DOI:
10.1039/D2QI01977A
(Research Article)
Inorg. Chem. Front., 2023,
10, 240-249
In situ surface/interface generation on Cu2O nanostructures toward enhanced electrocatalytic CO2 reduction to ethylene using operando spectroscopy†
Received
14th September 2022
, Accepted 10th November 2022
First published on 11th November 2022
Abstract
Electrocatalytic CO2 reduction reactions (CO2RRs), an efficient method of converting carbon dioxide into valuable fuels and chemicals, are attractive as well as challenging. In this work, Cu2O nanostructures with active facets (face-raised cubic structures (F-Cu2O) with the (100) facet, octahedral structures (O-Cu2O) with the (111) facet and edge- and corner-truncated octahedral structures (T-Cu2O) with both the (100) and (111) facets) were synthesized by a wet chemical reduction method. The surface of Cu2O nanostructures was reconstructed in situ to form Cu2O/Cu with a highly active interface during the conversion of CO2 into C2H4, which is named F-Cu2O/Cu, O-Cu2O/Cu and T-Cu2O/Cu. The C2H4 selectivity on Cu2O/Cu catalysts follows the order of O-Cu2O/Cu < F-Cu2O/Cu < T-Cu2O/Cu, and the faradaic efficiencies of C2H4 are 11.2%, 24.9%, and 58.0% at −1.1 V versus the reversible hydrogen electrode, respectively. The experimental results combined with operando surface-enhanced Raman spectroscopy reveal that the Cu2O/Cu interface enhances *CO adsorption and decreases the activation energy of C–C coupling, which is also supported by density functional theory (DFT) calculations. This study will pave a feasible pathway for electrochemical energy storage and convention by crystal facet engineering and interface engineering.
Introduction
Carbon dioxide (CO2) is greatly responsible for global warming, rising sea levels and other extreme weather patterns, making it imperative to reduce the amount of CO2 released into the environment by human activities.1–3 Electrocatalytic CO2 reduction reactions (CO2RRs) have received widespread attention due to their functions including but not limited to efficient conversion of CO2 into value-added chemical feedstocks with high efficiency, providing an opportunity for clean renewable energy storage.4–6 However, the activity and selectivity of multi-carbon (C2+) products with high commercial values and high energy densities will be severely limited due to the slow kinetics of the C–C coupling step during CO2RRs.7,8 The design of catalysts with high activity and selectivity for C2+ products is the key to promote the development of this field.
Among the various electrocatalysts, copper is a distinct metal catalyst, which can produce considerable amounts of hydrocarbons and alcohols,9–13 because it can adjust the adsorption energy of intermediates and then promote the C–C coupling to form C2+ products.14–16 C2H4 is the main C2+ product and an important organic raw material, which is widely used in various industries.17 Thus far, how to improve the selectivity of Cu-based catalysts for the conversion of CO2 into C2H4 and inhibit the generation of H2 and C1 species is the focus of research in terms of experimental and theoretical aspects.18–22 Extensive research has focused on optimizing compositions,23–25 morphologies,26,27 crystal facets28,29 and other aspects30–32 of Cu-based catalysts to improve the Faraday efficiency of C2H4. For example, Cu nanocubes with three different sizes were synthesized by a colloidal chemistry method to investigate the influence of Cu nanocrystal size on the selectivity and activity of electrocatalytic CO2 reduction products.33 The 44 nm Cu nanocubes were optimal for ethylene production with a maximum FE of 41%. Moreover, Cu2O NPs are more favorable to the selective formation of ethylene with high selectivity as compared to Cu NPs. The controllable construction of Cu0 and Cu+ sites was achieved by controlling the loading of cupric oxide on copper phyllosilicate lamella to steer CO2 electroreduction toward C2H4 production with high performance,34 which proves that Cu+ sites are more beneficial to produce ethylene.
For the metal oxide catalyst, the exposed facet surface not only determines the geometry of catalysts but also has a great effect on the electrocatalytic property of catalysts.35 Cu2O NPs with different crystal facets show different stabilities and catalytic activities.36,37 The product of C2H4 on Cu2O NPs depends on diverse crystal facets, which determine the active sites that stabilize crucial intermediates during CO2RRs. Therefore, crystal facets should be particularly considered in designing efficient CO2RR catalysts. The studies revealed that Cu0/Cu+ sites play a key role in improving C2+ products on Cu2O catalysts.38 However, there are still no systematic studies on the effect of the Cu2O/Cu interface with highly active facets in situ generated for electrocatalytic CO2 reduction to C2H4 on Cu2O nanostructures.
In this work, we prepared Cu2O catalysts with active facets (F-Cu2O, O-Cu2O, T-Cu2O) using diverse surfactant templates. High-resolution transmission electron microscopy (HR-TEM) exhibits that Cu2O is enclosed with different facets, which are F-Cu2O structures with the (100) facet, O-Cu2O structures with the (111) facet and T-Cu2O structures with the (111) and (100) facets, respectively. The surface of Cu2O was reconstructed in situ to form a Cu2O/Cu interface (F-Cu2O/Cu, O-Cu2O/Cu and T-Cu2O/Cu) during the CO2RR. The experimental results indicated that the C2H4 activity and selectivity are intensely influenced by the surface reconstruction and exposed active facets of Cu2O catalysts. The C2H4 selectivity on Cu2O/Cu catalysts follows the order of O-Cu2O/Cu < F-Cu2O/Cu < T-Cu2O/Cu, and the faradaic efficiencies of C2H4 are 11.2%, 24.9%, and 58.0% at −1.1 V vs. RHE, respectively, relating to the exposure of different highly active facets and in situ generated Cu+/Cu interface. The experimental results combined with the operando surface-enhanced Raman spectroscopy and DFT calculations confirmed that the presence of Cu+/Cu interfaces and highly active facets enhances *CO adsorption and decreases the adsorption energy of C–C coupling, thus improving the C2H4 selectivity.
Experimental section
Materials
Sodium citrate tribasic dihydrate (C6H5Na3O7·2H2O), ascorbic acid (AA), sodium hydroxide (NaOH), cupric sulfate pentahydrate (CuSO4·5H2O), copper chloride dihydrate (CuCl2·2H2O), lauryl sodium sulfate (SDS) and polyvinyl pyrrolidone (PVP, Mw = 58
000) were all purchased from Aladdin. Glucoses were obtained from Tianli Chemical Reagents. Nafion (5 wt%) were obtained from Aldrich. All gases such as carbon dioxide and nitrogen were obtained from Airgas.
Catalyst preparation
Synthesis of F-Cu2O.
A F-Cu2O catalyst was prepared by a wet chemical reduction method on the basis of previous reports.39 Specifically, C6H5Na3O7·2H2O (0.1940 g) was first dissolved in 400 mL deionized water and stirred for 20 min. Then, 1 mL CuSO4·5H2O (0.77 M) aqueous solution was dropped into this solution. After stirring for 5 minutes, 1 mL NaOH (4.8 M) aqueous solution was slowly added to this mixed solution at room temperature, and 1 mL ascorbic acid (1.2 M) was added under stirring for 30 min to form a yellow solution. The solution was centrifuged after standing aged for 6 hours, washed three times with ethanol and deionized water and finally dried in a vacuum dryer.
Synthesis of O-Cu2O.
In a typical process,40 9.67 g PVP was added to a round-bottom flask (250 mL) containing 100 mL CuCl2·2H2O (1 mM) aqueous solution and stirred continuously in a 60 °C water bath. Then, 10 mL NaOH (2 M) aqueous solution was subsequently added to the round-bottom flask and stirred continuously for 30 min. Glucose (1.2 g) was added to this mixture and stirred vigorously for 3 h. All of the processes were maintained at 60 °C. The precipitates were centrifuged three times in deionized water and anhydrous ethanol, and dried under vacuum at 60 °C for 6 h.
Synthesis of T-Cu2O.
In a typical synthesis,40 0.085 g CuCl2·2H2O (5.65 mM) was added to 88.2 mL solution containing 0.87 g SDS (34.01 mM) and then stirred vigorously at 60 °C for 10 min. After that, 1.8 mL NaOH (1 M) and 5 mL glucose (0.1 M) were rapidly added to this solution and maintained under magnetic stirring for 1 h. The color of the suspension gradually changed to orange. The orange product was collected via centrifugation, washed several times with ethanol and deionized water to remove excess SDS, and finally dried under vacuum for further use.
Characterization of the catalysts
TEM was performed using a JEM 2100 to characterize the morphologies and size of the as-synthesized Cu2O catalysts. An X′Pert3 Powder instrument at 45 kV and 40 mA was used to analyze the powder X-ray diffraction (XRD) pattern of Cu2O catalysts. X-ray photoelectron spectroscopy (XPS) and Cu LMM spectra were recorded using a Thermo Scientific K-Alpha instrument to obtain the structure of catalysts. The chemical structures of Cu2O samples were analyzed by Infrared Spectroscopy (IR).41,42
Electrocatalytic activity measurements
The catalyst ink was prepared by dispersing 20 mg Cu2O and 5 μL of Nafion solution (5 wt%) in 1 mL isopropanol for at least 30 min by ultrasonication.43 Subsequently, the ink was coated onto the polished glassy carbon electrode (diameter: 3 mm; area: 0.07 cm2) surface. The electrode was then dried in ambient air to obtain the working electrode for later electrochemical performance tests. Electrochemical performance tests were operated on a workstation (CHI 760E) equipped with an air-tight three-electrode cell, which used a glassy carbon (GC) electrode coated with catalysts as the working electrode. A Pt sheet and a Ag/AgCl (saturated KCl) electrode were used as the counter and reference electrodes, respectively. First, 0.1 M KHCO3 solution was used as the electrolyte for all electrochemical CO2RRs. The solution in the cathodic cell was saturated with N2 or CO2 before electrochemical testing and 20 sccm flow rate was maintained during the CO2RR. The potential was converted into the reversible hydrogen electrode (RHE) as follows:
E(vs. RHE) = E(vs. Ag AgCl) + 0.197 V + 0.0591 V × pH |
The gaseous products (i.e., H2, CO, CH4 and C2H4) were quantified using a gas chromatograph (GC, Panna) equipped with a thermal conductivity detector (TCD) and a flame ionization detector (FID). The GC was directly linked to an electrochemical cell for online analysis. The faradaic efficiency of gaseous products was calculated using the following equation:44
where
n is the number of electrons required to produce a molecular product (
e.g., 2, 2, 8, and 12 e
− for CO, H
2, CH
4 and C
2H
4),
F is the Faraday constant (96
![[thin space (1/6-em)]](https://www.rsc.org/images/entities/char_2009.gif)
485 C mol
−1),
Ci is the measured concentration of the products by
GC,
G is the gas flow rate,
j is the current density,
p is the atmospheric pressure, 1.013 × 10
5 Pa,
R = 8.314 J mol
−1 K
−1, and
T = 273.15 K.
The liquid mixture was analyzed by 1H nuclear magnetic resonance, which was recorded using a Bruker Avance NEO 400 MHz spectrometer in deuterium oxide-d2 with DMSO as internal standards.9
Double-layer capacitance (Cdl)
The Cdl value was relative to the electrochemical active surface area. The Cdl value was estimated by cyclic voltammetry (CV) to measure the relationship between the scanning rate and capacitive current density. The potential of CV ranged from 0.4 V to 0.5 V vs. RHE. The Cdl value was estimated by plotting Δj (ja − jc) at −0.15 V vs. RHE against the scan rates (ja = anodic current density and jc = cathodic current density). The slope of Cdl was estimated to be twice that of Cdl.41
In situ surface-enhanced Raman spectroscopy (SERS)
The potential-dependent SERS was conducted by combining an in situ surface enhanced Raman spectrometer25 with an optical microscope (excitation wavelength 633 nm, sample power 3 mW). A glass carbon electrode, a Pt wire and a Ag/AgCl (saturated KCl) electrode were used as the working, counter and reference electrodes, respectively. During the SERS test, 0.1 M KHCO3 saturated by high-purity CO2 was continuously fed into the electrolytic cell. Raman spectra were recorded in the range of 100 to 3000 cm−1 using a 633 nm laser. The Raman spectra were acquired at about 10 min after the potential was initially applied.
DFT calculations
CASTEP calculation of Materials Studio was used for DFT calculations. We used the Perdew–Burke–Ernzerhof (PBE) exchange correlation functional of the generalized gradient approximation (GGA) with a cutoff energy of 450 eV. A customized (3 × 3 × 1) k-point grid was adopted for all the calculations.45 The convergence criterion of residual force and energy was set to 0.03 eV Å−1 and 10−5 eV in order to avoid the interaction between periodic units during structural relaxation, and the vacuum space in the z direction is larger than 20 Å. The Cu2O/Cu interface model is supported by a two-layer Cu(100)-(3 × 3), four-layer Cu2O(100)-(2 × 2) slab or nine-layer Cu2O(111)-(2 × 2) slab with 15 Å vacuum space. The change in free energy was calculated by the computational hydrogen electrode (CHE) method.46
Results and discussion
Composition and morphology of Cu2O nanocatalysts
Cu2O nanostructures with highly active facets were successfully prepared by a wet chemical reduction method with diverse surfactants. In this process, surfactants play a key role in regulating the exposed crystal facets of Cu2O by changing the crystallization agent, especially meaning that different types and amounts of surfactant applications lead to crystal growth differential rate and direction, thus laying the foundation for the ultimate morphology of Cu2O nanostructures. The morphology of as-synthesized Cu2O was investigated by TEM. As shown in representative TEM images, the as-synthesized F-Cu2O, O-Cu2O, and T-Cu2O nanostructures exhibit the desired face-raised cubic structure (Fig. S1a, ESI†), octahedral structure (Fig. S2a, ESI†) and edge- and corner-truncated octahedral structure (Fig. S3, ESI†). In detail, Fig. S1b† shows the HR-TEM image of the F-Cu2O catalyst enclosed with excellent crystallinity of the (100) facets. Besides, the F-Cu2O catalyst possesses uniform particle size distribution, as shown in Fig. S1c.† Similarly, the O-Cu2O catalyst is composed of the (111) facet (Fig. S2b, ESI†) and the T-Cu2O catalyst is composed of the (100) and (111) facets (Fig. 1a and d), which are related to the types and amounts of surfactants (PVP and SDS) during the synthesis process. The different d-spacing values of lattice fringes from HR-TEM images also confirm the existence of the abovementioned crystal facets. Fig. 1a, d, and S3† give the TEM, high HR-TEM, and EDS-elemental mapping images of T-Cu2O viewed along the different zone axis directions, from which it could be found that the T-Cu2O catalyst is composed of 18 (100) facets and 8 (111) facets. Fig. S3b and e† show the HR-TEM images of the T-Cu2O catalyst and show the distinct lattice fringes with d spacings of 2.13 and 2.45 Å, which correspond to the (100) and (111) lattice facets of T-Cu2O. The lattice plane directions are also in compliance with the respective particle orientation. For the F-Cu2O catalyst, it shows a cubic shape, which can be assumed that the shape of F-Cu2O is enclosed with 6 protruded (100) facets with 12 edges. The lattice fringe of the F-Cu2O catalyst is 2.13 Å and matches the (100) facet, as shown in Fig. S1b.† For the O-Cu2O catalyst, it is enclosed with 8 (111) facets, with a lattice spacing of 2.45 Å corresponding to the (111) facet. Cu2O nanostructures with different morphologies and facets were characterized by EDS-elemental mapping technique. Fig. S1c, 2c and 3† indicate that Cu and O elements are averagely distributed through Cu2O catalysts.
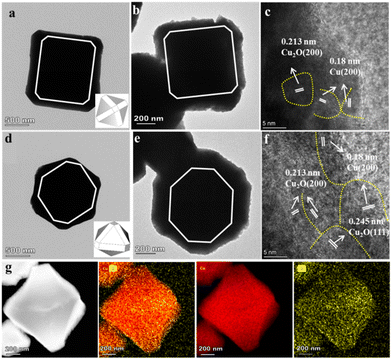 |
| Fig. 1 Structural characterization of catalysts. The TEM images of T-Cu2O viewed along the (a) [100] and (d) [111] directions, and the (b and e) TEM images, (c and f) HR-TEM images, (g) HAADF-STEM and elemental mapping images (Cu species were in red, and O species were in yellow) of surface-reconstructed T-Cu2O samples after reduction at −1.1 V vs. RHE. | |
The catalysts are reduced at −1.1 V vs. RHE to investigate the surface reconstruction of Cu2O and the formation of Cu+/Cu0 interface during CO2RRs. TEM, HR-TEM and EDS-elemental mapping images of F-Cu2O, O-Cu2O and T-Cu2O catalysts after stability test during CO2RRs are shown in Fig. 1, S1d–f and S2d–f.† It is clear that Cu2O structures are well preserved and the Cu+/Cu0 interface was formed, as revealed from the lattice fringes (Fig. S1e, S2e, 1c and f†). The surface reconstruction of F-Cu2O, O-Cu2O and T-Cu2O catalysts is named F-Cu2O/Cu, O-Cu2O/Cu and T-Cu2O/Cu.
XRD and XPS techniques were used to investigate the crystalline state and chemical valence states of Cu2O before and after surface reconstruction. As shown in Fig. 2a–c, the three samples with different exposed active facets are in good agreement with the simulated diffraction patterns of Cu2O (PDF#05-0667) in the range of 29.55°–77.32°, and the XRD results also show that all the as-prepared Cu2O catalysts manifest two intensive peaks at 36.4°and 42.3°, which belong to the (111) and (200) facets of Cu2O before CO2RRs. In addition, all these peaks are almost unchanged after CO2RRs and two new peaks appeared at 43.3°and 50.43°, corresponding to the (111) and (200) facets of metallic Cu (PDF# 04-0836). The XRD results demonstrate that the structure of the as-synthesized Cu2O catalyst is relatively stable, and partial Cu2O is reduced to Cu, which is consistent with the TEM images. The chemical state of F-Cu2O, O-Cu2O, and T-Cu2O catalysts was further studied by XPS measurement. The XPS results supported by XRD before CO2RRs show that there are O and Cu elements in all three samples, among which Cu+ species appeared in the Cu 2p spectrum, as shown in Fig. S4.† Fig. S4a† reveals that Cu2O catalysts have a similar sharp Cu+ peak at 932.5 eV. The presence of satellite peaks at 945.8 eV also proves the presence of Cu+.47 In addition, low-intensity characteristic peaks at 933.5 eV and 953.0 eV are associated with Cu2+ 2p3/2 and Cu2+ 2p1/2, respectively, which indicates that there is a small amount of CuO in the Cu2O nanostructure, due to the natural surface oxidation during the ex situ XPS measurement.48 Subsequently, the valence states of samples immediately after CO2RRs was characterized by XPS. After CO2RRs, the weak peak of Cu2+ disappeared (Fig. 2d) and had a main Cu 2p3/2 feature at 932.5 eV, which can be vested in either Cu2O (Cu+) or Cu (Cu0) but is not distinguishable, as the binding energies between Cu+ and Cu0 differ by only 0.1 eV (Fig. 2d).49 The characteristic peak at 531 eV is related to the existence of lattice oxygen and oxygen vacancies (Fig. 2e). In addition, the Raman spectra (Fig. S5†) of the reconstructed Cu2O catalysts exhibit an absorption peak around 150 cm−1, which could be ascribed to an oxygen defect excited mode. Fig. 2f provides the Cu LMM spectra to further distinguish Cu+ and Cu0. The characteristic peak of Cu0 at 568.0 eV is presented in the Cu LMM spectrum (Fig. 2f) and the main peak of Cu+ is retained (569.9 eV), which further indicates that Cu2O is reduced only on the surface, but the bulk structure is relatively stable. The surface compositions of Cu+ and Cu0 on the different Cu2O catalysts after CO2RRs are clearly distinguished by Cu LMM spectra and XPS analysis, as shown in Table S1.† The Cu+ content of T-Cu2O catalyst is significantly higher than that of F-Cu2O and O-Cu2O catalysts after surface reconstruction.
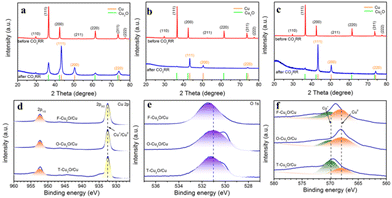 |
| Fig. 2 XRD patterns of catalysts: (a) F-Cu2O, (b) O-Cu2O and (c) T-Cu2O. XPS spectra of (d) Cu 2p, (e) O 1s and (f) Cu LMM of F-Cu2O, O-Cu2O and T-Cu2O after CO2RRs at −1.1 V vs. RHE until stable. | |
Catalyst performance
The electrocatalytic CO2RR performances of F-Cu2O/Cu, O-Cu2O/Cu and T-Cu2O/Cu were also investigated in CO2-saturated 0.1 M KHCO3 (pH = 6.8) aqueous solutions in a typical air-tight three-electrode electrochemical system. The electrocatalytic activity of Cu2O/Cu for CO2RRs is assessed in a N2 and CO2-saturated 0.1 M KHCO3 solution by linear sweep voltammetry (LSV), respectively, and the LSV curves are recorded with a sweep speed of 20 mV s−1 in the potential range from 0 to −1.4 V (vs. RHE). Obviously, the total current densities of three Cu2O/Cu catalysts are all higher in CO2-saturated electrolytes than those in N2-saturated electrolytes, as shown in Fig. S6,† which suggests that the electrocatalytic activity of the catalysts for CO2RRs is better than that for hydrogen evolution reactions (HERs).50 In addition, the LSV curves (Fig. 3a) exhibit the onset potentials decreasing following the order of T-Cu2O/Cu > F-Cu2O/Cu > O-Cu2O/Cu, indicating that the onset potential and the electrocatalytic activity of T-Cu2O/Cu catalyst are higher than those of the other two catalysts. Moreover, the electrochemical double-layer capacitance measurement was implemented to evaluate the electrochemical surface area (ECSA) of Cu2O/Cu catalysts. According to CV curves at different scanning rates, the ECSA of catalysts was obtained through calculating the double-layer capacitance (Cdl) of the catalysts. T-Cu2O/Cu exhibits the largest ECSA value among the three catalysts (Fig. S7†), which indicates that T-Cu2O/Cu can provide a more accessible active site as compared with the other catalysts. Gas chromatography (GC) and 1H NMR spectroscopy (Fig. S8, S9 and Table S2†) are used to analyze the effluent gaseous products and liquid products formed in CO2RRs at different applied potentials between −0.8 and −1.2 V vs. RHE. The products from CO2RR and the relevant faradaic efficiencies are displayed in Fig. 3b. Compared with the electrocatalytic performances of Cu2O/Cu catalysts, T-Cu2O/Cu exhibits better activity and electrocatalytic conversion efficiency than those of F-Cu2O/Cu and O-Cu2O/Cu. Specifically, the total faradaic efficiency reaches 97.8% over T-Cu2O/Cu at −1.1 V vs. RHE. Notably, the C2+ products of CO2RRs are mainly C2H4 and C2H5OH on the three Cu2O/Cu samples and the maximum FE of C2+ products achieve 69.0% at T-Cu2O/Cu. The FE values of C2H4 and H2 on T-Cu2O/Cu as a function of applied potential are compared in Fig. 3c. As presented, T-Cu2O/Cu displays the highest selectivity to C2H4, achieving 58.0% at −1.1 V vs. RHE, while F-Cu2O/Cu and O-Cu2O/Cu reach 24.9% and 11.2%, respectively. The evolution of H2 is inhibited by T-Cu2O/Cu, and the FE of H2 is less than 30% in the whole potential range. Therefore, the T-Cu2O/Cu catalyst has high selectivity for CO2RRs to C2+ products. The detailed faradaic efficiencies for all products at various potentials are listed in Fig. S10.† It is apparently noted that the selectivity of the products varied with the applied potentials. CO was found to be the major product at relatively low overpotentials (from −0.8 to −0.9 V vs. RHE). With the applied potential being more negative, the FEs for CO production decrease and the FEs for ethylene production increase. This is consistent with the widely accepted hypothesis that the adsorbed CO is an intermediate in the formation of C2+ products.51,52 In addition, the electrocatalytic performance of T-Cu2O/Cu is significantly better than that of Cu-based catalysts reported in the literature under similar experimental conditions (Table S3†).53–61
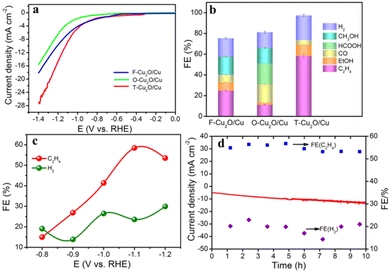 |
| Fig. 3 Electrocatalytic CO2RR performance. (a) LSV curves in a 0.1 M KHCO3 aqueous solution saturated with CO2 for Cu2O/Cu catalysts; (b) faradaic efficiencies of various products at −1.1 V vs. RHE; (c) contrast of FEC2H4 and FEH2 for CO2RRs catalyzed by T-Cu2O/Cu at different applied potentials (−0.8 V to −1.2 V vs. RHE); and (d) stability of T-Cu2O/Cu at a potential of −1.1 V vs. RHE for 10 h. | |
To gain more accurate facet features of the Cu2O catalysts, we applied electrochemical adsorption of OH− on the Cu2O catalysts before and after reconstruction, which take into account their facet-dependent OH− adsorption behaviors.62 As shown in Fig. S11a,† the cyclic voltammetry (CV) curves of the three Cu2O catalysts before reconstruction did not exhibit the OH− adsorption/desorption peaks. As for the reconstructed Cu2O samples, the CV curves show OH− adsorption peaks at −0.34 and −0.43 V vs. RHE for F-Cu2O/Cu and O-Cu2O/Cu, which can be assigned to the OH− adsorption on the Cu(100) and Cu(111) surfaces,63 respectively. Such two adsorption peaks exist simultaneously on the T-Cu2O/Cu catalyst, manifesting that T-Cu2O/Cu is dominated by both the {100} and {111} facets (Fig. S11b†). Above all, it can be considered that metallic Cu with the corresponding crystal facets is generated on the surface of the reconstructed Cu2O samples.
The stability is an important factor during CO2RRs in practical application. The electrocatalytic stability of the T-Cu2O/Cu catalyst for CO2RR was examined under −1.1 V vs. RHE for 10 h in 0.1 M KHCO3 by chronopotentiometry technique. The stability result of T-Cu2O/Cu catalyst is given in Fig. 3d, which implies that the T-Cu2O/Cu catalyst has good working stability. It is also noticeable that the increase in FEC2H4 is greatest between initial one and two hours and then decreases slightly. Furthermore, the FE for C2H4 reaches a maximum value of 58.0% when the reduction time is 2 h, indicating that CO2RR performance is stable in the long-term test. The electrocatalytic durability of the T-Cu2O/Cu catalyst was further valued by cycling 2000 cycles between −1.4 and 0 V vs. RHE at a scan rate of 50 mV s−1.As shown in Fig. S12,† the test results showed only a slight decrease in activity at high potentials. The IR and PXRD patterns (Fig. S13 and 14†) of the recycled catalysts showed that the three catalysts can be well maintained during the catalytic reaction.
CO2 electroreduction mechanisms on Cu2O/Cu catalysts
It is widely accepted that CO2RRs to multi-carbon products experience a critical CO dimerization step.64,65 We thus compared the coverage of surface-adsorbed CO (*CO) on T-Cu2O/Cu, F-Cu2O/Cu, and O-Cu2O/Cu catalysts by in situ Raman spectroscopy at an open circuit potential (OCP) and between −0.5 and −0.9 V vs. RHE from 250 to 650 cm−1 and 1550 to 2800 cm−1, and this technique showed good sensitivity to *CO intermediates. In in situ Raman spectroscopy (Fig. S15†), low potentials were employed because the intermediate could be retained and there were not many bubbles on the electrode to affect the Raman spectral signal. As shown in Fig. 4a–c, the characteristic peaks were located at 218, 527 and 623 cm−1, corresponding to Cu2O at the OCP. At the reduction potential from −0.5 V to −0.9 V vs. RHE, the peaks at 360, 532 and 1070 cm−1 were assigned to the Cu–CO stretching mode, Cu–CO, the adsorbed OH group (vibration of Cu–OH), and CO32− modes,66 respectively, indicating the presence of Cu. XRD and XPS results also confirmed the existence of Cu peaks (Fig. 2). With the increase in applied potentials, two noticeable Raman peaks appeared on the T-Cu2O/Cu electrode (Fig. 4d), where the peak at ∼2070 cm−1 originated from the stretching vibration mode of absorbed CO* at the top site.67,68 The peak at ∼1980 cm−1 could be attributable to the interaction between absorbed CO* intermediates,69,70 which benefited C2+ production. However, as for F-Cu2O/Cu and O-Cu2O/Cu, the *CO peak at a lower shift was so weak that could not be observed clearly. There was only the single mode at ∼2070 cm−1, manifesting a less favorable C–C coupling on the two electrodes (Fig. 4e and f), which was consistent with the electrochemical CO2RR results that it mainly produced CH3OH/H2 and could not produce much C2H4 products.71 Moreover, both Raman signals disappeared on the T-Cu2O/Cu electrode at high potentials, indicating that these intermediates reacted to CO2 reduction kinetics at very fast rates.
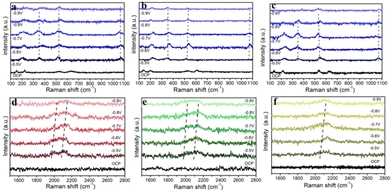 |
| Fig. 4
In situ Raman spectra of CO2RR over (a and d) T-Cu2O/Cu, (b and e) F-Cu2O/Cu and (c and f) O-Cu2O/Cu as a function of the applied potentials. | |
DFT calculation
To gain deeper insights into the high selectivity of C2H4 on T-Cu2O/Cu with the (111)/(100) facet compared with F-Cu2O/Cu with the (100) facet and O-Cu2O/Cu with the (111) facet catalysts, the DFT calculation was carried out to study the free energy of *CO intermediates on Cu2O/Cu with the (111), (100) and (111)/(100) facet. F-Cu2O/Cu(100), O-Cu2O/Cu(111) and T-Cu2O/Cu(111)/(100) models were built based on our HR-TEM results to explore the mechanism of CO2RRs in depth. Fig. 5 shows that the adsorption of *CO intermediate on the surface of Cu2O/Cu(100) and Cu2O/Cu(111)/(100) catalysts was stronger than that on Cu2O/Cu(111), which would subsequently promote the C–C coupling to produce C2+ products in the CO2RR process, and was consistent with the in situ Raman result (Fig. 4d–f). In addition, the adsorption of C2H4 on Cu2O/Cu(111)/(100) and Cu2O/Cu(111) was weaker than that on Cu2O/Cu(100), indicating that Cu2O/Cu(100) could promote the C–C coupling to generate C2+ products, but the C2+ products formed (e.g., C2H4) were difficult to escape from the surface of Cu2O/Cu(100) due to the strong adsorption ability of C2H4. Associated with the experimental results, the synergistic effect of Cu0 and Cu+ was the activation of CO2 on the Cu0 site to promote the following electron transfers, and strengthen the *CO adsorption on the Cu+ site to further facilitate C–C coupling. According to Cu LMM results after reconstruction (see Table S1, ESI†), the T-Cu2O/Cu sample has more Cu+ active sites than the other two catalysts, so *CO adsorption on the T-Cu2O/Cu catalyst is stronger and can further promote C–C coupling.32 Although the adsorption ability of the *CO intermediate on O-Cu2O/Cu(100) was low, C2H4 could be desorbed from the surface of O-Cu2O/Cu(100), due to its weak adsorption energy. Moreover, the *CO intermediate could not only be strongly adsorbed on the surface of T-Cu2O/Cu(111)/(100) to facilitate the C–C coupling, but also C2H4 formed was easily desorbed from the surface of T-Cu2O/Cu(111)/(100). Besides, T-Cu2O/Cu(111)/(100) had better catalytic performance than F-Cu2O/Cu(100) and O-Cu2O/Cu(111), which might be related to the fact that the Fermi energy level of Cu2O on the (111) facet was lower than that on the (100) facet, which promoted the charge transfer between the Cu2O (111) and (100) facets and further facilitated the multi-electron participation kinetics of C2H4 formation (Fig. 5c–d).
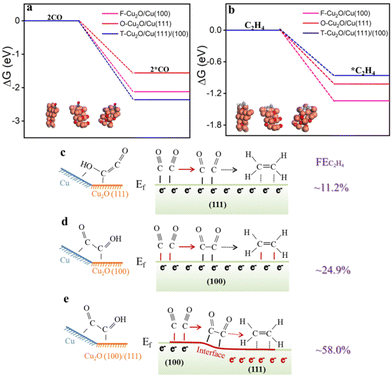 |
| Fig. 5 Adsorption energies of (a) CO and (b) C2H4 on F-Cu2O/Cu(100), O-Cu2O/Cu(111) and T-Cu2O/Cu(111)/(100) catalysts. Formation of C2H4 on (c) O-Cu2O/Cu(111), (d) F-Cu2O/Cu(100) and (e) T-Cu2O/Cu(111)/(100) catalysts. | |
Conclusion
In summary, F-Cu2O, O-Cu2O and T-Cu2O catalysts enclosed with the (111), (100) and (111)/(100) facets have been synthesized by a wet chemical reduction method, which has been reconstructed in situ to form F-Cu2O/Cu(100), O-Cu2O/Cu(111) and T-Cu2O/Cu(111)/(100) catalysts. The experimental results indicated that the T-Cu2O/Cu catalyst shows maximum FEC2H4 values of 58.0% for CO2RRs at −1.1 V vs. RHE, which is much better than those of the F-Cu2O/Cu catalyst (24.9%) and O-Cu2O/Cu catalyst (11.2%). The combination of in situ spectroscopy, electrochemical measurement and DFT calculation results revealed that the faradaic efficiencies of C2H4 on reconstructed Cu2O catalysts is related to the exposure of highly active facets and the synergistic effect of Cu+ and Cu0 (Cu+/Cu0) generated in situ, which can enhance the *CO adsorption and decrease the activation energy of C–C coupling, thus improving the selectivity of the C2H4. This study will pave a feasible pathway for electrochemical energy storage and convention by crystal facet engineering and interface engineering.
Conflicts of interest
The authors declare no conflict of interest.
Acknowledgements
This work was supported by the 111 Project (Grant No. D17007), Henan Center for Outstanding Overseas Scientists (Grant No. GZS2022017), the National Science Foundation of China (Grant No. 21908045, 52072114, 51922008 and 51872075).
References
- M. He, Y. Sun and B. Han, Green carbon science: Scientific basis for integrating carbon resource processing, utilization, and recycling, Angew. Chem., Int. Ed., 2013, 52, 9620–9633 CrossRef CAS PubMed.
- Q. Lu, J. Rosen, Y. Zhou, G. S. Hutchings, Y. C. Kimmel, J. G. Chen and F. Jiao, A selective and efficient electrocatalyst for carbon dioxide reduction, Nat. Commun., 2014, 5, 3242 CrossRef.
- W. Wang, L. Shang, G. Chang, C. Yan, R. Shi, Y. Zhao, G. I. N. Waterhouse, D. Yang and T. Zhang, Intrinsic carbon-defect-driven electrocatalytic reduction of carbon dioxide, Adv. Mater., 2019, 31, 1808276 CrossRef.
- K. Ye, A. Cao, J. Shao, G. Wang, R. Si, N. Ta, J. Xiao and G. Wang, Synergy effects on Sn-Cu alloy catalyst for efficient CO2 electroreduction to formate with high mass activity, Sci. Bull., 2020, 65, 711–719 CrossRef CAS.
- F. F. Chang, M. L. Xiao, R. F. Miao, Y. P. Liu, M. Y. Ren, Z. C. Jia, D. D. Han, Y. Yuan, Z. Y. Bai and L. Yang, Copper-based catalysts for electrochemical carbon dioxide reduction to multicarbon products, Electrochem. Energy Rev., 2022, 5, 4 CrossRef CAS.
- F. Yang, A. Chen, P. L. Deng, Y. Zhou, Z. Shahid, H. Liu and B. Y. Xia, Highly efficient electro conversion of carbon dioxide into hydrocarbons by cathodized copper-organic frameworks, Chem. Sci., 2019, 10, 7975–7981 RSC.
- L. Fan, C. Xia, F. Yang, J. Wang, H. Wang and Y. Lu, Strategies in catalysts and electrolyzer design for electrochemical CO2 reduction toward C2+ products, Sci. Adv., 2020, 6, eaay3111 CrossRef CAS.
- Y. Zheng, A. Vasileff, X. Zhou, Y. Jiao, M. Jaroniec and S. Z. Qiao, Understanding the roadmap for electrochemical reduction of CO2 to multi-carbon oxygenates and hydrocarbons on copper-based catalysts, J. Am. Chem. Soc., 2019, 141, 7646–7659 CrossRef CAS.
- D. Gao, I. Sinev, F. Scholten, R. M. Aran-Ais, N. J. Divins, K. Kvashnina, J. Timoshenko and B. R. Cuenya, Selective CO2 electroreduction to ethylene and multicarbon alcohols via electrolyte-driven nanostructuring, Angew. Chem., Int. Ed., 2019, 58, 17047–17053 CrossRef CAS.
- D. Kim, C. S. Kley, Y. Li and P. Yang, Copper nanoparticle ensembles for selective electroreduction of CO2 to C2−C3 products, Proc. Natl. Acad. Sci. U. S. A., 2017, 114, 10560–10565 CrossRef CAS.
- S. Nitopi, E. Bertheussen, S. B. Scott, X. Liu, A. K. Engstfeld, S. Horch, B. Seger, I. E. L. Stephens, K. Chan, C. Hahn, J. K. Nørskov, T. F. Jaramillo and I. Chorkendorff, Progress and perspectives of electrochemical CO2 reduction on copper in aqueous electrolyte, Chem. Rev., 2019, 119, 7610–7672 CrossRef CAS PubMed.
- C. Xu, X. Zhi, A. Vasileff, D. Wang, B. Jin, Y. Jiao, Y. Zheng and S. Z. Qiao, Highly selective two-electron electrocatalytic CO2 reduction on single-atom Cu catalysts, Small Struct., 2021, 2, 2000058 CrossRef CAS.
- D. Zang and H. Wang, Polyoxometalate-based nanostructures for electrocatalytic and photocatalytic CO2 reduction, Polyoxometalates, 2022, 1, 9140006 CrossRef.
- K. P. Kuhl, E. R. Cave, D. N. Abra and T. F. Jaramillo, New Insights into the electrochemical reduction of carbon dioxide on metallic copper surfaces, Energy Environ. Sci., 2012, 5, 7050–7059 RSC.
- H. Mistry, A. S. Varela, C. S. Bonifacio, I. Zegkinoglou, I. Sinev, Y. W. Choi, K. Kisslinger, E. A. Stach, J. C. Yang and P. Strasser, Highly selective plasma-activated copper catalysts for carbon dioxide reduction to ethylene, Nat. Commun., 2016, 7, 12123 CrossRef.
- F. S. Roberts, K. P. Kuhl and A. Nilsson, High Selectivity for Ethylene from carbon dioxide reduction over copper nanocube electrocatalysts, Angew. Chem., Int. Ed., 2015, 54, 5179–5182 CrossRef CAS PubMed.
- S. Sen, D. Liu and G. T. R. Palmore, Electrochemical reduction of CO2 at copper nanofoams, ACS Catal., 2014, 4, 3091–3095 CrossRef CAS.
- O. A. Baturina, Q. Lu, M. A. Padilla, L. Xin, W. Z. Li, A. Serov, K. Artyushkova, P. Atanassov, F. Xu, A. Epshteyn, T. Brintlinger, M. Schuette and G. E. Collins, CO2 electroreduction to hydrocarbons on carbon-supported Cu nanoparticles, ACS Catal., 2014, 4, 3682–3695 CrossRef CAS.
- J. Kim, W. Choi, J. W. Park, C. Kim, M. Kim and H. Song, Branched copper oxide nanoparticles induce highly selective ethylene production by electrochemical carbon dioxide reduction, J. Am. Chem. Soc., 2019, 141, 6986–6994 CrossRef CAS.
- X. Nie, M. R. Esopi, M. J. Janik and A. Asthagiri, Selectivity of CO2 reduction on copper electrodes: the role of the kinetics of elementary steps, Angew. Chem., Int. Ed., 2013, 52, 2459–2462 CrossRef CAS PubMed.
- G. Wang, J. Chen, Y. Ding, P. Cai, L. Yi, Y. Li, C. Tu, Y. Hou, Z. Wen and L. Dai, Electrocatalysis for CO2 conversion: from fundamentals to value-added products, Chem. Soc. Rev., 2021, 50, 4993–5061 RSC.
- T. Hoang, S. Verma, S. Ma, T. T. Fister, J. Timoshenko and A. I. Frenkel, Nano porous copper-silver alloys by additive-controlled electro-deposition for the selective electroreduction of CO2 to ethylene and ethanol, J. Am. Chem. Soc., 2018, 140, 5791–5797 CrossRef CAS PubMed.
- S. Ma, M. Sadakiyo, M. Heima, R. Luo and P. Kenis, Electroreduction of carbon dioxide to hydrocarbons using bimetallic cu-pd catalysts with different mixing patterns, J. Am. Chem. Soc., 2016, 139, 47–50 CrossRef PubMed.
- X. Yan, C. Chen, Y. Wu, S. Liu, Y. Chen and R. Feng, Efficient electroreduction of CO2 to C2+ products on CeO2 modified CuO, Chem. Sci., 2021, 12, 6638–6645 RSC.
- K. Jiang, R. B. Sandberg, A. J. Akey, X. Liu, D. C. Bell, J. K. Nørskov, K. Chan and H. Wang, Metal ion cycling of Cu foil for selective C−C coupling in electrochemical CO2 reduction, Nat. Catal., 2018, 1, 111–119 CrossRef CAS.
- P. De Luna, R. Quintero-Bermudez, C. T. Dinh, M. B. Ross, O. S. Bushuyev, P. Todorovic, T. Regier, S. O. Kelley, P. Yang and E. H. Sargent, Catalyst electro-redeposition controls morphology and oxidation state for selective carbon dioxide reduction, Nat. Catal., 2018, 1, 103–110 CrossRef CAS.
- G. De Gregorio, T. Burdyny, A. Loiudice, P. Iyengar, W. A. Smith and R. Buonsanti, Facet-dependent selectivity of Cu catalysts in electrochemical CO2 reduction at commercially viable current densities, ACS Catal., 2020, 10, 4854–4862 CrossRef CAS PubMed.
- Y. Gao, Q. Wu, X. Liang, Z. Wang, Z. Zheng, P. Wang, Y. Liu, Y. Dai, M. H. Whangbo and B. Huang, Cu2O nanoparticles with both {100} and {111} facets for enhancing the selectivity and activity of CO2 electroreduction to ethylene, Adv. Sci., 2020, 7, 1902820 CrossRef CAS PubMed.
- Y. Zhou, F. Che, M. Liu, C. Zou, Z. Liang, P. De Luna, H. Yuan, J. Li, Z. Wang, H. Xie, H. Li, P. Chen, E. Bladt, R. Quintero-Bermudez, T. K. Sham, S. Bals, J. Hofkens, D. Sinton, G. Chen and E. H. Sargent, Dopant-induced electron localization drives CO2 reduction to C2 hydrocarbons, Nat. Chem., 2018, 10, 974–980 CrossRef CAS.
- T. T. Zhuang, Z. Q. Liang, A. Seifitokaldani, Y. Li, P. De Luna, T. Burdyny, F. Che, F. Meng, Y. Min, R. Quintero-Bermudez, C. T. Dinh, Y. Pang, M. Zhong, B. Zhang, J. Li, P.-N. Chen, X. L. Zheng, H. Liang, W. N. Ge, B. J. Ye, D. Sinton, S. H. Yu and E. H. Sargent, Steering post-C-C coupling selectivity enables high efficiency electroreduction of carbon dioxide to multi-carbon alcohols, Nat. Catal., 2018, 1, 421–428 CrossRef CAS.
- Y. Wang, Z. Wang, C. T. Dinh, J. Li, A. Ozden, M. G. Kibria, A. Seifitokaldani, C. S. Tan, C. M. Gabardo, M. Luo, H. Zhou, F. Li, Y. Lum, C. McCallum, Y. Xu, M. Liu, A. Proppe, A. Johnston, P. Todorovic, T. T. Zhuang, D. Sinton, S. O. Kelley and E. H. Sargent, Catalyst synthesis under CO2 electroreduction favours faceting and promotes renewable fuels electrosynthesis, Nat. Catal., 2020, 3, 98–106 CrossRef CAS.
- D. Zang, Q. Li, G. Dai, M. Zeng, Y. Huang and Y. Wei, Interface engineering of Mo8/Cu heterostructures toward highly selective electrochemical reduction of carbon dioxide into acetate, Appl. Catal., B, 2021, 281, 119426 CrossRef CAS.
- A. Loiudice, P. Lobaccaro, E. A. Kamali, T. Thao, B. H. Huang, J. W. Ager and R. Buonsanti, Tailoring copper nanocrystals towards C2 products in electrochemical CO2 reduction, Angew. Chem., Int. Ed., 2016, 55, 5789–5792 CrossRef CAS PubMed.
- X. Yuan, S. Chen, D. Cheng, L. Li, W. Zhu, D. Zhong, Z. J. Zhao, J. Li, T. Wang and J. Gong, Controllable Cu0-Cu+ sites for electrocatalytic reduction of carbon dioxide, Angew. Chem., Int. Ed., 2021, 60, 15344–15347 CrossRef CAS.
- J. Albo, G. Beobide, P. Castano and A. Irabien, Methanol Electrosynthesis from CO2 at Cu2O/ZnO prompted by pyridine-based aqueous solutions, J. CO2 Util., 2017, 18, 164–172 CrossRef CAS.
- W. Huang, Oxide nanocrystal model catalysts, Acc. Chem. Res., 2016, 49, 520–527 CrossRef CAS.
- K. Jiang, R. B. Sandberg, A. J. Akey, X. Liu, D. C. Bell, J. K. Nørskov, K. Chan and H. Wang, Metal ion cycling of Cu foil for selective C–C coupling in electrochemical CO2 reduction, Nat. Catal., 2018, 1, 111–119 CrossRef CAS.
- Z. Z. Wu, F. Y. Gao and M. R. Gao, Regulating the oxidation state of nanomaterials for electrocatalytic CO2 reduction, Energy Environ. Sci., 2021, 14, 1121–1139 RSC.
- C. H. Kuo, C. H. Chen and M. H. Huang, Seed-mediated synthesis of monodispersed Cu2O nanocubes with five different size ranges from 40 to 420 nm, Adv. Funct. Mater., 2007, 17, 3773–3780 CrossRef CAS.
- B. Liu, X. Yao, Z. Zhang, C. Li, J. Zhang, P. Wang, J. Zhao, Y. Guo, J. Sun and C. Zhao, Synthesis of Cu2O nanostructures with tunable crystal facets for electrochemical CO2 reduction to alcohols, ACS Appl. Mater. Interfaces, 2021, 13, 39165–39177 CrossRef CAS.
- B. Zhang, J. Zhang, M. Hua, Q. Wan and G. Mo, Highly electrocatalytic ethylene production from CO2 on nano-defective Cu nanosheets, J. Am. Chem. Soc., 2020, 142, 13606–13613 CrossRef CAS PubMed.
- F. F. Chang, J. C. Wei, Q. Zhang, Z. C. Jia, Y. P. Liu, L. Yang, X. L. Wang and Z. Y. Bai, Modulating the multiple intrinsic properties of platinum–iron alloy nanowires towards enhancing collaborative electrocatalysis, Mater. Chem. Front., 2021, 5, 8118–8126 RSC.
- F. F. Chang, Y. P. Liu, Q. Zhang, Z. C. Jia, X. L. Wang, L. Yang and Z. Y. Bai, Regulating the lattice strain of platinum–copper catalysts for enhancing collaborative electrocatalysis, Inorg. Chem. Front., 2022, 9, 249–258 RSC.
- W. Zhang, C. Huang, Q. Xiao, L. Yu, L. Shuai, P. An, J. Zhang, M. Qiu, Z. Ren and Y. Yu, Atypical oxygen-bearing copper boosts ethylene selectivity toward electrocatalytic CO2 reduction, J. Am. Chem. Soc., 2020, 142, 11417–11427 CrossRef CAS PubMed.
- J. P. Perdew, K. Burke and M. Ernzerhof, Generalized gradient approximation made simple, Phys. Rev. Lett., 1996, 77, 3865–3868 CrossRef CAS.
- A. A. Peterson, F. Abild-Pedersen, F. Studt, J. Rossmeisl and J. K. Norskov, How copper catalyzes the electroreduction of carbon dioxide into hydrocarbon fuels, Energy Environ. Sci., 2010, 3, 1311–1315 RSC.
- W. Bai, M. Wu, X. Du, W. Gong, Y. Ding, C. Song and L. Liu, Synergistic effect of multiple-phase rGO/CuO/Cu2O heterostructures for boosting photocatalytic activity and durability, Appl. Surf. Sci., 2021, 544, 148607 CrossRef CAS.
- P. D. Luna, R. Quintero-Bermudez, C. T. Dinh, M. B. Ross, O. S. Bushuyev, P. Todorovi, T. Regier, S. O. Kelley, P. Yang and E. H. Sargent, Catalyst electro-redeposition controls morphology and oxidation state for selective carbon dioxide reduction, Nat. Catal., 2018, 1, 103–110 CrossRef.
- T. Ghodselahi, M. A. Vesaghi, A. Shafiekhani, A. Baghizadeh and M. Lameii, XPS study of the Cu@Cu2O core-shell nanoparticles, Appl. Surf. Sci., 2008, 255, 2730–2734 CrossRef CAS.
- D. X. Tan, J. L. Zhang, X. Y. Cheng, X. N. Tan, J. B. Shi, B. X. Zhang, B. X. Han, L. R. Zheng and J. Zhang, CuxNiy alloy nanoparticles embedded in a nitrogen-carbon network for efficient conversion of carbon dioxide, Chem. Sci., 2019, 10, 4491–4496 RSC.
- C. M. Gabardo, A. Ozden, C. T. Dinh, J. Li, Y. Wang, J. P. Edwards, Y. Xu, C. McCallum, L. Tao, Z. Q. Liang, M. Luo, X. Wang, H. Li, C. P. O'Brien, C. S. Tan, D. H. R. Nam, R. Quintero-Bermudez, T.-T. Zhuang, Y. C. Li, Z. Han, R. D. Britt, D. Sinton, T. Agapie, J. C. Peters and E. H. Sargent, Molecular tuning of CO2-to-ethylene conversion, Nature, 2020, 577, 509–513 CrossRef.
- C. T. Dinh, T. Burdyny, M. G. Kibria, A. Seifitokaldani, C. M. Gabardo, F. P. García de Arquer, A. Kiani, J. P. Edwards, P. De Luna, O. S. Bushuyev, C. Zou, R. Quintero-Bermudez, Y. Pang, D. Sinton and E. H. Sargent, CO2 electroreduction to ethylene via hydroxide-mediated copper catalysis at an abrupt interface, Science, 2018, 360, 783–787 CrossRef CAS PubMed.
- Z. Liang, T. Zhuang, A. Seifitokaldani, J. Li, C. Huang, C. Tan, Y. Li, P. De Luna, C. T. Dinh, Y. Hu, Q. Xiao, P. Hsieh, Y. Wang, F. Li, R. Quintero-Bermudez, Y. Zhou, P. Chen, Y. Pang, S. Lo, L. J. Chen, H. Tan, Z. Xu, S. Zhao, D. Sinton and E. Sargent, Copper-on-nitride enhances the stable electrosynthesis of multi-carbon products from CO2, Nat. Commun., 2018, 9, 3828 CrossRef.
- A. Loiudice, P. Lobaccaro, E. A. Kamali, T. Thao, B. H. Huang, J. W. Ager and R. Buonsanti, Tailoring copper nanocrystals towards C2 products in electrochemical CO2 reduction, Angew. Chem., Int. Ed., 2016, 55, 5789–5792 CrossRef CAS.
- H. Jung, S. Y. Lee, C. W. Lee, M. K. Cho, D. H. Won, C. Kim, H. S. Oh, B. K. Min and Y. J. Hwang, Electrochemical fragmentation of Cu2O nanoparticles enhancing selective C-C coupling from CO2 reduction reaction, J. Am. Chem. Soc., 2019, 141, 4624–4633 CrossRef CAS.
- Y. Zhou, F. Che, M. Liu, C. Zou, Z. Liang, P. De Luna, H. Yuan, J. Li, Z. Wang, H. Xie, H. Li, P. Chen, E. Bladt, R. Quintero-Bermudez, T. Sham, S. Bals, J. Hofkens, D. Sinton, G. Chen and E. Sargent, Dopant-induced electron localization drives CO2 reduction to C2 hydrocarbons, Nat. Chem., 2018, 10, 974–980 CrossRef CAS PubMed.
- W. Ma, S. Xie, T. Liu, Q. Fan, J. Ye, F. Sun, Z. Jiang, Q. Zhang, J. Cheng and Y. Wang, Electrocatalytic reduction of CO2 to ethylene and ethanol through hydrogen-assisted C–C coupling over fluorine-modified copper, Nat. Catal., 2020, 3, 478–487 CrossRef CAS.
- C. S. Chen, J. H. Wan and B. S. Yeo, Electrochemical reduction of carbon dioxide to ethane using nanostructured Cu2O-derived copper catalyst and palladium(II) chloride, J. Phys. Chem. C, 2015, 119, 26875–26882 CrossRef CAS.
- M. Zhong, K. Tran, Y. Min, C. Wang, Z. Wang, C. T. Dinh, P. De Luna, Z. Yu, A. S. Rasouli, P. Brodersen, S. Sun, O. Voznyy, C. S. Tan, M. Askerka, F. Che, M. Liu, A. Seifitokaldani, Y. Pang, S. C. Lo, A. Ip, Z. Ulissi and E. H. Sargent, Accelerated discovery of CO2 electrocatalysts using active machine learning, Nature, 2020, 581, 178 CrossRef CAS.
- D. Wu, C. Dong, D. Wu, J. Fu, H. Liu, S. Hu, Z. Jiang, S. Qiao and X. Du, Cuprous ions embedded in ceria lattice for selective and stable electrochemical reduction of carbon dioxide to ethylene, J. Mater. Chem. A, 2018, 6, 9373–9377 RSC.
- D. Tan, J. Zhang, L. Yao, X. Tan, X. Cheng, Q. Wan, B. Han, L. Zheng and J. Zhang, Multi-shelled CuO microboxes for carbon dioxide reduction to ethylene, Nano Res., 2020, 13, 768–774 CrossRef CAS.
- C. Choi, S. Kwon, T. Cheng, M. Xu, P. Tieu, C. Lee, J. Cai, H. M. Lee, X. Pan, X. Duan, W. A. Goddard and Y. Huang, Highly active and stable stepped Cu surface for enhanced electrochemical CO2 reduction to C2H4, Nat. Catal., 2020, 3, 804–812 CrossRef CAS.
- J. Gong, D. Zhong, Z.-J. Zhao, Q. Zhao, D. Cheng and B. Liu, Coupling of Cu(100) and (110) facets promotes carbon dioxide conversion to hydrocarbons and alcohols, Angew. Chem., Int. Ed., 2021, 60, 4929–4935 Search PubMed.
- H. A. Hansen, J. B. Varley, A. A. Peterson and J. K. Nørskov, Understanding trends in the electrocatalytic activity of metals and enzymes for CO2 reduction to CO, J. Phys. Chem. Lett., 2013, 4, 388–392 CrossRef CAS PubMed.
- J. T. Feaster, C. Shi, E. R. Cave, T. Hatsukade, D. N. Abram, K. P. Kuhl, C. Hahn, J. K. Nørskov and T. F. Jaramillo, Understanding selectivity for the electrochemical reduction of carbon dioxide to formic acid and carbon monoxide on metal electrodes, ACS Catal., 2017, 7, 4822–4827 CrossRef CAS.
- J. Gao, H. Zhang, X. Guo, J. Luo, S. M. Zakeeruddin, D. Ren and M. Grätzel, Selective C−C coupling in carbon dioxide electroreduction via efficient spillover of intermediates as supported by operando raman spectroscopy, J. Am. Chem. Soc., 2019, 141, 18704–18714 CrossRef CAS PubMed.
- J. Applied Energy Gao, H. Zhang, X. Guo, J. Luo, S. M. Zakeeruddin, D. Ren and M. Grätzel, Selective C−C coupling in carbon dioxide electroreduction via efficient spillover of intermediates as supported by operando Raman spectroscopy, J. Am. Chem. Soc., 2019, 141, 18704–18714 CrossRef PubMed.
- M. B. Ross, C. T. Dinh, Y. Li, D. Kim, P. De Luna, E. H. Sargent and P. Yang, Tunable Cu enrichment enables designer syngas electrosynthesis from CO2, J. Am. Chem. Soc., 2017, 139, 9359–9363 CrossRef CAS.
- Y. Kim, S. Park, S. J. Shin, W. Choi, B. K. Min, H. Kim, W. Kim and Y. J. Hwang, Time-resolved observation of C−C coupling intermediates on Cu electrodes for selective electrochemical CO2 reduction, Energy Environ. Sci., 2020, 13, 4301–4311 RSC.
- Z. Z. Niu, F. Y. Gao, X. L. Zhang, P. P. Yang, R. Liu, L. P. Chi, Z. Z. Wu, S. Qin, X. Yu and M. R. Gao, Hierarchical copper with inherent hydrophobicity mitigates electrode flooding for high-rate CO2 electroreduction to multicarbon products, J. Am. Chem. Soc., 2021, 143, 8011–8021 CrossRef CAS.
- Y. Jiang, X. Wang, D. Duan, C. He, J. Ma, W. Zhang, H. Liu, R. Long, Z. Li, T. Kong, X. J. Loh, L. Song, E. Ye and Y. Xiong, Structural reconstruction of Cu2O superparticles toward electrocatalytic CO2 reduction with high C2+ products selectivity, Adv. Sci., 2022, 9, 2105292 CrossRef CAS.
|
This journal is © the Partner Organisations 2023 |