DOI:
10.1039/D3RA00199G
(Paper)
RSC Adv., 2023,
13, 9099-9108
Magnetocaloric effect and Griffiths phase analysis in a nanocrystalline Ho2NiMnO6 and Ho2CoMnO6 double perovskite
Received
10th January 2023
, Accepted 11th March 2023
First published on 20th March 2023
Abstract
Rare-earth double perovskite oxides have intriguing magnetocaloric properties at cryogenic temperatures. In this study, Ho2NiMnO6 and Ho2CoMnO6 were synthesized using the sol–gel method, which crystallized in a monoclinic structure in the P21/n space group. The magnetic phase transition was observed at 81.2 K for Ho2NiMnO6 and 73.5 K for Ho2CoMnO6. The presence of a paramagnetic matrix and short-range ferromagnetic clusters causes magnetic disorder in these double perovskites, resulting in Griffiths phase formation. The Arrott plot confirms that compounds undergo second-order phase transition. At an applied magnetic field of 5 T, the maximum magnetic entropy change (−ΔS) for the studied compounds is 1.7 and 2.2 J kg−1 K−1, respectively. The transition metals Ni and Co in a double perovskite cause lattice distortion in the structural parameters and oxidation states of manganese (Mn3+/Mn4+), which changes the magnetic and magnetocaloric properties. The quantitative approach provides a systematic study of magnetocaloric properties of the rare earth double perovskite compounds with ferromagnetic 3d transition elements.
1. Introduction
Magnetic refrigeration is a cooling technology based on the magnetocaloric effect (MCE), which has recently attracted much attention because of its high conversion efficiency and environmental friendliness.1–4 The MCE is defined as the magneto-thermal response of a magnetic material arising due to the coupling of the magnetic sublattice with the external magnetic field. The temperature of MCE materials increases or decreases when we apply or remove the magnetic field.5 So far, numerous magnetocaloric materials have been explored for possible applications in magnetic refrigeration (MR) at a wide range of temperatures from ambient to liquid He temperature. The various materials with a substantial MCE at low temperatures, such as rare-earth based alloys,6–8 rare-earth amorphous alloys,9,10 rare earth oxides,11,12 and double perovskites13–18 have been extensively investigated over the years. Among them, magnetic oxides are a class of materials that are being explored for their potential applications in magnetic refrigeration technology, due to their high resistivity and low eddy current loss. They are also attractive for fundamental studies, as they are simple to prepare and chemically stable.19,20 However, in the literature it was reported that the adiabatic temperature change of magnetic oxides is limited by their relatively high heat capacity.21
On the other hand, the rare-earth based double perovskite (DP) compounds having the general formula A2B′B′′O6 (A = rare earth metal, B′ = di-valent transition metal, B′′ = tri-valent transition metal, O = oxygen) are discovered to have a variety of remarkable properties, including ferroelectricity, half-metallic transport, piezoelectricity, magnetic ordering, and a large MCE.22–24 By carefully selecting and combining the constituent ions, it is possible to realize various exciting properties based on changes in the ionic radii and electronic configurations of B′ and B ions.25 Among the double perovskite families, R2MMnO6 (R = rare earth element, M = Ni, Co) has recently attracted substantial interest due to its controllability of magnetic and electronic properties.26,27 These DP compounds contain Co2+ ions with electronic configuration d7 tse2g and Mn4+ with electronic configuration d3 t2g3 e0g, which exhibit ferromagnetic ordering due to Co2+ and Mn4+ superexchange interactions.28,29 These types of DP compounds are mostly ferromagnetic and insulating, with the formation of ferromagnetic cluster. The evolution of a Griffiths-like phase is observed in the paramagnetic region for some cases. Due to the antisite disorder in this type of DP, antiferromagnetic ordering has also been observed at lower temperatures. In some compounds, anti-parallel ordering of Co2+/Mn4+ and R3+ spins have also been observed, resulting in antiferromagnetic state at low temperature. Among the various rare-earth based DP materials, Ho2B′B′′O6 is relatively less studied compound.13,15,17,22 However holmium, with its high magnetic moment and smaller ionic radius compared to other rare-earth elements, could be an intriguing element for A-site in determining structural and magnetic properties of DP materials system.
It is known that non-Ho DP compounds, such as Gd2NiMnO6 and Gd2CoMnO6 exhibits significant MCE at low temperatures, with – ΔS values of 35.5 J kg−1 K−1 and 24 J kg−1 K−1, respectively, for H = 0–7 T.30 In a study by Zhang et al.31 cryogenic magnetic properties and magnetocaloric effect in Pr2NiMnO6 and Pr2CoMnO6 compounds, reporting the second-order phase transition with −ΔS values of 3.15 at 208 K and 3.91 J kg−1 K−1 at 170 K under an applied field of 7 T. In our previous work,32 we reported that the varying the B-site in double perovskite materials prepared by solid-state reaction with different transition metal elements results in variation of Curie temperature. Li et al.33 recently published a study on the effect of B′-site ordering on magnetocaloric and critical behavior in Nd2BMnO6 with B = Ni and Co. It should be mentioned that the preparation conditions highly influence the microstructure of the DP materials, with determination of related physical properties of DP materials.34 Recently, magnetocaloric properties in distorted double perovskite due to different ionic radius of rare earth elements in RE2NiTiO6 have been reported. It was found that in case of Gd2NiTiO6 compound MCE properties are better than other two compounds.35 The transition temperature and magnetocaloric effect can be enhanced by substituting non-magnetic Ti3+ ion in Gd2CoMnTiO6 compound by Zheng et al.36 It was found that MCE enhanced from 16.64 J kg−1 K−1 to 27.16 J kg−1 K−1 under ΔH = 6 T at cryogenic temperature. Patra et al.37 reported the synthesis of Ho2CoMnO6 nanorod by using hydrothermal route and magnetocaloric effect gives a change in entropy value of 12.4 J kg−1 K−1 at low temperature for 7 T.
Here, we report the fabrication of nanocrystalline Ho2NiMnO6 and Ho2CoMnO6 compounds using the sol–gel method. The structural, magnetic, and magnetocaloric behavior have been systematically investigated, where the Griffiths phase (GP) behavior and first-/second-order phase transition behavior are intensively explored.
2. Experimental
Double perovskite Ho2NiMnO6 (HNMO) and Ho2CoMnO6 (HCMO) were synthesized using the sol–gel method. The stoichiometric amounts of high purity Ni(NO3)2·6H2O, Co(NO3)2·6H2O, and Mn(NO3)2·xH2O from Sigma Aldrich were dissolved in distilled water. The trivalent rare earth Ho2O3 oxide was dissolved in concentrated nitric acid along with few drops of oxalic acid. In the metallic salt mixture, an appropriate amount of citric acid and ethylene glycol is added, and the solution pH is maintained to 8 using ammonia. The final mixture was kept on the hot plate at 80 °C with constant stirring for 4 h to get a homogeneous gel. The temperature of hot plate was increased to 100 °C to burn the gel to get the final product in the form of powder. The resulting powders were grounded mechanically and sintered at 1200 °C for 48 h. The phase purity of the prepared compounds and crystal structures were confirmed using powder X-ray diffraction (PXRD) with Cu-Kα radiation at room temperature by using the Bruker D8 Advance diffractometer. The high-resolution transmission electron microscopy (HRTEM) studies were carried out with a HITACHI HF 5000 electron microscope. The magnetic properties were measured by using the Magnetic Properties Measurement System (MPMS, Quantum design). The temperature-dependent magnetization curve was measured in FC (field cooling) and ZFC (zero field cooling) mode at an applied magnetic field (H = 100 Oe, 500 Oe, 1000 Oe, and 10
000 Oe) in a temperature range of 2–200 K. The temperature dependence of magnetization isotherms were recorded with 3 K steps in the 0–5 T range around the Curie temperature (TC) for each compound to estimate magnetic entropy change.
3. Results and discussion
The X-ray diffraction (XRD) patterns for Ho2NiMnO6 (HNMO) and Ho2CoMnO6 (HCMO) powder compounds are illustrated in Fig. 1(a and b). The crystallographic information of the present compounds has been extracted by Rietveld refinement using the Topas software and structural analysis software VESTA.38,39 The XRD analysis confirms that the HNMO and HCMO compounds crystallize in a monoclinic structure with space group P21/n. The refinement parameters such as Rexp, RWP, GoF, lattice constant, volume, bond angle, and bond length for both compounds are summarized in Table 1. It is confirmed that determined parameters are in good agreement with the literature.40,41 The peak located at 2θ = 29.2° corresponds to the impurity phase of Ho2O3, and the impurity phase contributions are approximately 10.35 and 7.63 wt% for HNMO and HCMO, respectively. NiO weak impurity phase is observed as well in case of HNMO compound. The impurity phases of rare-earth oxides exist particularly in DP compounds prepared by sol–gel method as reported by Zhang et al.42 Inset of Fig. 1 shows the three-dimensional atomic arrangement of the monoclinic structure of HNMO and HCMO. The octahedral structure of CoO6/NiO6 and MnO6 are shared in such a way to form the alternate layer in a unit cell of double perovskite as seen in the figure. The octahedron tilt angle and the perovskite structure stability change with the difference in the radius of the A and B/B′ metal ions in double perovskite.43 To confirm the presence of the double perovskite structure and the degree of distortion from the ideal cubic structure, we have calculated the Goldschmidt tolerance factor given by the following formula. |
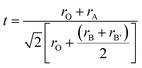 | (1) |
where the rA, rB, and rB′ are the ionic radii of A, B, B′, and O ions, respectively. The t values of HNMO and HCMO determined by using the eqn (1) are 0.869 and 0.878, respectively. The tolerance factor less than 1 indicates that the ionic radius of A-site cation in DP is smaller, which causes stress in structure, correspondingly resulting in the change in the bond length or octahedral tilting.44
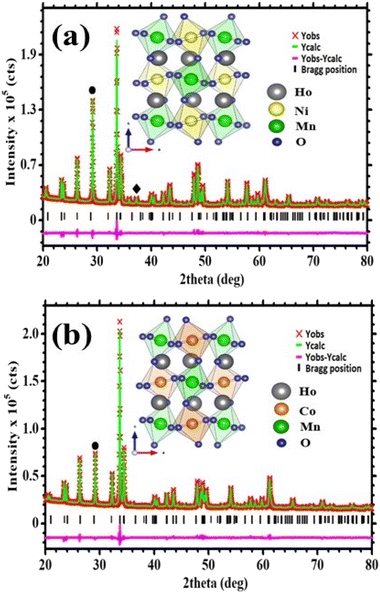 |
| Fig. 1 Room temperature XRD patterns with Rietveld refinement analysis for (a) Ho2NiMnO6 (b) Ho2CoMnO6. Insets: crystal structure of corresponding compounds. | |
Table 1 List of parameters obtained from Rietveld refinement of HNMO and HCMO powder XRD pattern and tolerance parameter
|
Ho2NiMnO6 |
Ho2CoMnO6 |
Space group |
P21/n |
P21/n |
Cell mass (g mol−1) |
1078.98 |
1079.46 |
Cell volume (Å3) |
215.13 |
214.37 |
Crystal density (g cm−3) |
8.33 |
8.36 |
Lattice parameters |
a (Å) |
5.2102 |
5.2016 |
b (Å) |
5.5293 |
5.5370 |
c (Å) |
7.4677 |
7.4431 |
β (°) |
90.115 |
89.981 |
Bond angle (°) |
M–O1–Mn |
147.6 |
147.5 |
M–O2–Mn |
145.3 |
145.2 |
M–O3–Mn |
149.7 |
149.5 |
Av. bond length (Å) |
M–O |
1.99 |
2.07 |
Rexp (%) |
1.10 |
0.77 |
Rwp (%) |
3.98 |
2.38 |
GoF |
3.61 |
3.11 |
t |
0.869 |
0.878 |
μeff (μB) cal. |
15.00 |
15.41 |
μeff (μB) theo. |
15.73 |
15.95 |
Fig. 2(a and b) shows the transmission electron microscope images for HNMO and HCMO compounds. The particles size and shape are not uniform due to high-temperature sintering process. From these images, the nanocrystalline nature of prepared DP compounds is confirmed to have a particle size of about 50–200 nm. The selected area electron diffraction (SAED) pattern for HNMO and HCMO compounds shown in Fig. 2(c and d), exhibits ordered spot diffraction patterns with bright spots at regular positions, evidencing their nanocrystalline nature.
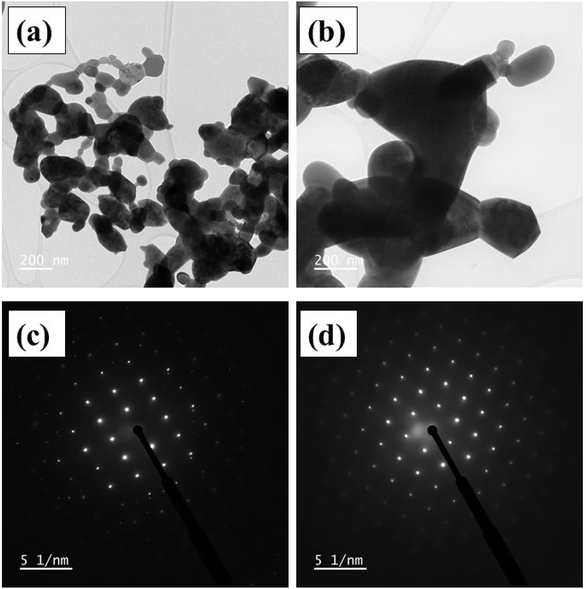 |
| Fig. 2 (a and b) Transmission electron microscope images (c and d) selected area electron diffraction (SAED) patterns of HNMO and HCMO compounds. | |
The temperature-dependent magnetization was measured in zero-field cooled (ZFC) and field cooled (FC) mode as shown in Fig. 3(a and b) for the HNMO and HCMO at different magnetic fields of H = 100, 500, 1000, and 10
000 Oe. In ZFC mode sample was cooled down in the absence of a magnetic field from 300 K to 2 K, and measurement was taken in heating, while in FC mode, the magnetic field was applied, the sample was cooled from 300 K to 2 K, and measurement was taken in heating. The behavior of M–T plots indicates the paramagnetic (PM) to the ferromagnetic (FM) phase transition at the Curie-temperature (TC) which can be determined precisely from the first derivative of the magnetization curve (dM/dT). The determined values of TC are 81 K and 73 K for HNMO and HCMO, respectively, and slightly lower than the reported values in the literature.16,22 The observed divergence between the FC and ZFC curves in HNMO and HCMO was like previous reports on double perovskite compounds, which is considered to be due to the magnetic frustration present in the compounds at a low magnetic field. This magnetic frustration occurs due to the mixed oxidation states of the Mn3+/Mn4+ cations present in the compound. As we increase an applied magnetic field, divergence in ZFC and FC is reduced due to the spin reorientation process, reducing, in turn, the magnetic frustrations present in the compounds. It is evident that the magnetic susceptibility in the paramagnetic phase follows the Curie–Weiss law, the magnetic susceptibility
, where C represents the Curie constant and θ is the Curie–Weiss temperature. The inset of Fig. 3(a and b) shows the temperature dependence of inverse magnetic susceptibility with Curie–Weiss fitting. The experimental value of effective moment in paramagnetic region allows us to calculate μexpeff in the unit of Bohr magneton by using the following equation:45
|
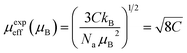 | (2) |
where
Na and
kB represent Avogadro number and Boltzmann constant, respectively. The estimated value of experimental paramagnetic moment was found to be 15.0 and 15.4
μB/f.u for HNMO and HCMO, respectively. The calculated effective magnetic moments are in good agreement with the theoretically calculated values given by using the following equation:
46 |
 | (3) |
where the effective paramagnetic moments for
μeff(Ho
3+) = 10.6
μB,
μeff(Ni
2+) = 2.83
μB,
μeff(Co
2+) = 3.87
μB,
μeff(Mn
4+) = 3.87
μB. The calculated
μTheoeff (
μB) values are 15.73
μB and 15.95
μB for HNMO and HCMO, respectively. It is worth noting that the values obtained from experiments are highly consistent with the theoretical predictions.
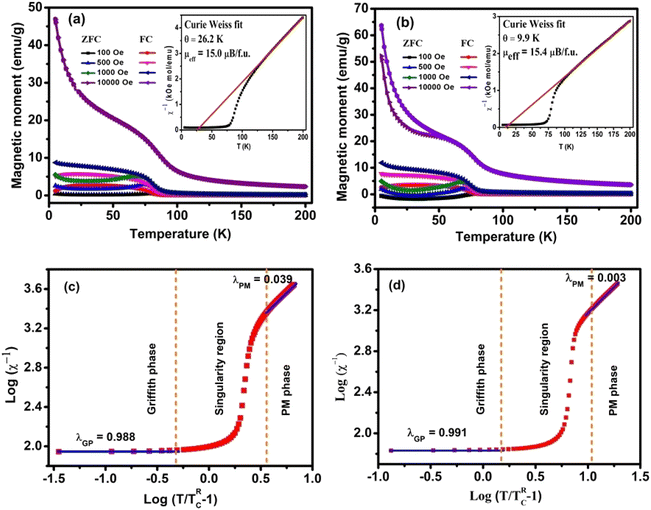 |
| Fig. 3 (a and b) M–T FC-ZFC curves at various applied magnetic fields. Inset shows magnetic inverse susceptibility (χ−1) as a function of temperature (T) under H = 100 Oe. Solid red line denotes Curie–Weiss fitting (C–W) for HNMO and HCMO compounds. (c and d) Log–log plot of χ−1 and (T/TRC − 1) for inverse susceptibility measured at H = 100 Oe by following the eqn (4). | |
It is observed that there is a deviation in the paramagnetic region, indicated by the downturn in the χ−1 vs. T curve. This deviation is characteristic of the Griffiths phase that exists between the disordered paramagnetic phase and the ordered ferromagnetic phase. Both HNMO and HCMO compounds show the presence of the Griffiths phase. The evolution of such phase in Ho2NiMnO6 was reported by Bhatti et al.29 and our previous work. The GP follows a power law,
where
TRC is the random critical temperature and
λ is the magnetic susceptibility exponent which lies between 0 ≤
λ ≤ 1.
47,48 The double logarithmic plot of
χ−1 vs. (
T -
TRC) is shown in
Fig. 3(c and d) for HNMO and HCMO compounds. The exponent
λ is a measure of the deviation from the Curie–Weiss law. The accurate choice of
TRC is essential for the accurate determination of exponent
λ. The value of
TRC was found to be consistent, since
TRC is recognized as the temperature for which fitting the data in the PM region yielded a
λ value of nearly zero.
49 The
λGP is estimated as 0.988 and 0.991 for HNMO and HCMO, respectively, while
λPM is 0.064 and 0.005 from the linear portion of the curve at the applied magnetic field of 100 Oe. The larger values of
λGP (∼1) implies the Griffiths singularity.
The temperature-dependent magnetization M (H) curves with ΔT = 3 K around their TC with an applied magnetic field of 0–5 T for HNMO and HCMO are illustrated in Fig. 4(a and b). These M (H) data have been used to evaluate the magnetocaloric effect and the order of the magnetic phase transition. The magnetization curves show non-linear behavior, where, above TC, linear behavior is observed, implying typical characteristics of ferromagnetic materials at finite temperatures due to thermal agitation disorienting the magnetic moments. The nature of the magnetic phase transition has been studied by analyzing the Arrott plots (M2 vs. H/M) from M–H measurements, as shown in Fig. 4(c and d) for HNMO and HCMO compounds. According to Banerjee's criterion, a positive slope corresponds to the second-order magnetic transition, while a negative slope corresponds to the first-order magnetic transition.50 From Fig. 4(a) and (b), the positive slopes without inflection in the low and high field regions are observed, indicating that the samples in the present study undergo the second order FM–PM phase transition.
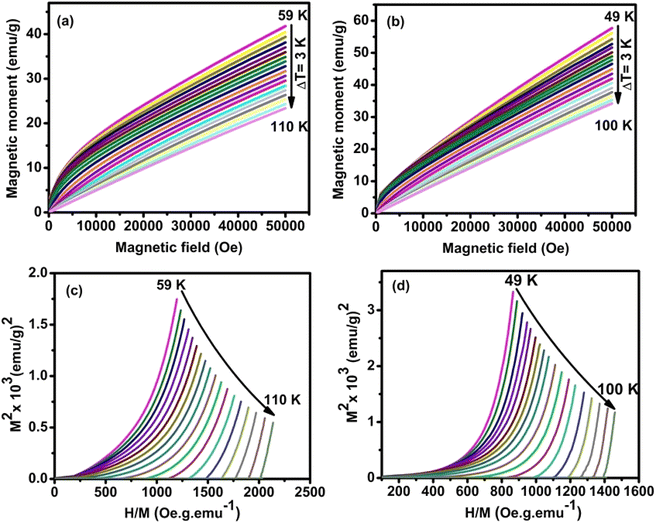 |
| Fig. 4 (a–d) Isothermal magnetization curves and Arrot plots (M2 vs. H/M) at a various temperature in the vicinity of TC with ΔT = 3 K for HNMO and HCMO compounds. | |
The total entropy in a system consist of isothermal magnetic entropy (Sm), lattice entropy (Slat), and electronic entropy (Sele) and is given as,
The contributions of lattice and electronic entropy are considered to be negligible, hence, the total entropy can be approximated as the isothermal entropy change (ΔS), which can be estimated from the magnetization isotherms M (H, T) shown in Fig. 4(a and b). Based on the thermodynamic theory, the isothermal field-induced magnetic entropy change (ΔS) from 0 to Hmax can be calculated by using the relation:12–15
|
 | (6) |
The relationship between entropy and magnetization can be obtained by using the Maxwell equation (∂S/∂H)T = (∂M/∂T)H, then eqn (6) becomes,
|
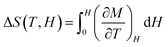 | (7) |
Fig. 5(a and b) shows the temperature dependence of magnetic entropy change (ΔSM) calculated at different applied magnetic fields from 1 to 5 T for HNMO and HCMO compounds. Both compounds show similar behavior in MCE curve, –ΔSM value reaches a maximum ΔSMax, near the transition temperature (TC) at low applied fields, and it increases with respect to H due to the improved ferromagnetic interactions. The calculated –ΔSMax around 86 K, and 77 K are 1.7 and 2.2 J kg−1 K−1 for HNMO and HCMO samples, respectively, at ΔH = 0 – 5 T. There might be a room for enhancement magnetocaloric properties at cryogenic temperatures, while it should be mentioned that the HNMO and HCMO samples in the present study are prepared by sol–gel method with nanocrystalline features. The magnetocaloric properties for studied HNMO and HCMO, along with other double perovskite compounds, are tabulated in Table 2. The comparison of −ΔS values shows significant difference in values for HNMO whereas, in the case of HCMO, it is increased slightly. The TC values are different from our previous report as well as values reported by Chakraborty et al.15 The differences in isothermal entropy change between studied samples with the literature is associated with the mass density, homogeneity, and quantitative relations between used reactants in the sample synthesis which are responsible for change in crystallite size and particle size. With decreasing crystallite size there is suppression of TC, magnetization, and magnetic entropy change reported by Phan et al.51 The shape of – ΔS curves is symmetric around TC while it is not exactly symmetric in literature,14 leading to the difference in – ΔS peak and – ΔSMax values. The calculated values of – ΔSMax are plotted as a function of H as shown in Fig. 6. It is clearly observed that ΔSMax is proportional to an applied magnetic field.
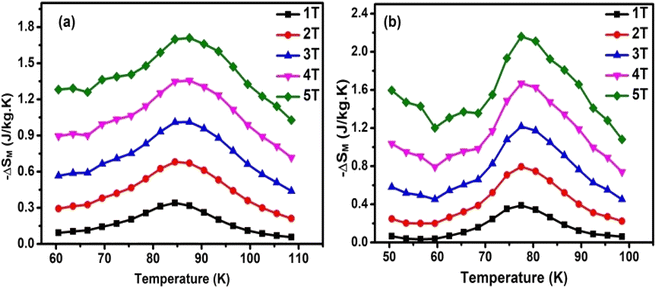 |
| Fig. 5 Temperature dependence of magnetic entropy changes under different magnetic fields for (a) HNMO (b) HCMO compounds. | |
Table 2 ble 2Comparison of MCE properties of HNMO and HCMO with other rare earth based double perovskite compounds
Compound |
TC (K) |
ΔH (T) |
−ΔSMax (J kg−1 K) |
References |
Ho2NiMnO6 |
81.2 |
5 |
1.7 |
Present |
Ho2CoMnO6 |
73.5 |
5 |
2.2 |
Present |
Ho2NiMnO6 |
71.5 |
5 |
3.1 |
32 |
Ho2CoMnO6 |
69 |
5 |
1.8 |
32 |
Ho2NiMnO6 |
86 |
5 |
6.4 |
15 |
Ho2CoMnO6 |
8 |
5 |
8.7 |
17 |
Nd2NiMnO6 |
156 |
5 |
2.9 |
33 |
Nd2CoMnO6 |
199 |
5 |
2.7 |
33 |
Pr2NiMnO6 |
208 |
7 |
3.15 |
24 |
Pr2CoMnO6 |
170 |
7 |
3.91 |
24 |
La2NiMnO6 |
260 |
3 |
0.98 |
56 |
La2CoMnO6 |
225 |
5 |
1.2 |
57 |
Eu2NiMnO6 |
143 |
5 |
3.2 |
13 |
Gd2NiMnO6 |
130 |
5 |
3.8 |
13 |
Tb2NiMnO6 |
112 |
5 |
3.5 |
13 |
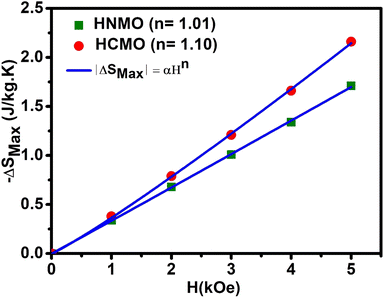 |
| Fig. 6 The ΔSMax values at a different magnetic field of HNMO and HCMO compounds fitted with a function ΔSMax = α × Hn, the values of exponent “n” are labeled in the figure. | |
Another method was used for crosscheck to determine the order of magnetic phase transitions. The field dependence of – ΔS of the sample is determined by utilizing the relation ΔSMax = aHn, where a is constant and “n” is an exponent related to the magnetic order. Fig. 6 depicts – ΔSMax with respect to the magnetic field along with power law fitting, and the resulting values of “n” are 1.01 and 1.10 for HNMO and HCMO compounds, respectively, which are higher than the reported values (n = 0.67) for mean field ferromagnetic materials.12 The magnetic order can be analyzed from the equation ΔSMax ∝ Hn, where the value of n depends on magnetic field and temperature, which can be calculated as follows:52–55
|
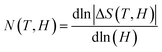 | (8) |
Fig. 7(a and b) shows temperature dependence of N (T, H) at different magnetic field variation ΔH with 1, 2, 3, 4, and 5 T for HNMO and HCMO compounds. The overshoot of N (T, H) value above 2 in case of HCMO compound at lower temperature region confirms the presence of FOMT in the compound along with SOMT while in case of HNMO compound N (T, H) below the value 2 is confirming SOMT present in the compound. The shape of the N (T, H) curves also indicates the FM–PM transition separated by the minimum around the Curie temperature. According to mean field theory (MFT), for long-range ferromagnet, at T = TC, N tends to be the minimum value equal to 2/3 and n = N (TC), while it tends to be 1 and 2 as T ≪ TC and T ≫ TC, respectively. The observed values of n near TC are 1.03 and 1.15 for HNMO and HCMO, respectively, which are higher than MFT values, confirming the presence of the short-range magnetic order in these compounds. The experimentally determined values of n and N (T, H) at T = Tc suggest that both the HNMO and the HCMO experienced the second-order magnetic phase transition.
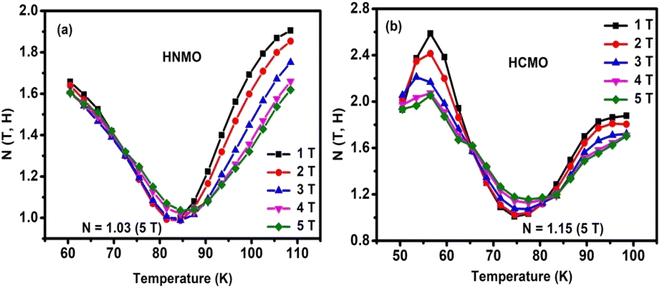 |
| Fig. 7 Temperature dependence of N (T, H) curves for (a) HNMO and (b) HCMO compounds at different magnetic field. | |
4. Conclusions
In summary, nanocrystalline double perovskite HNMO and HCMO were successfully synthesized by the sol–gel method. The Rietveld refinement revealed the formation of DP with space group P21/n, having a monoclinic crystal structure, along with the secondary phase of Ho2O3. The transmission electron micrographs confirmed the formation of agglomerated nanocrystallites with particle size in the range of 50–200 nm. The calculated effective magnetic moments are consistent and close to theoretically predicted values. The downturn behavior in the inverse of magnetic susceptibility indicates the existence of the Griffiths phase, which has been further confirmed by the power law analysis. This feature arises in DP due to B-site lattice distortion, which originates from the smaller radius of the rare earth Ho atom. It is observed that both compounds show maximum MCE around TC. The maximum values of – ΔS are 1.7 J kg−1 K−1 for Ho2NiMnO6 and 2.2 J kg−1 K−1 for Ho2CoMnO6 at H = 50 kOe. The Arrott plot confirms the second-order magnetic phase transition present in both compounds, also consistent with the analysis of magnetic order parameters n and N (T, H), implying the short-range magnetic order present in the studied compounds.
Conflicts of interest
There are no conflicts of interests to declare.
Acknowledgements
This work was supported by the Science Research Program through the National Research Foundation of Korea funded by the Ministry of Education, Science and Technology (NRF-2020R1I1A3070554) and Regional Innovation Strategy (RIS) through the National Research Foundation of Korea (2021RIS-004). Dr Y. Jo acknowledges the support by KBSI grant (No. 300200). Prof. D.-H. Kim would like to acknowledge the support by the Ministry of Science and ICT, (Project Number: (2022) ERIC02_2) and Commercialization Promotion Agency for R&D Outcomes (COMPA).
References
- B. G. Shen, J. R. Sun, F. X. Hu, H. W. Zhang and Z. H. Cheng, Recent progress in exploring magnetocaloric materials, Adv. Mater., 2009, 21, 4545 CrossRef CAS.
- V. Franco, J. S. Blazquez, J. J. Ipus, J. Y. Law, L. Moreno-Ramirez and A. Conde, Magnetocaloric effect: from materials research to refrigeration devices, Prog. Mater. Sci., 2017, 93, 112 CrossRef.
- L. W. Li, Review of magnetic properties and magnetocaloric effect in the intermetallic compounds of rare earth with low boiling point metals, Chin. Phys. B, 2016, 25, 037502 CrossRef.
- L. Li and M. Yan, Recent progresses in exploring the rare earth- based intermetallic compounds for cryogenic magnetic refrigeration, J. Alloys Compd., 2020, 823, 153810 CrossRef CAS.
- F. Z. Kassimi, H. Zaari, A. Benyoussef, A. Rachadi, M. Balli and A. E. Kenz, A theoretical study of the electronic, magnetic and magnetocaloric properties of the TbMnO3 multiferroic, J. Magn. Magn. Mater., 2022, 543, 168397 CrossRef CAS.
- L. W. Li, Y. Yuan, Y. Qi, Q. Wang and S. Zhou, Achievement of a table-like magnetocaloric effect in the dual-phase ErZn/ErZn2 composite, Mater. Res. Lett., 2018, 6, 67 CrossRef CAS.
- Y. K. Zhang, D. Guo, B. Wu, H. Wang, R. Guan, X. Li and Z. Ren, Magnetic properties, and magneto-caloric performances in RCo2B2C (R = Gd, Tb and Dy) compounds, J. Alloys Compd., 2020, 817, 152780 CrossRef CAS.
- Y. M. Wang, D. Guo, B. B. Wu, S. H. Geng and Y. K. Zhang, Magnetocaloric effect and refrigeration performance in RE60Co20Ni20 (R = Ho and Er) amorphous ribbons, J. Magn. Magn. Mater., 2020, 498, 166179 CrossRef CAS.
- Y. Zhang, B. Wu, D. Guo, J. Wang and Z. Ren, Magnetic properties, and promising cryogenic magneto-caloric performances of Gd20Ho20Tm20Cu20Ni20 amorphous ribbons, Chin. Phys. B, 2021, 30, 017501 CrossRef CAS.
- K. Laajimi, M. Khlifi, E. K. Hlil, M. H. Gazzah and J. Dhahri, Enhancement of magnetocaloric effect by Nickel substitution in La0.67Ca0.33Mn0.98Ni0.2O3 manganite oxide, J. Magn. Magn. Mater., 2019, 491, 165625 CrossRef CAS.
- T. L. Phan, N. T. Dang, T. A. Ho, J. S. Rhyee, W. H. Shon, K. Tarigan and T. V. Manh, Magnetic and magnetocaloric properties of Sm1-xCaxMnO3 (x = 0.88) nanoparticles, J. Magn. Magn. Mater., 2017, 443, 233 CrossRef CAS.
- K. P. Shinde, E. J. Lee, M. Manawan, A. Lee, S.-Y. Park, Y. Jo, K. Ku, J. M. Kim and J. S. Park, Structural, magnetic, and magnetocaloric properties of R2NiMnO6 (R = Eu, Gd, Tb), Sci. Rep., 2021, 11, 20206 CrossRef CAS PubMed.
- I. N. Bhatti, I. N. Bhatti, R. N. Mahato and M. A. H. Ahsan, Physical properties in nanocrystalline Ho2CoMnO6, Ceram. Int., 2020, 46, 46 CrossRef.
- J. Y. Moon, M. K. Kim, Y. J. Choi and N. Lee, Giant Anisotropic Magnetocaloric Effect in Double-perovskite Gd2CoMnO6 Single Crystals, Sci. Rep., 2017, 7, 16099 CrossRef CAS PubMed.
- T. Chakraborty, H. Nhalil, R. Yadav, A. A. Wagh and S. Elizabeth, Magnetocaloric properties of R2NiMnO6 (R=Pr, Nd, Tb, Ho and Y) double perovskite family, J. Magn. Magn. Mater., 2017, 428, 59 CrossRef CAS.
- L. Li, P. Xu, S. Ye, Y. Li, G. Liu, D. Huo and M. Yan, Magnetic properties and excellent cryogenic magnetocaloric performances in B-site ordered RE2ZnMnO6 (RE = Gd, Dy and Ho) perovskites, Acta Mater., 2020, 194, 354 CrossRef CAS.
- Y. Jia, Q. Wang, P. Wang and L. Li, Structural, magnetic and magnetocaloric properties in R2CoMnO6 (R = Dy, Ho, and Er), Ceram. Int., 2017, 43, 15856 CrossRef CAS.
- L. Li and M. Yan, Recent progress in the magnetic and cryogenic magnetocaloric properties of RE2TMTM’O6 double perovskite oxides, J. Mater. Sci. Technol., 2023, 136, 1 CrossRef.
- R. Das, R. Prabhu, N. Venkataramani, S. Prasad, L. Li, M.-H. Phan, V. Keppens, D. Mandrus and H. Srikanth, Giant low-field magnetocaloric effect and refrigerant capacity in reduced dimensionality EuTiO3 multiferroics, J. Alloys Compd., 2021, 850, 156819 CrossRef CAS.
- M. Balli, B. Roberge, P. Fournier and S. Jandl, Review of the Magnetocaloric Effect in RMnO3 and RMn2O5 Multiferroic Crystals, Crystals, 2017, 7, 44 CrossRef.
- M.-H. Phan and S.-C. Yu, Review of the magnetocaloric effect in manganite materials, J. Magn. Magn. Mater., 2007, 308, 325 CrossRef CAS.
- B. Wu, D. Guo, Y. Wang and Y. Zhang, Crystal structure, magnetic properties, and magnetocaloric effect in B-site disordered RE2CrMnO6 (RE = Ho and Er) perovskites, Ceram. Int., 2020, 46, 11988 CrossRef CAS.
- A. Pal, P. Singh, V. Gangwar, S. Ghosh, P. Prakash, S. Saha, A. Das, M. Kumar, A. Ghosh and S. Chatterjee, B-site disordered driven multiple-magnetic phase: Griffiths phase, re-entrant cluster glass, and exchange bias in Pr2CoFeO6, Appl. Phys. Lett., 2019, 114, 252403 CrossRef.
- A. Hossain, P. Bandyopadhyay and S. Roy, An overview of double perovskites A2BB’O6 with small ions at A site: Synthesis, structure, and magnetic properties, J. Alloys Compd., 2018, 740, 414 CrossRef CAS.
- S. Ivanov, M. Andersson, J. Cedervall, E. Lewin, M. Sahlberg, G. Bazuev, P. Nordblad, R. S. I. Mathieu, M. Andersson, J. Cedervall, E. Lewin, M. Sahlberg, G. Bazuev, P. Nordblad and R. Mathieu, Temperature-dependent structural and magnetic properties of R2MMnO6 double perovskites (R = Dy, Gd; M = Ni, Co), J. Mater. Sci.: Mater. Electron., 2018, 29, 18581 CrossRef CAS.
- Z. Q. Zhang, P. Xu, Y. S. Jia and L. W. Li, Structural, magnetic and magnetocaloric properties in distorted RE2NiTiO6 double perovskite compounds, JPhys Energy, 2023, 5, 014017 CrossRef.
- M. Balli, S. Mansouri, P. Fournier, S. Jandl, K. D. Truong, S. Khadechi-Haj Khlifa, P. de Rango, D. Fruchart and A. Kedous-Lebouc, Enlarging the magnetocaloric operating window of the Dy2NiMnO6 double perovskite by lanthanum doping, J. Phys. D: Appl. Phys., 2020, 53, 095001 CrossRef CAS.
- J. B. Goodenough, Magnetism and the Chemical Bond, Inter-Science, New York, 1976 Search PubMed.
- I. N. Bhatti, I. N. Bhatti, R. N. Mahato and M. A. H. Ahsan, Griffiths phase and magneto-transport study in 3d based nanocrystalline double perovskite Pr2CoMnO6, Phys. Lett. A, 2019, 383, 2326 CrossRef CAS.
- J. K. Murthy, K. D. Chandrasekhar, S. Mahana and D. Topwal, Giant magnetocaloric effect in Gd2NiMnO6 and Gd2CoMnO6 ferromagnetic insulators, J. Phys. D: Appl. Phys., 2015, 48, 355001 CrossRef.
- Y. Zhang, H. Li, D. Guo, L. Hou, X. Li, Z. Ren and G. Wilde, Cryogenic magnetic properties and magnetocaloric performance in double perovskite Pr2NiMnO6 and Pr2CoMnO6 compounds, Ceram. Int., 2018, 44, 20762 CrossRef CAS.
- K. P. Shinde, M. Manawan, S.-Y. Park, Y. Jo, V. M. Tien, Y. Pham, S.-C. Yu, N. Kalanda, M. Yarmolich, A. Petrov and D.-H. Kim, Structural, magnetic and magnetocaloric properties of double perovskite Ho2MMnO6 (M = Fe, Co, and Ni), J. Magn. Magn. Mater., 2022, 544, 168666 CrossRef CAS.
- Y. Li, Q. Lv, S. Feng, K. M. Rehman, X. Kan and X. Liu, A comparative investigation of B-site ordering and structure, magnetic, magnetocaloric effect, critical behavior in double perovskite Nd2BMnO6 (B = Co and Ni), Ceram. Int., 2021, 47, 32599 CrossRef CAS.
- M. Cernea, F. Vasiliu, C. Plapcianu, C. Bartha, I. Mercioniu, I. Pasuk, R. Lowndes, R. Trusca, G. V. Aldica and L. Pintilie, Preparation by sol–gel and solid state reaction methods and properties investigation of double perovskite Sr2FeMoO6, J. Eur. Ceram. Soc., 2013, 33, 2483 CrossRef CAS.
- Z. Q. Zhang, P. Xu, Y. S. Jia and L. W. Li, Structural, magnetic and magnetocaloric properties in distorted RE2NiTiO6 double perovskite compounds, JPhys Energy, 2023, 5, 014017 CrossRef.
- S. S. Zheng, C. L. Li, C. X. Bai, K. X. Zhou, P. Wang, Y. Lu, Y. Qiu and Y. S. Luo, Magnetic properties and enhanced cryogenic magnetocaloric effect in Ti-substituted Gd2CoMnO6 double perovskites, J. Magn. Magn. Mater., 2022, 564, 170162 CrossRef CAS.
- K. Pushpanjali Patra and S. Ravi, Re-entrant Spin Glass and Magnetocaloric Effect in Frustrated Double Perovskite Ho2CoMnO6 Flat Nanorod, J. Magn. Magn. Mater., 2022, 559, 69537 Search PubMed.
- A. A. Coelho, TOPAS and TOPAS-Academic: An optimization program integrating computer algebra and crystallographic objects written in C++, J. Appl. Crystallogr., 2018, 51, 210 CrossRef CAS.
- K. Momma and F. Izumi, VESTA 3 for three-dimensional visualization of crystal, volumetric and morphology data, J. Appl. Crystallogr., 2011, 44, 1272 CrossRef CAS.
- T. Chakraborty, H. S. Nair, H. Nhalil, K. R. Kumar, A. M. Strydom and S. Elizabeth, Disordered ferromagnetism in Ho2NiMnO6 double perovskite, J. Phys.: Condens. Matter, 2017, 29, 025804 CrossRef PubMed.
- Y. S. Jia, Q. Wang, Y. Qi and L. W. Li, Multiple magnetic phase transitions and magnetocaloric effect in double perovskites R2NiMnO6 (R = Dy, Ho, and Er), J. Alloys Compd., 2017, 726, 1132 CrossRef CAS.
- Y. Zhang, B. Zhang, S. Li, J. Zhu, B. Wu, J. Wang and Z. Ren, Cryogenic magnetic properties and magnetocaloric effects (MCE) in B-site disordered RE2CuMnO6 (RE = Gd, Dy, Ho and Er) double perovskites (DP) compounds, Ceram. Int., 2021, 47, 18205 CrossRef CAS.
- S. Vasala and M. Karppinen, A2B’B’’O6 perovskites: a review, Prog. Solid State Chem., 2015, 43, 1 CrossRef CAS.
- W. Z. Yang, X. Q. Liu, H. J. Zhao, Y. Q. Lin and X. M. Chen, Structure, magnetic, and dielectric characteristics of Ln2NiMnO6 (Ln = Nd and Sm) ceramics, J. Appl. Phys., 2012, 112, 064104 CrossRef.
- D. Kumar, P. Jena and A. K. Sing, Structural, magnetic, and dielectric studies on half-doped Nd0.5Ba0.5CoO3 perovskite, J. Magn. Magn. Mater., 2020, 516, 167330 CrossRef CAS.
- M. Retuerto, A. Munoz, M. J. Martinez-Lope, J. A. Alonso, F. J. Mompean, M. T. Fernandez-Diaz and J. Sanchez-Benitez, Magnetic interactions in the double perovskites R2NiMnO6 (R = Tb, Ho, Er, Tm) investigated by neutron diffraction, Inorg. Chem., 2015, 54, 10890 CrossRef CAS PubMed.
- R. B. Griffiths, Nonanalytic behavior above the critical point in a random Ising ferromagnet, Phys. Rev. Lett., 1969, 23, 17 CrossRef.
- K. Anand, M. Alam, A. Pal, P. Singh, S. Kumari, A. G. Joshi, A. Das, A. Mohan and S. Chatterjee, Existence of Griffiths phase and unusual spin dynamics in double perovskite Tb2CoMnO6, J. Magn. Magn. Mater., 2021, 528, 167697 CrossRef CAS.
- S. Karmakar, S. Majumdar, T. K. Nath and S. Giri, Observation of Griffiths phase in antiferromagnetic La0.32Eu0.68MnO3, J. Phys.: Condens. Matter, 2012, 24, 126003 CrossRef PubMed.
- S. K. Banerjee, On a generalised approach to first and second order magnetic transitions, Phys. Lett., 1964, 12, 16 CrossRef.
- T. L. Phan, T. D. Thanh, P. Zhang, D. S. Yang and S. C. Yu, The magnetic phase transition and magnetocaloric effect in Sm0.58Sr0.42MnO3 nanoparticles, Solid State Commun., 2013, 166, 32 CrossRef CAS.
- V. Franco, J. S. Blázquez, J. J. Ipus, J. Y. Law, L. M. Moreno-Ramírez and A. Conde, Magnetocaloric effect: From materials research to refrigeration devices, Prog. Mater. Sci., 2017, 93, 112 CrossRef.
- Y. Zhang, Y. Tian, Z. Zhang, Y. Jia, B. Zhang, M. Jiang, J. Wang and Z. Ren, Magnetic properties and giant cryogenic magnetocaloric effect in B-site ordered antiferromagnetic Gd2MgTiO6 double perovskite oxide, Acta Mater., 2022, 226, 117669 CrossRef CAS.
- Y. Zhang, J. Zhu, S. Li, Z. Zhang, J. Wang and Z. Ren, Magnetic properties and promising magnetocaloric performances in the antiferromagnetic GdFe2Si2 compound, Sci. China Mater., 2022, 65, 1345 CrossRef CAS.
- D. Guo, L. M. Moreno-Ramírez, C. Romero-Muñiz, Y. Zhang, J. Y. Law, V. Franco, J. Wang and Z. Ren, First- and second-order phase transitions in RE6Co2Ga (RE = Ho, Dy or Gd) cryogenic magnetocaloric materials, Sci. China Mater., 2021, 64, 2846 CrossRef CAS.
- T. V. Manh, T. A. Ho, T. D. Thanh, T. L. Phan, M. H. Phan and S. Yu, Critical Behavior and Magnetocaloric effect in La2NiMnO6 Nanocrystals, IEEE Trans. Magn., 2015, 51, 1 Search PubMed.
- M. Balli, P. Fournier, S. Jandl, K. D. Truong and M. M. Gospodinov, Analysis of the phase transition and magneto-thermal properties in La2CoMnO6 single crystals, J. Appl. Phys., 2014, 116, 073907 CrossRef.
|
This journal is © The Royal Society of Chemistry 2023 |
Click here to see how this site uses Cookies. View our privacy policy here.