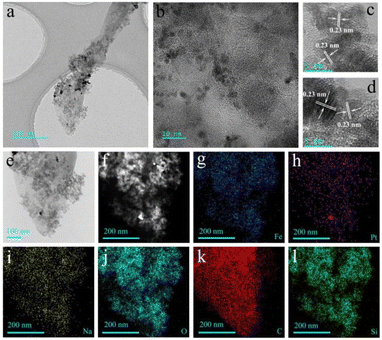 |
| Fig. 6 (a–d) TEM image of wheat spike shaped sodium sesquicarbonate-supporting Fe2O3@Pt, (e–l) corresponding elemental mappings of Fe, Pt, Na, O, C and Si according to the image in (e). | |
Catalytic performance of sodium sesquicarbonate-supporting Fe2O3@Pt for 4-NP reduction
The catalytic performances of Fe3O4@Pt@SiO2 (before etching) and sodium sesquicarbonate-supporting Fe2O3@Pt (after etching) on 4-NP reduction reaction with different conditions were investigated. As shown in Fig. 7a, the catalytic activity of Fe3O4@Pt@SiO2 prepared under different conditions (without MES, MES, MES + PVP and MES + CTAB) are similar, and the catalytic rate are very slow due to the encapsulation of SiO2. During the etching process (Fig. 7b–e), the catalytic rate becomes slower and slower. When the excess Na2CO3 is washed away after 9 hours of etching, the catalytic rate is significantly higher than that before etching. It is believed that the rate determining step of the reaction is the reaction between the adsorbed 4-NP and the surface hydrogen species released from NaBH4 on the catalyst. Wheat spike shaped sodium sesquicarbonate-supporting Fe2O3@Pt has an interconnected network structure to provide sufficient channels for mass transfer. At the same time, a large amount of Fe2O3@Pt is exposed on its surface, which is conducive to the direct contact between Fe2O3@Pt reaction sites and reactant molecules, thus improving the catalytic rate of 4-NP reduction reaction.
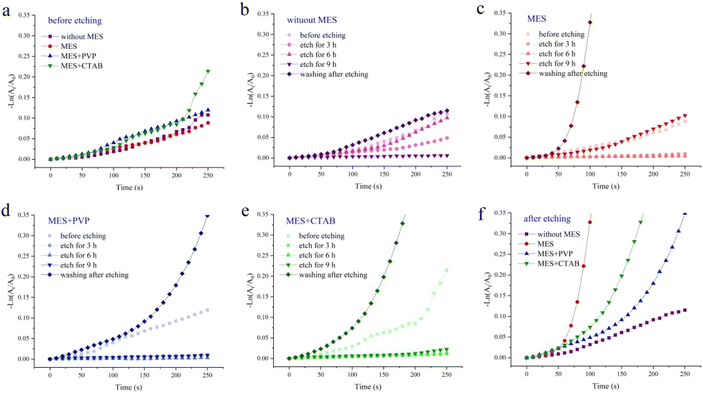 |
| Fig. 7 Plots of −ln(At/A0) versus the reaction time t for the reduction of 4-NP catalyzed by: (a) Fe3O4@Pt@SiO2, (b–f) sodium sesquicarbonate-supporting Fe2O3@Pt under different preparation conditions. | |
The sodium sesquicarbonate-supporting Fe2O3@Pt prepared under different conditions (without MES, MES, MES + PVP and MES + CTAB) showed great differences in their catalytic properties (Fig. 7f). Owing to the hindrance of PVP and CTAB, the etched sodium sesquicarbonate-supporting Fe2O3@Pt with PVP or CTAB showed the lower catalytic rate. However, sodium sesquicarbonate-supporting Fe2O3@Pt with MES showed the best catalytic performance due to the reduction protection characteristics of MES.32,40 The rate constants kapp was calculated to be 13.98 × 10−3 s−1 from the slope of the plots (Fig. S7†). Another significant parameter turnover frequency (TOF), defined as moles of reactant reduced through per mole of catalyst per second, was calculated to be 78 h−1. The comparison of the kinetic parameter of sodium sesquicarbonate-supporting Fe2O3@Pt with that of previous work was shown in Table S1.†
To further verify the synergistic effect between Fe and Pt, the catalytic effects of sodium sesquicarbonate-supporting Fe2O3@Pt and sodium sesquicarbonate-supporting Pt and Fe2O3 alone on 4-NP reduction were compared. It can be seen from Fig. S8† that the catalytic effect of sodium sesquicarbonate-supporting Fe2O3@Pt is much better than that of sodium sesquicarbonate-supporting Pt and Fe2O3 alone. And the conversion of 4-NP was up to 90% in 4 min (Fig. S9†), which is much better than loading Pt and Fe separately. Therefore, the introduction of non-metallic Fe can not only reduce the consumption of precious metal Pt, but also improve the catalytic efficiency due to the synergistic effect.
In conclusion, the prepared sodium sesquicarbonate structure can not only prevent the aggregation of Pt and Fe2O3, but also ensure the interaction between Pt and Fe, and improve the catalytic activity for 4-NP reduction. The material could be used in heterogeneous catalysis, water treatment and green chemistry.
Conclusions
In summary, the sodium sesquicarbonate-supporting Fe2O3@Pt with interconnected one-dimensional network structure is developed via etching Fe3O4@Pt@SiO2. The supporting role of sodium sesquicarbonate is not only prevent the aggregation of pt clusters and Fe2O3 nanoparticles, but also provide sufficient channels for mass transfer. At the same time, due to the synergistic effect between Pt and Fe, sodium sesquicarbonate-supporting Fe2O3@Pt shows good catalytic activity for 4-NP reduction. Moreover, the introduction of non-metallic Fe can not only reduce the consumption of precious metal Pt, but also improve the catalytic efficiency due to the synergistic effect. In conclusion, we believe the one-dimensional sodium sesquicarbonate-supporting Fe2O3@Pt will be attractive for broad applications in heterogeneous catalysis, water treatment and green chemistry.
Author contributions
Xia Xu: methodology, writing – original draft. Liming Yang: characterization of the TEM and collected the data performed the analysis. Mingqiang Li: synthesis nanomaterials collected the data. Bing Hu: writing – review & editing, funding acquisition. Xia Xu: designed the experiment and wrote the revised the paper.
Conflicts of interest
There are no conflicts to declare
Acknowledgements
The authors are grateful for the financial aid from the National Natural Science Foundation of China (Grant Nos. 21961001), Natural Science Foundation of Gansu Province (21JR7RA829), Innovation fund of Gansu Provincial Department of Education (2021B-121) and the Scientific research start-up funds for openly-recuited doctors provided by Gansu Agricultural University (GAU-KYQD-2018-14).
Notes and references
- T. Aditya, A. Pal and T. Pal, Nitroarene reduction: a trusted model reaction to test nanoparticle catalysts, Chem. Commun., 2015, 51, 9410 RSC
. - J. Q. Li, L. X. Zhong, L. M. Tong, Y. Yu, Q. Liu, S. C. Zhang, C. Yin, L. Qiao, S. Z. Li, R. Si and J. zhang, Atomic Pd on graphdiyne/graphene heterostructure as efficient catalyst for aromatic nitroreduction, Adv. Funct. Mater., 2019, 29, 1905423 CrossRef CAS
. - T. J. Martin, A. K. Goodhead, K. Acharya, I. M. Head, J. R. Snape and R. J. Davenport, High throughput biodegradation-screening test to prioritize and evaluate chemical biodegradability, Environ. Sci. Technol., 2017, 51, 7236 CrossRef CAS PubMed
. - S. Y. Liu, C. Lai, B. S. Li, C. Zhang, M. M. Zhang, D. L. Huang, L. Qin, H. Yi, X. G. Liu, F. L. Huang, X. R. Zhou and L. Chen, Role of radical and non-radical pathway in activating persulfate for degradation of p-nitrophenol by sulfurdoped ordered mesoporous carbon, Chem. Eng. J., 2020, 384, 123304 CrossRef CAS
. - S. S. Cao, J. Chang, L. Fang and L. M. Wu, Metal nanoparticles confined in the nanospace of doubleshelled hollow silica spheres for highly efficient and selective catalysis, Chem. Mater., 2016, 28, 5596 CrossRef CAS
. - M. J. Vaidya, S. M. Kulkarni and R. V. Chaudhari, Synthesis of p-aminophenol by catalytic hydrogenation of p-nitrophenol, Org. Process Res. Dev., 2003, 7, 202 CrossRef CAS
. - A. Chinnappan, S. K. Eshkalak, C. Baskar, M. Khatibzadeh, E. Kowsari and S. Ramakrishna, Flower-like 3-dimensional hierarchical Co3O4/NiO microspheres for 4-nitrophenol reduction reaction, Nanoscale Adv., 2019, 1, 303 RSC
. - A. S. Hashimi, M. A. N. M. Nohan, S. X. Chin, S. Zakaria and C. H. Chia, Rapid catalytic reduction of 4-nitrophenol and clock reaction of methylene blue using copper nanowires, Nanomaterials, 2019, 9, 936 CrossRef CAS PubMed
. - A. A. Kassem, H. N. Abdelhamid, D. M. Fouad and S. A. Ibrahim, Catalytic reduction of 4-nitrophenol using copper terephthalate frameworks and CuO@C composite, J. Environ. Chem. Eng., 2021, 9, 104401 CrossRef CAS
. - N. Li, P. X. Zhao and D. Astruc, Anisotropic gold nanoparticles: synthesis, properties, applications, and toxicity, Angew. Chem., Int. Ed., 2014, 53, 1756 CrossRef CAS PubMed
. - X. L. Sun, D. G. Li, Y. Ding, W. L. Zhu, S. J. Guo, Z. L. Wang and S. H. Sun, Core/shell Au/CuPt nanoparticles and their dual electrocatalysis for both reduction and oxidation reactions, J. Am. Chem. Soc., 2014, 136, 5745 CrossRef CAS PubMed
. - W. B. Lu, R. Ning, X. Y. Qin, Y. W. Zhang, G. H. Chang, S. Liu, Y. L. Luo and X. P. Sun, Synthesis of Au nanoparticles decorated graphene oxide nanosheets: Noncovalent functionalization by TWEEN 20 in situ reduction of aqueous chloroaurate ions for hydrazine detection and catalytic reduction of 4-nitrophenol, J. Hazard. Mater., 2011, 197, 320 CrossRef CAS PubMed
. - G. H. Chang, Y. L. Luo, W. B. Lu, X. Y. Qin, A. M. Asiri, A. O. Al-Youbi and X. P Sun, Ag nanoparticles decorated polyaniline nanofibers: synthesis, characterization, and applications toward catalytic reduction of 4-nitrophenol and electrochemical detection of H2O2 and glucose, Catal. Sci. Technol., 2021, 2, 800 RSC
. - Z. P. Dong, X. D. Le, C. X. Dong, W. Zhang, X. L. Li and J. T. Ma, Ni@Pd core-shell nanoparticles modified fibrous silica nanospheres as highly efficient and recoverable catalyst for reduction of 4-nitrophenol and hydrodechlorination of 4-chlorophenol, Appl. Catal., B, 2015, 162, 372 CrossRef CAS
. - J. Zeng, Q. Zhang, J. Y. Chen and Y. N. Xia, A comparison study of the catalytic properties of Au-based nanocages, nanoboxes, and nanoparticles, Nano Lett., 2010, 10, 30 CrossRef CAS PubMed
. - S. M. Ansar and C. L. Kitchens, Impact of gold nanoparticle stabilizing ligands on the colloidal catalytic reduction of 4-nitrophenol, ACS Catal., 2016, 6, 5553 CrossRef CAS
. - J. A. Johnson, J. J. Makis, K. A. Marvin, S. E. Rodenbusch and K. J. Stevenson, Size-dependent hydrogenation of p-nitrophenol with Pd nanoparticles synthesized with poly(amido)amine dendrimer templates, J. Phys. Chem. C, 2013, 117, 22644 CrossRef CAS
. - A. M. Kalekar, K. K. K. Sharma, M. N. Luwang and G. K. Sharma, Catalytic activity of bare and porous palladium nanostructures in the reduction of 4-nitrophenol, RSC Adv., 2016, 6, 11911 RSC
. - K. D. O. Santos, W. C. Elias, A. M. Signori, F. C. Giacomelli, H. Yang and J. B. Domingos, Synthesis and catalytic properties of silver nanoparticle-linear polyethylene imine colloidal systems, J. Phys. Chem. C, 2012, 116, 4594 CrossRef CAS
. - B. Baruah, G. J. Gabriel, M. J. Akbashev and M. E. Booher, Facile synthesis of silver nanoparticles stabilized by cationic polynorbornenes and their catalytic activity in 4-nitrophenol reduction, Langmuir, 2013, 29, 4225 CrossRef CAS PubMed
. - S. Wunder, F. Polzer, Y. Lu, Y. Mei and M. Ballauff, Kinetic analysis of catalytic reduction of 4-nitrophenol by metallic nanoparticles immobilized in spherical polyelectrolyte brushes, J. Phys. Chem. C, 2010, 114, 8814 CrossRef CAS
. - S. Pandey and S. B. Mishra, Catalytic reduction of p-nitrophenol by using platinum nanoparticles stabilised by guar gum, Carbohydr. Polym., 2014, 113, 525 CrossRef CAS PubMed
. - S. Varshney, R. Bar-Ziv and T. Zidki, On the remarkable performance of silver-based alloy nanoparticles in 4-nitrophenol catalytic reduction, ChemCatChem, 2020, 12, 4680 CrossRef CAS
. - L. R. Shultz, L. Hu, K. Preradovic, M. J. Beazley, X. F. Feng and T. Jurca, A broader-scope analysis of the catalytic reduction of nitrophenols and azo dyes with noble metal nanoparticles, ChemCatChem, 2019, 11, 2590 CrossRef CAS
. - J. Zhang, S. F. Lu, Y. Xiang and S. P. Jiang, Intrinsic effect of carbon supports on the activity and stability of precious metal based catalysts for electrocatalytic alcohol oxidation in fuel cells, ChemSusChem, 2020, 13, 2484 CrossRef CAS PubMed
. - A. N. Chishti, F. Guo, A. Aftab, Z. Y. Ma, Y. Liu, M. Chen, J. Gautam, C. Chen, L. B. Ni and G. W. Diao, Synthesis of silver doped Fe3O4/C nanoparticles and its catalytic activities for the degradation and reduction of methylene blue and 4-nitrophenol, Appl. Surf. Sci., 2021, 546, 149070 CrossRef CAS
. - F. Saira, N. Firdous, R. Qureshi and A. Ihsan, Preparation and catalytic evaluation of Au/γ-Al2O3 nanoparticles for the conversion of 4-nitrophenol to 4-aminophenol by spectrophotometric method, Turk. J. Chem., 2020, 44, 448 CrossRef CAS PubMed
. - F. F. Zhang, P. Yang and K. Matras-Postolek, Au catalyst decorated silica spheres: synthesis and high-performance in 4-nitrophenol reduction, J. Nanosci. Nanotechnol., 2016, 16, 5966 CrossRef CAS PubMed
. - G. Z. Chen, Y. Wang, Y. W. Wei, W. Zhao, D. W. Gao, H. X. Yang and C. C. Li, Successive interfacial reaction-directed synthesis of CeO2@Au@CeO2-MnO2 environmental catalyst with sandwich hollow structure, ACS Appl. Mater. Interfaces, 2018, 10, 11595 CrossRef CAS PubMed
. - Y. Long, J. Li, L. L. Wu, J. Q. Li, X. Wang, S. Yao, S. Y. Song and H. J. Zhang, One-pot synthesis of cobalt-doped Pt-Au alloy nanoparticles supported on ultrathin α-Co(OH)2 nanosheets and their enhanced performance in the reduction of p-nitrophenol, Eur. J. Inorg. Chem., 2017, 1, 146 CrossRef
. - A. T. Ezhil Vilian, S. R. Choe, K. Giribabu, S. C. Jang, C. Roh, Y. S. Huh and Y. K. Han, Pd nanospheres decorated reduced graphene oxide with multi-functions: highly efficient catalytic reduction and ultrasensitive sensing of hazardous 4-nitrophenol pollutant, J. Hazard. Mater., 2017, 333, 54 CrossRef PubMed
. - M. R. Fu, M. Q. Li, Y. Y. Zhao, Y. X. Bai, X. Z. Fang, X. L. Kang, M. Yang, Y. P. Wei and X. Xu, A study on the high efficiency reduction of pnitrophenol (4-NP) by a Fe(OH)3/Fe2O3@Au composite catalyst, RSC Adv., 2021, 11, 26502 RSC
. - Q. Ding, Z. W. Kang, L. P. Cao, M. S. Lin, H. T. Lin and D.-P. Yang, Conversion of waste eggshell into difunctional Au/CaCO3 nanocomposite for 4-nitrophenol electrochemical detection and catalytic reduction, Appl. Surf. Sci., 2020, 510, 145526 CrossRef CAS
. - J. Feng, L. Su, Y. H. Ma, C. L. Ren, Q. Guo and X. G. Chen, CuFe2O4 magnetic nanoparticles: A simple and efficient catalyst for the reduction of nitrophenol, Chem. Eng. J., 2013, 221, 16 CrossRef CAS
. - Y. M. Hunge, A. A. Yadav, S. W. Kang, H. Kim, A. Fujishima and C. Terashima, Nanoflakes-like nickel cobaltite as active electrode material for 4-nitrophenol reduction and supercapacitor applications, J. Hazard. Mater., 2021, 419, 126453 CrossRef CAS PubMed
. - S. L. Xie, Z. Xu, C. Yu, X. L. Yu, Z. H. Zhang and J. B. Li, Highly efficient reduction of 4-nitrophenol by Cu nanoparticle decorated graphdiyne, ChemistrySelect, 2021, 6, 13572 CrossRef CAS
. - B. Y. Xia, H. B. Wu, N. Li, Y. Yan, X. W. Lou and X. Wang, One-pot synthesis of Pt-Co alloy nanowire assemblies with tunable composition and enhanced electrocatalytic properties, Angew. Chem., Int. Ed., 2015, 54, 3797 CrossRef CAS PubMed
. - X. F. Tan, J. Qin, Y. Li, Y. T. Zeng, G. X. Zheng, F. Feng and H. Li, Self-supporting hierarchical PdCu aerogels for enhanced catalytic reduction of 4-nitrophenol, J. Hazard. Mater., 2020, 397, 122786 CrossRef CAS PubMed
. - J. Qin, X. F. Tan, F. Feng and H. Li, Facile and controllable synthesis of AuCu aerogels for the enhanced degradation of 4-nitrophenol, Appl. Surf. Sci., 2021, 561, 150024 CrossRef CAS
. - X. Xu, M. R. Fu, M. Yang, B. Hu, J. T. Yang, W. J. Gui and J. X. Guo, NaYF4:Yb3+(58%),Tm3+@NaYF4@Au nanocomposite for 4-nitrophenol ultrasensitive quantitative detection and highly efficient catalytic reduction, New J. Chem., 2022, 46, 7385 RSC
. - G. R. Zhang and B. Q. Xu, Surprisingly strong effect of stabilizer on the properties of Au nanoparticles and Pt^Au nanostructures in electrocatalysis, Nanoscale, 2010, 2, 2798 RSC
. - C. Wang, W. D. Tian, Y. Ding, Y. Q. Ma, Z. L. Wang, N. M. Markovic, V. R. Stamenkovic, H. Daimon and S. H. Sun, Rational synthesis of heterostructured nanoparticles with morphology control, J. Am. Chem. Soc., 2010, 132, 6524 CrossRef CAS PubMed
.
|
This journal is © The Royal Society of Chemistry 2023 |