DOI:
10.1039/D2NR04021B
(Paper)
Nanoscale, 2023,
15, 237-247
A dual-response drug delivery system with X-ray and ROS to boost the anti-tumor efficiency of TPZ via enhancement of tumor hypoxia levels†
Received
21st July 2022
, Accepted 29th October 2022
First published on 4th November 2022
Abstract
The selective anti-tumor activity and less toxic nature of hypoxia-activated prodrugs including tirapazamine (TPZ) are harbored by hypoxia levels in tumors, the inadequacy of which leads to failure in clinical trials. Thus, the development of effective clinical applications of TPZ requires advanced strategies to intensify hypoxia levels in tumors effectively and safely. In this study, we designed and fabricated a paclitaxel (PTX)-loaded dual-response delivery system with a low dose (e.g., 2 Gy) of X-ray and reactive oxygen species on the basis of diselenide block copolymers. Upon the external X-ray stimulus, the system accurately released encapsulated PTX at tumor sites and remarkably improved tumor hypoxia levels by causing severe damage to tumor blood vessels. Subsequently, these enhanced tumor hypoxia levels effectively activated the reduction of TPZ into benzotriazinyl free radicals, which significantly improved the antitumor efficacy of our system against 4T1 breast cancer cells with an initial tumor volume of 500 mm3. Moreover, the dual-stimulus coordinated and controlled release of PTX was found to largely avoid the off-target effects of PTX on normal cells while exhibiting very limited side effects in experimental mice. The current novel strategy for regulating tumor hypoxia levels offers an effective and safe way to activate TPZ for the treatment of large solid tumors.
Introduction
Tumor hypoxia is a characteristic feature of large solid tumors harboring several therapeutic strategies in clinical practice. Moreover, it also emerges as a promising therapeutic target by distinguishing the outlines of tumor tissues from normal tissues.1,2 Several low-toxic hypoxia-activated prodrugs (HAPs) are reduced to highly toxic agents under hypoxic conditions.3–6 These HAPs are capable of killing hypoxic tumor cells selectively while avoiding severe toxicity to normal cells.7 For instance, a representative HAP called tirapazamine (TPZ) can be reduced to benzotriazinyl (BTZ) radicals under hypoxic conditions. Surprisingly, the toxicity of BTZ is much higher by 300-fold than that of its original form (i.e., TPZ), causing severe structural damages to intracellular DNA and eventually inducing cell apoptosis.1,8 However, TPZ has failed in phase III clinical trials because of inadequate hypoxia in most tumors.9 Therefore, the enhancement of hypoxia levels in tumors effectively and safely has become a vital challenge to the clinical applications of TPZ (or other HAPs).10
The first main strategy deployed to overcome this challenge targets the direct consumption of oxygen.11–22 For instance, Li and coworkers prepared a TPZ-encapsulated core–shell up-conversion nanoparticle@porphyrinic MOF that can absorb near-infrared (NIR) light and trigger photochemical reactions to consume oxygen. Similarly, Liu and coworkers developed a camouflaged bionic cascaded-enzyme nanoreactor based on Ti3C2 nanosheets and cell-derived membrane vesicles that can accelerate oxygen consumption during photodynamic processes.23,24 The second strategy involves damaging tumor blood vessels to reduce oxygen supply.25–27 Previously, Chen and coworkers28,29 have synthesized a vascular-disrupting nanomedicine, poly(L-glutamic acid)-graft-methoxy poly(ethylene glycol)/combretastatin A4 nanoparticles, to selectively destroy tumor blood vessels. Similarly, Ye and coworkers30 developed a prodrug Z-GP-DAVLBH from the tubulin-binding vascular-disrupting agent (desacetylvinblastine monohydrazide), which was able to destroy the cytoskeleton of FAPα-expressing tumor pericytes and disrupt the blood vessels both within the core and around the periphery of tumors. The third strategy is a hybrid scheme of the first two. Earlier, Shi and coworkers prepared polyvinyl pyrrolidone–modified inorganic magnesium silicide (Mg2Si) nanoparticles. In a mild acidic tumor environment, the silane released from these nanoparticles efficiently reacted with both tissue-dissolved and hemoglobin-bound oxygen to form aggregates of silicon oxide (SiO2). These aggregates were able to block tumor capillaries and disrupt the oxygen supply of tumors.31 Furthermore, Wu and coworkers also synthesized perfluorocarbon nanoparticles that not only can damage blood vessels via photodynamic effects but also can act as an oxygen sponge to absorb oxygen from tumor tissues.32 Despite the notable advancements, these strategies suffer from several disadvantages including the very limited tissue penetration depth (40–50 mm) and the inability of NIR laser beams to reach deep tumor sites.33 Additionally, the low biodegradability of inorganic nanoparticles may also introduce non-negligible risks for their potential use in clinical treatment.34–37
In this study, we designed and fabricated a dual-response drug delivery system with a low dose (e.g., 2 Gy) of X-ray and reactive oxygen species (ROS) on the basis of diselenide block copolymers. Here, the successful loading of paclitaxel (PTX) into the system was followed by X-ray-stimulated and controlled release of PTX at the tumor sites. Consequently, a remarkable enhancement in tumor hypoxia levels via selective damage of the tumor's blood vessels was effectively achieved. These augmented hypoxia levels then activated the reduction of TPZ into BTZ free radicals and demonstrated significant antitumor efficiency against 4T1 breast cancer cells. Furthermore, this dual-stimulus coordinated and controlled release greatly avoided the off-target effects of chemotherapeutics while enhancing the drug accumulation rate in tumors as compared to other regimens. Therefore, the strategy described in this study for regulating tumor hypoxia levels offers an effective and safe way to activate TPZ for the treatment of large solid tumors.
Results and discussion
Preparation and characterization of nanoparticles
The preparation protocol of amphiphilic copolymers containing multiple diselenide groups in the main chain (Se-polymer) followed our previous work (Fig. S1–S4†).38 The self-assembly of these micelle nanoparticles in aqueous solutions is mainly facilitated by the amphiphilic nature of the Se-polymer and the hydrophobicity of PTX (named P-NPs hereafter). The morphology of these P-NPs was first observed using a transmission electron microscope (TEM). It was followed by the measurement of particle size distribution (
z) and polydispersity index (PDI) via dynamic light scattering (DLS). These TEM and DLS analyses demonstrated that P-NPs have a spherical morphology with an average diameter of ∼103 nm and a PDI of 0.22 (Fig. 1A). High-performance liquid chromatography (HPLC) experiment revealed the PTX loading content (DLC) and drug loading efficiency (DLE) of about 11.45% and 57.25%, respectively. The CMC of these diselenide nanocarriers has already been explored in our previous study.38 Moreover, the stability of P-NPs in different media (e.g., Milli-Q water, phosphate-buffered saline (PBS), and fetal bovine serum (FBS)) was measured by monitoring the changes in their average diameters for 5 days, and the results indicated that P-NPs were highly stable under these conditions (Fig. S5†).
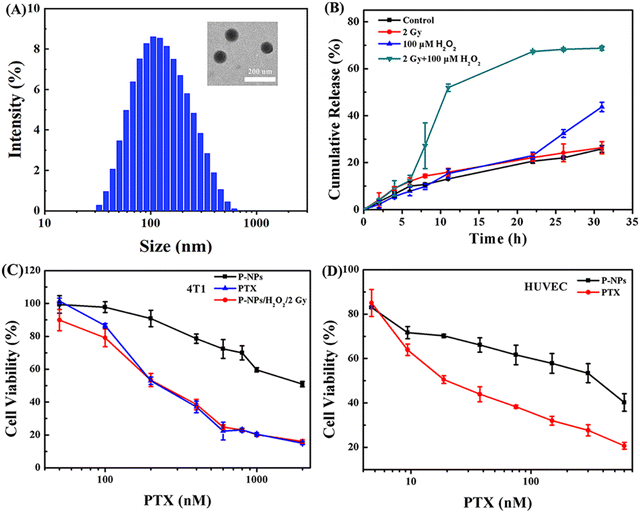 |
| Fig. 1 (A) TEM image of PTX-loaded NPs (P-NPs) in a PBS solution (scale bar = 200 nm) and histogram of the particle-size distribution of the sample. (B) In vitro release of Nile red (NR) from NR-loaded NPs under different conditions. In vitro cytotoxicity of PTX and P-NPs in (C) 4T1 cells and (D) HUVEC cells at 48 h. Data are presented as average ± standard deviation, n ≥ 3. | |
In vitro drug release
The in vitro drug release behavior of P-NPs was first investigated. Nile red (NR) was utilized as the model cargo because of its high stability and detectability after treatment with H2O2 and X-ray irradiation (Fig. S6†).39–41 The release condition of P-NPs was mainly determined by comparing four treatment groups. These experimental design groups were (a) PBS control group, (b) single 2 Gy X-ray irradiation treatment group, (c) single 100 μM H2O2 treatment group, and (d) 2 Gy X-ray and 100 μM H2O2 cotreatment group. In this experiment, H2O2 concentration was set to 100 μM for the optimal simulation of biological ROS levels in the tumor microenvironment.42,43 The X-ray dose was set to 2 Gy to satisfy the criteria of single irradiation dose (single irradiation dose is 1.8–2.0 Gy in actual clinical routine fractionated radiotherapy). In the single 2 Gy X-ray irradiation treatment group (Fig. 1B, red curve), the drug release rate was the same as that in the control group, suggesting very limited influence of single 2 Gy X-ray irradiation. Under the condition of H2O2, a very similar trend in the drug release behavior with a slight increase after the time point of 22 h was observed, as compared to that in the control group. This mechanism is mainly regulated by the oxidation of Se–Se bonds, leading to partial breakage of P-NPs and the subsequent cargo release. In sharp contrast, the 2 Gy X-ray and H2O2 cotreatment led to a very rapid (<10 h) drug release rate exceeding ∼50% initially, and then slowly (>22 h) converged to ∼70%. The mechanism underlying this intriguing behavior has been studied in our previous work by 1H NMR spectroscopy, gel-permeation chromatography analysis, and density functional theory calculations.38 X-rays could break the Se–Se bond, while the generated selenyl moieties would reconnect to diselenides due to the low Se–Se bond energy. However, these irradiation-produced selenyl moieties could be stabilized by ROS (e.g., H2O2) via oxidation reactions, thus preventing the broken Se–Se bond from recombination, and eventually, facilitating Se-NP disintegration upon exposure to X-rays. These results indicated that the enrichment of P-NPs in the tumor tissues followed by both the external X-ray stimulus and the high ROS level in the tumor microenvironment is a result of the precise control of the release of P-NPs’ cargo at the tumor sites. Moreover, this dual-stimulus coordinated and controlled release strategy also successfully avoided the off-target effects of chemotherapeutics while enhancing the drug accumulation rate in the tumors.
Furthermore, the cytotoxicity of P-NPs and drug release behavior were assessed at the cellular level under different stimuli using 4T1 and HUVEC cell lines. In general, P-NPs (IC50 = 686 nM) showed a considerably lower cytotoxicity to 4T1 cells than free PTX (IC50 = 162 nM). However, the cotreatment of P-NPs with ROS and 2 Gy X-ray boosted the cytotoxicity to the same level of PTX in 4T1 cells. The triggered release of cargo from P-NPs showing a reduced IC50 value (194.6 nM) was evident of causing severe toxicity in the tumor cells (Fig. 1C). However, these P-NPs showed substantially lower cytotoxicity in HUVEC cells than free PTX (Fig. 1D). The dual-responsive property of P-NPs at the cellular level was verified to ensure the ability of the Se–Se-containing NP carrier system to enhance drug accumulation at tumor sites and minimize the cytotoxicity of free drugs to normal cells.
Biodistribution
Tumor targetability and enrichment time of drug-loaded diselenide nanoparticles were evaluated in this study as well. The in vivo distribution of Se–Se-containing NPs via fluorescence imaging was monitored by using easily detectable model compounds called cyanine 7 (Cy) dyes and DOX dyes.44 Cy-loaded Se-NPs (Cy-NPs) and DOX-loaded NPs (D-NPs) had a very similar particle size distribution to that of P-NPs (∼89 and ∼108 nm vs. ∼103 nm, Fig. S7†). First, Cy-NPs were administered to mice bearing human 4T1 larger tumor xenografts (≈500 mm3) intravenously. Then, the time evolution of the fluorescence intensity of Cy in mice was monitored using a three-dimensional imaging instrument (IVIS Spectrum), which can reflect the changes in the biodistribution of Cy-NPs over time. As shown in Fig. 2A, the resulting fluorescence gradually intensified around the tumor site at 12 h of Cy-NP injection. After 24 h, the fluorescence intensity at the tumor site was much stronger than that in other regions, suggesting efficient enrichment of most Cy-NPs in tumors. Furthermore, the D-NPs and free DOX (F-DOX, which served as the control) were intravenously injected to mice bearing human 4T1 larger tumor xenografts (≈500 mm3). After 24 and 48 h of injection, the mice were sacrificed for biodistribution studies. As shown in Fig. 2B, D-NPs had a considerably increased tumor enrichment rate than free DOX at the two monitoring time points. Specifically, the tumor uptake rate of DOX delivered by D-NPs was ∼7.5% and ∼7.6% of the injected dose per gram of tissue (%ID g−1) at 24 and 48 h, respectively. In comparison, the free DOX uptake rate was ∼3.0% and 2.7% at 24 and 48 h, respectively. Both of these uptake rates were considerably lower for F-DOX than for D-NPs at the same time point. Hence, the data strongly demonstrated that D-NPs had substantially higher tumor accumulation and retention rates than those of free DOX. We also noted that D-NPs had a relatively high accumulation rate at liver and kidney sites, but both still lower than that of F-DOX. Moreover, 24 h was the optimal irradiation time point to trigger P-NPs for the release of cargo because the tumor uptake rate of P-NPs converged beyond this time point.
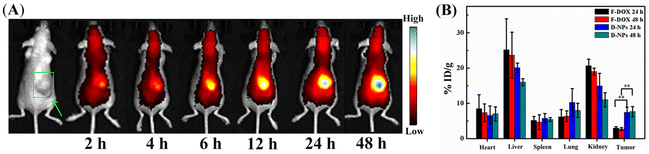 |
| Fig. 2 (A) In vivo distribution and tumor-targeting delivery of Cy-NPs at different time points. (B) In vivo pharmacokinetics and biodistribution of free DOX (F-DOX) and DOX-loaded NPs (D-NPs). | |
Modulation of tumor hypoxia microenvironment in vivo
The role of P-NPs in mediating the hypoxia levels of tumor microenvironments was then studied using a 4T1 tumor-bearing BALB/c mouse model. Photoacoustic (PA) imaging and hypoxia probe immunofluorescence staining were applied to monitor the hypoxia status in the tumor areas. PA imaging was performed at 24 and 48 h after 2 Gy X-ray irradiation and the scanning area was focused on the tumor site (Fig. 3A, green circle). Similarly, the levels of oxygenated hemoglobin (HbO2, red signal) and hemoglobin (Hb, blue signal) were detected to measure the extent of hypoxia in the tumors. As shown in Fig. 3A, both the PTX + Rad treatment and the P-NPs + Rad cotreatment alleviated the hypoxia level of tumor microenvironments with more improved effect observed in the latter than in the former. However, the PTX + Rad cotreatment at 24 h moderately decreased the HbO2 and Hb levels to 68.9% and 58.2% while both remained static at 48 h. In sharp contrast, the P-NPs + Rad treatment at 24 h remarkably decreased the HbO2 and Hb levels to ∼48.6% and ∼49.9%; while at 48 h, the treatment further decreased these levels to ∼38.1% and ∼24.4%, respectively. These results were further confirmed by hypoxia-inducible factor 1α (HIF-1α) immunofluorescence staining, indicating the positive correlation of HIF-1α expression levels with tumor hypoxic levels.45 Indeed, the level of HIF-1α expression in the P-NPs + Rad treatment group was considerably higher than that in the PTX + Rad treatment group (Fig. 3B). Furthermore, semiquantitative analysis of hypoxia-positive signals in the tumor slices revealed that the P-NPs + Rad treatment group had larger positive hypoxic regions (∼79%) than those of the PTX + Rad treatment group (∼60%).
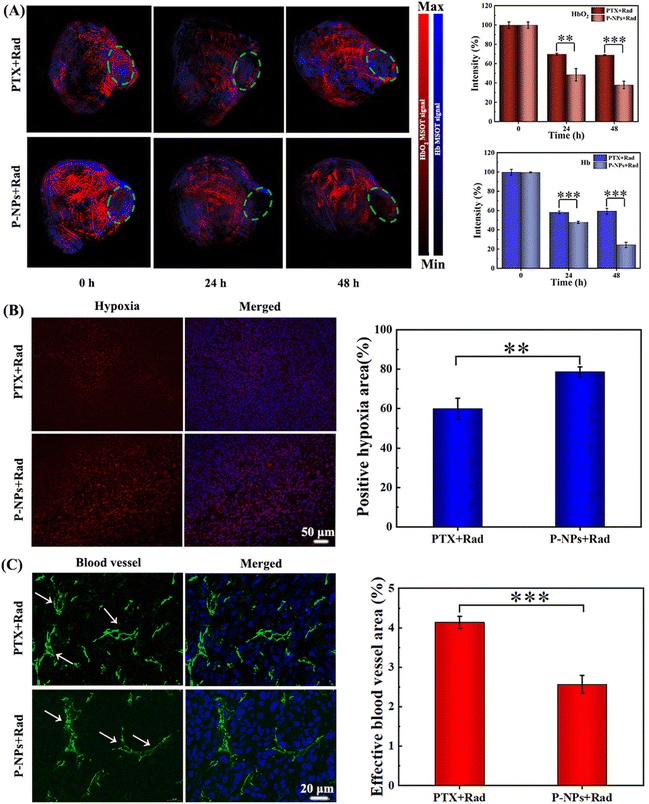 |
| Fig. 3 Visualization of hypoxia induced by P-NPs + Rad and PTX + Rad in 4T1 tumor-bearing mice. (A) PA images, Hb, and HbO2 within tumors determined from PA imaging. (B) Confocal laser scanning microscopic images of hypoxia immunofluorescence and semiquantitative analysis of the percentages of positive hypoxia regions. (C) Tumor vessels (anti-CD31 staining) and semiquantitative analysis of the effective blood vessel areas at 48 h. | |
The effective role of the P-NPs + Rad treatment as compared with the PTX + Rad treatment in the regulation of tumor hypoxia was further determined by examining the vascular status in tumors via CD31 immunofluorescence staining. During the procedure, the damage caused to the tumor blood vessels directly impeded oxygen supply to the tumors and resulted in enhanced tumor hypoxia. Our findings showed that the P-NPs + Rad treatment induced more serious damage to the blood vessels than the PTX + Rad treatment causing them to become more shrunk (Fig. 3C). Moreover, the semiquantitative analysis by depicting the percentage of effective blood vessel area was performed to unveil vascular patency. After the PTX + Rad treatment, the effective vessel area was found to be higher (∼4.1%) by 1.7-fold than that of the P-NPs + Rad treatment (∼2.5%) group. The higher efficiency of the P-NPs + Rad treatment in damaging the tumor blood vessels might have originated from the higher accumulation of P-NPs in the tumor sites. Moreover, this may also have contributions from the precise and sustained release of PTX from P-NPs triggered via X-ray radiations.
In vivo antitumor effect on large tumors
The significantly upregulated tumor hypoxia levels by the P-NPs + X-ray treatment provide a compelling rationale to examine the activation of TPZ in a 4T1 mammary adenocarcinoma model. BALB/c mice bearing an average volume of 500 mm3 4T1 tumors were used to investigate the antitumor effects of our designed treatment regimen. The mice were divided into seven groups: (1) PBS (control), (2) TPZ (20 mg kg−1), (3) TPZ + Rad (20 mg kg−1 + 2 Gy), (4) TPZ + PTX (20 mg kg−1 + 7.5 mg kg−1), (5) TPZ + P-NPs (20 mg kg−1 + 7.5 mg kg−1 on PTX basis), (6) PTX + Rad + TPZ (7.5 mg kg−1 + 2 Gy + 20 mg kg−1), and (7) P-NPs + Rad + TPZ (7.5 mg kg−1 on PTX basis + 2 Gy + 20 mg kg−1). Mice in all groups received two rounds of treatments. At the first round, mice in groups 4, 5, 6 and 7 were injected with PTX or P-NPs on day 0. In groups 3, 6 and 7, 2 Gy X-ray irradiation was administered to mice at 24 h after the injection of PTX or P-NPs. After 12 h of X-ray irradiation, TPZ was intraperitoneally administered to mice. The second-round treatment was launched on day 2, during which the therapeutic regimen for each group was the same as that in the first round (Fig. 4A). The doses of PTX (intravenous) and TPZ (intraperitoneal) administered in all relevant groups in each round were 7.5 mg and 20 mg kg−1, respectively. The treatment efficacy of the applied therapeutic strategies was then depicted by assessing the tumor volume and changes in the body weight of experimental mice (Fig. 4). As shown in Fig. 4B and C, the single TPZ treatment (group 2) only showed a very mild tumor-inhibition effect (∼22.8%) as compared to the PBS control group (group 1). It also suggested that the activation of TPZ was very limited because of the relatively low hypoxia level of tumors under normal natural conditions. In the TPZ + Rad group (group 3), the tumor-inhibiting effects (∼32.1%) were slightly stronger, but this cotreatment also caused very serious side effects manifested by the rapid weight loss among the mice. In comparison with the TPZ + PTX group (group 4), the TPZ + P-NPs group (group 5) showed a slightly higher tumor inhibition efficiency (∼49.2% vs. ∼38.3%) and lower side effects, as demonstrated by the slight increase in the bodyweight of the mice (Fig. 4D). These results could be attributed to the fact that P-NPs enhanced the PTX accumulation in the tumor sites and thereby reduced the off-target effects of free PTX. Unsurprisingly, the PTX + Rad + TPZ treatment (group 6) showed encouraging improvement in antitumor efficacy (∼51.9%) because of the superposition of demonstrated multiple therapeutic effects. However, the superimposed toxicities by the off-target effects of free PTX and X-ray radiation damage also caused the most serious side effects (e.g., most notable body weight loss), thus leading to very limited clinical potential. In sharp contrast, the mice in the P-NPs + Rad + TPZ treatment (group 7) exhibited the highest tumor-inhibition efficiency (∼61.5%) and the lowest side effects with the steady and evident body weight increase. These results were further confirmed by observations of tumor sections (Fig. 4E). The remarkable antitumor advantages of the P-NPs + Rad + TPZ treatment may be attributed to the highly improved tumor hypoxia levels causing destruction of tumor blood vessels. Similarly, the tremendous ability of this treatment to activate the transformation of TPZ to BTZ free radicals was a major contributor in its eventual boosting of the antitumor efficiency.
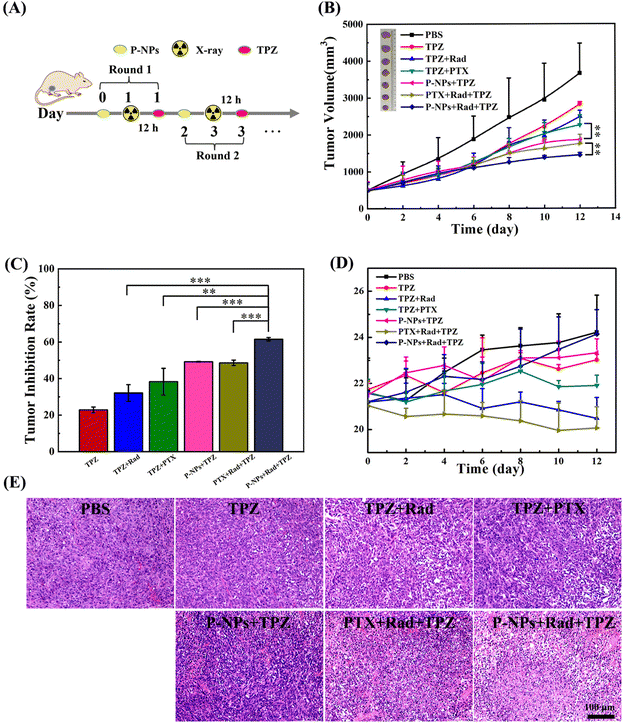 |
| Fig. 4 (A) A sketch map to show two rounds of treatments. (B) Changes in tumor volume in mice treated with PBS (control), TPZ, TPZ + Rad, TPZ + PTX, P-NPs + TPZ, PTX + Rad + TPZ, and P-NPs + Rad + TPZ within 12 days. (C) Tumor inhibitory rate. (D) Body weight changes in 4T1 tumor-bearing mice in different treatment groups. (E) H&E staining of tumor tissues from different treatment groups (400× magnification). All statistical data are presented as mean ± standard deviation (n = 5, *P < 0.05, **P < 0.01, ***P < 0.001). | |
Additionally, the P-NPs increased enrichment of PTX at the tumor sites and application of X-ray radiation also precisely controlled the release of PTX from P-NPs at the tumor sites. Thus, the efficiency of PTX in killing tumor cells was enhanced while reducing its side effects induced by the off-target effects on normal cells. Furthermore, X-ray radiotherapy is also considered beneficial to increase tumor hypoxia. Previous studies have emphasized that diselenium is capable of reducing the damage caused by radiation to mice by regulating the immune system.46,47 We speculate that the diselenium contained in our P-NPs might play a key role in lowering the side effects of X-ray radiation in mice as well.
Finally, the side effects of the P-NPs + Rad + TPZ treatment were also examined on vital internal tissues including the spleen, heart, liver, lungs, and kidneys via hematoxylin and eosin (H&E) staining. As shown in Fig. 5, these tissue sections exhibited no obvious pathological symptoms as compared to the control group. Considering that the liver and kidney were the major off-target accumulation sites when free PTX and P-NPs were intravenously injected to mice, we further examined several conventional function markers of the liver and kidney using a biochemical analyzer at 24 h (Table S1†). In P-NP treatment group, among the tested three liver-related function markers, alanine transaminase (ALT) remained at the same level to the control group, while alkaline phosphatase (ALP) and aspartate transaminase (AST) both showed a mild decrease. However, kidney-related function markers (e.g., blood urea nitrogen (BUN) and creatinine (CREA)) remained unchanged compared with the control group. In the free PTX treatment group, all these markers increased to different degrees. Therefore, these data suggest that P-NPs may have some moderate adverse effects on the liver functions while showing relatively weak influences on the kidney of mice. Nevertheless, free PTX could provoke broader toxicity in the functions of both liver and kidney.
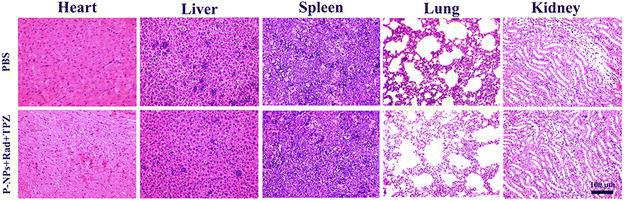 |
| Fig. 5 Histopathological (H&E) analyses of sections of internal organs (i.e., heart, liver, spleen, lungs, and kidneys) from 4T1-bearing mice treated with PBS (control) and P-NPs + Rad + TPZ. | |
Conclusion
The development of an efficient and safe schema for enhancing tumor hypoxia levels is a prerequisite to ensure the clinical application of TPZ against tumors. In this study, we designed and synthesized a PTX-loaded dual-response drug delivery system with a low dose (e.g., 2 Gy) of X-ray and ROS on the basis of diselenide block copolymers (P-NPs). In vitro drug release experiments first revealed that only the cotreatment with 2 Gy X-ray radiation (the most commonly used conventional fractionated radiotherapy dose) and 100 μM H2O2 (simulating biological ROS levels in tumor microenvironments) allows P-NPs to release their cargo with high efficiency (release rate of above 70% after 22 hours of treatment). Then, in vivo cellular assays demonstrated that this dual-response strategy resulted in highly enriched drug accumulation at tumor sites with minimal off-target effects of free drugs on normal cells. The biodistribution studies further confirmed that our delivery system substantially enhanced the drug accumulation rate up to 1.7-fold higher than that of free drugs at the tumor sites. After stimulation with 2 Gy X-ray, PTX inside the system was effectively released to the tumor sites causing damage to the tumor blood vessels, and thus, elevating the tumor hypoxia level. This was followed by vigorously activated reduction of TPZ into BTZ free radicals, which sequentially boosted the antitumor efficiency of our system against 4T1 breast cancer cells with an initial tumor volume of 500 mm3 in experimental mice. After only two rounds of treatments in mice with P-NPs, X-ray irradiation, and TPZ administration, the combined therapy (P-NPs + Rad + TPZ) exhibited the highest tumor inhibition efficiency while showing the least side effects. The tumor inhibitory rate of this treatment was found to be much higher (∼62%) than that of other groups. Meanwhile, the body weight of these mice steadily increased and was considerably more evident than that of mice in the other groups during the entire treatment phase. Therefore, our dual-response delivery system may provide an effective and safe way for modulating tumor hypoxia status to enhance the efficacy of TPZ in treating tumors.
Materials and methods
Materials
All purchased chemicals can be divided into two categories. The first category of chemicals that required no further purification are as follows: selenium powder (99%, Aladdin Reagents), sodium borohydride (98%, Aladdin Reagents), hydrogen peroxide (H2O2, 30%), isophorone diisocyanate (IPDI), dichloromethane (CH2Cl2, A.R., Enox), dibutyltin dilaurate (DBTDL), 11-bromoundecanol (98%, Aladdin Reagents), dimethyl sulfoxide (DMSO, A.R., Enox), and sodium sulfate anhydrous (Na2SO4, 99%, Enox). The second category of chemicals that required further purification are as follows: tetrahydrofuran (THF, A.R., Sinopharm Chemical Reagent) was dried over potassium hydroxide (KOH) for at least two days and then refluxed over sodium. Poly(ethylene glycol) monomethyl ether (mPEG, Mw = 2000, Aladdin Reagents) was dried by azeotropic distillation of toluene.
Characterizations
A dynamic light scattering (DLS) instrument (Nano ZS90, Malvern) was used to analyze the size and size polydispersity index (size PDI) of the nanoparticles at 25 °C. The morphology of the nanoparticle samples was observed using a transmission electron microscope (TEM, HT7700, 120 kV, Hitachi). The encapsulation efficiency of PTX in P-NPs was measured by the HPLC method (UltiMate3000, Thermo Fisher Scientific).
P-NP fabrication
P-NPs were prepared by the method of solvent exchange between Se-polymer and paclitaxel (PTX) in an aqueous solution. In short, 1.5 mg PTX and 10 mg Se-polymer were dissolved in 2 mL DMF and stirred for 2 h. At room temperature, 10 mL of deionized water was added dropwise through an autosampler (WZSFOF) at a flow rate of 2 mL h−1. After dripping, the solution was constantly stirred for 24 h, placed in a dialysis bag (MWCO 3500), and then dialyzed with deionized water for 48 h. A 0.22 μm membrane filter was used to remove bacteria out of the solution. Finally, the solution was centrifuged at 3500 rpm in an ultrafiltration centrifuge tube (MWCO 50 K). The centrifuged supernatant solution was collected and diluted to the required volume with water.
A transmission electron microscope (TEM, HT7700, 120 kV, Hitachi) was used to observe the morphology of the nanoparticle samples. The concentration of PTX was measured by HPLC-MS (UltiMate 3000, Shimadzu), X-ray (RS-2000 Pro Biological Irradiator) and photoacoustic (PA) imaging (IVIS Spectrum, PerkinElmer).
The PTX loading content (DLC) and drug loading efficiency (DLE) were measured by HPLC.
|  | (1) |
|  | (2) |
Statistical analysis
All data are presented as mean ± SD of at least 3 independent culture experiments. Significance was indicated by *P < 0.05, **P < 0.01 and ***P < 0.001 versus untreated controls using Student's t-test (Microsoft Excel, Microsoft Corporation, Redmond, WA, USA).
In vitro drug release
The NR-loaded NPs solution was treated with four different strategies: (a) PBS control group, (b) single 2 Gy X-ray irradiation treatment group, (c) single 100 μM H2O2 treatment group, and (d) 2 Gy X-ray and 100 μM H2O2 cotreatment group. The concentration of NR in NPs at different time intervals was determined using a fluorescence spectrophotometer with excitation at 543 nm over a wavelength range from 500 to 700 nm.
Cell culture
Mouse breast cancer cells (4T1 cells) and human umbilical vein endothelial cells (HUVEC cells) were purchased from the American Type Culture Collection (ATCC). 4T1 cells and HUVEC cells were cultured in RPMI 1640 and a high-glucose DMEM medium (containing 10% fetal bovine serum (FBS) and 1% penicillin–streptomycin solution), respectively.
In vitro cytotoxicity test
The cytotoxicity of P-NPs in HUVEC cells and 4T1 cells was tested. Briefly, 100 μL medium containing cells was added to each well of 96-well plates at a density of 1 × 105 cells per well and incubated for 12 h, and the culture medium was replaced by a medium containing drugs (free PTX or P-NPs at doses of 2000, 1000, 800, 400, 200, 100, and 50 nM PTX). After 48 h of further incubation, 10 μL of CCK-8 solution was added to each well and then incubated for another 1–2 h; the optical density (OD) values of each well were obtained using a microplate reader (SpectraMax, 450 nm). The cell viability was calculated using eqn (3): | Cell viability (%) = ODtest/ODcontrol × 100 | (3) |
where ODtreated and ODcontrol stand for the OD values of the wells treated with drugs and without drugs, respectively.
Animals
Female BALB/c mice with an initial weight of ∼20 g were used in animal experiments. All mice experiments were supervised and approved by the Animal Ethics Committee of Soochow University (permit number: SCXK2018-0006). Each mouse was subcutaneously injected with 2 × 106 4T1 cells on the back to build a tumor-bearing mouse model. The experiments were not carried out until the tumor volume of the mice reached ∼500 mm3.
Detection of liver and kidney function indexes
PBS, free PTX and P-NPs were injected into mice through the tail vein, and mouse blood was collected by enucleation at 24 h. Mice were fasted 12 h before blood collection. The whole blood was placed in EP tubes, centrifuged at 3000 rpm for 10 min, and the supernatant was collected, stored at low temperature and sent to the laboratory on time. The liver and kidney function indexes of mice were measured using an automatic biochemical analyzer. Liver function indexes mainly include alkaline phosphatase (ALP), alanine transaminase (ALT) and aspartate transaminase (AST). Renal function indexes mainly include blood biochemical indexes such as blood urea nitrogen (BUN) and creatinine (CREA).
Multispectral optoacoustic tomographic (MSOT) test
The MSOT test was used to conduct the photoacoustic imaging experiment in mice in order to directly observe the hypoxia status within tumors. PBS, free PTX, and P-NPS (the dose of PTX was 7.5 mg kg−1) were injected into the 4T1 nude mice through the tail vein. The tumors of mice were locally irradiated by 2 Gy X-ray after 24 h. Then, 2% isoflurane was used to anesthetize mice in an MSOT system at 0 h, 24 h, and 48 h after X-ray irradiation. The multispectral scanning wavelengths were set at 680, 715, 730, 760, 800, 850, and 900 nm (0.5 mm step scan). The experimental results were rebuilt into a linear model using software, and the initial image was obtained by linear regression through multi-spectral processing after all experiments were finished. The images of each group were processed uniformly, and the signal intensity in the tumor region was integrated.
Hypoxia immunofluorescence staining
After photoacoustic imaging, tumor tissue sections of each group were taken out and sliced; and the slices were incubated with HIF-1α antibodies (1
:
100) for 12 h. After removal of HIF-1α antibody, tumor tissue sections were incubated with Cy3 goat anti-rabbit IgG secondary antibodies (1
:
100) at room temperature for another 1.5 h. Nuclei were stained with DAPI.
In vivo cancer therapy
Mice bearing 4T1 tumors (∼500 mm3) were randomly divided into different groups and treated with different formulations of P-NPs or free PTX (the dose of PTX was 7.5 mg kg−1). Tumors were exposed to X-ray irradiation at 24 h after each intravenous injection, and 20 mg kg−1 TPZ was intraperitoneally injected into mice at the same time.
The tumor volume (V) and body weight of mice were measured every other day using a vernier caliper and an electronic balance. The tumor volume and tumor suppression rates (TSR, %) were used to evaluate the efficacy of tumor treatment in each treatment group. The TSR and V values were calculated using eqn (4) and (5), respectively. At the end of the experiment, the tumors and major organs (heart, liver, spleen, lung, and kidney) of mice in each group were harvested. The tumors of mice were photographed, and parts of the heart, liver, spleen, lung, kidney, and tumors were sliced for further hematoxylin and eosin (H&E) analysis.
| Tumor volume (V, mm3) = a × b2/2 | (4) |
| Tumor suppression rates (TSR, %) = [(Ac − Ax)/Ac] × 100% | (5) |
In
eqn (4),
a and
b stand for the longest and shortest diameter of tumors, respectively. In
eqn (5),
Ac stands for the tumor volume of the control group and
Ax the tumor volume of the treatment groups.
Author contributions
R. Z. conceived and designed the research. L. Z. and P. H. synthesized the title material and performed characterization. J. H. and P. N. helped with material synthesis. P. H. and L. Z. carried out the molecular, cellular, and animal experiments and analyzed data. Y. Fu., Y. F., J. H., X. Y., and Y. J. helped with various experiments. Z. Y., L. Z., P. H., T. K. and R. Z. co-wrote the paper. All authors discussed and commented on the manuscript.
Conflicts of interest
The authors declare no competing financial interest.
Acknowledgements
This work was partially supported by the National Key R&D Program of China (2021YFA1201200 and 2021YFF1200404), the National Natural Science Foundation of China (U1967217 and 22176137), the Fundamental Research Funds for Central Universities (226-2022-00043, 226-2022-00192), the National Independent Innovation Demonstration Zone Shanghai Zhangjiang Major Projects (ZJZX2020014), the Starry Night Science Fund of Zhejiang University Shanghai Institute for Advanced Study (SN-ZJU-SIAS-003), BirenTech Research (BR-ZJU-SIAS-001), and the Natural Science Foundation of Zhejiang Province (2022LQ22H220001). R. Z. also acknowledges the financial support from W. M. Keck Foundation (Grant award 2019-2022).
References
- W. R. Wilson and M. P. Hay, Targeting Hypoxia in Cancer Therapy, Nat. Rev. Cancer, 2011, 11, 393–410 CrossRef CAS
.
- A. Sahu, W. I. Choi and G. Tae, Recent Progress in the Design of Hypoxia-Specific Nano Drug Delivery Systems for Cancer Therapy, Adv. Ther., 2018, 1, 1800026 CrossRef
.
- D. H. Liang, G. H. Miller and G. K. Tranmer, Hypoxia Activated Prodrugs: Factors Influencing Design and Development, Curr. Med. Chem., 2015, 22, 4313–4325 CrossRef CAS PubMed
.
- F. W. Hunter, B. G. Wouters and W. R. Wilson, Hypoxia-activated Prodrugs: Paths Forward in the Era of Personalised Medicine, Br. J. Cancer, 2016, 114, 1071–1077 CrossRef CAS PubMed
.
- E. Anduran, L. L. Dubois, P. Lambin and J. Y. Winum, Hypoxia-activated Prodrug Derivatives of Anti-cancer Drugs: a Patent Review 2006–2021, Expert Opin. Ther. Pat., 2022, 32, 1–12 CrossRef CAS PubMed
.
- I. N. Mistry, M. Thomas, E. D. D. Calder, S. J. Conway and E. M. Hammond, Clinical Advances of Hypoxia-Activated Prodrugs in Combination with Radiation Therapy, Int. J. Radiat. Oncol., 2017, 98, 1183–1196 CrossRef CAS PubMed
.
- J. M. Brown and W. R. William, Exploiting Tumour Hypoxia in Cancer Treatment, Nat. Rev. Cancer, 2004, 4, 437–447 CrossRef CAS
.
- E. M. Zeman, J. M. Brown, M. J. Lemmon, V. K. Hirst and W. W. Lee, SR-4233: A New Bioreductive Agent with High Selective Toxicity for Hypoxic Mammalian-Cells, Int. J. Radiat. Oncol., 1986, 12, 1239–1242 CrossRef CAS PubMed
.
- K. B. Peters and J. M. Brown, Tirapazamine: A Hypoxia-activated Topoisomerase II Poison, Cancer Res., 2002, 62, 5248–5253 CAS
.
- D. Rischin, R. J. Hicks, R. Fisher, D. Binns, J. Corry, S. Porceddu and L. J. Peters, Prognostic Significance of [18F]-Misonidazole Positron Emission Tomography-Detected Tumor Hypoxia in Patients With Advanced Head and Neck Cancer Randomly Assigned to Chemoradiation With or Without Tirapazamine: A Substudy of Trans-Tasman Radiation Oncology Group Study 98.02, J. Clin. Oncol., 2006, 24, 2098–2104 CrossRef PubMed
.
- J. Chen, X. Wang, Y. Yuan, H. Chen, L. Zhang, H. Xiao, J. Chen, Y. Zhao, J. Chang, W. Guo and X. J. Liang, Exploiting the Acquired Vulnerability of Cisplatin-resistant Tumors with a Hypoxia-amplifying DNA Repair-inhibiting (HYDRI) Nanomedicine, Sci. Adv., 2021, 7, eabc5267 CrossRef CAS PubMed
.
- C. Qian, J. Yu, Y. Chen, Q. Hu, X. Xiao, W. Sun, C. Wang, P. Feng, Q. D. Shen and Z. Gu, Light-Activated Hypoxia-Responsive Nanocarriers for Enhanced Anticancer Therapy, Adv. Mater., 2016, 28, 3313–3320 CrossRef CAS
.
- C. Qian, P. Feng, J. Yu, Y. Chen, Q. Hu, W. Sun, X. Xiao, X. Hu, A. Bellotti, Q. D. Shen and Z. Gu, Anaerobe-Inspired Anticancer Nanovesicles, Angew. Chem., Int. Ed., 2017, 56, 2588–2593 CrossRef CAS PubMed
.
- Y. Dai, B. Wang, Z. Sun, J. Cheng, H. Zhao, K. Wu, P. Sun, Q. Shen, M. Li and Q. Fan, Multifunctional Theranostic Liposomes Loaded with a Hypoxia-Activated Prodrug for Cascade-Activated Tumor Selective Combination Therapy, ACS Appl. Mater. Interfaces, 2019, 11, 39410–39423 CrossRef CAS PubMed
.
- Y. Yang, Y. Lu, P. L. Abbaraju, I. Azimi, C. Lei, J. Tang, M. Jambhrunkar, J. Fu, M. Zhang, Y. Liu, C. Liu and C. Yu, Stepwise Degradable Nanocarriers Enabled Cascade Delivery for Synergistic Cancer Therapy, Adv. Funct. Mater., 2018, 28, 1800706 CrossRef
.
- J. Li, Z. Wei, X. Lin, D. Zheng, M. Wu, X. Liu and J. Liu, Programmable Therapeutic Nanodevices with Circular Amplification of H2O2 in the Tumor Microenvironment for Synergistic Cancer Therapy, Adv. Healthcare Mater., 2019, 8, e1801627 CrossRef
.
- L. Shan, W. Fan, W. Wang, W. Tang, Z. Yang, Z. Wang, Y. Liu, Z. Shen, Y. Dai, S. Cheng, O. Jacobson, K. Zhai, J. Hu, Y. Ma, D. O. Kiesewetter, G. Gao and X. Chen, Organosilica-Based Hollow Mesoporous Bilirubin Nanoparticles for Antioxidation-Activated Self-Protection and Tumor-Specific Deoxygenation-Driven Synergistic Therapy, ACS Nano, 2019, 13, 8903–8916 CrossRef CAS
.
- Y. Wang, Y. Liu, H. Wu, J. Zhang, Q. Tian and S. Yang, Functionalized Holmium-Doped Hollow Silica Nanospheres for Combined Sonodynamic and Hypoxia-Activated Therapy, Adv. Funct. Mater., 2019, 29, 1805764 CrossRef
.
- Y. C. Ma, Y. Y. Zhao, N. K. Bejjanki, X. F. Tang, W. Jiang, J. X. Dou, M. I. Khan, Q. Wang, J. X. Xia, H. Liu, Y. Z. You, G. Q. Zhang, Y. C. Wang and J. Wang, Nanoclustered Cascaded Enzymes for Targeted Tumor Starvation and Deoxygenation-Activated Chemotherapy without Systemic Toxicity, ACS Nano, 2019, 13, 8890–8902 CrossRef CAS PubMed
.
- Y. J. Li, J. J. Dang, Q. J. Liang and L. C. Yin, Carbon Monoxide (CO)-Strengthened Cooperative Bioreductive Anti-tumor Therapy via Mitochondrial Exhaustion and Hypoxia Induction, Biomaterials, 2019, 209, 138–151 CrossRef CAS
.
- Y. Li, L. Sutrisno, Y. Hou, Y. Fei, C. Xue, Y. Hu, M. Li and Z. Luo, A Redox-activatable Biopolymer-based Micelle for Sequentially Enhanced Mitochondria-targeted Photodynamic Therapy and Hypoxia-Dependent Chemotherapy, Chem. Commun., 2020, 56, 9978–9981 RSC
.
- L. Dai, K. Li, M. Li, X. Zhao, Z. Luo, L. Lu, Y. Luo and K. Cai, Size/Charge Changeable Acidity-Responsive Micelleplex for Photodynamic-Improved PD-L1 Immunotherapy with Enhanced Tumor Penetration, Adv. Funct. Mater., 2018, 28, 1707249 CrossRef
.
- Y. Shao, B. Liu, Z. Di, G. Zhang, L. D. Sun, L. Li and C. H. Yan, Engineering of Upconverted Metal-Organic Frameworks for Near-Infrared Light-Triggered Combinational Photodynamic/Chemo-/Immunotherapy Against Hypoxic Tumors, J. Am. Chem. Soc., 2020, 142, 3939–3946 CrossRef CAS
.
- Y. Liu, Y. Liu, W. Bu, C. Cheng, C. Zuo, Q. Xiao, Y. Sun, D. Ni, C. Zhang, J. Liu and J. Shi, Hypoxia Induced by Upconversion-Based Photodynamic Therapy: Towards Highly Effective Synergistic Bioreductive Therapy in Tumors, Angew. Chem., Int. Ed., 2015, 54, 8105–8109 CrossRef CAS PubMed
.
- Z. Ma, Y. Zhang, X. Dai, W. Zhang, M. F. Foda, J. Zhang, Y. Zhao and H. Han, Selective Thrombosis of Tumor for Enhanced Hypoxia-Activated Prodrug Therapy, Adv. Mater., 2021, 33, e2104504 CrossRef
.
- M. K. Zhang, J. J. Ye, Y. Xia, Z. Y. Wang, C. X. Li, X. S. Wang, W. Y. Yu, W. Song, J. Feng and X. Z. Zhang, Platelet-Mimicking Biotaxis Targeting Vasculature-Disrupted Tumors for Cascade Amplification of Hypoxia-Sensitive Therapy, ACS Nano, 2019, 13, 14230–14240 CrossRef CAS
.
- K. Zhang, P. P. Yang, P. P. He, S. F. Wen, X. R. Zou, Y. Fan, Z. M. Chen, H. Cao, Z. Yang, K. Yue, X. X. Zhang, H. Zhang, L. Wang and H. Wang, Peptide-Based Nanoparticles Mimic Fibrillogenesis of Laminin in Tumor Vessels for Precise Embolization, ACS Nano, 2020, 14, 7170–7180 CrossRef CAS
.
- S. Yang, Z. Tang, C. Hu, D. Zhang, N. Shen, H. Yu and X. Chen, Selectively Potentiating Hypoxia Levels by Combretastatin A4 Nanomedicine: Toward Highly Enhanced Hypoxia-Activated Prodrug Tirapazamine Therapy for Metastatic Tumors, Adv. Mater., 2019, 31, e1805955 CrossRef
.
- L. Feng, L. Cheng, Z. Dong, D. Tao, T. E. Barnhart, W. Cai, M. Chen and Z. Liu, Theranostic Liposomes with Hypoxia-Activated Prodrug to Effectively Destruct Hypoxic Tumors Post-Photodynamic Therapy, ACS Nano, 2017, 11, 927–937 CrossRef CAS
.
- G. Ye, M. Huang, Y. Li, J. Ouyang, M. Chen, Q. Wen, X. Li, H. Zeng, P. Long, Z. Fan, J. Yin, W. Ye and D. Zhang, The FAPα-Activated Prodrug Z-GP-DAVLBH Inhibits the Growth and Pulmonary Metastasis of Osteosarcoma Cells by Suppressing the AXL Pathway, Acta Pharm. Sin. B, 2022, 12, 1288–1304 CrossRef CAS
.
- C. Zhang, D. Ni, Y. Liu, H. Yao, W. Bu and J. Shi, Magnesium Silicide Nanoparticles as a Deoxygenation Agent for Cancer Starvation Therapy, Nat. Nanotechnol., 2017, 12, 378–386 CrossRef CAS
.
- W. G. Wang, Y. H. Cheng, P. Yu, H. R. Wang, Y. Zhang, H. H. Xu, Q. S. Ye, A. H. Yuan, Y. Q. Hu and J. H. Wu, Perfluorocarbon Regulates the Intratumoural Environment to Enhance Hypoxia-based Agent Efficacy, Nat. Commun., 2019, 10, 1580 CrossRef
.
- S. Wu and H. J. Butt, Near-infrared Photochemistry at Interfaces Based on Upconverting Nanoparticles, Phys. Chem. Chem. Phys., 2017, 19, 23585–23596 RSC
.
- H. Zhou, J. X. Ge, Q. Q. Miao, R. Zhu, L. Wen, J. F. Zeng and M. Y. Gao, Biodegradable Inorganic Nanoparticles for Cancer Theranostics: Insights into the Degradation Behavior, Bioconjugate Chem., 2020, 31, 315–331 CrossRef CAS PubMed
.
- S. Rogalla, K. Flisikowski, D. Gorpas, A. T. Mayer, T. Flisikowska, M. J. Mandella, X. P. Ma, K. M. Casey, S. A. Felt, D. Saur, V. Ntziachristos, A. Schnieke, C. H. Contag, S. S. Gambhir and S. Harmsen, Biodegradable Fluorescent Nanoparticles for Endoscopic Detection of Colorectal Carcinogenesis, Adv. Funct. Mater., 2019, 29, 1904992 CrossRef CAS PubMed
.
- Y. Zhao, B. L. Allen and A. Star, Enzymatic Degradation of Multiwalled Carbon Nanotubes, J. Phys. Chem. A, 2011, 115, 9536–9544 CrossRef CAS
.
- K. Kummerer, J. Menz, T. Schubert and W. Thielemans, Biodegradability of Organic Nanoparticles in the Aqueous Environment, Chemosphere, 2011, 82, 1387–1392 CrossRef PubMed
.
- L. Zhang, S. Zhang, J. Xu, Y. Li, J. He, Y. Yang, T. Huynh, P. Ni, G. Duan, Z. Yang and R. Zhou, Low-Dose X-ray-Responsive Diselenide Nanocarriers for Effective Delivery of Anticancer Agents, ACS Appl. Mater. Interfaces, 2020, 12, 43398–43407 CrossRef CAS
.
- Y. Y. Yuan, S. D. Xu, C. J. Zhang and B. Liu, Light-responsive AIE Nanoparticles with Cytosolic Drug Release to Overcome Drug Resistance in Cancer Cells, Polym. Chem., 2016, 7, 3530–3539 RSC
.
- Y. Yuan, Z. Wang, P. Cai, J. Liu, L. D. Liao, M. Hong, X. Chen, N. Thakor and B. Liu, Conjugated Polymer and Drug Co-encapsulated Nanoparticles for Chemo- and Photo-thermal Combination Therapy with Two-photon Regulated Fast Drug Release, Nanoscale, 2015, 7, 3067–3076 RSC
.
- L. Song, B. Zhang, E. Jin, C. S. Xiao, G. Li and X. S. Chen, A Reduction-sensitive Thermo-responsive Polymer: Synthesis, Characterization, and Application in Controlled Drug Release, Eur. Polym. J., 2018, 101, 183–189 CrossRef CAS
.
- P. T. Schumacker, Reactive Oxygen Species in Cancer Cells: Live by the Sword, Die by the Sword, Cancer Cell, 2006, 10, 175–176 CrossRef CAS PubMed
.
- C. de Gracia Lux, S. Joshi-Barr, T. Nguyen, E. Mahmoud, E. Schopf, N. Fomina and A. Almutairi, Biocompatible Polymeric Nanoparticles Degrade and Release Cargo in Response to Biologically Relevant Levels of Hydrogen Peroxide, J. Am. Chem. Soc., 2012, 134, 15758–15764 CrossRef CAS PubMed
.
- J. Yang, R. M. Jin, S. Y. Wang, X. T. Xie, W. Hu, H. F. Tang and B. Liu, Co-delivery of Paclitaxel and Doxorubicin using Polypeptide-engineered Nanogels for Combination Therapy of Tumor, Nanotechnology, 2022, 33, 155101 CrossRef PubMed
.
- J. Rius, M. Guma, C. Schachtrup, K. Akassoglou, A. S. Zinkernagel, V. Nizet, R. S. Johnson, G. G. Haddad and M. Karin, NF-kappaB Links Innate Immunity to the Hypoxic Response Through Transcriptional Regulation of HIF-1alpha, Nature, 2008, 453, 807–811 CrossRef CAS PubMed
.
- L. Wang, Z. Y. Wang, Y. Cao, W. H. Lu, L. J. Kuang and D. B. Hua, Strategy for Highly Efficient Radioprotection by a Selenium-Containing Polymeric Drug with Low Toxicity and Long Circulation, ACS Appl. Mater. Interfaces, 2020, 12, 44534–44540 CrossRef CAS
.
- W. Cao, N. C. McCallum, Q. Z. Ni, W. Y. Li, H. Boyce, H. C. Mao, X. H. Zhou, H. Sun, M. P. Thompson, C. Battistella, M. R. Wasielewski, A. Dhinojwala, M. D. Shawkey, M. D. Burkart, Z. Wang and N. C. Gianneschi, Selenomelanin: An Abiotic Selenium Analogue of Pheomelanin, J. Am. Chem. Soc., 2020, 142, 12802–12810 CrossRef CAS PubMed
.
|
This journal is © The Royal Society of Chemistry 2023 |
Click here to see how this site uses Cookies. View our privacy policy here.