Redox-neutral rhodium(III)-catalyzed divergent synthesis of tetrasubstituted 1,3-enynes and alkynylated benzofurans†
Received
3rd October 2022
, Accepted 25th November 2022
First published on 28th November 2022
Abstract
With the assistance of the acetamido directing group (DG), a rhodium-catalyzed C–H alkenylation/DG migration cascade for the synthesis of tetrasubstituted 1,3-enynes from N-phenoxyacetamides and 1,3-diynes has been achieved in this work. Alternatively, a rhodium-catalyzed [3 + 2] annulation for the synthesis of alkynylated benzofurans from the same set of substrates has also been achieved by simply changing the reaction conditions. This work highlights the tunable divergent synthesis of valuable compounds triggered by C–H activation.
Introduction
Transition-metal (TM)-catalyzed C–H functionalization assisted by directing groups (DGs) has become a powerful tool for the efficient and straightforward construction of carbon–carbon/heteroatom bonds in recent decades.1 Initially, DGs are designed as auxiliary groups, which coordinate with TMs and then help to activate the ortho-C–H bond to give an active nucleophilic metallacycle that couples with diverse coupling partners (CPs) (Scheme 1a).2 Thus, DGs usually stay at their original positions when reactions complete in most cases or further undergo intramolecular annulation in situ in some cases.3 However, it should be noted that the chemical traces of DGs may not be desired in the products and may block further transformations, and extra steps are often needed to disconnect them, thus leading to poor atom- and step-economy. Apparently, further synthetic applications of DGs would be provided if they could play more roles than auxiliary groups such as migrating functional reagents if they could migrate onto the CPs after the step of C–H functionalization, thus achieving excellent step- and atom-economy and simultaneously increasing the diversity of the products. It is quite fascinating but also challenging to achieve the migration of DGs, mainly because of the challenge in identifying suitable substrates carrying a matching DG which has a dual role of an auxiliary group and a migrating functional reagent. Nevertheless, the synthetic community has made their pioneering efforts in developing C–H functionalization/DG migration cascades.4 In particular, the C–H alkenylation/DG migration with alkynes via Co(III),5 Ru(II),6 Rh(III)7 and Ir(III)8 catalysis has been well studied for the synthesis of tetrasubstituted alkenes (Scheme 1b). By contrast, the C–H alkenylation/DG migration with 1,3-diynes, which carry two adjacent carbon–carbon triple bonds, has been seldom explored (Scheme 1c). This is mainly because of the inherent challenges of achieving high levels of chemo-, regio- and stereoselectivity in the step of migratory insertion of 1,3-diynes into nucleophilic metallacycles, and the selectivity between mono- and difunctionalization of two alkyne moieties.9 To the best of our knowledge, only two examples involving C–H alkenylation/DG migration with 1,3-diynes have been reported to date. Our group firstly disclosed a rhodium-catalyzed C–H alkenylation/carbamoyl DG migration between N-carbamoyl indoles and 1,3-diynes very recently.10a Soon afterwards, the group of Kanchupalli reported a similar cascade but focused on aromatic substituted 1,3-diynes.10b Based on our experience in functional group migration11 and interest in C–H functionalization,12 and inspired by the emerging strategy of DG migration,4 herein we reveal a C–H alkenylation/acetamido DG migration between N-phenoxyacetamides13 and 1,3-diynes for the synthesis of more challenging tetrasubstituted 1,3-enynes with the catalytic system of [Cp*RhCl2]2/Zn(OAc)2/MeOH (Scheme 1d). Of note, the acetamido DG not only works as an auxiliary group, but also acts as an internal amidation reagent that migrates onto the alkene unit of the products after the step of C–H alkenylation. Very interestingly, a [3 + 2] annulation for the assembly of C2-alkynylated benzofurans occurs instead from the same set of substrates with the catalytic system of [Cp*RhCl2]2/NaOPiv·H2O/CH2Cl2. Considering the large presence of the 1,3-enyne and benzofuran scaffolds in bioactive molecules (Fig. 1),14 our method is quite appealing as it allows the tunable divergent synthesis of challenging tetrasubstituted 1,3-enynes and C2-alkynylated benzofurans via rhodium catalysis by simply switching the reaction conditions. This protocol features complexity- and diversity-generation and tunable product selectivity.
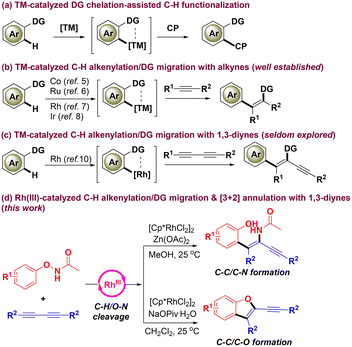 |
| Scheme 1 TM-catalyzed C–H alkenylation/DG migration with alkynes or 1,3-diynes. | |
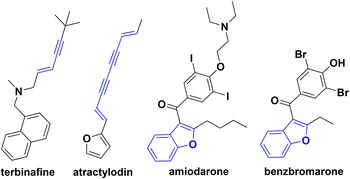 |
| Fig. 1 Representative bioactive molecules carrying the 1,3-enyne or benzofuran scaffolds. | |
Results and discussion
N-Phenoxyacetamide 1aa and deca-4,6-diyne 2aa were used as model substrates to optimize the reaction conditions (Table 1).
Table 1 Optimization of the reaction conditionsa
At first, substrates 1aa and 2aa were treated with various metal catalysts in MeOH at 25 °C for 24 h employing CsOAc as the additive (entries 1–5). To our delight, [Cp*RhCl2]2 could catalyze the C–H alkenylation/DG migration highly regio- and stereoselectively (entry 5), providing the cis-adduct 3aa with the phenyl moiety exclusively located at the less hindered position as the only isomer in a good yield (78%). Then, with [Cp*RhCl2]2 and CsOAc as the catalyst and additive, respectively, diverse solvents were screened. Very interestingly, the C–H alkenylation/DG migration product 3aa was found to be preferred in polar solvents such as CH3CN, MeOH, EtOH and DMF, while the [3 + 2] annulation product 4aa was favoured instead in nonpolar or medium polar solvents such as toluene, CH2Cl2, DCE, THF, acetone and 1,4-dioxane (entries 6–10).15 Of note, MeOH was proved to be the best choice for the exclusive production of 3aa with 78% yield, while CH2Cl2 was found to be the most suitable solvent for the selective preparation of 4aa with 40% yield. Subsequently, a series of additives were screened in MeOH to further improve the yield of product 3aa (entries 11–17), and Zn(OAc)2 turned out to be the best additive, with which product 3aa was isolated in 86% yield exclusively (entry 13).16 Similarly, an investigation of various additives in CH2Cl2 (entries 18–23) revealed that NaOPiv·H2O was the optimal additive, with which product 4aa was obtained selectively in 63% yield (entry 23).17 Finally, blank experiments showed that both the catalyst and additive are crucial for the title C–H alkenylation/DG migration and [3 + 2] annulation (entries 15–18, Table S1†).
With the optimal reaction conditions identified, we explored the substrate scope of the Rh(III)-catalyzed C–H alkenylation/DG migration (Table 2). In general, a broad range of N-phenoxyacetamides bearing diverse substituents at R1 could react with 1,3-diynes carrying various substituents at R2 to give the desired tetrasubstituted 1,3-enynes with excellent regio- and stereoselectivity in good to high yields. At first, the scope of N-phenoxyacetamides with 2aa as the model coupling partner was explored. The reaction of N-phenoxyacetamide carrying a Me group at the ortho-position worked well to provide product 3ab with a good yield (67%). The reactions of N-phenoxyacetamides having an electron-donating group (Me), halogens (Cl, Br) or electron-withdrawing groups (CF3, NO2) at the meta-position took place smoothly at the less hindered position irrespective of the electronic nature of the substituents, affording products 3ac–3ag in 71–82% yields. Likewise, N-phenoxyacetamides possessing electron-donating groups (Me, t-Bu), halogens (F, Cl, Br) or electron-withdrawing groups (Ph, CO2Me, CF3) at the para-position could also undergo this reaction to give products 3ah–3ao in 66–80% yields. Of note, the structure of compound 3al was unambiguously confirmed by X-ray crystallography.18 In addition, despite the steric hindrance, 3,5-di-Me and 3,5-di-F substituted N-phenoxyacetamides were also converted into the corresponding products 3ap and 3aq in 47% or 85% yields, respectively. To our delight, this transformation was compatible with N-(naphthalen-2-yloxy)acetamide, which underwent the reaction at the less hindered position to produce product 3ar in 72% yield. Then, the scope of 1,3-diynes was examined with 1aa as the reaction partner. For example, the reactions of symmetrical 1,3-diynes bearing alkyl groups (n-Bu, n-Pent, n-Hex) happened successfully to assemble products 3as–3au in 64–87% yields. Similarly, this reaction could also be extended to symmetrical 1,3-diynes carrying aryl groups, which reacted with 1aa to give products 3av–3ax in 42–63% yields. A representative unsymmetrical alkyl/aryl 1,3-diyne, namely octa-1,3-diyn-1-ylbenzene, was also converted into the desired product 3ay, albeit with a small amount of regioisomer 3ay′. It is worth noting that products 3aa–3ax were obtained as single isomers, indicating the exclusive regio- and stereoselectivity of this Rh(III)-catalyzed C–H alkenylation/DG migration.
Table 2 Substrate scope of the Rh(III)-catalyzed C–H alkenylation/DG migrationa,b,c
Reaction conditions: 1 (0.25 mmol), 2 (0.275 mmol), [Cp*RhCl2]2 (5 mol%), Zn(OAc)2 (0.25 mmol), MeOH (4.0 mL), 25 °C, 24 h.
Isolated yields.
Combined yield.
|
|
Next, the scope of the Rh(III)-catalyzed [3 + 2] annulation was checked (Table 3). Overall, various N-phenoxyacetamides bearing substituents at R1 and 1,3-diynes carrying substituents at R2 turned out to be suitable substrates, and the desired benzofurans were prepared in moderate to good yields with excellent regioselectivity. For instance, the reactions of non-substituted or ortho-substituted N-phenoxyacetamides with 2aa took place smoothly to afford products 4aa–4ac in 40–63% yields. Notably, meta-substituted N-phenoxyacetamides could undergo this reaction with 2aa highly regioselectively at the less hindered position irrespective of the electronic nature of the substituents, delivering products 4ad–4af in 42–49% yields. As expected, the reactions of para-substituted N-phenoxyacetamides with 2aa occurred smoothly to furnish products 4ag–4ak in 45–58% yields. With respect to 1,3-diynes, representative symmetrical 1,3-diynes possessing alkyl groups or aryl groups could be converted into products 4al–4ap in 20–59% yields. It is noteworthy that all the indicated products shown in Table 3 were observed as single regioisomers, suggesting the excellent regioselectivity of this Rh(III)-catalyzed [3 + 2] annulation.
Table 3 Substrate scope of the Rh(III)-catalyzed [3 + 2] annulationa,b
Reaction conditions: 1 (0.25 mmol), 2 (0.275 mmol), [Cp*RhCl2]2 (5 mol%), NaOPiv·H2O (0.25 mmol), CH2Cl2 (4.0 mL), 25 °C, 24 h.
Isolated yields.
|
|
To further prove the synthetic application of this methodology, the Rh(III)-catalyzed C–H alkenylation/DG migration between 1aa and 2aa was scaled up (Scheme 2a). Impressively, the corresponding product 3aa was still obtained with a comparable yield (82%) as the small-scale reaction, indicating the potential industrial application of this reaction. In addition, products 3 and 4 could also undergo further downstream transformations. For example, the hydrogenation of the alkyne moiety of product 4aa afforded compound 5aa in 84% yield (Scheme 2b). Additionally, a preliminary biological screening of the obtained tetrasubstituted 1,3-enynes 3 and alkynylated benzofurans 4 to evaluate their inhibitory activities against the human cancer cell lines A549 and HL-60 was also carried out. Unfortunately, most compounds showed poor inhibition rates at a concentration of 10 μM (Tables S2 and S3, ESI†).
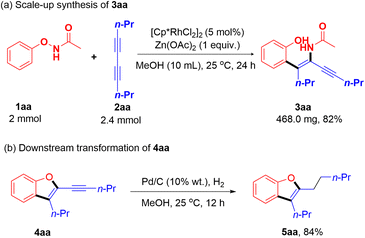 |
| Scheme 2 Synthetic applications. | |
To probe the reaction mechanism, a series of mechanistic studies were performed. Deuterium incorporation experiments were conducted at first (Scheme 3a). Treating 1aa in CD3OD under the standard conditions for 5 minutes resulted in 19% deuteration at the ortho-position. Additionally, the reaction of 1aa and 2aa in CD3OD under the same reaction conditions gave deuterated 3aa with 16% deuteration at the ortho-position of the hydroxyl group. These results suggested that the step of C–H bond cleavage is reversible. Besides, the kinetic isotope effect (KIE) study through intermolecular competition experiments gave a KIE value of 2.45, suggesting that the step of the C–H bond cleavage could be the rate-limiting step (Scheme 3b). Finally, intermolecular competition experiments between electron-rich N-phenoxyacetamide 1ah and electron-deficient N-phenoxyacetamide 1ao resulted in a ratio of 2/1 of the corresponding products 3ah/3ao (Scheme 3c), indicating that electron-rich N-phenoxyacetamides were favoured.
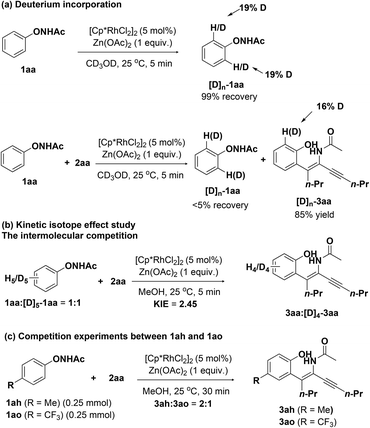 |
| Scheme 3 Mechanistic studies. | |
Based on the preliminary mechanistic studies and literature reports,13a,19 a plausible reaction mechanism was proposed as shown in Scheme 4. At first, DG-assisted C–H activation at the ortho-position occurs to give rhodacycle A. The following regioselective migratory insertion of 1,3-diynes into the Rh–C bond of rhodacycle A gives intermediate B. The product selectivity in different solvents could be explained by the coordinating ability of the solvents. On the one hand, intermediate B could be stabilized by weakly coordinating solvents (e.g. MeOH), which may coordinate with rhodium to form an 18-electron species. In this case, intermediate B undergoes a sequential 1st reductive elimination/oxidative addition/2nd reductive elimination to provide tetrasubstituted 1,3-enynes 3 with the regeneration of the rhodium catalyst (pathway a). On the other hand, when the reaction is performed in non-coordinating solvents (e.g. CH2Cl2), intermediate B prefers to undergo protonation to provide intermediate E, which subsequently undergoes an intramolecular substitution to deliver C2-alkynylated benzofurans 4 with the release of the rhodium catalyst (pathway b).
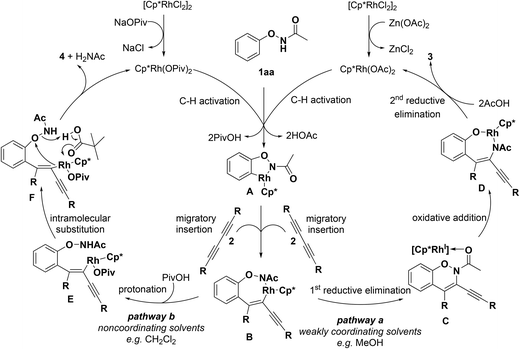 |
| Scheme 4 Proposed reaction mechanism. | |
Conclusions
In conclusion, we have developed the tunable divergent synthesis of tetrasubstituted 1,3-enynes and alkynylated benzofurans via rhodium-catalyzed C−H alkenylation/DG migration and [3 + 2] annulation, respectively. This protocol is characterized by excellent regio- and stereoselectivity, excellent monofunctionalization over difunctionalization, broad substrate scope, good functional group tolerance, moderate to high yields, and mild redox-neutral conditions. The features of complexity- and diversity-generation and tunable product selectivity highlight the potential of this methodology in the synthesis of tetrasubstituted 1,3-enynes and alkynylated benzofurans. Further biological studies of these compounds are currently underway in our laboratory.
Conflicts of interest
There are no conflicts to declare.
Acknowledgements
We gratefully acknowledge the financial support from the Key Research and Development Program of Ningxia Province (Grant 2022CMG03143), State Key Laboratory of Drug Research (Grant SIMM2105KF-09), the National Natural Science Foundation of China (Grant 21602022), the Chengdu Talents Program, the 1000 Talents Program of Sichuan Province, the Longquanyi District Talents Program, the Science and Technology Program of Sichuan Province (Grant 2018JY0345) and the Chengdu University New Faculty Start-up Funding (Grant 2081915037).
References
- For selected reviews, see:
(a) F. Collet, R. H. Dodd and P. Dauban, Chem. Commun., 2009, 5061–5074 RSC;
(b) S. H. Cho, J. Y. Kim, J. Kwak and S. Chang, Chem. Soc. Rev., 2011, 40, 5068–5083 RSC;
(c) D. A. Colby, A. S. Tsai, R. G. Bergman and J. A. Ellman, Acc. Chem. Res., 2012, 45, 814–825 CrossRef CAS;
(d) T. A. Ramirez, B. Zhao and Y. Shi, Chem. Soc. Rev., 2012, 41, 931–942 RSC;
(e) P. B. Arockiam, C. Bruneau and P. H. Dixneuf, Chem. Rev., 2012, 112, 5879–5918 CrossRef CAS PubMed;
(f) J. Wencel-Delord and F. Glorius, Nat. Chem., 2013, 5, 369–375 CrossRef CAS PubMed;
(g) A. Ros, R. Fernández and J. M. Lassaletta, Chem. Soc. Rev., 2014, 43, 3229–3243 RSC;
(h) Z. Chen, B. Wang, J. Zhang, W. Yu, Z. Liu and Y. Zhang, Org. Chem. Front., 2015, 2, 1107–1295 RSC;
(i) J. He, M. Wasa, K. S. L. Chan, Q. Shao and J.-Q. Yu, Chem. Rev., 2017, 117, 8754–8786 CrossRef CAS;
(j) Y. Park, Y. Kim and S. Chang, Chem. Rev., 2017, 117, 9247–9301 CrossRef CAS;
(k) M. T. Mihai, G. R. Genov and R. J. Phipps, Chem. Soc. Rev., 2018, 47, 149–171 RSC;
(l) P. Gandeepan, T. Müller, D. Zell, G. Cera, S. Warratz and L. Ackermann, Chem. Rev., 2019, 119, 2192–2452 CrossRef CAS.
- For selected reviews, see:
(a) D. A. Colby, R. G. Bergman and J. A. Ellman, Chem. Rev., 2010, 110, 624–655 CrossRef CAS PubMed;
(b) N. Yoshikai, Synlett, 2011, 1047–1051 CrossRef CAS;
(c) G. Song, F. Wang and X. Li, Chem. Soc. Rev., 2012, 41, 3651–3678 RSC;
(d) L. Ackermann, Acc. Chem. Res., 2014, 47, 281–295 CrossRef CAS;
(e) B. Zhao, Z. Shi and Y. Yuan, Chem. Rec., 2016, 16, 886–896 CrossRef CAS PubMed;
(f) D. D. Subhedar, A. A. Mishra and B. M. Bhanage, Adv. Synth. Catal., 2019, 361, 4149–4195 CrossRef CAS;
(g) G. Kuang, G. Liu, X. Zhang, N. Lu, Y. Peng, Q. Xiao and Y. Zhou, Synthesis, 2020, 993–1006 CAS;
(h) J. Zhang, X. Lu, C. Shen, L. Xu, L. Ding and G. Zhong, Chem. Soc. Rev., 2021, 50, 3263–3314 RSC.
- For selected reviews, see:
(a) G. Rouquet and N. Chatani, Angew. Chem., Int. Ed., 2013, 52, 11726–11743 CrossRef CAS PubMed;
(b) M. Corbet and F. D. Campo, Angew. Chem., Int. Ed., 2013, 52, 9896–9898 CrossRef CAS;
(c) X. Yang, G. Shan, L. Wang and Y. Rao, Tetrahedron Lett., 2016, 57, 819–836 CrossRef CAS;
(d) J. Liu, G. Chen and Z. Tan, Adv. Synth. Catal., 2016, 358, 1174–1194 CrossRef CAS;
(e) Y. Kommagalla and N. Chatani, Coord. Chem. Rev., 2017, 350, 117–135 CrossRef CAS;
(f) W. Ma, P. Gandeepan, J. Li and L. Ackermann, Org. Chem. Front., 2017, 4, 1435–1467 RSC;
(g) L. D. Caspers and B. J. Nachtsheim, Chem. – Asian J., 2018, 13, 1231–1247 CrossRef CAS;
(h) K. Ghosh, R. K. Rit, M. Shankar, K. Mukherjee and A. K. Sahoo, Chem. Rec., 2020, 20, 1017–1042 CrossRef CAS;
(i) M. Kapoor, A. Singh, K. Sharma and M. H. Hsu, Adv. Synth. Catal., 2020, 362, 4513–4542 CrossRef CAS;
(j) S. Rej, Y. Ano and N. Chatani, Chem. Rev., 2020, 120, 1788–1887 CrossRef CAS PubMed;
(k) L. S. Fitzgerald and M. L. O'Duill, Chem. – Eur. J., 2021, 27, 8411–8436 CrossRef CAS.
- For a recent review and examples on C−H functionalization/DG migration, see: Y. Wu, C. Pi, Y. Wu and X. Cui, Chem. Soc. Rev., 2021, 50, 3677–3689 RSC , and references cited therein.
-
(a) H. Ikemoto, R. Tanaka, K. Sakata, M. Kanai, T. Yoshino and S. Matsunaga, Angew. Chem., Int. Ed., 2017, 56, 7156–7160 CrossRef CAS;
(b) K. Sakata, M. Eda, Y. Kitaoka, T. Yoshino and S. Matsunaga, J. Org. Chem., 2017, 82, 7379–7387 CrossRef CAS;
(c) C. Zhu, R. Kuniyil, B. B. Jei and L. Ackermann, ACS Catal., 2020, 10, 4444–4450 CrossRef CAS;
(d) X. Xu, L. Zhang, H. Zhao, Y. Pan, J. Li, Z. Luo, J. Han, L. Xu and M. Lei, Org. Lett., 2021, 23, 4624–4629 CrossRef CAS.
-
(a) M. Li, T.-Y. Yao, S.-Z. Sun, T.-X. Yan, L.-R. Wen and L.-B. Zhang, Org. Biomol. Chem., 2020, 18, 3158–3163 RSC;
(b) H. Mao, J. Chen, X. Zhang, N. Yu, Y. Lu and F. Zhao, ChemistrySelect, 2022, 7, e202200292 CAS.
-
(a) Y. Chen, D. Wang, P. Duan, R. Ben, L. Dai, X. Shao, M. Hong, J. Zhao and Y. Huang, Nat. Commun., 2014, 5, 4610 CrossRef CAS;
(b) J.-L. Pan, P. Xie, C. Chen, Y. Hao, C. Liu, H.-Y. Bai, J. Ding, L.-R. Wang, Y. Xia and S.-Y. Zhang, Org. Lett., 2018, 20, 7131–7136 CrossRef CAS PubMed;
(c) X. Wu, Y. Lu, J. Qiao, W. Dai, X. Jia, H. Ni, X. Zhang, H. Liu and F. Zhao, Org. Lett., 2020, 22, 9163–9168 CrossRef CAS PubMed;
(d) F. Zhao, J. Qiao, Y. Lu, X. Zhang, L. Dai, X. Gong, H. Mao, S. Lu, X. Wu and S. Liu, Org. Lett., 2021, 23, 5766–5771 CrossRef CAS;
(e) F. Zhao, Z. Zhou, Y. Lu, J. Qiao, X. Zhang, X. Gong, S. Liu, S. Lin, X. Wu and W. Yi, ACS Catal., 2021, 11, 13921–13934 CrossRef CAS;
(f) X. Xu, C. Luo, H. Zhao, Y. Pan, X. Zhang, J. Li, L. Xu, M. Lei and P. J. Walsh, Chem. – Eur. J., 2021, 27, 8811–8821 CrossRef CAS PubMed;
(g) R. Mi, H. Chen, X. Zhou, N. Li, D. Ji, F. Wang, Y. Lan and X. Li, Angew. Chem., Int. Ed., 2022, 61, e202111860 CrossRef CAS PubMed.
- S. Liu, H. Mao, J. Qiao, X. Zhang, Y. Lu, X. Gong, A. Jia, L. Gu, X. Wu and F. Zhao, Asian J. Org. Chem., 2021, 10, 3308–3320 CrossRef CAS.
- D.-G. Yu, F. de Azambuja, T. Gensch, C. G. Daniliuc and F. Glorius, Angew. Chem., Int. Ed., 2014, 53, 9650–9654 CrossRef CAS.
-
(a) F. Zhao, X. Gong, Y. Lu, J. Qiao, X. Jia, H. Ni, X. Wu and X. Zhang, Org. Lett., 2021, 23, 727–733 CrossRef CAS PubMed;
(b) S. Kumar, S. Nunewar, K. M. Usama and V. Kanchupalli, Eur. J. Org. Chem., 2021, 2223–2229 CrossRef CAS.
- F. Zhao, D. Zhang, Y. Nian, L. Zhang, W. Yang and H. Liu, Org. Lett., 2014, 16, 5124–5127 CrossRef CAS PubMed.
-
(a) F. Zhao, X. Jia, J. Zhao, C. Fei, L. Liu, G. Liu, D. Wang and F. Chen, RSC Adv., 2017, 7, 25031–25040 RSC;
(b) X. Jia, P. Li, X. Liu, J. Lin, Y. Chu, J. Yu, J. Wang, H. Liu and F. Zhao, Molecules, 2019, 24, 988 CrossRef PubMed;
(c) H. Ni, X. Shi, Y. Li, X. Zhang, J. Zhao and F. Zhao, Org. Biomol. Chem., 2020, 18, 6558–6563 RSC;
(d) X. Wu, P. Li, Y. Lu, J. Qiao, J. Zhao, X. Jia, H. Ni, L. Kong, X. Zhang and F. Zhao, Adv. Synth. Catal., 2020, 362, 2953–2960 CrossRef CAS;
(e) F. Zhao, J. Chen, J. Qiao, Y. Lu, X. Zhang, H. Mao, S. Lu, X. Gong, S. Liu, X. Wu and L. Dai, Adv. Synth. Catal., 2021, 363, 4380–4389 CrossRef CAS;
(f) F. Zhao, J. Qiao, Y. Lu, X. Zhang, L. Dai, S. Liu, H. Ni, X. Jia, X. Wu and S. Lu, J. Org. Chem., 2021, 86, 10591–10607 CrossRef CAS;
(g) H. Ni, Y. Li, X. Shi, Y. Pang, C. Jin and F. Zhao, Tetrahedron Lett., 2021, 68, 152915 CrossRef CAS.
- For selected examples on TM-catalyzed C–H functionalization of N-phenoxyacetamides, see:
(a) G. Liu, Y. Shen, Z. Zhou and X. Lu, Angew. Chem., Int. Ed., 2013, 52, 6033–6037 CrossRef CAS;
(b) J. Zhou, J. Shi, Z. Qi, X. Li, H. E. Xu and W. Yi, ACS Catal., 2015, 5, 6999–7003 CrossRef CAS;
(c) Z. Hu, X. Tong and G. Liu, Org. Lett., 2016, 18, 1702–1705 CrossRef CAS;
(d) G. Zheng, Z. Zhou, G. Zhu, S. Zhai, H. Xu, X. Duan, W. Yi and X. Li, Angew. Chem., Int. Ed., 2020, 59, 2890–2896 CrossRef CAS;
(e) L. Wu, L. Li, H. Zhang, H. Gao, Z. Zhou and W. Yi, Org. Lett., 2021, 23, 3844–3849 CrossRef CAS PubMed.
-
(a) S. L. Iverson and J. P. Uetrecht, Chem. Res. Toxicol., 2001, 14, 175–181 Search PubMed;
(b) A. L. K. Shi Shun and R. R. Tykwinski, J. Org. Chem., 2003, 68, 6810–6813 CrossRef;
(c) Z. Xu, S. Zhao, Z. Lv, L. Feng, Y. Wang, F. Zhang, L. Bai and J. Deng, Eur. J. Med. Chem., 2019, 162, 266–276 CrossRef CAS PubMed.
- For information on the screening of more solvents, see entries 2–5 in Table S1 in the ESI.†.
- For information on the screening of more additives in MeOH, see entries 6–9 in Table S1 in the ESI.†.
- For information on the screening of more additives in CH2Cl2, see entries 10–14 in Table S1 in the ESI.†.
- CCDC 2201175† contains the supplementary crystallographic data for compound 3al.
-
(a) Y.-F. Yang, K. N. Houk and Y.-D. Wu, J. Am. Chem. Soc., 2016, 138, 6861–6868 CrossRef CAS;
(b) X. Wang, A. Lerchen, T. Gensch, T. Knecht, C. G. Daniliuc and F. Glorius, Angew. Chem., Int. Ed., 2017, 56, 1381–1384 CrossRef CAS;
(c) W. Yi, W. Chen, F.-X. Liu, Y. Zhong, D. Wu, Z. Zhou and H. Gao, ACS Catal., 2018, 8, 9508–9519 CrossRef CAS.
Footnotes |
† Electronic supplementary information (ESI) available: Experimental procedures, characterization data of products, and copies of 1H, 13C and 19F NMR spectra. CCDC 2201175. For ESI and crystallographic data in CIF or other electronic format see DOI: https://doi.org/10.1039/d2ob01800d |
‡ These authors contributed equally to this work. |
|
This journal is © The Royal Society of Chemistry 2023 |
Click here to see how this site uses Cookies. View our privacy policy here.