DOI:
10.1039/D3RA01222K
(Paper)
RSC Adv., 2023,
13, 8220-8226
Construction of defected MOF-74 with preserved crystallinity for efficient catalytic cyanosilylation of benzaldehyde†
Received
23rd February 2023
, Accepted 3rd March 2023
First published on 13th March 2023
Abstract
Numerous open metal sites and well-developed micropores are the two most significant characteristics that should be imparted to design metal–organic frameworks (MOFs) as effective catalysts. However, the construction of the best MOF catalyst with both these characteristics is challenging because the creation of numerous open metal sites generally triggers some structural collapse of the MOF. Herein, we report the construction of well-structured but defected MOFs through the growth of defected MOFs, where some of the original organic linkers were replaced with analog organic linkers, on the surface of a crystalline MOF template (MOF-on-MOF growth). Additional open metal sites within the MOF-74 structure were generated by replacing some of the 2,5-dihydroxy-1,4-bezenedicarboxylic acid presenting in MOF-74 with 1,4-benzenedicarboxylic acid due to the missing hydroxyl groups. And the resulting additional open metal sites within the MOF-74 structure resulted in enhanced catalytic activity for the cyanosilylation of aldehydes. However, the collapse of some of the well-developed MOF-74 structure was also followed by structural defects. Whereas, the growth of defected MOF-74 (D-MOF-74) on the well-crystallized MOF-74 template led to the production of relatively well-crystallized D-MOF-74. Core–shell type MOF-74@D-MOF-74 having abundant open metal sites with a preserved crystallinity exhibited the efficient catalytic cyanosilylation of several aldehydes. Additionally, MOF-74@D-MOF-74 displayed excellent recyclability during the consecutive catalytic cycles.
Introduction
Metal–organic frameworks (MOFs) are an important class of porous crystalline materials used in various practical applications, including gas storage, recognition, sensing, separation, and catalysis.1–6 In particular, the use of MOFs in catalysis has received considerable attention and the design of MOFs to yield excellent activity is critical for their practical applications.7–17 Well-defined channels or pores in MOFs are beneficial for their excellent catalytic activity, and unsaturated Lewis acid metal sites (open metal sites) are known to be active sites for the catalytic reactions.8–17 There are several approaches to generate additional open metal sites within an MOF structure for improved catalytic activity.11–17 Removing some of the existing organic linkers in an original MOF structure can generate extra open metal sites, just as replacing some of them with analog linkers having fewer coordination sites can also generate extra open metal sites. For example, Eder et al. reported the generation of defected MIL-125 with open metal sites through removing some of the 2-amino-1,4-benzenedicarboxylic acid within an MIL-125 structure,13 and Li et al. reported the production of defected CoBDC-Fc by replacing some of the 1,4-benzenedicarboxylic acid (BDC) linker by ferrocenecarboxylic acid.11 However, in most cases of producing defected MOFs with extra open metal sites, removing or replacing the original organic linkers has resulted in structural defects and instability.16–20 In such cases, the MOFs consequently lose some of their crystallinity, resulting in lowered catalytic activity as excessive defects and poor crystallinity in MOFs interrupt smooth chemical transportation during catalytic reactions.15–17 Therefore, the construction of defected MOFs having many open metal sites without losing their crystallinity is most desirable.
Herein, we report the construction of defected MOFs having extra open metal sites with preserved crystallinity through the growth of defected MOFs on the crystalline MOF template (MOF-on-MOF growth). Replacing some of the 2,5-dihydroxy-1,4-bezenedicarboxylic acid (DHBDC) within the MOF-74 structure with BDC resulted in defected MOF-74 (D-MOF-74) having additional open metal sites owing to the missing hydroxyl groups. The resulting extra open metal sites within defected MOFs caused the enhancement of catalytic activity for the cyanosilylation of benzaldehyde. However, structural defects also caused the collapse of the well-organized MOF-74 structure, negatively impacting the catalytic reaction. Whereas the directed growth of D-MOF-74 on the surface of the well-crystallized MOF-74 template led to the formation of relatively well-crystallized D-MOF-74 shell. Eventually, core–shell type MOF-74@D-MOF-74 with abundant open metal sites and a preserved well-developed porosity displayed the best performance for the cyanosilylation of several aldehydes. Moreover, they exhibited excellent recyclability during seven successive catalytic cycles.
Experimental
Materials and characterizations
All solvents and chemicals were purchased from commercial sources and used as received, unless otherwise stated. SEM images were captured using a JEOL JSM-7001F field-emission SEM (Yonsei Center for Research Facilities, Yonsei University). EDX spectra were acquired using a Hitachi SU 1510 SEM equipped with a Horiba EMAX Energy E-250 EDX system. PXRD patterns were obtained using a Rigaku Ultima IV equipped with a graphite monochromated Cu Kα radiation source (40 kV, 40 mA). The adsorption–desorption isotherms of N2 (77 K) were measured using a BELSORP Max volumetric adsorption instrument. All isotherms of samples were measured after soaking in methanol for 10 h and a pretreatment under a dynamic vacuum at 120 °C for 12 h. 1H NMR spectra were recorded on a Bruker Avance III HD 300 spectrometer (1H NMR, 300 MHz) with chemical shifts reported relative to residual deuterated solvent peaks. TGA curves were acquired using a Shimadzu TGA-50 system under a nitrogen atmosphere at a heating rate of 5 °C min−1. Inductively coupled plasma-mass spectrometer analysis was performed using a PerkinElmer NexION 350D instrument to quantify cobalt in the supernatant after catalytic reaction.
Preparation of MOF-74
CoCl2·6H2O (0.30 mmol, 71.4 mg), DHBDC (0.075 mmol, 14.9 mg), and polyvinylpyrrolidone (PVP; 250 mg) were dissolved in 5 mL N,N-dimethylformamide (DMF). The resulting mixture was heated in a 120 °C oil bath for 2 h, then isolated via centrifugation, washed with fresh DMF and methanol, and dried in vacuum for 1 h. Large scale production of MOF-74 was also conducted using CoCl2·6H2O (2.40 mmol, 571.2 mg), DHBDC (0.6 mmol, 119.2 mg), PVP (2 g), and 40 mL DMF.
Preparation of D-MOF-74
CoCl2·6H2O (0.30 mmol, 71.4 mg), DHBDC (0.01, 0.015, 0.024, or 0.028 mmol), BDC (0.040, 0.060, 0.096, or 0.112 mmol), and PVP (250 mg) were dissolved in 5 mL of DMF. The resulting mixture was placed in a 120 °C oil bath for 2 h, then isolated via centrifugation, washed with fresh DMF and methanol, and dried in vacuum for 1 h. The products obtained from four varied concentrations of reactants are denoted as D1-, D2-, D3-, and D4-MOF-74, respectively. Large scale production of D3-MOF-74 was also conducted using CoCl2·6H2O (2.40 mmol, 571.2 mg), DHBDC (0.192 mmol, 38.4 mg), BDC (0.768 mmol, 127.2 mg), PVP (2 g), and 40 mL DMF.
Preparation of MOF-74@D-MOF-74
CoCl2·6H2O (0.30 mmol, 71.4 mg), DHBDC (0.01, 0.015, 0.024, or 0.028 mmol), BDC (0.040, 0.060, 0.096, or 0.112 mmol), and PVP (250 mg) were dissolved in 5 mL of DMF. 4.0 mg of MOF-74 was added to the resulting mixture, and the resulting suspension was placed in a 120 °C oil bath for 2 h, then isolated via centrifugation, washed with fresh DMF and methanol, and dried in vacuum for 1 h. Large scale production of MOF-74@D3-MOF-74 was also conducted using CoCl2·6H2O (2.40 mmol, 571.2 mg), DHBDC (0.192 mmol, 38.4 mg), BDC (0.768 mmol, 127.2 mg), PVP (2 g), MOF-74 (32.0 mg), and 40 mL DMF.
Cyanosilylation of benzaldehyde
Benzaldehyde (1 mmol), trimethylsilyl cyanide (TMSCN; 3 mmol), and MOF catalyst (MOF-74, D-MOF-74, or MOF-74@D-MOF-74; 3.0 mg) were mixed in a 4 mL vial. The resulting mixture was reacted at 60 °C for 30 min with constant stirring. After 30 min, the catalyst was isolated via centrifugation, and 1H NMR spectra were measured to determine the conversion of benzaldehyde. Similar cyanosilylation reactions were also conducted using 4-methoxybenzaldehyde, 4-methylbenzaldehyde, and 4-fluorobenzaldehyde instead of benzaldehyde. For the recycling test, seven cycles of benzaldehyde cyanosilylation were repeated with the MOF-74@D2-MOF-74 (or D3-MOF-74) catalyst. After the first catalytic reaction, the catalyst was isolated via centrifugation, washed with methanol several times, and dried under vacuum for 1 h. Subsequently, fresh benzaldehyde and TMSCN were added into the vial containing the refreshed catalyst for a second catalytic cycle. The same procedure was repeated for a seventh catalytic cycle.
Results and discussion
Firstly, a three-dimensional (3D) hexagonal structured MOF (MOF-74) with a chemical composition of [Co2(DHBDC)(H2O)2]n was synthesized from the solvothermal reaction of CoCl2 and DHBDC (Scheme 1 and Fig. S1, ESI†).21 Scanning electron microscopy (SEM) image (Fig. 1a) of the resulting product revealed the uniform production of hexagonal rods with a high aspect ratio, and powder X-ray diffraction (PXRD) patterns (Fig. 1d) of the product, which matched the representative PXRD pattern of MOF-74, confirmed the production of well-crystallized MOF-74. Next, defected MOF-74 (denoted as D-MOF-74; [Co2(DHBDC)1−x(BDC)x(H2O)2Yz]n) was prepared via similar solvothermal reactions of CoCl2, but in the presence of two organic linkers, DHBDC and BDC (the products obtained from four varied concentrations of reactants are denoted as D1-, D2-, D3-, and D4-MOF-74, respectively. See experimental section for details). The incorporation of BDC instead of some of the original DHBDC within an MOF-74 structure should invoke structural defects owing to the missing hydroxyl moieties within the structure (Scheme 1 and Fig. S2, ESI†). Typically, hydroxyl groups and carboxyl groups of DHBDC are coordinated to metal ions to form the CoO6 octahedra of an MOF-74 structure (Fig. S1, ESI†);22 thus, the missing hydroxyl moieties should result in additional open metal sites (Fig. S2, ESI†) within the MOF-74 structure that should be beneficial for its catalytic activity. The additional open metal sites provoked from the missing hydroxyl moieties are located toward the hexagonal channels of the MOF-74 structure, as shown in Fig. S3 (ESI†). However, the replacement of some of DHBDC with BDC also causes structural instability owing to the missing hydroxyl moieties, which originally participate in building the MOF-74 skeleton.
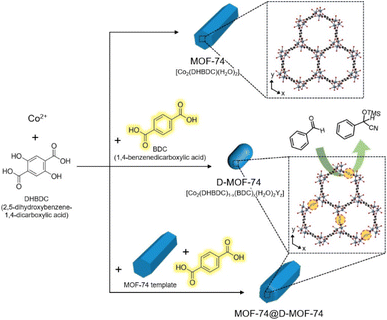 |
| Scheme 1 Schematic of the synthesis of MOF-74, D-MOF-74, and core–shell type MOF-74@D-MOF-74. | |
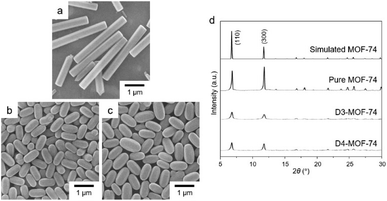 |
| Fig. 1 SEM images of (a) pure MOF-74, (b) D3-MOF-74, and (c) D4-MOF-74. (d) PXRD patterns of pure MOF-74, D3-MOF-74, and D4-MOF-74. The simulated PXRD pattern of MOF-74 is also shown. | |
The morphologies of the resulting D-MOF-74 were validated through SEM images of the samples. As shown in Fig. 1, the production of rounded ovals was confirmed from the reactions of CoCl2 with the two mixed organic linkers. PXRD patterns of D-MOF-74 samples verified that they principally had a 3D hexagonal MOF-74 structure (Fig. 1d). However, the peaks broadened and decreased,17,18,23 possibly due to the gradual loss of its crystallinity as BDC is included. In general, rounded MOF particles represent amorphous characteristic of the MOF.17,24,25 Therefore, the rounded shape of D-MOF-74 samples supported the amorphous nature of defected MOF-74. Energy dispersive X-ray (EDX) spectra of the samples displayed the detection of carbon, oxygen, and cobalt elements, confirming the existence of cobalt ions and organic linkers (Fig. S4, ESI†). In addition, extra anions must be existed in D-MOF-74 for the charge balance due to the incorporation of BDC instead of some of DHBDC. Indeed, EDX spectra of D-MOF-74 samples exhibited the presence of Cl element (Fig. S4, ESI†). Moreover, the incorporated amounts of DHBDC and BDC within D-MOF-74 were analyzed by 1H NMR spectroscopy. D-MOF-74 samples were added to a mixed deuterated solvent of DCl and DMSO-d6 to digest solid D-MOF-74 samples and measure 1H NMR spectra. The incorporated amounts of BDC within D3-MOF-74 and D4-MOF-74 were found to be 4.6 and 3.5%, respectively (Fig. S5, ESI†). PXRD patterns and 1H NMR spectra of D1-MOF-74 and D2-MOF-74 could not be measured because only trace amounts of D1-MOF-74 and D2-MOF-74 were produced during the reactions at relatively dilute concentrations.
The amounts of D-MOF-74 generated from the reactions were small (calculated yields for D3-MOF-74 and D4-MOF-74 were 21 and 83%, respectively, and the yields for D1-MOF-74 and D2-MOF-74 were less than 3%). In addition, the gradual loss of its crystallinity with the incorporation of BDC was resulted as mentioned before. For a more efficient production of D-MOF-74 with preserving its crystallinity, the similar reactions of CoCl2 with mixed two organic linkers were conducted in the presence of the MOF-74 template to generate D-MOF-74 in the form of core–shell MOF-74@D-MOF-74. The growth of second MOF on the surface of template MOF (MOF-on-MOF growth) has been well established for the conjugation of two different MOFs or the production of unique MOFs.26–35 Especially, a well-crystallized template MOF can induce the growth of a well-crystallized second MOF.31–35 The presence of well-crystallized MOF-74 should induce not only the effective growth of D-MOF-74 but also the production of D-MOF-74 with a preserved crystallinity by providing well-ordered lattices to the reactants.31–35 First, the yields of the reactions dramatically increased in the presence of the MOF-74 template. The amounts of D-MOF-74 produced were calculated based upon the initial weight of the MOF-74 template and the final weight of MOF-74@D-MOF-74. Even though no meaningful formation (less than 3%) of D1-MOF-74 and D2-MOF-74 was observed without the template, considerable amounts of D1-MOF-74 (53%) and D2-MOF-74 (60%) were produced on the surface of the MOF-74 template. In addition, the yields of D3-MOF-74 and D4-MOF-74 were 82 and 78%, respectively. SEM images (Fig. 2) of the resulting products revealed that the hexagonal MOF-74 shape was well maintained even after a MOF-on-MOF growth process for the production of core–shell MOF-74@D-MOF-74. In addition, progressively increased widths of hexagonal rods were obviously detected in SEM images of a series of MOF-74@D-MOF-74 samples (Fig. 2). PXRD patterns of a series of MOF-74@D-MOF-74 samples displayed the representative peaks for MOF-74 (Fig. 2f). In addition, there was no significant peak broadening after D-MOF-74 formation on the surface of MOF-74 template, indicating no critical loss of its crystallinity in MOF-74@D-MOF-74. The incorporated amounts of BDC within the D-MOF-74 shell were calculated based on their 1H NMR spectra and the relative amounts of the MOF-74 core and the D-MOF-74 shell within MOF-74@D-MOF-74. The calculations indicated that the incorporated amounts of BDC within the D-MOF-74 shell of MOF-74@D-MOF-74 were 20.8, 5.9, 3.0, and 2.2%, respectively (Fig. S6, ESI†). Meanwhile, large scale productions of D-MOF-74 and MOF-74@D-MOF-74 were also successful using large amounts of reactants (Fig. S7, ESI†).
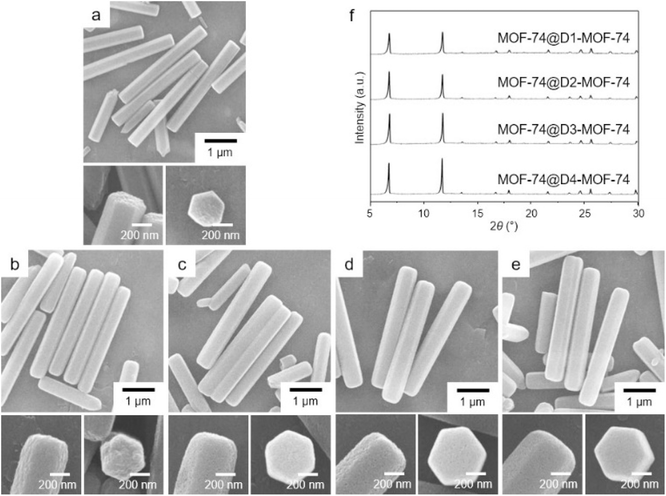 |
| Fig. 2 SEM images of (a) MOF-74 template, (b) MOF-74@D1-MOF-74, (c) MOF-74@D2-MOF-74, (d) MOF-74@D3-MOF-74, and (e) MOF-74@D4-MOF-74. (f) PXRD patterns of a series of MOF-74@D-MOF-74. | |
The porosities of the D-MOF-74 and MOF-74@D-MOF-74 samples were then analyzed from their N2 sorption isotherms (Fig. 3). Brunauer–Emmett–Teller (BET) surface areas and total pore volumes of the D-MOF-74 samples prepared in the absence of template decreased compared to those of pure MOF-74. The BET surface area and total pore volume of pure MOF-74 were 1180.8 m2 g−1 and 0.50 cm3 g−1, respectively, whereas the BET surface areas of D3-MOF-74 and D4-MOF-74 were 925.7 and 919.3 m2 g−1 and their total pore volumes were 0.43 and 0.40 cm3 g−1, respectively. These decreasing values of D-MOF-74 are originated from some structural collapse and a gradual loss of its crystallinity as the DHBDC linker is replaced with the BDC linker. However, no significant decreases in N2 sorption, BET surface area, and total pore volume were observed in the MOF-74@D-MOF-74 samples (Fig. 3 and Table S1, ESI†). For examples the BET surface areas of MOF-74@D3-MOF-74 and MOF-74@D4-MOF-74 were 1158.9 and 1172.3 m2 g−1 and their total pore volumes were 0.50 and 0.50 cm3 g−1, respectively. In addition, the relative values of the D-MOF-74 shells within a series of MOF-74@D-MOF-74 were estimated based on the relative amounts of the MOF-74 core and the D-MOF-74 shell. The estimated BET surface areas of D3-MOF-74 and D4-MOF-74 within MOF-74@D-MOF-74 samples were 1146.4 and 1167.9 m2 g−1 and their total pore volumes estimated were 0.50 and 0.50 cm3 g−1, respectively (Table S2, ESI†). These values are higher than those of the corresponding D-MOF-74 generated without the template. In addition, no critical difference was found in the pore size distributions of pure MOF-74 and MOF-74@D-MOF-74 calculated using non-local density functional theory (Fig. S8, ESI†). No difference in thermal stability of pure MOF-74 and MOF-74@D-MOF-74 was also detected in the thermogravimetric analysis curves (Fig. S9, ESI†).
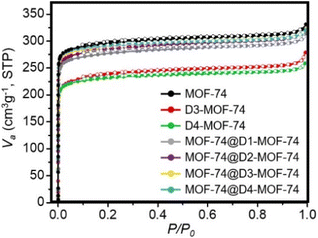 |
| Fig. 3 N2 sorption isotherms of MOF-74, a series of D-MOF-74, and a series of MOF-74@D-MOF-74. | |
The improvement of the catalytic activity of the D-MOF-74 samples through providing additional open metal sites, which can function as Lewis acid catalytic sites, was verified from their enhanced activity on the cyanosilyation of benzaldehyde. Cyanosilylation of aldehydes is significant because it is a critical method to synthesize cyanohydrins, which are key compounds for the synthesis of several fine chemicals, including pharmaceuticals.36–38 Benzaldehyde and trimethylsilyl cyanide (TMSCN) were reacted in the presence of a catalytic amount of pure MOF-74 or a series of D-MOF-74 under solvent-free conditions at 60 °C for 30 min (Table 1). After the reactions, 1H NMR spectroscopy was used to determine the conversion of benzaldehyde to 2-phenyl-2-[(trimethylsilyl)oxy] acetonitrile in the absence or presence of an internal standard (Tables 1, S3, and Fig. S10, ESI†). The conversion of benzaldehyde in the presence of D-MOF-74 was much higher than that in the presence of pure MOF-74. Pure MOF-74 displayed 65% conversion of the initial benzaldehyde for 30 min; however, 93 and 92% conversions were observed in the presence of D3-MOF-74 and D4-MOF-74, respectively. The catalytic activity of MOF-74 was enhanced up to 28% by replacing only 4.6% of DHBDC with BDC. MOF-74@D3-MOF-74 and MOF-74@D4-MOF-74 also exhibited enhanced conversion efficiency at 93 and 91%, respectively. However, when the actual amount of D-MOF-74 within MOF-74@D-MOF-74 is considered, the catalytic activity of D-MOF-74 in MOF-74@D-MOF-74 is more dramatically enhanced compared to that of D-MOF-74 generated without the template. In fact, only 1.9% of the BDC was incorporated within MOF-74@D3-MOF-74. MOF-74@D2-MOF-74, where 2.7% of the BDC was incorporated, displayed a best catalytic activity with 96% conversion. Conserving its crystallinity during the construction of D-MOF-74 due to the efficient guide of well-crystallized MOF-74 is a reason for the improvement of catalytic activity of D-MOF-74 within MOF-74@D-MOF-74. The plots for the conversions of benzaldehyde to 2-phenyl-2-[(trimethylsilyl)oxy] acetonitrile by pure MOF-74 and MOF-74@D2-MOF-74 in varied time points were also obtained as shown in Fig. S11a (ESI†). Moreover, a linear relationship between ln(Ct/C0) and the reaction time was observed, which revealed first-order reaction kinetics (Fig. S11b, ESI†).39,40
Table 1 Cyanosilylation of benzaldehyde with TMSCN catalyzed by pure MOF-74, a series of D-MOF-74, and a series of MOF-74@D-MOF-74a

|
Entry |
Catalyst |
Conversion (%) |
Reaction conditions: benzaldehyde (1 mmol), MOF catalyst (3.0 mg, 1.7 mol% based on Co(II)), trimethylsilyl cyanide (TMSCN; 3 mmol). |
1 |
MOF-74 |
65 |
2 |
D3-MOF-74 |
93 |
3 |
D4-MOF-74 |
92 |
4 |
MOF-74@D1-MOF-74 |
89 |
5 |
MOF-74@D2-MOF-74 |
96 |
6 |
MOF-74@D3-MOF-74 |
93 |
7 |
MOF-74@D4-MOF-74 |
91 |
Meanwhile, cobalt ions can bleach out from the catalyst during the catalytic reaction; however, no critical bleaching of cobalt ions from the catalyst (MOF-74@D2-MOF-74) was found during the catalytic reaction. Inductively coupled plasma-mass spectrometer analysis revealed that ca. 0.8% of cobalt bleached out from the catalyst after the catalytic reaction. Control experiments for cyanosilylation of benzaldehyde were also conducted. Cyanosilylation of benzaldehyde without any catalyst was occurred but showed very low activity.41–43 And the salt (CoCl2) was also active for cyanosilylation of benzaldehyde under homogeneous condition, as already known.41–43 Moreover, cyanosilylation reactions of three other aldehydes, such as 4-methoxybenzaldehyde, 4-methylbenzaldehyde, and 4-fluorobenzaldehyde were performed in the presence of a catalytic amount of pure MOF-74 and MOF-74@D2-MOF-74 (Fig. S12–S14, ESI†). In general, the catalytic activity of MOF-74@D2-MOF-74 was higher than that of pure MOF-74 for the cyanosilylation of three aldehydes (Table 2). The cyanosilylation of aldehyde having electron donating groups is normally faster than that of aldehyde bearing electron withdrawing groups.44,45 The recyclability of the catalyst was examined using MOF-74@D2-MOF-74 and D3-MOF-74. The cyanosilyation activity was well maintained even after seven successive catalytic cycles (Fig. 4). Moreover, the SEM image and PXRD pattern of MOF-74@D2-MOF-74 after seven catalytic cycles verified no critical morphological change and no structural change even after seven successive catalytic reactions (Fig. 4). D3-MOF-74 also exhibited good recyclability as shown in its catalytic activity during seven successive catalytic reactions (Fig. S15, ESI†).
Table 2 Cyanosilylation of various aldehydes with TMSCN catalyzed by pure MOF-74 and MOF-74@D2-MOF-74a
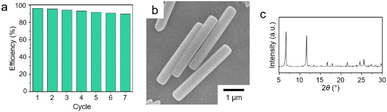 |
| Fig. 4 (a) Recyclability of MOF-74@D2-MOF-74 over seven successive catalytic cycles. (b) SEM image and (c) PXRD pattern of MOF-74@D2-MOF-74 after seven successive catalytic reactions. | |
Conclusions
In conclusion, two series of defected MOF-74 were successfully prepared by replacing a small amount of DHBDC linker originally present in MOF-74 with BDC linker. Owing to the missing hydroxyl groups in the BDC linker compared to the DHBDC linker, extra open metal sites were produced within the D-MOF-74 structure. Two series of D-MOF-74 were prepared from a series of reactions in the absence or presence of the MOF-74 template. All the resulting D-MOF-74 displayed an enhanced catalytic activity for the cyanosilyation of benzaldehyde compared to that of pure MOF-74. D-MOF-74 generated in the absence of the MOF-74 template was found to lose its crystallinity. On the other hand, the well-crystallized MOF-74 template invoked a more efficient growth of D-MOF-74 without losing its crystallinity. Finally, core–shell type MOF-74@D-MOF-74 with many open metal sites and preserved well-developed porosity exhibited the best performance as a catalyst. Moreover, excellent recyclability was demonstrated during several consecutive catalytic reactions. The synthetic approach suggested here for the production of defected MOFs having many open metal sites with preserved original porosity should be beneficial in the development of highly active MOFs applicable in catalysis, sensing, and adsorption.
Author contributions
Moonhyun Oh designed, coordinated the research, and drafted the manuscript. Chul Hwan Shim, Sojin Oh, Sujeong Lee, and Gihyun Lee conducted experiments and data analysis. Sojin Oh, Sujeong Lee, Gihyun Lee, and Moonhyun Oh revised the manuscript. The authors read and approved the final manuscript.
Conflicts of interest
There are no conflicts to declare.
Acknowledgements
This research was supported by the Challengeable Future Defense Technology Research and Development Program through the Agency for Defense Development (ADD) funded by the Defense Acquisition Program Administration (DAPA) in 2022 (No. 915019201).
Notes and references
- M. Bonneau, C. Lavenn, J. J. Zheng, A. Legrand, T. Ogawa, K. Sugimoto, F. X. Coudert, R. Reau, S. Sakaki, K. Otake and S. Kitagawa, Nat. Chem., 2022, 14, 816–822 CrossRef CAS PubMed
. - L. Li, R. B. Lin, R. Krishna, H. Li, S. Xiang, H. Wu, J. Li, W. Zhou and B. Chen, Science, 2018, 362, 443–446 CrossRef CAS PubMed
. - T. Y. Luo, P. Das, D. L. White, C. Liu, A. Star and N. L. Rosi, J. Am. Chem. Soc., 2020, 142, 2897–2904 CrossRef CAS PubMed
. - R. L. Siegelman, E. J. Kim and J. R. Long, Nat. Mater., 2021, 20, 1060–1072 CrossRef CAS PubMed
. - J. Liu, T. A. Goetjen, Q. Wang, J. G. Knapp, M. C. Wasson, Y. Yang, Z. H. Syed, M. Delferro, J. M. Notestein, O. K. Farha and J. T. Hupp, Chem. Soc. Rev., 2022, 51, 1045–1097 RSC
. - H. Yoon, S. Lee, S. Oh, H. Park, S. Choi and M. Oh, Small, 2019, 15, 1805232 CrossRef PubMed
. - F. Zhang, J. Zhang, B. Zhang, L. Zheng, X. Cheng, Q. Wan, B. Han and J. Zhang, Green Chem., 2020, 22, 5995–6000 RSC
. - Y. Wen, J. Zhang, Q. Xu, X. T. Wu and Q. L. Zhu, Coord. Chem. Rev., 2018, 376, 248–276 CrossRef CAS
. - Y. Shen, T. Pan, L. Wang, Z. Ren, W. Zhang and F. Huo, Adv. Mater., 2021, 33, 2007442 CrossRef CAS PubMed
. - S. Dissegna, K. Epp, W. R. Heinz, G. Kieslich and R. A. Fischer, Adv. Mater., 2018, 30, 1704501 CrossRef PubMed
. - Z. Xue, K. Liu, Q. Liu, Y. Li, M. Li, C. Y. Su, N. Ogiwara, H. Kobayashi, H. Kitagawa, M. Liu and G. Li, Nat. Commun., 2019, 10, 5048 CrossRef PubMed
. - G. Cai and H. L. Jiang, Angew. Chem., Int. Ed., 2017, 56, 563–567 CrossRef CAS PubMed
. - S. Naghdi, A. Cherevan, A. Giesriegl, R. Guillet-Nicolas, S. Biswas, T. Gupta, J. Wang, T. Haunold, B. C. Bayer, G. Rupprechter, M. C. Toroker, F. Kleitz and D. Eder, Nat. Commun., 2022, 13, 282 CrossRef CAS PubMed
. - H. Jiang, Q. Wang, H. Wang, Y. Chen and M. Zhang, ACS Appl. Mater. Interfaces, 2016, 8, 26817–26826 CrossRef CAS PubMed
. - Z. Fan, J. Wang, W. Wang, S. Burger, Z. Wang, Y. Wang, C. Wöll, M. Cokoja and R. A. Fischer, ACS Appl. Mater. Interfaces, 2020, 12, 37993–38002 CrossRef CAS PubMed
. - Z. Jiang, L. Ge, L. Zhuang, M. Li, Z. Wang and Z. Zhu, ACS Appl. Mater. Interfaces, 2019, 11, 44300–44307 CrossRef CAS PubMed
. - H. Jun, S. Oh, G. Lee and M. Oh, Sci. Rep., 2022, 12, 14735 CrossRef CAS PubMed
. - O. Kozachuk, I. Luz, F. X. Llabrés i Xamena, H. Noei, M. Kauer, H. B. Albada, E. D. Bloch, B. Marler, Y. Wang, M. Muhler and R. A. Fischer, Angew. Chem., Int. Ed., 2014, 53, 7058–7062 CrossRef CAS PubMed
. - A. F. Möslein, L. Donà, B. Civalleri and J. C. Tan, ACS Appl. Nano Mater., 2022, 5, 6398–6409 CrossRef PubMed
. - A. W. Thornton, R. Babarao, A. Jain, F. Trousselet and F. X. Coudert, Dalton Trans., 2016, 45, 4352–4359 RSC
. - C. Young, J. Kim, Y. V. Kaneti and Y. Yamauchi, ACS Appl. Energy Mater., 2018, 1, 2007–2015 CrossRef CAS
. - P. D. C. Dietzel, Y. Morita, R. Blom and H. Fjellvåg, Angew. Chem., Int. Ed., 2005, 44, 6354–6358 CrossRef CAS PubMed
. - T. D. Bennett and A. K. Cheetham, Acc. Chem. Res., 2014, 47, 1555–1562 CrossRef CAS PubMed
. - Z. Gao, B. Xu, Y. Fan, T. Zhang, S. Chen, S. Yang, W. Zhang, X. Sun, Y. Wei, Z. Wang, X. Wang, X. Meng and Y. S. Zhao, Angew. Chem., Int. Ed., 2021, 60, 6362–6366 CrossRef CAS PubMed
. - H. J. Lee, J. We, J. O. Kim, D. Kim, W. Cha, E. Lee, J. Sohn and M. Oh, Angew. Chem., Int. Ed., 2015, 54, 10564–10568 CrossRef CAS PubMed
. - L. Chai, J. Pan, Y. Hu, J. Qian and M. Hong, Small, 2021, 17, 2100607 CrossRef CAS PubMed
. - M. Abdollahzadeh, M. Chai, E. Hosseini, M. Zakertabrizi, M. Mohammad, H. Ahmadi, J. Hou, S. Lim, A. H. Korayem, V. Chen, M. Asadnia and A. Razmjou, Adv. Mater., 2022, 34, 2107878 CrossRef CAS PubMed
. - S. Lee, S. Oh and M. Oh, Angew. Chem., Int. Ed., 2020, 59, 1327–1333 CrossRef CAS PubMed
. - S. Choi, T. Kim, H. Ji, H. J. Lee and M. Oh, J. Am. Chem. Soc., 2016, 138, 14434–14440 CrossRef CAS PubMed
. - Y. Wang, Z. Zhang, J. Li, Y. Yuan, J. Yang, W. Xu, P. An, S. Xi, J. Guo, B. Liu and J. Li, Angew. Chem., Int. Ed., 2022, 61, e202211031 CAS
. - H. Ji, S. Lee, J. Park, T. Kim, S. Choi and M. Oh, Inorg. Chem., 2018, 57, 9048–9054 CrossRef CAS PubMed
. - S. Lee, G. Lee and M. Oh, ACS Nano, 2021, 15, 17907–17916 CrossRef CAS PubMed
. - G. Lee, S. Lee, S. Oh, D. Kim and M. Oh, J. Am. Chem. Soc., 2020, 142, 3042–3049 CrossRef CAS PubMed
. - M. Zhao, J. Chen, B. Chen, X. Zhang, Z. Shi, Z. Liu, Q. Ma, Y. Peng, C. Tan, X. J. Wu and H. Zhang, J. Am. Chem. Soc., 2020, 142, 8953–8961 CrossRef CAS PubMed
. - C. Liu, Q. Sun, L. Lin, J. Wang, C. Zhang, C. Xia, T. Bao, J. Wan, R. Huang, J. Zou and C. Yu, Nat. Commun., 2020, 11, 4971 CrossRef CAS PubMed
. - N. Kurono, K. Arai, M. Uemura and T. Ohkuma, Angew. Chem., Int. Ed., 2008, 47, 6643–6646 CrossRef CAS PubMed
. - W. B. Wu, J. S. Yu and J. Zhou, ACS Catal., 2020, 10, 7668–7690 CrossRef CAS
. - X. P. Zeng, J. C. Sun, C. Liu, C. B. Ji and Y. Y. Peng, Adv. Synth. Catal., 2019, 361, 3281–3305 CrossRef CAS
. - M. North, M. Omedes-Pujol and C. Young, Biomol. Chem., 2012, 10, 4289–4298 RSC
. - Z. Zhang, J. Chen, Z. Bao, G. Chang, H. Xing and Q. Ren, RSC Adv., 2015, 5, 79355–79360 RSC
. - H. An, J. Zhang, S. Chang, Y. Hou and Q. Zhu, Inorg. Chem., 2020, 59, 10578–10590 CrossRef CAS PubMed
. - H. F. Yao, Y. Yang, H. Liu, F. G. Xi and E. Q. Gao, J. Mol. Catal. A: Chem., 2014, 394, 57–65 CrossRef CAS
. - Y. Jiang, J. Xu, Z. Zhu, C. Jiang, L. Ma, H. Wang, L. Wang and Y. Fan, Inorg. Chim. Acta, 2020, 510, 119735 CrossRef CAS
. - D. Kumar, A. P. Prakasham, M. K. Gangwar and P. Ghosh, Inorg. Chim. Acta, 2019, 495, 119003 CrossRef CAS
. - L. M. Aguirre-Díaz, M. Iglesias, N. Snejko, E. Gutiérrez-Puebla and M. Á. Monge, CrystEngComm, 2013, 15, 9562–9571 RSC
.
|
This journal is © The Royal Society of Chemistry 2023 |