DOI:
10.1039/D3RA01842C
(Paper)
RSC Adv., 2023,
13, 9924-9931
Magnetization reversal of perpendicular magnetic anisotropy regulated by ferroelectric polarization in CoFe3N/BaTiO3 heterostructures: first-principles calculations
Received
21st March 2023
, Accepted 29th March 2023
First published on 5th April 2023
Abstract
Exploring the electric-field switching of perpendicular magnetic anisotropy (PMA) in multiferroic heterostructures has important physical significance, which attracts great interest due to its promising application for energy-efficient information storage. Herewith, we investigate the effect of ferroelectric polarization on magnetic anisotropy in CoFe3N/BaTiO3 heterostructures using first-principles calculations. The calculations reveal that the magnetic anisotropy of CoFe3N can be regulated by ferroelectric polarization of BaTiO3. When the ferroelectric polarization reverses, the PMA of FeCo–TiO2 and FeN–BaO configurations remains, but in the FeN–TiO2 and FeCo–BaO cases, magnetic anisotropy inverses between out-of-plane and in-plane direction. Further orbital-resolved analysis indicates that the transition of magnetic anisotropy is mainly attributed to the orbital hybridization of interfacial Fe/Co atoms with O atoms induced by the magnetoelectric effect. This study may open an effective approach toward modulating PMA and lays a foundation to the development of low energy consumption memory devices.
Introduction
With the rapid development of information technology and the continuous advancement of spintronics, perpendicular magnetic anisotropy (PMA) has attracted intensive attention with potential application in energy-efficient information memory.1–3 PMA is beneficial to obtain higher storage density, higher thermal stability and lower critical switching current density in non-volatile magnetic random access memory (MRAM).4–6 PMA mainly arises at ferromagnetic/oxide interfaces, such as Fe/MgO,6,7 CoFeB/MgO,5 CoFeB/BaTiO3,8 Mn3Ga/SrTiO3,9 and (Co/Pt)3/PMN-PT.10 It is also interesting that the magnetism of memory devices could be controlled by using an electric field through magnetoelectric (ME) coupling in multiferroic heterostructures. The ME effect at ferromagnetic/ferroelectric heterostructures also reduces the energy dissipation by Joule heating, which becomes another hot topic of information memory.11–15 Various mechanisms on ME effect have been proposed and confirmed, such as strain,16,17 charge,18 and exchange-coupling.19–21 More importantly, multiferroic heterostructures with PMA provide more effective method for achieving the magnetization switching via electric field, which will not only save energy consumption, but also expand the future application for non-volatile MRAM device.22,23
Among ferromagnets, anti-perovskite material Fe4N has been reported as a very attractive candidate for spintronics applications due to high spin polarization, small coercivity and high Curie temperature.24–26 In our previous experiment, epitaxial Fe4N films can be grown on MgO, PMN-PT, and SrTiO3 substrates and they all exhibit good interface and magnetic properties.27–29 Experiment results show that the ME effect and magnetic anisotropy of Fe4N/PMN-PT heterostructure can be tailored by electric field,27,30 further confirmed by theoretical calculation.31 Fe4N/MgO/Fe4N magnetic tunnel junction (MTJ) has been predicted to produce an ultrahigh tunnel magnetoresistance (TMR),32 Fe4N/Alq3/Co and LSMO/C60/Fe4N-MTJs exhibit a negative TMR and a regulable interface.33,34 PMA has been obtained in Fe4N/BiFeO3, Fe4N/MgO, and Fe4N/PMN-PT heterostructure and it also can be tuned by strain, interfacial oxidation, ferroelectric polarization, and electric field.31,35–38 However, the electric field switching of PMA does not achieve in Fe4N-based heterostructure by ferroelectric polarization. Bulk Fe4N is cubic symmetry and it does not have PMA, but the substitution of Co in the Fe4N lattice induces a large PMA of tetragonal CoFe3N.39 By adsorbing organic molecules, organic/CoFe3N spinterface exhibits enhanced PMA.40 Moreover, the Mn-substitution doping at the interfacial FeII position in the Fe4N/BaTiO3 heterostructure may obtain a large ME effect.41 In order to further improve the interfacial PMA and achieving the electric field switching of PMA, the substitute CoFe3N is introduced as the ferromagnetic layer of multiferroic heterostructure, which has a great application prospect for spintronics.
In this work, we construct the CoFe3N/BaTiO3 multiferroic heterostructures and investigate the impact of ferroelectric polarization on magnetic anisotropy using first-principles calculations. Our studies indicate that the magnetic anisotropy of CoFe3N can be regulated by ferroelectric polarization of BaTiO3. Ferroelectric polarization reversal makes flipping of magnetization between the out-of-plane and in-plane direction in FeN–TiO2 and FeCo–BaO interface configurations. Further orbital-resolved analysis indicates that the transition of magnetic anisotropy is mainly due to the strong interfacial ME coupling and orbital hybridization between interfacial Fe/Co atom and O atom. These results lay the foundation for realizing electric-field control of PMA in multiferroic heterostructures.
Computational details and models
First-principles calculations are carried out based on density functional (DFT) theory using Vienna Ab initio Simulation Package code.42–44 Exchange and correlation effects are accounted for by the generalized gradient approximation (GGA) as parameterized by Perdew, Burke, and Ernzerhof (PBE).45 Although the PBE functional has an overestimation for unit cell volume,46 extensive studies demonstrate that it is suitable for the study of multiferroic heterostructure.17,47,48 Structural relaxations are performed until the force becomes less than 10−2 eV Å−1 and the change in the total energy between two ionic relaxation steps is smaller than 10−5 eV. An energy cutoff of 500 eV and 9 × 9 × 9, 11 × 11 × 1 k-point meshes are used for the bulk and heterostructures, respectively. The CoFe3N/BaTiO3 heterostructures comprise seven-layered CoFe3N and seven-layered BaTiO3, followed by a 15 Å vacuum layer. The experimental lattice constant of bulk BaTiO3 is 3.99 Å and the in-plane lattice constant of bulk CoFe3N is 3.78 Å,39 which has a lattice mismatch of 5.3%. Here, we build the CoFe3N/BaTiO3 heterostructures is based on the previous experiment research of Fe4N/oxide heterostructure.27,28,30 The binding energy (EB) calculation is preformed to display the structural stability of different interface configurations. The magnetic anisotropy energy (MAE) is determined using the magnetic force theorem with spin–orbit coupling (SOC).49–51 The MAE depends on the nonzero coupling matrix element between the occupied and unoccupied d-orbital states.51 The total MAE values of CoFe3N/BaTiO3 heterostructures are calculated from the energy difference between the magnetic moment aligning in the in-plane (x axis) and out-of-plane (z axis) orientations. The positive value of MAE represents PMA and negative value represents in-plane magnetic anisotropy (IMA).
Results and discussion
Prior to investigating the regulated magnetic anisotropy by ferroelectric polarization, we first discuss the properties of bulk CoFe3N and BaTiO3, as shown in Fig. 1. In tetragonal CoFe3N, the corner site is occupied by Fe atom, named it FeI, the Fe atoms at face-centered site are named by FeII, and another face-centered site is occupied by Co atom, as shown in Fig. 1(a). The strong hybridization between FeII and N atoms makes distinct density of states in FeI and FeII atoms,32,52 so FeI and FeII atoms exhibit outstanding difference in magnetic properties. In bulk CoFe3N, the magnetic moments of FeI, FeII, and Co atoms are 2.987 μB, 2.229 μB, and 1.180 μB, respectively. In Fig. 1(c), the MAE results of CoFe3N show that FeI, FeII, and Co atoms have a noticeable difference. FeI atom has an IMA contribution whereas FeII atom exhibits a PMA. Furthermore, Co atom shows a large PMA of 0.319 meV. We then display the orbital-resolved MAE of Fe and Co atoms in Fig. 1(d)–(f) to analysis the orbital contribution for MAE. In FeI, nonzero coupling matrix element 〈x2−y2|
z|xy〉 favors IMA, but it turns to support PMA in FeII and Co atoms. The difference in chemical environment of FeI, FeII, and Co atoms determines the d-orbital distribution near the Fermi level,32,38,52 finally altering the magnetic moment and MAE of Fe and Co atoms. Fig. 1(g) displays the total density of states (DOS) and partial DOS of bulk CoFe3N. The results show that there is spin majority states of FeII and Co atoms pass through the Fermi level whereas there are not spin majority d states near the Fermi level in FeI atom, which leads to the distinct magnetic properties. Fig. 1(b) shows that bulk BaTiO3 has a typical perovskite structure and the DOS result in Fig. 1(h) indicates that it is a non-magnetic insulator.53
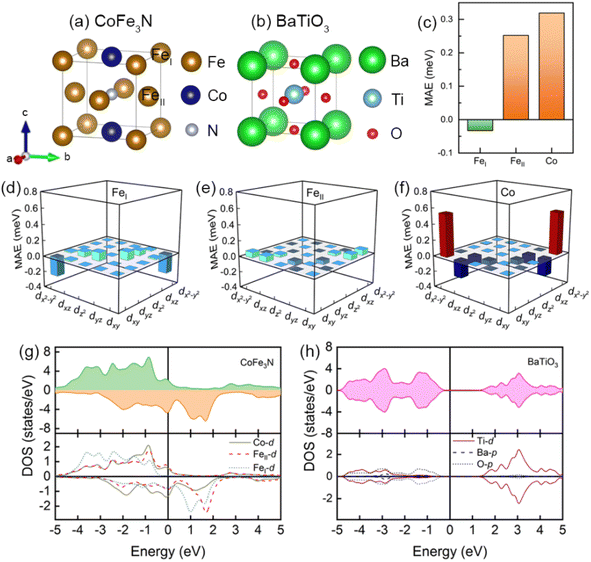 |
| Fig. 1 (a) and (b) The lattice structure of bulk CoFe3N and BaTiO3. (c) MAE of Fe and Co atoms in bulk CoFe3N. (d)–(f) Orbital-resolved MAE of Fe and Co atoms in bulk CoFe3N. (g) and (h) Total and partial density of states (DOS) of bulk CoFe3N and BaTiO3. | |
We then built CoFe3N/BaTiO3 heterostructures with ideal interface, and complex interface situations such as oxygen diffusion, disorder, and lattice distortion are not considered in this work. Four possible ideal interface structures are taken into account, namely, FeCo–TiO2, FeN–TiO2, FeCo–BaO, and FeN–BaO type interface, as shown in Fig. 2. The grey arrows indicate the polarization direction of BaTiO3. The positive ferroelectric polarization along the z axis is pointing away from the BaTiO3. It should be pointed that interfacial Fe atom in FeCo termination is FeI and interfacial Fe atom in FeN termination is FeII. In order to explore the influence of interface structure on magnetic anisotropy, we first analyze the interface bonding and structure difference. In FeCo–TiO2 configuration, interfacial Co atom occupies atop sites on Ti atom. Polarization reversal makes the interfacial Co atom shift away from the interface. In FeN–TiO2 configuration, interfacial FeII atom bonds with O atom. Such a Fe–O bond leads to d-orbital reconstruction, which in turn affects the magnetic properties of CoFe3N part with the presence of ferroelectric polarization. Intriguingly, interfacial Co and O atoms are bonded strongly and the bond length is only 1.811 Å in FeCo–BaO (P↓) case, making the overlap between Co-3d and O-2p states stronger, whereas Co and O atoms are far away from each other in FeCo–BaO (P↑) case. In FeN–BaO configuration, regardless of polarization direction, they all exhibit a large interface distance. The significant change of interface structure with the process of polarization reversal may cause the change in electronic structure, spin magnetism, and MAE.53,54
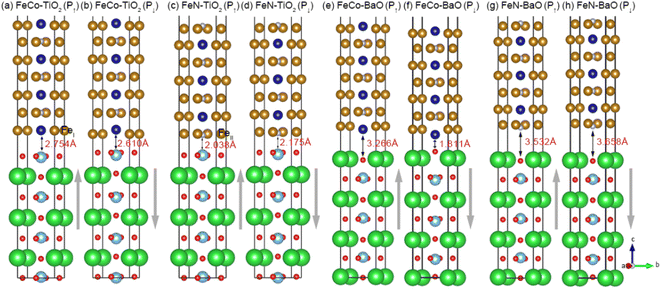 |
| Fig. 2 The CoFe3N/BaTiO3 heterostructures with different configurations. (a) and (b) FeCo–TiO2, (c) and (d) FeN–TiO2, (e) and (f) FeCo–BaO, and (g) and (h) FeN–BaO. The grey arrows indicate the polarization direction of BaTiO3. The interfacial Fe and Co atoms and interface distance are marked. | |
In Table 1, we first list the EB of CoFe3N/BaTiO3 heterostructures with different configurations to determine the stability of heterostructures, which is defined as EB = ECoFe3N/BaTiO3 − ECoFe3N − EBaTiO3. ECoFe3N and EBaTiO3 represent the energies of the surface of CoFe3N part and BaTiO3 part, and ECoFe3N/BaTiO3 is the total energy of CoFe3N/BaTiO3 heterostructure.47,53 The negative EB indicates that FeCo–BaO and FeN–TiO2 configurations are experimentally feasible. FeCo–TiO2 (P↑) and FeN–BaO (P↓) configurations have positive values, indicating the process of polarization reversal may affect the interface stability.
Table 1 EB (eV), magnetic moment (μB), and MAE (mJ m−2) of CoFe3N/BaTiO3 heterostructures with different configurations
Configuration |
EB |
μTi/Bas |
μOs |

|
μCos |
MAE |
FeCo–TiO2 (P↑) |
0.181 |
−0.229 |
0.012 |
3.114 |
1.206 |
0.978 |
FeCo–TiO2 (P↓) |
−0.062 |
−0.116 |
0.012 |
3.251 |
−0.562 |
1.138 |
FeN–TiO2 (P↑) |
−2.476 |
−0.025 |
0.056 |
2.610 |
— |
−0.043 |
FeN–TiO2 (P↓) |
−1.302 |
0.006 |
0.049 |
2.646 |
— |
5.139 |
FeCo–BaO (P↑) |
−0.338 |
−0.006 |
−0.158 |
2.956 |
1.257 |
0.894 |
FeCo–BaO (P↓) |
−1.336 |
0.016 |
0.158 |
2.846 |
1.591 |
−0.181 |
FeN–BaO (P↑) |
−0.367 |
−0.001 |
−0.138 |
2.476 |
— |
2.532 |
FeN–BaO (P↓) |
1.005 |
−0.000 |
0.004 |
2.467 |
— |
1.958 |
Table 1 also gives the magnetic moments of the interfacial Ti, Ba, O, Fe, and Co atoms in CoFe3N/BaTiO3 heterostructures with P↑ and P↓. The appearance of magnetic moment in Ti and O atoms can be attributed to the orbital hybridization with Fe/Co atom, which demonstrates strong ME effect at the interface.48,55 In FeCo–TiO2 configuration, the magnetic moment of FeI atom has a marked increase compared to the bulk CoFe3N, owing to the strong Fe 3d and O 2p orbital hybridization. The polarization reversal gives rise to an inversion of Co magnetic moment from 1.206 μB (P↑) to −0.562 μB (P↓), indicating that the magnetic structure can be regulated by BaTiO3 polarization. In FeN–TiO2 configuration, the magnetic moment of FeII atom has a tiny change with the polarization reversal. In FeCo–BaO configuration, the magnetic moment of O atom can be switched from parallel to antiparallel to the Co atom. This means that a strong ME coupling is formed in FeCo–BaO configuration, which has a good application prospect in spintronics. In addition, the total MAEs of CoFe3N/BaTiO3 heterostructures are also shown in Table 1. For FeN–TiO2 and FeCo–BaO configuration, by changing the polarization direction, the easy magnetic axis is switched between out-of-plane direction and in-plane direction. Especially, in FeN–TiO2 configuration, negative polarization makes the PMA increase significantly, confirming that polarization reversal produces a sizable change in magnetic anisotropy. However, regardless of polarization direction, PMA remains in FeCo–TiO2 and FeN–BaO configurations.
To further clarify the origin of MAE, layer-resolved MAEs are shown in Fig. 3(a) and (b). For both polarization directions, MAE changes mainly in the interfacial and surface layer, owing to the anisotropic nature resides at the interface or surface.48 In Fig. 3(a), interfacial MAE has a sharp decline in FeCo–TiO2 configuration when the magnetoelectric effect is applied as P↑ → P↓. In FeN–TiO2 configuration, the magnitude of MAE in every layer has an increase with the polarization reversal. In Fig. 3(b), FeCo–BaO configurations with P↑ and P↓ states exhibit distinct trends in interfacial MAE, being positive and negative in sign, respectively. This indicates that the magnetization direction can reorient from out-of-plane easy axis to in-plane by reversing the polarization direction. In FeN–BaO configuration, interfacial MAE is almost unchanged as P↑ → P↓. Further, to get more insights, we analyze the atom-resolved MAE of interfacial Fe and Co atoms, as shown in Fig. 3(c) and (d). The interfacial Fe/Co atom plays an important role in the electronic structure near the Fermi level and interfacial magnetic anisotropy. In FeCo–TiO2 configuration, the MAE of FeI and Co atoms decrease severely. In FeN–TiO2 configuration, both FeII atoms favor the PMA and PMA increases at P↓ state. In FeCo–BaO configuration, FeI favors PMA and yet Co supports IMA, which is contrary to the bulk CoFe3N. When the polarization reverses, the opposite MAE of FeI and Co atoms brings about the changed sign of interfacial MAE. In FeN–BaO configuration, the MAE of interfacial FeII atoms changes little during P↑ → P↓.
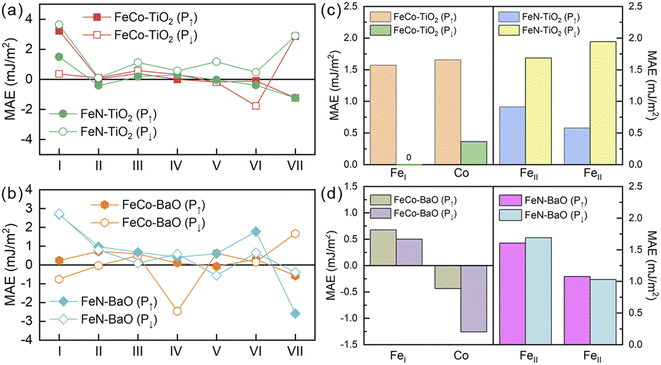 |
| Fig. 3 (a) and (b) The layer-resolved MAE of CoFe3N/BaTiO3 heterostructures with TiO2 and BaO terminations. (c) and (d) The MAE of interfacial Fe and Co atoms of CoFe3N/BaTiO3 heterostructures with TiO2 and BaO terminations. | |
To elucidate the effect of interfacial structure and polarization reversal on PMA, we show the d-orbital-resolved MAE of interfacial Fe and Co atoms in Fig. 4. According to the second-order perturbation theory,50,51 the MAE depends on the nonzero coupling matrix element between the occupied and unoccupied d-orbital states. The MAE can be defined as
, where ψo and ψu indicate the occupied and unoccupied states with the energies Eo and Eu, respectively. ξ is the SOC constant.
z(x) is the z(x) component of the orbital angular momentum operator. These nonzero coupling matrix elements include 〈xz|
z|yz〉 = 1, 〈x2−y2|
z|xy〉 = 2, 〈z2|
x|yz〉 = 3, 〈xy|
x|xz〉 = 1, and 〈x2−y2|
x|yz〉 = 1.50
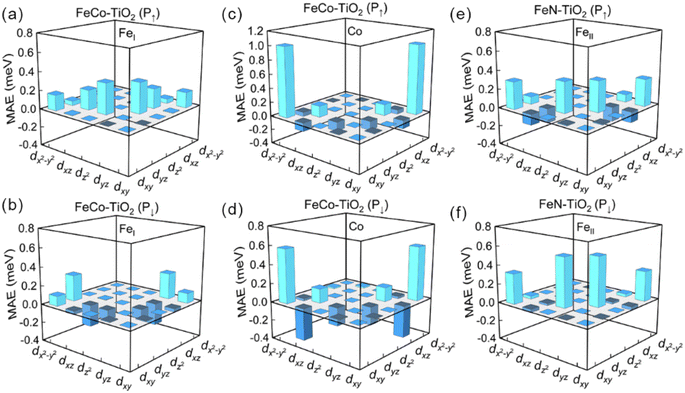 |
| Fig. 4 The orbital-resolved MAE of interfacial Fe and Co atoms in CoFe3N/BaTiO3 heterostructures with TiO2 termination. (a) and (b) FeI of FeCo-TiO2, (c) and (d) Co of FeCo-TiO2, (e) and (f) FeII of FeN-TiO2. | |
Meanwhile, in order to better explain the PMA contribution of matrix elements, we analyze the DOS of interfacial Fe and Co atoms from the d-orbital perspective in Fig. 6. In FeCo–TiO2 (P↑) case, for FeI atom, all nonzero matrix elements provide the positive contribution to MAE. Among them, the contribution of 〈z2|
x|yz〉 is the most and it favors PMA. In FeCo–TiO2 (P↓) case, these two terms of 〈z2|
x|yz〉 and 〈xz|
z|yz〉 turn to support IMA, which cancels out the PMA contribution and makes the MAE of FeI atom zero. According to DOS of Fig. 6(a) and (b), during the P↑ → P↓, spin majority electron in dyz occupied states of FeI atom disappears, which produces the negative contribution of 〈z2|
x|yz〉 and 〈xz|
z|yz〉 terms. For Co atom, the 〈x2−y2|
z|xy〉 matrix element always has a large PMA. However, the IMA contribution of 〈xy|
x|xz〉 increases as P↑ → P↓, causing the PMA of Co atom decreases, which is attributed to spin transition of dxz occupied state near the Fermi level. In FeN–TiO2 configuration, polarization reversal (P↑ → P↓) gives rise to the increase of PMA in interfacial FeII atom, which mainly comes from the growing PMA contribution of the matrix element 〈z2|
x|yz〉 and the reduced IMA contribution of 〈xz|
x|yz〉 term. Based on the DOS of FeII atom, dyz occupied state with spin down shifts to the Fermi level, decreasing the energy level difference and leading to the growing PMA contribution of the matrix element 〈z2|
x|yz〉 and the reduce of IMA contribution of 〈xz|
x|yz〉. It suggests that the MAE of the FeII atom is more sensitive to the external condition, which is consistent with Fe4N/MgO heterostructure.38
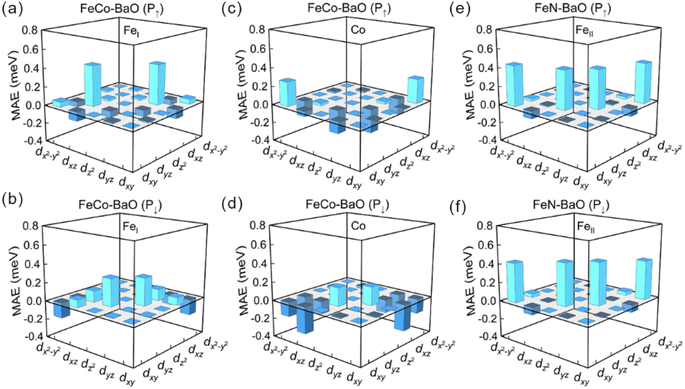 |
| Fig. 5 The orbital-resolved MAE of interfacial Fe and Co atoms in CoFe3N/BaTiO3 heterostructures with BaO termination. (a) and (b) FeI of FeCo-BaO, (c) and (d) Co of FeCo-BaO, (e) and (f) FeII of FeN-BaO. | |
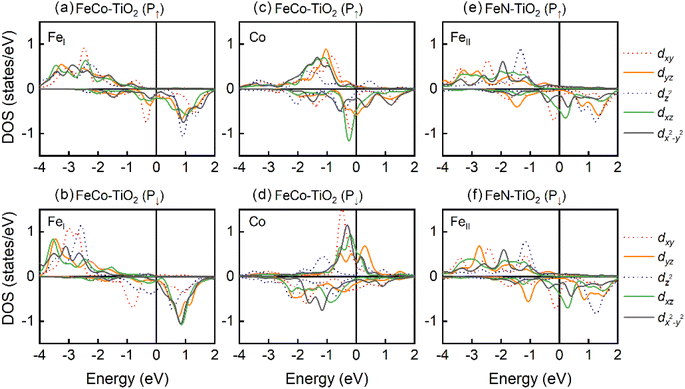 |
| Fig. 6 The DOS of interfacial Fe and Co atoms in CoFe3N/BaTiO3 heterostructures with TiO2 termination. (a) and (b) FeI of FeCo-TiO2, (c) and (d) Co of FeCo-TiO2, (e) and (f) FeII of FeN-TiO2. | |
Fig. 5 and 7 give the d-orbital-resolved MAE and DOS of interfacial Fe and Co atoms in FeCo–BaO and FeN–BaO structures. In FeCo–BaO configuration, during the P↑ → P↓, most of zero matrix elements of FeI atom changes the sign, which is ascribed to the appearance of majority 3d electrons including dyz, dz2,
occupied states. Distinguishingly, the PMA contribution of 〈x2−y2|
z|xy〉 of Co atom is no longer the dominant, even the contribution of 〈x2−y2|
z|xy〉 turn into IMA with the polarization reversal. The inversion of MAE contribution of 〈x2−y2|
z|xy〉 comes from the disappearance of dxy majority occupied states near the Fermi level. What's more, the MAE contributions of 〈x2−y2|
x|yz〉 and 〈z2|
x|yz〉 terms are also changed in sign. On the basis of DOS result, the disappearance of dyz minority occupied states plays a key role in the change of the MAE sign. In FeN–BaO termination, 〈x2−y2|
z|xy〉 and 〈z2|
x|yz〉 of interfacial FeII atom have a positive contribution. The contribution of all matrix elements remains stable during the polarization reversal as a result of the weak ME coupling and unchanged DOS.
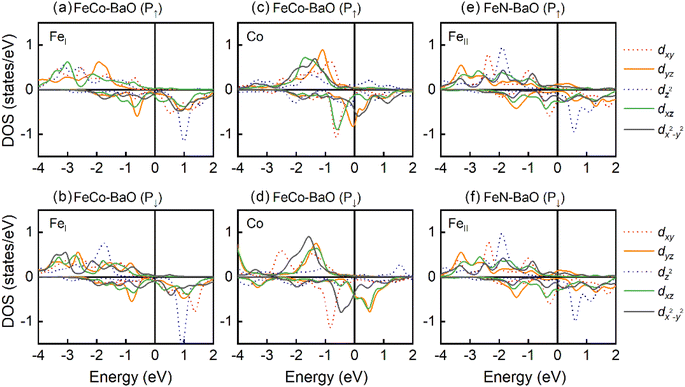 |
| Fig. 7 The DOS of interfacial Fe and Co atoms in CoFe3N/BaTiO3 heterostructures with BaO termination. (a) and (b) FeI of FeCo-BaO, (c) and (d) Co of FeCo-BaO, (e) and (f) FeII of FeN-BaO. | |
Conclusions
In summary, the effect of ferroelectric polarization on magnetic anisotropy in CoFe3N/BaTiO3 heterostructure has been demonstrated by first-principles calculations. We found that ferroelectric polarization reverses magnetic anisotropy of FeN–TiO2 and FeCo–BaO configurations. The interfacial FeI, FeII, and Co atoms play a crucial role in determining the magnetic anisotropy of CoFe3N/BaTiO3 heterostructures, which is attributed to the orbital hybridization between interfacial Fe/Co atom and O atom due to the ME coupling effect. This work paves the way for nonvolatile electrical control of multiferroic heterostructure and applications in spintronics devices.
Author contributions
Zirun Li conducted first-principles calculations and drafted this paper. Bo Chen, Shimin Shan and Yongmei Zhang have taken part in the revision.
Conflicts of interest
There are no conflicts of interest to declare.
Acknowledgements
This work was supported by the National Natural Science Foundation of China (Grant No. 12204438), and the Shanxi Province Science Foundation for Youths (Grant No. 201901D211264), and the Fundamental Research Program of Shanxi Province (Grant No. 202103021224185 and 202203021212116), and the Scientific and Technological Innovation Programs of Higher Education Institutions in Shanxi (Grant No. 2020L0289), and the Science Foundation of North University of China (Grant No. XJJ201905).
References
- I. Žutić, J. Fabian and S. D. Sarma, Rev. Mod. Phys., 2004, 76, 323–410 CrossRef.
- C. Song, B. Cui, F. Li, X. J. Zhou and F. Pan, Prog. Mater. Sci., 2017, 87, 33–82 CrossRef.
- S. Bhatti, R. Sbiaa, A. Hirohata, H. Ohno, S. Fukami and S. N. Piramanayagam, Mater. Today, 2017, 20, 530–548 CrossRef.
- B. Dieny and M. Chshiev, Rev. Mod. Phys., 2017, 89, 025008 CrossRef.
- S. Ikeda, K. Miura, H. Yamamoto, K. Mizunuma, H. D. Gan, M. Endo, S. Kanai, J. Hayakawa, F. Matsukura and H. Ohno, Nat. Mater., 2010, 9, 721–724 CrossRef CAS PubMed.
- J. W. Koo, S. Mitani, T. T. Sasaki, H. Sukegawa, Z. C. Wen, T. Ohkubo, T. Niizeki, K. Inomata and K. Hono, Appl. Phys. Lett., 2013, 103, 192401 CrossRef.
- F. Ibrahim, A. Hallal, A. Kalitsov, D. Stewart, B. Dieny and M. Chshiev, Phys. Rev. Appl., 2022, 17, 054041 CrossRef.
- W. Lin, B. Yang, A. P. Chen, X. Wu, R. Guo, S. Chen, L. Liu, Q. Xie, X. Shu, Y. Hui, G. M. Chow, Y. Feng, G. Carlotti, S. Tacchi, H. Yang and J. Chen, Phys. Rev. Lett., 2020, 124, 217202 CrossRef CAS PubMed.
- B. S. Yang, L. N. Jiang, W. Z. Chen, P. Tang, J. Zhang, X. G. Zhang, Y. Yan and X. F. Han, Appl. Phys. Lett., 2018, 112, 142403 CrossRef.
- B. Peng, Z. Zhou, T. Nan, G. Dong, M. Feng, Q. Yang, X. Wang, S. Zhao, D. Xian, Z. D. Jiang, W. Ren, Z. G. Ye, N. X. Sun and M. Liu, ACS Nano, 2017, 11, 4337–4345 CrossRef CAS PubMed.
- Y. H. Chu, L. W. Martin, M. B. Holcomb, M. Gajek, S. J. Han, Q. He, N. Balke, C. H. Yang, D. Lee, W. Hu, Q. Zhan, P. L. Yang, A. Fraile-Rodríguez, A. Scholl, S. X. Wang and R. Ramesh, Nat. Mater., 2008, 7, 478–482 CrossRef CAS PubMed.
- V. Garcia, M. Bibes, L. Bocher, S. Valencia, F. Kronast, A. Crassous, X. Moya, S. Enouz-Vedrenne, A. Gloter, D. Imhoff, C. Deranlot, N. D. Mathur, S. Fusil, K. Bouzehouane and A. Barthélémy, Science, 2010, 327, 1106–1110 CrossRef CAS PubMed.
- N. A. Spaldin and M. Fiebig, Science, 2005, 309, 391–392 CrossRef CAS PubMed.
- W. Eerenstein, N. D. Mathur and J. F. Scott, Nature, 2006, 442, 759–765 CrossRef CAS PubMed.
- N. A. Spaldin, S. W. Cheong and R. Ramesh, Phys. Today, 2010, 63, 38–43 CrossRef.
- S. Geprägs, A. Brandlmaier, M. Opel, R. Gross and S. T. B. Goennenwein, Appl. Phys. Lett., 2010, 96, 142509 CrossRef.
- D. Odkhuu and N. Kioussis, Phys. Rev. B, 2018, 97, 094404 CrossRef CAS.
- C. G. Duan, J. P. Velev, R. F. Sabirianov, Z. Zhu, J. Chu, S. S. Jaswal and E. Y. Tsymbal, Phys. Rev. Lett., 2008, 101, 137201 CrossRef PubMed.
- G. Radaelli, D. Petti, E. Plekhanov, I. Fina, P. Torelli, B. R. Salles, M. Cantoni, C. Rinaldi, D. Gutiérrez, G. Panaccione, M. Varela, S. Picozzi, J. Fontcuberta and R. Bertacco, Nat. Commun., 2014, 5, 3404 CrossRef CAS PubMed.
- J. T. Heron, M. Trassin, K. Ashraf, M. Gajek, Q. He, S. Y. Yang, D. E. Nikonov, Y.-H. Chu, S. Salahuddin and R. Ramesh, Phys. Rev. Lett., 2011, 107, 217202 CrossRef CAS PubMed.
- J. T. Heron, J. L. Bosse, Q. He, Y. Gao, M. Trassin, L. Ye, J. D. Clarkson, C. Wang, J. Liu, S. Salahuddin, D. C. Ralph, D. G. Schlom, J. Íñiguez, B. D. Huey and R. Ramesh, Nature, 2014, 516, 370–373 CrossRef CAS PubMed.
- J. M. Hu, T. Yang, J. Wang, H. Huang, J. Zhang, L. Q. Chen and C.-W. Nan, Nano Lett., 2015, 15, 616–622 CrossRef CAS PubMed.
- D. B. Gopman, P. Chen, J. W. Lau, A. C. Chavez, G. P. Carman, P. Finkel, M. Staruch and R. D. Shull, ACS Appl. Mater. Interfaces, 2018, 10, 24725–24732 CrossRef CAS PubMed.
- S. Kokado, N. Fujima, K. Harigaya, H. Shimizu and A. Sakuma, Phys. Rev. B: Condens. Matter Mater. Phys., 2006, 73, 172410 CrossRef.
- W. B. Mi, Z. B. Guo, X. P. Feng and H. L. Bai, Acta Mater., 2013, 61, 6387–6395 CrossRef CAS.
- Y. Komasaki, M. Tsunoda, S. Isogami and M. Takahashi, J. Appl. Phys., 2009, 105, 07C928 CrossRef.
- Z. Lai, C. Li, Z. Li, X. Liu, Z. Zhou, W. Mi and M. Liu, J. Mater. Chem. C, 2019, 7, 8537–8545 RSC.
- Z. R. Li, X. P. Feng, X. C. Wang and W. B. Mi, Mater. Res. Bull., 2015, 65, 175–182 CrossRef CAS.
- Z. Lai, Z. Li, X. Liu, L. Bai, Y. Tian and W. Mi, J. Phys. D: Appl. Phys., 2018, 51, 245001 CrossRef.
- Z. Lai, P. Li and W. Mi, J. Appl. Phys., 2019, 126, 113901 CrossRef.
- Z. R. Li, W. B. Mi and H. L. Bai, J. Phys. D: Appl. Phys., 2019, 52, 335001 CrossRef CAS.
- B. Yang, L. Tao, L. Jiang, W. Chen, P. Tang, Y. Yan and X. Han, Phys. Rev. Appl., 2018, 9, 054019 CrossRef.
- Z. Li, X. Wang, H. Dai, W. Mi and H. Bai, Thin Solid Films, 2015, 588, 26–33 CrossRef CAS.
- X. Han, W. Mi and D. Wang, J. Mater. Chem. C, 2020, 8, 3137–3146 RSC.
- L. Yin, W. Mi and X. Wang, Phys. Rev. Appl., 2016, 6, 064022 CrossRef.
- L. Yin, X. Wang and W. Mi, ACS Appl. Mater. Interfaces, 2017, 9, 15887–15892 CrossRef CAS PubMed.
- L. Yin, X. Wang and W. Mi, Appl. Phys. Lett., 2017, 111, 032404 CrossRef.
- Z. R. Li, W. B. Mi and H. L. Bai, Appl. Phys. Lett., 2018, 113, 132401 CrossRef.
- Z. R. Li, W. B. Mi and H. L. Bai, Comput. Mater. Sci., 2018, 142, 145–152 CrossRef CAS.
- Z. R. Li, W. B. Mi and H. L. Bai, ACS Appl. Mater. Interfaces, 2018, 10, 16674–16680 CrossRef CAS PubMed.
- L. Yu, G. Gao, G. Ding, Y. Duan, Y. Liu, Y. He and K. Yao, RSC Adv., 2016, 6, 29504–29511 RSC.
- G. Kresse and J. Furthmüller, Phys. Rev. B: Condens. Matter Mater. Phys., 1996, 54, 11169 CrossRef CAS PubMed.
- G. Kresse and J. Hafner, Phys. Rev. B: Condens. Matter Mater. Phys., 1993, 48, 13115 CrossRef CAS PubMed.
- G. Kresse and J. Furthmüller, Comput. Mater. Sci., 1996, 6, 15–50 CrossRef CAS.
- J. Perdew, K. Burke and M. Ernzerhof, Phys. Rev. Lett., 1996, 77, 3865 CrossRef CAS PubMed.
- P. Ghosez and J. Junquera, Annu. Rev. Condens. Matter Phys., 2022, 13, 325–364 CrossRef.
- N. Feng, W. Mi, X. Wang and H. Bai, RSC Adv., 2014, 4, 48848–48859 RSC.
- D. Odkhuu, Phys. Rev. B, 2017, 96, 134402 CrossRef.
- G. H. O. Daalderop, P. J. Kelly and M. F. H. Schuurmans, Phys. Rev. B: Condens. Matter Mater. Phys., 1990, 41, 11919 CrossRef CAS PubMed.
- D. Wang, R. Wu and A. J. Freeman, Phys. Rev. B: Condens. Matter Mater. Phys., 1993, 47, 14932 CrossRef CAS PubMed.
- B. S. Yang, J. Zhang, L. N. Jiang, W. Z. Chen, P. Tang, X. G. Zhang, Y. Yan and X. F. Han, Phys. Rev. B, 2017, 95, 174424 CrossRef.
- A. Houari, S. F. Matar and M. A. Belkhir, J. Magn. Magn. Mater., 2010, 322, 658–660 CrossRef CAS.
- W. Wang, W. Sun, G. Zhang, F. Ren, Y. Wang, C. You and Z. Cheng, J. Adv. Res., 2020, 24, 371–377 CrossRef CAS PubMed.
- M. Zhao, C. Jin, W. Sun, W. Zhai, F. Ren and B. Wang, Results Phys., 2022, 37, 105538 CrossRef.
- C. G. Duan, S. S. Jaswal and E. Y. Tsymbal, Phys. Rev. Lett., 2006, 97, 047201 CrossRef PubMed.
|
This journal is © The Royal Society of Chemistry 2023 |
Click here to see how this site uses Cookies. View our privacy policy here.