Brønsted acid-catalysed desilylative heterocyclisation to form substituted furans†
Received
6th October 2022
, Accepted 29th November 2022
First published on 30th November 2022
Abstract
Heterocyclisation of tert-butyldimethylsilyl (TBS) protected γ-hydroxy-α,β-unsaturated ketones catalysed by para-toluenesulfonic acid (p-TSA) to form substituted furans is reported. The reaction proceeds under mild conditions at room temperature in methanol to give a range of furan products (21 examples, up to 98% yield). Mechanistic experiments suggest the reaction proceeds via in situ deprotection followed by catalytic dehydrative heterocyclisation.
Introduction
Small heterocyclic ring systems are ubiquitous molecular scaffolds within synthetic chemistry. For example, substituted furans are found within natural products and synthetic biologically active compounds, and they also have many uses as reaction substrates and/or intermediates. Consequently, various protocols have been developed for their preparation from a wide array of starting materials.1 Advances in the practical synthesis of heterocycles, including substituted furans, under mild and sustainable conditions from readily available substrates is essential for continued development in a range of fields, including the pharmaceutical and agrochemical industries.2
The Nazarov cyclisation is an established strategy for the synthesis of cyclopentenones from divinyl ketones. Activation with either a Lewis or Brønsted acid forms an intermediate pentadienyl cation, which undergoes 4π-electrocyclisation to construct the cyclopentyl ring. Modern variations of this process have found different ways of accessing the key pentadienyl cation intermediate,3 including through dehydration of divinyl alcohols to form substituted cyclopentadienes (Scheme 1a).4 However, this strategy has rarely been extended to the synthesis of five-membered heterocycles. Würthwein and co-workers reported that α-hydroxy-β,γ-unsaturated oximes undergo dehydration in the presence of stoichiometric triflic acid to form 1-azapentadienyl cations, with subsequent electrocyclisation giving trisubstituted pyrroles.5 Das and co-workers found that highly functionalised Baylis–Hillman adducts also undergo dehydrative heterocyclisation promoted by stoichiometric methanesulfonic acid to form functionalised benzofuran derivatives.6 Subsequently, Krische and co-workers showed that β,γ-unsaturated α-hydroxyketones, formed from ruthenium-catalysed hydrohydroxyalkylation of alkynes with diols, undergo dehydrative heterocyclisation in the presence of catalytic para-toluenesulfonic acid (p-TSA) to form a range of tetrasubstituted furans (Scheme 1b).7 There are also a few sporadic reports of isomeric γ-hydroxy-α,β-unsaturated ketones and their derivatives undergoing Brønsted acid-promoted dehydrative cyclisation.8 Similarly, γ-hydroxy-α,β-unsaturated ketones formed through in situ Knoevenagel condensation of 1,3-diketones and α-hydroxyaldehydes undergo facile cyclisation and elimination to form highly substituted furans, a process that is used in the valorisation of aldose sugars (known as the Garcia-Gonzalez reaction).9
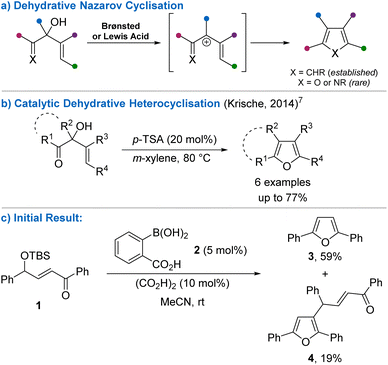 |
| Scheme 1 Catalytic heterocyclisation reactions. | |
Building upon our interest in the dehydrative substitution of alcohols using arylboronic acids as Brønsted acid catalysts,10,11 we were interested in investigating such systems as mild, catalytic methods of generating 1-oxapentadienyl cations that would undergo 4π-electrocyclisation to give substituted furans. To investigate this, tert-butyldimethylsilyl (TBS) protected γ-hydroxy-α,β-unsaturated ketone 1 was prepared as a precursor to the desired alcohol substrate. However, silyl ether 1 did not undergo smooth deprotection using tetrabutylammonium fluoride (TBAF), and resulted in either unexpected oxidation of the enone when performed under air, or deprotection followed by tautomerisation into the corresponding 1,4-diketone under an inert atmosphere.12 As TBS ethers are also known to undergo acidic deprotection, it was reasoned that a one-pot deprotection-heterocyclisation process may be possible to form the furan directly from 1. For example, Sammond and Sammakia reported that TBS protected γ-hydroxy-α,β-unsaturated ketones similar to 1 undergo deprotection/cyclisation in the presence of stoichiometric triethylamine trihydrofluoride.13a A furan side product was observed in the synthesis of sacrolide A when a more complex unsaturated system was reacted with HF pyridine complex,13b while single examples of similar furan formations have been reported using p-TSA13c and H2SO4.13d To investigate whether this process could be rendered catalytic under mild reaction conditions, 1 was treated with 2-carboxybenzeneboronic acid 2 (5 mol%) and oxalic acid (10 mol%) in acetonitrile at room temperature (Scheme 1c). Pleasingly, furan 3 was obtained in 59% yield, alongside a side product that was isolated and characterised as substituted furan 4. Arylboronic acids are known to catalyse dehydrative Friedel–Crafts alkylation reactions of electron-rich (hetero)arenes, accounting for the formation of 4.14 Given the promising initial result for the one-pot deprotection-heterocyclisation, we decided to optimise this protocol with the aim of developing milder conditions compared to the previous reports and fully exploring the reaction scope and limitations.
Results and discussion
First, the reaction of 1 was further optimised through variation of key reaction parameters (Table 1).12 An initial solvent screen revealed that the reaction also occurred in methanol forming furan 3 in a modest 17% yield by NMR analysis but, importantly, in the absence of side product 4 and the remainder of the material was unreacted 1 (entry 1). Next, a range of alternative Brønsted acid catalysts (5 mol%) were screened in methanol at room temperature. Acetic acid, oxalic acid, and trifluoroacetic acid (entry 2) showed little reactivity but strongly acidic methanolic HCl gave a promising 45% conversion to furan 3 with no formation of side product 4 (entry 3). The use of para-toluenesulfonic acid (p-TSA·H2O) resulted in a further improvement, providing a clean 63% conversion into furan 3 (entry 4). Increasing the catalyst loading to 10 mol% and the time to 18 hours gave complete conversion of 1, allowing furan 3 to be isolated in 76% yield (entry 5). The use of substrates bearing bulkier silyl protecting groups such as triisopropylsilyl (TIPS) 5 and tert-butyldiphenylsilyl (TBDPS) 6 gave reduced yields (entries 6 and 7),15 while in the absence of a Brønsted acid catalyst no conversion of starting material 1 was observed (entry 8). The scope and limitations of the catalytic heterocyclisation were then explored under the optimised conditions (Scheme 2).
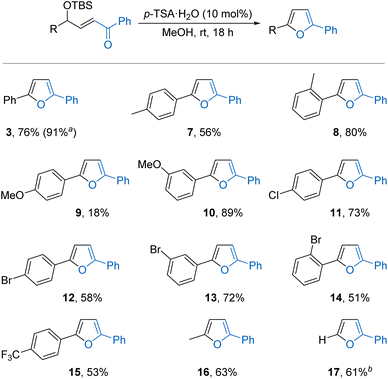 |
| Scheme 2 Scope and limitations. Reactions performed on a 0.2 mmol scale in MeOH (1 M). a Performed on 5.7 mmol scale. b Performed on a 0.4 mmol scale in MeOH (0.1 M). | |
Table 1 Reaction optimisation
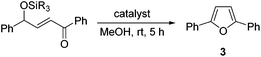
|
Entry |
SiR3 |
Catalyst (mol%) |
Yielda (%) |
Determined by 1H NMR using 1,4-dinitrobenzene as an internal standard.
18 hours.
Isolated yield.
|
1 |
SiMe2t-Bu 1 |
2 (5) + (CO2H)2 (10) |
17 |
2 |
SiMe2t-Bu 1 |
TFA (5) |
<5 |
3 |
SiMe2t-Bu 1 |
HCl (5) |
45 |
4 |
SiMe2t-Bu 1 |
p-TSA·H2O (5) |
63 |
5b |
SiMe2t-Bu 1 |
p-TSA·H2O (10) |
99 (76)c |
6 |
Si(i-Pr)35 |
p-TSA·H2O (5) |
50 |
7 |
SiPh2t-Bu 6 |
p-TSA·H2O (5) |
10 |
8 |
SiMe2t-Bu 1 |
None |
0 |
The synthetic potential was initially demonstrated by performing the reaction on gram scale (5.7 mmol of 1) to give 1.13 g of furan 3 in 91% yield. Next, a series of TBS protected γ-hydroxy-α,β-unsaturated ketones was conveniently prepared through a Wittig reaction between the corresponding TBS protected α-hydroxy aldehyde and an acyl phosphorane.12,16 First, various aryl substituents on the aldehyde component were trialled. Mildly electron-donating 2- and 4-methyl substituents were tolerated, forming products 7 and 8 in good yields. However, a strongly electron-donating 4-methoxy group was highly reactive, giving only 18% of furan 9 alongside a complex mixture of side-products. It may be that the increased stability of a possible cationic intermediate formed in this case facilitates unwanted reactivity, including solvolysis and Friedel–Crafts alkylations.10a,14 Moving the methoxy group to the 3-position where it is formally electron-withdrawing to the benzylic position was more successful,17 forming furan 10 in 89% yield. Aryl rings bearing halogen substituents in either the 2-, 3-, or 4-positions were well tolerated, forming products 11–14 in good yields. A strongly electron-withdrawing 4-trifluoromethyl group was also tolerated to give 15 in 53% yield. Alkyl substitution on this portion of the starting material was also possible, with 2-methyl-5-phenylfuran 16 formed in a good 63% yield. Singly substituted 2-phenylfuran 17 underwent side reactions at the standard 1 M substrate concentration in methanol but could be effectively isolated in 61% yield from a more dilute 0.1 M reaction.
The acyl phosphorane component was then varied using 2-silyloxy-2-phenlacetaldehyde as the standard aldehyde (Scheme 3). In contrast to aryl substitution in the γ-position, electron-rich aryl rings were much better tolerated in the α′-position with 4-methyl and 4-methoxy substituted furans 7 and 9 isolated in 80% and 97% yield, respectively. The use of 3-methoxy and 4-benzyloxy substituted rings was also successful, giving products 10 and 18 in excellent yields. In these cases, the electron-donating aryl substituents are not conjugated with the allylic cation intermediate and therefore have less of an influence on the outcome of the cyclisation compared with substitution in the γ-position. Strongly electron-withdrawing aryl substituents including 4-trifluoromethyl, 4-nitro, and 4-cyano, were also better tolerated in the α′-position compared with the γ-position, with furans 15, 19, and 20 formed in reasonable yields. Alkyl substitution was again possible, with 2-methyl and 2-cyclopropyl substituted furans 16 and 21 isolated in 71% and 80% yields, respectively. Attempts to form tri-substituted furans through α-substitution of the enone were unsuccessful as the substrates could not be prepared.
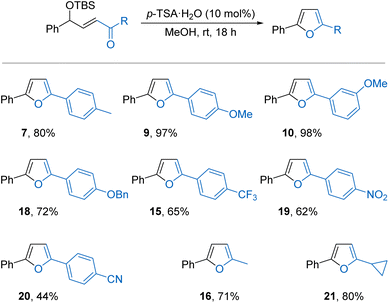 |
| Scheme 3 Scope and limitations. Reactions performed on a 0.2 mmol scale in MeOH (1 M). | |
Next, a series of control experiments were performed to gain further mechanistic understanding of the cyclisation process (Scheme 4). The likely silyl deprotection of the starting material was initially studied using model TBS protected allylic alcohol 22 that is incapable of undergoing heterocyclisation. Reacting 22 under the standard conditions in MeOD-d4 and monitoring over time by 1H NMR using 1,4-dinitrobenzene as an internal standard showed rapid silyl deprotection into the corresponding allylic alcohol, which underwent solvolysis over time to form a mixture of branched and linear methyl ethers 23 and 24 (Scheme 4a). To investigate the possibility of solvolysis occurring in the heterocyclisation process, methyl ether 25 was used as a substrate (Scheme 4b). No furan formation occurred after 18 hours; however, a small amount of conjugate addition product 26 was observed as a 59
:
41 mixture of diastereoisomers with the remainder unreacted starting material. This is surprising, as methyl ether 25 would likely have similar ionisation potential compared with the corresponding allylic alcohol yet is not a viable intermediate, but this experiment does show that heterocyclisation outcompetes solvolysis under the reaction conditions. However, the analogous methyl ether bearing a γ-4-methoxyphenyl substituent did form the expected furan 9 under the standard conditions, alongside the conjugate addition products.12 These results therefore suggest that different reaction mechanisms may be in operation depending on the substituents present. The reaction of TBS γ-hydroxy-enone 1 was then studied by in situ1H NMR in MeOD-d4 (Scheme 4c). As with model substrate 22, TBS protected 1 was rapidly consumed, with deprotected alcohol 27 initially building-up in solution before its concentration decreased in line with formation of furan 3. No other significant reaction intermediates were observed, while further time points could not be taken as furan 3 precipitates from solution at higher concentrations. Isolated γ-hydroxy-enone 27 was demonstrated to be a competent starting material, with furan 3 formed in 76% yield under the standard conditions.11 The possibility of in situ isomerisation of alcohol 27 into the corresponding 1,4-diktone followed by Paal–Knorr furan formation was discounted, as the isolated 1,4-diketone does not react with p-TSA (10 mol%) in methanol. The reaction of 1 was also successful in the dark, forming furan 3 in 71% yield. This excludes possible photochemical alkene isomerisation and cyclisation as recently reported by Donohoe and co-workers for a set of related γ-hydroxy-α,β-unsaturated ketone substrates.18 Isomeric TBS α-hydroxy-β,γ-unsaturated ketone 28 was also trialled in the heterocyclisation reaction. The more sterically demanding tertiary substrate did not cyclise at room temperature, with starting material 28 returned alongside 15% of the γ-solvolysis product.12 However, heating the reaction to 70 °C gave trisubstituted furan 29 in 76% yield (Scheme 4d). This provides support for the formation of an allylic cation intermediate that can be accessed irrespective of the isomer of allylic alcohol used.
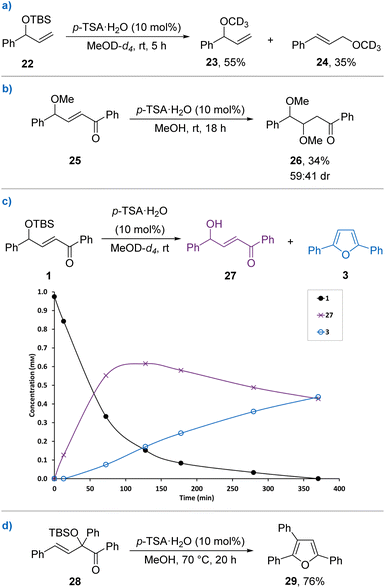 |
| Scheme 4 Control experiments. | |
The available evidence cannot unambiguously determine the mechanism for heterocyclisation and suggests that more than one pathway may occur depending on the substrate (Scheme 5). The in situ NMR shows that acid-promoted deprotection of TBS γ-hydroxy-α,β-unsaturated ketone 30 is facile and forms alcohol 31. The alcohol may then be protonated and ionised to form allylic cation (E)-32, which must be isomerised into (Z)-32 before undergoing rapid 4π-electrocyclisation followed by deprotonation to give the furan product 33.19 Alternatively, alcohol 31 may undergo acid-catalysed conjugate addition of methanol to form 34, which can cyclise to form hemiacetal 35.20 Successive acid-promoted eliminations of water and methanol would lead to furan 33.
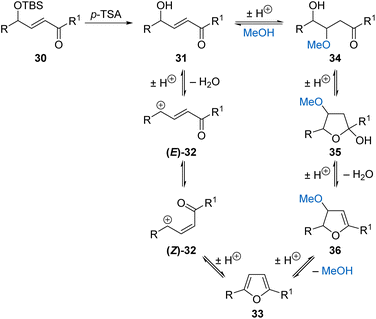 |
| Scheme 5 Plausible mechanistic pathways. | |
Conclusions
In conclusion, p-TSA (10 mol%) is an efficient catalyst for the heterocyclisation of TBS protected γ-hydroxy-α,β-unsaturated ketones to form substituted furans under mild conditions in methanol at room temperature. The process is thought to occur via in situ deprotection, followed by acid-promoted heterocyclisation with elimination of one equivalent of water. Further work on the catalytic activation of allylic alcohols for heterocycle formation is underway in our laboratory.21
Experimental
General procedure for catalytic heterocyclisation
The requisite TBS-protected γ-hydroxy-α,β-unsaturated ketone was dissolved in MeOH (1 M) and p-TSA·H2O (10 mol%) was added. The reaction was stirred at rt for 18 h before being concentrated under reduced pressure. The crude material was purified by silica gel column chromatography (100% to 97/3 cyclohexane/Et2O).
Author contributions
E. G. B. and M. S. R. performed all experimental work and collected the data. J. E. T. conceptualised the idea, supervised, and coordinated the project. E. G. B. and J. E. T. prepared the manuscript and ESI.†
Conflicts of interest
There are no conflicts to declare.
Acknowledgements
The authors thank the University of Bath (studentship to E. G. B) and the EPSRC (Grant code: EP/V051423/1) for funding. We thank a reviewer for insightful comments regarding the alternative reaction mechanisms.
References
- For selected recent reviews on the preparation of furans, see:
(a) A. V. Gulevich, A. S. Dudnik, N. Chernyak and V. Gevorgyan, Transition metal-mediated synthesis of monocyclic aromatic heterocycles, Chem. Rev., 2013, 113, 3084–3213 CrossRef CAS
;
(b) S. Ponra and K. C. Majumdar, Brønsted acid-promoted synthesis of common heterocycles and related bio-active and functional molecules, RSC Adv., 2016, 6, 37784–37922 RSC
;
(c) A. Deepthi, B. P. Babu and A. L. Balachandran, Synthesis of Furans – Recent Advances, Org. Prep. Proced. Int., 2019, 51, 409–442 CrossRef CAS
.
-
(a) R. D. Taylor, M. MacCoss and A. D. G. Lawson, Rings in drugs, J. Med. Chem., 2014, 57, 5845–5859 CrossRef CAS PubMed
;
(b) A. P. Taylor, R. P. Robinson, Y. M. Fobian, D. C. Blakemore, L. H. Jones and O. Fadeyi, Modern advances in heterocyclic chemistry in drug discovery, Org. Biomol. Chem., 2016, 14, 6611–6637 RSC
.
-
(a) W. T. Spencer III, T. Vaidya and A. J. Frontier, Beyond the divinyl ketone: innovations in the generation and Nazarov cyclization of pentadienyl cation intermediates, Eur. J. Org. Chem., 2013, 3621–3633 CrossRef
;
(b) M. J. Di Grandi, Nazarov-like cyclization reactions, Org. Biomol. Chem., 2014, 12, 5331–5345 RSC
;
(c) M. G. Vinogradov, O. V. Turova and S. G. Zlotin, Nazarov reaction: current trends and recent advances in the synthesis of natural compounds and their analogs, Org. Biomol. Chem., 2017, 15, 8245–8269 RSC
.
- For selected recent examples, see:
(a) H. Zheng, M. Lejkowski and D. G. Hall, Mild boronic acid catalyzed Nazarov cyclization of divinyl alcohols in tandem with Diels–Alder cycloaddition, Tetrahedron Lett., 2013, 54, 91–94 CrossRef CAS
;
(b) M. Sai and S. Matsubara, Lithium(1+)-catalyzed Nazarov-type cyclization of 1-arylbuta-2,3-dien-1-ols: synthesis of benzofulvene derivatives, Synlett, 2014, 25, 2067–2071 CrossRef CAS
;
(c) B. N. Kakde, A. Parida, N. Kumar, A. Mourya and A. Bisai, FeCl3-Catalyzed highly efficient Nazarov type cyclization of arylvinylcarbinols: Total synthesis of (±)-dichroanal B and related taiwaniaquinoids, ChemistrySelect, 2016, 1, 3357–3362 CrossRef CAS
;
(d) Z. Wang, X. Xu, Z. Gu, W. Feng, H. Qian, Z. Li, X. Sun and O. Kwon, Nazarov cyclization of 1,4-pentadien-3-ols: preparation of cyclopenta[b]indoles and spiro[indene-1,4′-quinoline]s, Chem. Commun., 2016, 52, 2811–2814 RSC
;
(e) M. C. Martin, M. J. Sandridge, C. W. Williams, Z. A. Francis and S. France, Dehydrative Nazarov-type electrocyclizations of alkenyl (hetero)aryl carbinols via calcium catalysis: Access to cyclopenta[b]thiophenes and indene derivatives, Tetrahedron, 2017, 73, 4093–4108 CrossRef CAS
;
(f) J. Jin, Y. Zhao, A. Gouranourimi, A. Ariafard and P. W. H. Chan, Chiral Brønsted acid catalyzed enantioselective dehydrative Nazarov-type electrocyclization of aryl and 2-thienyl vinyl alcohols, J. Am. Chem. Soc., 2018, 140, 5834–5841 CrossRef CAS PubMed
;
(g) M. Chakraborty, G. Mahesh, O. R. Nakel, G. Chavda, S. Anusha and G. Sudhakar, A Facile Approach to access multi-substituted indenes via Nazarov cyclisation of aryl, vinyl, and alkyl/aryl carbinols, ChemistrySelect, 2021, 6, 13842–13850 CrossRef CAS
.
-
(a) D. Alickmann, R. Fröhlich, A. H. Maulitz and E.-U. Würthwein, Electrocyclization reactions of 1-aza- and 1-oxapentadienyl and -heptatrienyl cations: synthesis of pyrrole and furan derivatives, Eur. J. Org. Chem., 2002, 1523–1537 CrossRef CAS
;
(b) R. Narayan, R. Fröhlich and E.-U. Würthwein, Synthesis of pyrroles through a 4π-electrocyclic ring-closure reaction of 1-azapentadienyl cations, J. Org. Chem., 2012, 77, 1868–1879 CrossRef CAS PubMed
.
- D. Basavaiah, S. Roy and U. Das, Toward understanding the scope of Baylis–Hillman reaction: synthesis of 3-(2-hydroxyphenyl)indolin-2-ones and polycyclic fused furans, Tetrahedron, 2010, 66, 5612–5622 CrossRef CAS
.
- E. L. McInturff, K. D. Nguyen and M. J. Krische, Redox-triggered C-C coupling of diols and alkynes: synthesis of β,γ-unsaturated α-hydroxyketones and furans by ruthenium-catalyzed hydrohydroxyalkylation, Angew. Chem., Int. Ed., 2014, 53, 3232–3235 CrossRef CAS PubMed
.
- For selected examples, see:
(a) S. W. Baldwin and M. T. Crimmins, Synthesis of the alleged genipic acid by photoannelation, J. Am. Chem. Soc., 1980, 102, 1198–1200 CrossRef CAS
;
(b) J. Nokami, A. Nishimura, M. Sunami and S. Wakabayashi, Facile formation of 4-hydroxyalk-2-enone functionality, Tetrahedron Lett., 1987, 28, 649–650 CrossRef CAS
;
(c) R. Iriye, T. Uno, I. Ohwa and A. Konishi, The formation of alkylfurans from (E,)-4-hydroxy-2-alkenals and (E)-4-oxo-2-alkenols, and a synthesis of rosefuran, Agric. Biol. Chem., 1990, 54, 1841–1843 CAS
;
(d) L. P. Ho, K. H. Sun and K. Sunggak, Efficient transformation of α,β-enone to substituted furans via phosphoniosilylation, Chem. Lett., 1994, 23, 2401–2402 CrossRef
;
(e) R. Díaz-Cortés, A. L. Silva and L. A. Maldonado, A simple approach to 2-substituted-4-furanmethanol compounds, Tetrahedron Lett., 1997, 38, 2207–2210 CrossRef
;
(f) T. D. Avery, D. K. Taylor and E. R. T. Tiekink, A New route to diastereomerically pure cyclopropanes utilizing stabilized phosphorus ylides and γ-hydroxy enones derived from 1,2-dioxines: Mechanistic investigations and scope of reaction, J. Org. Chem., 2000, 65, 5531–5546 CrossRef CAS PubMed
;
(g) M. Friedrich, A. Wächtler and A. de Meijere, Extending the scope of a known furan synthesis - A novel route to 1,2,4-trisubstituted pyrroles, Synlett, 2002, 0619–0621 CrossRef CAS
;
(h) A. Boto, D. Hernández and R. Hernández, Short and efficient synthesis of chiral furyl carbinols from carbohydrates, Org. Lett., 2007, 9, 1721–1724 CrossRef CAS PubMed
;
(i) P. Jakubec, D. M. Cockfield and D. J. Dixon, Total synthesis of (−)-nakadomarin A, J. Am. Chem. Soc., 2009, 131, 16632–16633 CrossRef CAS PubMed
;
(j) W. Yan, Z. Li and Y. Kishi, Selective activation/coupling of polyhalogenated nucleophiles in Ni/Cr-mediated reactions: Synthesis of C1−C19 building block of Halichondrin Bs, J. Am. Chem. Soc., 2015, 137, 6219–6225 CrossRef CAS PubMed
.
- For selected recent examples, see:
(a) N. Ronaghi, D. M. Fialho, C. W. Jones and S. France, Conversion of unprotected aldose sugars to polyhydroxyalkyl and C-glycosyl furans via zirconium catalysis, J. Org. Chem., 2020, 85, 15337–15346 CrossRef CAS
;
(b) N. Ronaghi, D. Shade, H. J. Moon, S. Najmi, J. W. Cleveland, K. S. Walton, S. France and C. W. Jones, Modulation and tuning of UiO-66 for Lewis acid catalyzed carbohydrate conversion: Conversion of unprotected aldose sugars to polyhydroxyalkyl and C-glycosyl furans, ACS Sustainable Chem. Eng., 2021, 9, 11581–11595 CrossRef CAS
;
(c) R. Zhang, A. Eronen, X. Du, E. Ma, M. Guo, K. Moslova and T. Repo, A catalytic approach via retro-aldol condensation of glucose to furanic compounds, Green Chem., 2021, 23, 5481–5486 RSC
;
(d) R. Zhang, A. Eronen, P. Vasko, X. Du, J. Install and T. Repo, Near quantitative conversion of xylose into bisfuran, Green Chem., 2022, 24, 5052–5057 RSC
.
-
(a) S. Estopiñá-Durán, L. J. Donnelly, E. B. Mclean, B. M. Hockin, A. M. Z. Slawin and J. E. Taylor, Aryl boronic acid catalysed dehydrative substitution of benzylic alcohols for C−O bond formation, Chem. – Eur. J., 2019, 25, 3950–3956 CrossRef PubMed
;
(b) S. Estopiñá-Durán, E. B. McLean, L. J. Donnelly, B. M. Hockin and J. E. Taylor, Arylboronic acid catalyzed C-alkylation and allylation reactions using benzylic alcohols, Org. Lett., 2020, 22, 7547–7551 CrossRef PubMed
.
- S. Estopiñá-Durán and J. E. Taylor, Brønsted acid-catalysed dehydrative substitution reactions of alcohols, Chem. – Eur. J., 2021, 27, 106–120 CrossRef PubMed
.
- See the ESI for details.†.
-
(a) D. M. Sammond and T. Sammakia, A mild synthesis of substituted furans from γ-hydroxy-α,β-unsaturated ketones, Tetrahedron Lett., 1996, 37, 6065–6068 CrossRef CAS
;
(b) T. Mohri, Y. Ogura, R. Towada and S. Kuwahara, Enantioselective total synthesis of sacrolide A, Tetrahedron Lett., 2017, 58, 4011–4013 CrossRef CAS
;
(c) J. S. Clark and C. Xu, Total synthesis of (–)-nakadomarin A, Angew. Chem., Int. Ed., 2016, 55, 4332–4335 CrossRef CAS PubMed
;
(d) T. Zhang and H. Maekawa, Synthesis of 4-(trifluoromethyl)cyclopentenones and 2-(trifluoromethyl)furans by reductive trifluoroacetylation of ynones, Org. Lett., 2017, 19, 6602–6605 CrossRef CAS PubMed
.
-
(a) J. A. McCubbin, H. Hosseini and O. V. Krokhin, Boronic acid catalyzed Friedel−Crafts reactions of allylic alcohols with electron-rich arenes and heteroarenes, J. Org. Chem., 2010, 75, 959–962 CrossRef CAS PubMed
;
(b) J. A. McCubbin and O. V. Krokhin, Organocatalyzed Friedel–Crafts arylation of benzylic alcohols, Tetrahedron Lett., 2010, 51, 2447–2449 CrossRef CAS
;
(c) X. Mo, J. Yakiwchuk, J. Dansereau, J. A. McCubbin and D. G. Hall, Unsymmetrical diarylmethanes by ferroceniumboronic acid catalyzed direct Friedel–Crafts reactions with deactivated benzylic alcohols: Enhanced reactivity due to ion-pairing effects, J. Am. Chem. Soc., 2015, 137, 9694–9703 CrossRef CAS PubMed
;
(d) E. Wolf, E. Richmond and J. Moran, Identifying lead hits in catalyst discovery by screening and deconvoluting complex mixtures of catalyst components, Chem. Sci., 2015, 6, 2501–2505 RSC
.
- Analogous substrates bearing less sterically demanding trimethylsilyl (TMS) and triethylsilyl (TES) protecting groups could not be prepared due to the instability of the substrates and their precursors.
- Alternative synthetic routes were trialled, including aldol condensation/elimination protocols, but these often gave complex mixtures of products. The Wittig route proved overall most reliable across a range of substrates.
- C. Hansch, A. Leo and R. W. Taft, A survey of Hammett substituent constants and resonance and field parameters, Chem. Rev., 1991, 91, 165–195 CrossRef CAS
.
- J. C. L. Walker, S. Werrel and T. J. Donohoe, Photochemical alkene isomerization for the synthesis of polysubstituted furans and pyrroles under neutral conditions, Chem. – Eur. J., 2019, 25, 13114–13118 CrossRef CAS PubMed
.
- Bond rotations in the model allyl cation have been studied computationally in the gas phase, which suggest the energy barrier is high; however, in solution the rotational barrier may be significantly lower.
(a) H. Mayr, W. Foerner and P. V. R. Schleyer, Methyl-substituted allyl cations. A comparison of experimental stability, rotational barrier, and solvolysis data with ab initio calculations, J. Am. Chem. Soc., 1979, 101, 6032–6040 CrossRef CAS
;
(b) Z. Li, T. Bally, K. N. Houk and W. T. Borden, Variations in rotational barriers of allyl and benzyl cations, anions, and radicals, J. Org. Chem., 2016, 81, 9576–9584 CrossRef CAS PubMed
.
- S. Sato, I. Matsuda and Y. Izumi, Aldol-type reactions between trimethylsilyl enol ethers and acetals with the aid of rhodium complex, Tetrahedron Lett., 1987, 28, 6657–6660 CrossRef CAS
.
- Research data supporting publication can be accessed at https://researchdata.bath.ac.uk/id/eprint/1178.
Footnote |
† Electronic supplementary information (ESI) available: Experimental procedures, characterization data, and NMR spectra for novel compounds (PDF). See DOI: https://doi.org/10.1039/d2ob01828d |
|
This journal is © The Royal Society of Chemistry 2023 |