DOI:
10.1039/D2NR02059A
(Paper)
Nanoscale, 2023,
15, 365-375
Self-assembling ferrimagnetic fluorescent micelles for bioimaging guided efficient magnetic hyperthermia therapy†
Received
14th April 2022
, Accepted 28th November 2022
First published on 12th December 2022
Abstract
Multifunctional magnet–fluorescent nanocomposites are widely applied in biomedical applications. Incorporating biocompatible quantum dots with highly ferrimagnetic magnetic nanoparticles into one nanoplatform for achieving efficient magnetic hyperthermia therapy (MHT) is very important. Herein, we reported an amphiphilic block copolymer with a flowable hydrophobic chain to encapsulate highly ferrimagnetic magnetic nanoparticles and ZnS/InP quantum dots via a facile self-assembly method. The obtained ferrimagnetic fluorescent micelle (FMFM) exhibited a uniform diameter of about 180 nm. In stark contrast, larger aggregation (400 nm in diameter) inevitably occurred using common poly(D,L-lactide) (PLA)-based amphiphilic block copolymer with a rigid hydrophobic chain, which was readily cleared by the reticuloendothelial system (RES). The flowable FMFM exhibited long-term colloidal stability within one month and desired fluorescent stability within 84 h. Benefiting from the high ferrimagnetism, the FMFM revealed excellent magnetic heating effect and magnetic resonance imaging capability. With accurate manipulation under an external magnetic field, FMFM realized in vitro enhanced fluorescence imaging sensitivity and accumulation efficiency at the tumor region, achieving in vitro and vivo improved MHT efficacy.
Introduction
Magnet–fluorescent nanocomposites (MFNCs), via integrating magnetic nanoparticles (MNPs) and fluorescent quantum dots (QDs) into one multifunctional nanoplatform, undoubtedly combine the complementary advantages of individual nanoparticles.1–3 Nowadays, several efforts have been made to optimize the structure, size, element of MFNCs for the development of biomedical applications such as cell biolabeling, cell sorting, in vivo imaging, and targeted drug delivery and hyperthermia therapy.4–11 In these applications, magnetic hyperthermia therapy (MHT) is a local hyperthermia effect induced by the MNP under alternating current magnetic field (AMF).12–14 With remarkable safety and remotely limitless penetration depth, MHT has been considered one of the most promising clinical cancer therapy approaches.15–17 Unfortunately, the low conversion heating efficiency of Food and Drug Administration (FDA)-approved magnetic nanomaterials (such as Resovist) was one of the key factors that limited the progress of the clinical transformation of MHT.18 Huge efforts have been dedicated to the promotion of the conversion heating efficiency of MNP.19,20 It is certain that the higher ferrimagnetism of MNP would contribute to higher conversion heating efficiency under AMF exposure.21 However, previous research on magneto-fluorescent nanoparticles has focused mostly on the incorporation of superparamagnetic iron oxide. Their limited ferrimagnetism restricts their broader application for fast magnetic responses such as efficient magnetic hyperthermia therapy. Thus, effectively incorporating quantum dots with highly ferrimagnetic magnetic nanoparticles into one nanoplatform for achieving efficient MHT is very important.
In the past decades, several feasible synthetic methods for the preparation of magneto-fluorescent nanoparticles have been approved, such as self-assembly, co-encapsulation into organic or inorganic structures, physical attachment, chemical bonding-based conjugation, element doping, and heterostructure crystal growth.2,6,22,23 Because major high-quality MNPs and QDs were synthesized by the high-temperature oil-phase method, these nanoparticles must be transformed into water-soluble compounds for biological applications.1,20 Compared with other synthetic methods, the self-assembly method based on amphiphilic polymers has revealed superiority and accessibility by easily achieved hydrophobic interactions between hydrophobic segments of polymers and hydrophobic MNPs/QDs. As an example, the representative PEG-modified phospholipid could encapsulate oleic acid-coated MNP and elongated trioctylphosphine-coated QD into one micelle by hydrophobic interactions.24 Nevertheless, the process of encapsulation of MNPs/QDs by amphiphilic polymers, especially for highly ferrimagnetic nanoparticles, needs to be precisely regulated for avoiding the aggregation of inorganic nanoparticles owing to incompatibility between the inorganic nanoparticles and polymerizable component.4 Therefore, it is still a big challenge to synthesize highly ferrimagnetic fluorescent nanoparticles with uniform and suitable size, long-term colloidal stability, and versatile functionality via a facile self-assembly method.
To meet such a requirement, herein, an amphiphilic block copolymer of poly(2-hexoxy-2-oxo-1,3,2-dioxaphospholane) (PHEP) and PEG (PHEP-PEG) was chosen for encapsulation. Compared with commonly used poly(D,L-lactide) (PLA) with a high glass transition temperature (Tg), PHEP has a much lower Tg and revealed a flowable state at room temperature.25 In our previous reports, we found that the flowable core (PHEP)-based micelle exhibited much quicker photothermal and magnetothermal-response behavior than the rigid core (PLA)-based micelle.25–27 We speculated that PHEP-PEG is a better alternative to encapsulate MNP/QD compared to PLA-PEG. As shown in Fig. 1, ZnS/InP core–shell QDs28 were a desirable choice owing to the remarkable biocompatibility when compared with heavy metal quantum dots (such as Cd and Pb29,30). The oleic acid-coated cube-shaped ferrimagnetic iron oxide nanocrystals (CION) were chosen owing to the high ferrimagnetism and conversion heating efficiency under AMF.26,31 Owing to the hydrophobic interaction, the flowable PEG-b-PHEP would encompass the ZnS/InP QDs and CION into the hydrophobic flowable core; the obtained nanoparticles were named as ferrimagnetic fluorescent micelles (FMFMPHEP). Compared with the rigid chain of PLA-PEG-based FMFMPLA, we found that FMFMPHEP with a flowable core exhibited a smaller size, which indicated that PHEP-based amphiphilic block copolymer could effectively avoid the aggregation of inorganic nanoparticles. Furthermore, we demonstrated that FMFMPHEP exhibited long-term colloidal stability, desired fluorescent stability, and excellent magnetic heating effect under AMF. With the assistance of magnetic targeting, the intracellular ZnS/InP QD's fluorescence uptake efficiency and FMFM accumulation at tumor would be enhanced and achieved more efficient MHT effect.
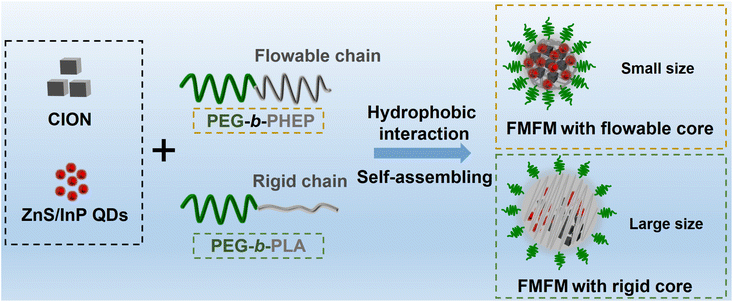 |
| Fig. 1 Schematic illustration of the preparation of ferrimagnetic fluorescent micelles (FMFM). | |
Results and discussion
Preparation and characterization of ferrimagnetic fluorescent micelle
To prepare the multifunctional magnetic fluorescent micelle, firstly, we synthesized the oleic acid-coated CION by a facile thermal decomposition method according to the previous report.32 The TEM image of Fig. 2a showed that the CION was cube-shaped with a uniform diameter of about 22 nm. The Zns/InP QDs as photoluminescent content were synthesized by a high-temperature organic solution method28 and the InP core was coated by a ZnS shell. The obtained highly biocompatible core–shell ZnS/InP QDs are shown in Fig. 2b and Fig. S1a† with a photoluminescent emission at 640 nm (Fig. S1b†). To obtain the micelles, hydrophobic CION and ZnS/InP QDs were transformed into DMSO solution and mixed with PHEP-b-PEG; after the mixture was dropped in water, the strong solvophobic interaction resulted in the assembly of CION and ZnS/InP QDs into the PHEP-based flowable core. As shown in Fig. 2c and the TEM image at large magnification (Fig. S2†), the resulting incorporating micelle has a similarly spherical shape with a diameter of ∼200 nm and the magnifying images further indicated the well-distinguished CION and ZnS/InP QDs. To further visually observe the joint encapsulation of two kinds of nanoparticles, the high-angle annular dark-field (HAADF)-STEM images (Fig. 2d) revealed the contrast distinction of the CION and ZnS/InP QDs inside the micelle, and the corresponding energy dispersive X-ray (EDX) elemental mapping (Fig. 2e and Fig. S3†) further showed the color distribution of Fe, In, P, Zn, and S, which denoted the simultaneous presence of CION and ZnS/InP QDs. The XRD pattern also demonstrated the successful co-encapsulation of CION and ZnS/InP QDs (Fig. S4†).
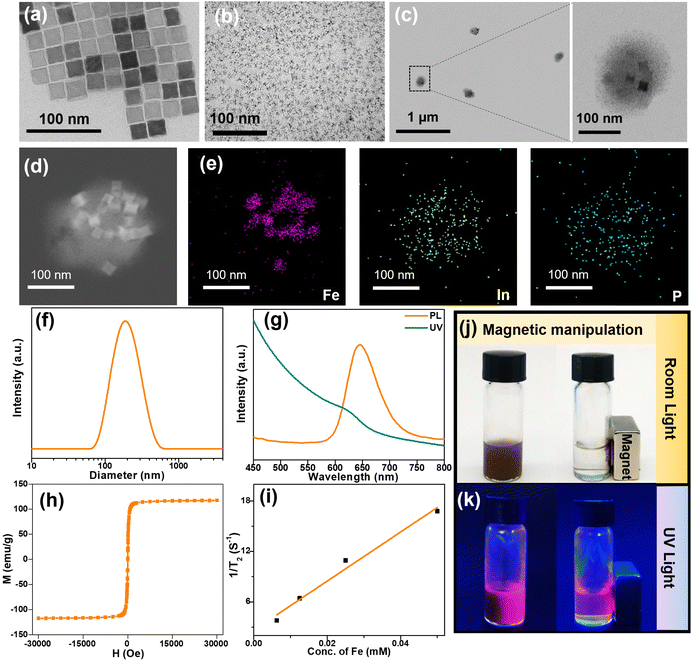 |
| Fig. 2 The preparation and characterization of FMFM. (a) The TEM images of hydrophobic CION and (b) ZnS/InP QDs. (c) The TEM image of FMFM and the corresponding magnifying image. (d) The HAADF-STEM images and corresponding (e) energy dispersive X-ray (EDX) elemental mapping images of FMFM. (f) The hydrodynamic size distribution of FMFM. (g) The UV and photoluminescent spectra of FMFM. (h) The hysteresis curve of FMFM at 300 K. (i) The T2 relaxation rate of FMFM solutions was measured at different Fe concentrations. (j) The photos of FMFM solution under room light or (k) UV light (360 nm) in the presence or absence of a magnet. | |
The dynamic light scattering (DLS) data of FMFM (Fig. 2f) showed a hydrodynamic diameter of ∼180 nm, which was consistent with the TEM results of FMFM. As far as we know, such a size of nanoparticles is feasible to accumulate into the tumor site.27Fig. 2g showed the UV-absorption and fluorescent emission spectra of FMFM, the emission peak of FMFM was located at 650 nm; the redshift of the emission peak (compared with ZnS/InP QDs, Fig. S1b†) might be attributed to the modification of the polymers. Furthermore, the magnetic behavior was investigated in Fig. 2h, the saturation magnetization of FMFM was as high as 117 emu g−1, and the inserted graph further demonstrated the strong ferrimagnetism of FMFM. Owning to the excellent ferrimagnetism, FMFM exhibited a T2 relaxivity as high as 291 mM−1 s−1 (Fig. 2i), which was three times as much as the clinical contrast agent such as Resovist.33,34
We further investigate the joint encapsulation of CION and ZnS/InP QDs by the optical image of the FMFM solution. As shown in Fig. 2j, under the room light, the FMFM was well dispersed with a homogeneous brown color. After the solution was exposed to a magnet, the FMFM was attracted to the magnet quickly and the solution almost was transparent. This sensitive magnetic manipulation was attributed to the high ferrimagnetism of FMFM. Furthermore, under UV (360 nm) light exposure, FMFM exhibited a homogeneous fluorescence in the absence of a magnet (Fig. 2k). In the presence of an external magnet, the fluorescence only appeared in the position of the magnet. This result further demonstrated the joint encapsulation of two functional nanoparticles into one micelle. This magnetic field responsive fluorescence enhancement of FMFM provides a feasible route to achieve a more sensitive fluorescence detection.
In our previous report,25,26 we found that the flowable core-based micelle exhibited much quicker thermal-response behavior than the rigid core-based micelle. Herein, we further investigate the physicochemical property of these two kinds of polymers-loaded CION and ZnS/InP QDs. The PHEP showed a very low phase transition temperature and was flowable at room temperature (Fig. 3a). After PEGylation, the PHEP core-based micelle (FMFMPHEP) showed a size of about 180 nm, and the low magnification TEM image was matched with the DLS data (Fig. 3b, c). By comparison, the commonly used PLA with a phase transition temperature at ∼53 °C was highly rigid (Fig. 3d). After being prepared by the same method, the size of the PLA core-based FMFM (FMFMPLA) was about 400 nm (Fig. 3e), and the corresponding TEM image also determined the much larger size of FMFMPLA than FMFMPHEP (Fig. 3f). Interestingly, we further synthesized other commonly used polymers (poly(lactic-co-glycolic acid) (PLGA) and polycaprolactone (PCL))-based FMFM; both FMFMPLGA and FMFMPCL also exhibited a diameter of about 400 nm (Fig. S5†). The experimental results showed that FMFMPLA, FMFMPLGA, and FMFMPCL possess larger sizes than FMFMPHEP. The excellent controllable CION and ZnS/InP QDs loading ability of PHEP might be attributed to its unique structural and physicochemical properties. In addition, we reasonably speculated that FMFMPHEP was more conducive to biomedical applications due to its appropriate size.32 In the biomedical application, the size of nanoparticles is one of the key factors to determines the tumor accumulation efficiency.35 Importantly, when the size of the nanoparticles is smaller than 200 nm, it would lead to efficient tumor accumulation, while larger nanoparticles (>200 nm) would be cleared by the reticulo-endothelial system (RES).35 We established a sample filtration experiment to investigate the superiority of FMFMPHEP. As shown in Fig. 3g, FMFMPHEP and FMFMPLA (Fe: 100 μg mL−1, In: 100 μg mL−1) were filtrated by a filter with a pore size of 220 nm. After filtration, the color of FMFMPHEP was almost unchanged and there were few sample residues on the filter. In contrast, there were few FMFMPLA that can pass through the filter, and the color of the filtered sample was transparent. After these samples were exposed to the UV light (360 nm) (Fig. 3h), the loaded ZnS/InP QDs exhibited red fluorescence, and the fluorescence intensity was matched with the result of Fig. 3g. Furthermore, we quantitatively measured the sample (n = 3) concentration change in the process of filtration by an inductively-coupled plasma emission spectrometer (ICP). The element (Fe, In) contents of filtered FMFMPHEP were as high as 80%, while only about 30% FMFMPLA can pass through the filter (Fig. 3i). As shown in the diagram in Fig. 3j, this controllable size of about 180 nm of FMFMPHEP indicated that the flowable PEHP was a superior candidate to load these functional nanoparticles when compared with the commonly used rigid core.
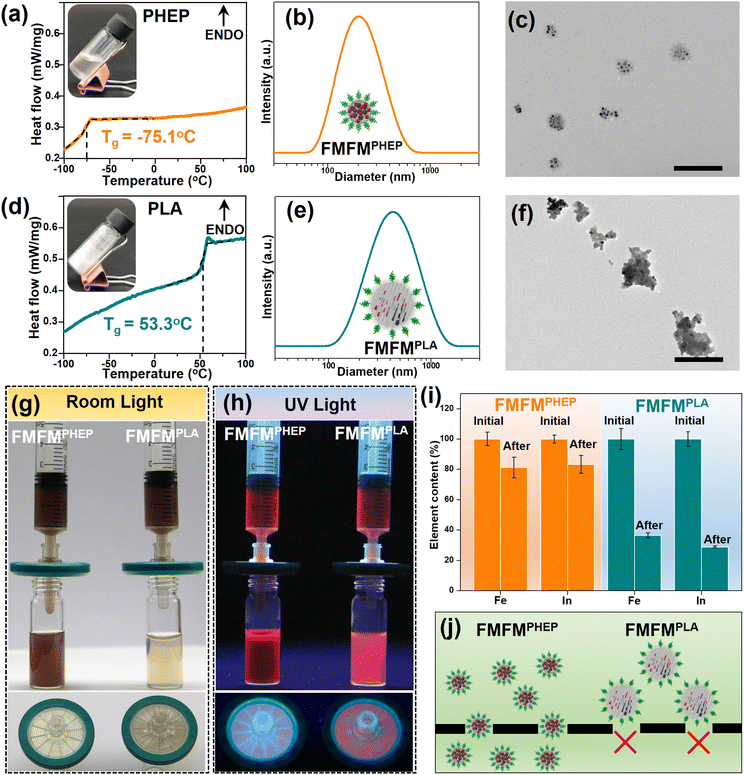 |
| Fig. 3 The differential scanning calorimetry (DSC) analysis and inserted digital images of (a) PHEP and commonly used (d) PLA. The hydrodynamic size and TEM images of (b and c) FMFMPHEP and (e and f) FMFMPLA. The scale bars are 500 nm. The digital images of the filtration experiment (0.22 μm filter) under (g) room light or (h) UV light exposure. The filterable solution was collected in a 4 mL glass bottle; the photos in the following line show the filter after the filtration. (i) The quantitative element contents (Fe, In) of FMFMPHEP and (e and f) FMFMPLA after filtration. (j) Schematic illustration of the superiority of FMFMPHEP in terms of size control. | |
As a therapeutic agent, the nanoparticles would circulate in the physiological environment for a long time.36 Thus, maintaining a highly stable fluorescence intensity and colloidal stability was highly required for FMFM. As shown in Fig. 4a, under room light and room temperature, the FMFM exhibited no photoluminescence intensity change in the storage time of as long as 84 h, suggesting that FMFM emerges with remarkable feasibility for sensitive photoluminescence imaging. Furthermore, within one month, the diameter of FMFM remained at about 180 nm (Fig. 4b) and the polydispersity index (PDI) was retained as low as 0.2, indicating the long-term excellent dispersibility of FMFM. This remarkable colloidal stability of FMFM was attributed to the unique physicochemical properties of PHEP and PEG coating. As inspired by the high ferrimagnetism of FMFM, we further investigated the in vitro magnetic heating effect (Fig. 4c). After exposure to AMF, a significant magnetic nanoparticles concentration-dependent magnetic heating was shown. Importantly, FMFM with an Fe concentration of 400 μg mL−1 rose around 27 °C in 10 min, which indicated that the desired magnetic hyperthermia effect could be achieved at a low agent dose. The infrared thermal images of Fig. 4d further visually confirmed the desired real-time concentration-dependent magnetic heating effect. In our previous report, the specific absorption rate (SAR) value of the CION-based micelle was nearly 20 times higher than that of clinical Resovist.26 Such an excellent magnetic heating effect of FMFM could fully ensure the biomedical application of magnetic hyperthermia therapy.
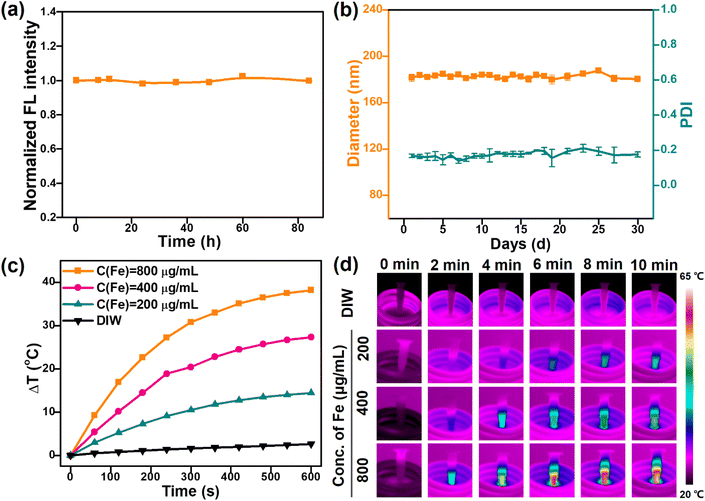 |
| Fig. 4 (a) The fluorescence intensity changes of FMFM within 84 h under room light exposure. (b) The diameter and corresponding polydispersity index change of FMFM within one month. (c) The time-dependent temperature curves and corresponding (d) infrared thermal images of FMFM at various concentrations. | |
In vitro imaging-guided tracking and magnetic hyperthermia therapy of FMFM
The intracellular uptake and distribution of FMFM in the presence or absence of magnetic field were investigated in 4T1 breast cancer cells. For the observation of the fluorescence images of FMFM-treated cells, the cell nuclei were stained by 4′,6-diamidino-2-phenylindole (DAPI) (blue fluorescence), and the cytoskeletal networks were stained by the Alexa 488-phalloidin (green fluorescence). After co-cultured for 12 h, the confocal laser scanning microscopy (CLSM) images of Fig. 5a showed evident red fluorescent (ZnS/InP QDs) distribution in the cytoplasm, indicating that FMFM could be effectively endocytosed by the cell. Moreover, the ZnS/InP QDs-based fluorescent intensity was stronger when the cells were treated with a magnetic field, indicating that the intracellular uptake efficiency could be enhanced by the strategy of magnetic targeting. In addition, the analysis of flow cytometry results (Fig. 5b) showed that the fluorescence intensity of FMFM + Magnet group was ∼2.6 times that of the FMFM group, indicating the advantage of magnetic targeting. The cell biocompatibility of FMFM was investigated, as shown in Fig. 5c, there was no significant cytotoxicity observed even when the concentration of FMFM was as high as 200 μg mL−1. This excellent cytocompatibility was attributed to the highly biocompatible iron oxide nanoparticles and ZnS/InP nanodots. This therapeutic agent design greatly reduced the biotoxicity of common heavy metal quantum dots (such as Cd and Pb). The magnetic hyperthermia therapy efficiency of FMFM against 4T1 cells was evaluated in Fig. 5d. After co-culturing with FMFM (0, 50, 100, 200 μg mL−1) for 12 h in the presence or absence of a magnet, the cells were exposed to AMF. The cell killing efficiency was found to be concentration-dependent. After the cells were exposed to the magnet, the magnetic hyperthermia therapy was further enhanced, which was attributed to the enhanced cell uptake of FMFM and enhanced intracellular magnetic heating.37 We further investigated the visible live and deed cell effect by calcein-acetoxymethyl (calcein-AM, green) and propidium iodide (PI, red) co-staining. As shown in Fig. 5e, these fluorescence images were consistent with the result of Fig. 5d, further confirming the superior MHT effect of FMFM with a magnetic targeting strategy.
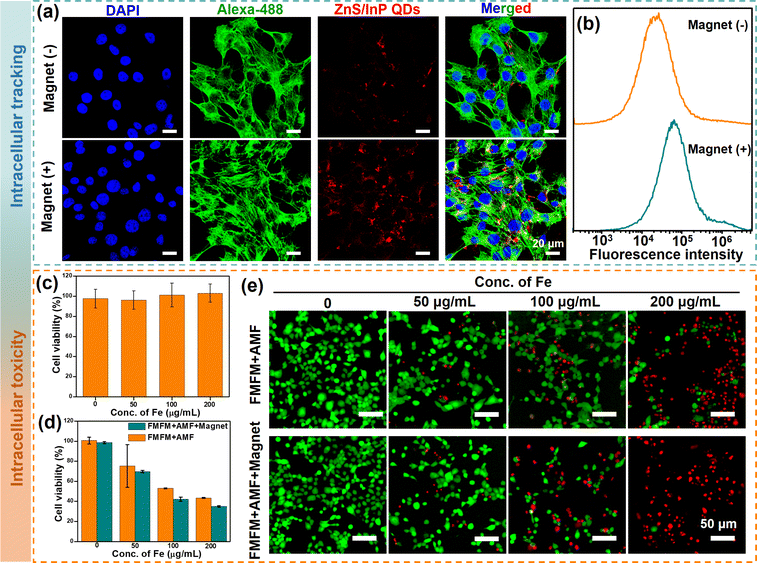 |
| Fig. 5
In vitro test. (a) The confocal laser scanning microscopy (CLSM) images and (b) corresponding flow cytometry analysis of FMFM-treated 4T1 cells with or without an external magnet; the scale bar is 20 μm. (c) The 4T1 cell viability after incubation with FMFM solution at different concentrations. (d) Viabilities and (e) corresponding live-dead co-staining images of 4T1 cells after co-culturing with FMFM at different concentrations with or without an external magnet under AMF exposure; the scale bar is 50 μm. | |
In vivo magnetic hyperthermia therapy of FMFM
Inspired by the remarkable magnetic heating effect and efficient magnetic targeting-enhanced cell uptake efficiency of FMFM, we further evaluated the in vivo MHT effect against 4T1-bearing tumor mouse. All the mice were divided into five groups randomly, namely, PBS group, PBS + AMF group, FMFM group, FMFM + AMF group, and FMFM + Magnet + AMF group. As shown in Table S1,† the initial tumor volumes of every group are very similar. The mice were intravenously injected with FMFM at a dosage of 10 mg Fe per kg, and the tumor region of one group was treated with a cylindrical magnet (Fig. S6a†) (diameter: 8 mm, height: 3 mm, surface magnetic strength: ∼200 mT). As shown in Fig. S6b,† medical tape was applied to fix the cylindrical magnet on the surface of the tumor. After fixing for 24 h, the magnet was removed and the mouse received AMF treatment. After 24 h post-injection, the mice were exposed to AMF (25 kA m−1) for 15 min. As shown in Fig. 6a and b, compared with the PBS + AMF group, the tumor region temperature of the FMFM + AMF group was raised to 39 °C, and the enhanced temperature was attributed to the FMFM accumulation, while such enhancement was difficult to efficiently inhibit the tumor growth. In comparison, with the help of a magnet, the intratumoral temperature of the FMFM + Magnet + AMF group rose quickly to 42 °C within 7 min and reached 45.7 °C in 15 min. Such excellent hyperthermia effect demonstrated efficient FMFM accumulation efficiency using magnetic targeting strategy. Moreover, we investigated the intratumoral delivery efficiency differences without or with magnets. As shown in Fig. S7,† the delivery efficiency of FMFM after intravenous injection was about 0.44%ID g−1. In contrast, with the help of a surface magnet, the delivery efficiency of FMFM was enhanced to about 1.72%ID g−1; this 3.7-fold enhancement significantly points out the function of magnetic targeting. After these treatments, the mice tumor volume and weight were measured every two days for 14 days. As shown in the tumor volume growth curve of Fig. 6c, the AMF treatment without FMFM injection was incapable of suppressing the tumor growth; in contrast, the FMFM + Magnet + AMF group exhibited significant therapeutic effects, while the non-magnet-treated group showed less effective inhibition of tumor growth. No significant difference was observed in the mice body weight curve (Fig. 6d). The tumor weight, tumor photographs, and mice photographs (Fig. 6e–g) further visually verified the superior magnetic field-assisted MHT therapeutic effects of FMFM. As shown in Fig. 6h, the collected tumor was examined by H&E staining. The FMFM + AMF group showed abundant necrocytosis and more cells were killed in the FMFM + Magnet + AMF group.
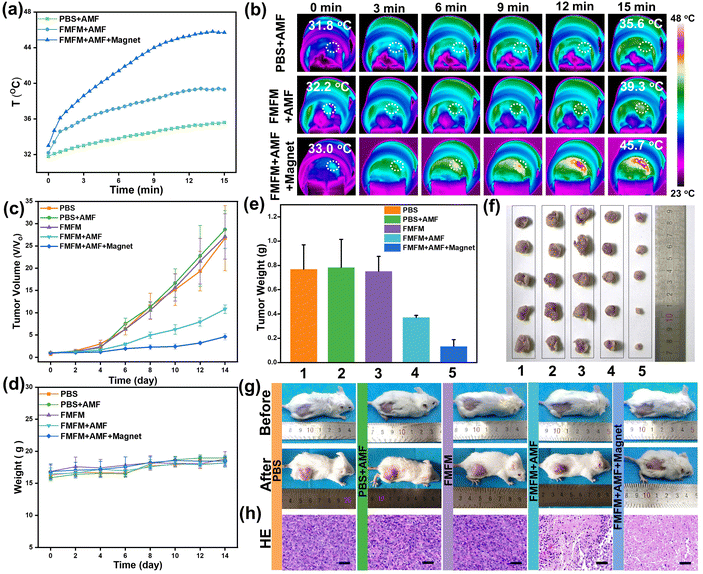 |
| Fig. 6 (a) Time-dependent temperature curves around the tumor region and (b) corresponding infrared thermal images of mice with intravenous administration of PBS, FMFM, or FMFM + magnet under AMF (H = 25 kA m−1, f = 312 kHz) for 15 min. (c) The growth curves, (d) mice weight from different groups after receiving treatments. (e) The tumor weight analysis and (f) corresponding digital images from each group. (g) The digital images of mice from each group before and after treatment. (h) The H&E staining analysis of collected tumors from each group after treatment. All scale bars are 50 μm. | |
The development of nanoheaters with a high conversion heating efficiency at safe conditions is crucial for the clinical translation of MHT.38,39 The H&E staining results (Fig. S8†) of major organs (heart, liver, spleen, lung, kidney) from every group showed no obvious abnormality. Besides, we applied blood biochemical analysis to evaluate the main liver function indexes. The healthy mice were intravenously injected with FMFM (10 mg kg−1); after 24 h, the mice were treated with MH experiment and then the serum was collected. As shown in Fig. 7, obviously, all values of biochemical liver indices of mice were almost consistent in a normal range. These results demonstrated the biosafety of FMFM-mediated MHT.
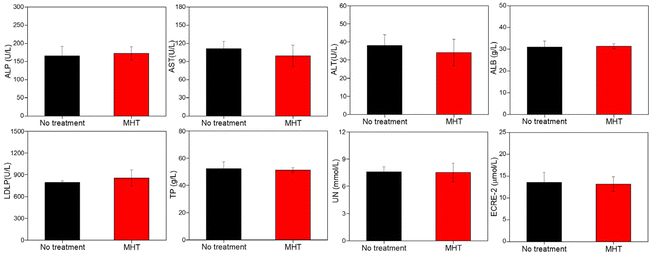 |
| Fig. 7 The biochemical indices from mice treated with intravenously injected FMFM (10 mg kg−1) or without treatment. | |
Conclusion
In summary, we fabricated a novel ferrimagnetic fluorescent micelle (FMFM) by applying amphiphilic block copolymer of PHEP-PEG to encapsulate the hydrophobic ferrimagnetic CION and ZnS/InP QDs into the flowable core. The uniform diameter (∼180 nm), long-term colloidal and fluorescent stability, high ferrimagnetism, and excellent magnetic heating effect ensured that FMFM could be considered a promising candidate used in cancer diagnosis and magnetic hyperthermia therapy. PLA-PEG-based FMFM with a rigid core served as the control nanoparticles and exhibited a much larger diameter of about 400 nm. The FMFM with flowable core performed enhanced cellular fluorescence imaging and enhanced tumor accumulation efficiency with the help of magnetic targeting, achieving the excellent antitumor effect using magnetic hyperthermia. Our ferrimagnetic micellar design on ZnS/InP core–shell QDs could be extended to many other hydrophobic QDs for a broad application.
Experimental
Materials
Iron acetylacetonate (99%), oleic acid (90%), 4-biphenylcarboxylic (99%), and benzyl ether (99%) were obtained from Sigma-Aldrich. Indium chloride (InCl3, 99%), tris(dimethylamino)phosphine ((Me2N)3P, 97%), zinc chloride (ZnCl2, 99%), 1-dodecanethiol (98%), and oleylamine (80%) were purchased from Aladdin. Chloroform, dimethyl sulfoxide, and methanol were obtained from Sinopharm Chemical Reagent.
Synthesis of ferrimagnetic fluorescent micelle
The diblock copolymer of mPEG-b-PHEP, mPEG-b-PLA, was prepared by a method according to the previous report.25 The CION was fabricated by the previous high-temperature decomposition method of iron acetylacetonate.32 InP/ZnS QDs were firstly synthesized by an InP core. The InP core was synthesized using indium trichloride (InCl3) and tris(dimethylamino) phosphine ((Me2N)3P) at high-temperature (250 °C) oleylamine solution, then the core–shell structure was obtained by injecting InP core solution into ZnCl2 oleylamine solution at 200 °C.28 To prepare the FMFM, typically, 1 mg CION, 1 mg InP/ZnS QDs, and 10 mg mPEG-b-PHEP were mixed and dispersed in 2 mL DMSO. Under constant ultrasonicaion, the mixed solution was dropped slowly into 10 mL DIW. After dialysis for 24 h, the solution was purified by a filter (450 nm). The mPEG-b-PLA-based micelle (FMFMPLA) was synthesized by the same procedure. The morphology of FMFM was observed by a transmission electron microscope (TEM, Hitachi HT7700). The hydrated diameter and zeta potential of FMFM were determined using a DLS instrument (ZS-90 nanosizer, Malvern Zetasizer). The UV-Vis and fluorescence spectra of FMFM were recorded on a UV-2600 (SHIMADZU, Kyoto, Japan) and a fluorescence spectrophotometer (HITACHI F-4700, Tokyo, Japan), respectively.
Magnetic heating effect of FMFM
0.5 mL FMFM, with different concentrations (200, 400, and 800 μg Fe per mL), were injected into a 1.5 mL tube. Each sample was placed in the center of the coil of the heating equipment (Shuangping SPG, China). The magnetic intensity was set as 25 kA m−1 and the frequency (f) was 312 kHz. The temperature change was recorded by an infrared thermography camera (Fluke, Ti400).
In vitro fluorescence imaging and magnetic hyperthermia therapy
The 4T1 cancerous cells, obtained from the University of Science and Technology of China, were exposed to FMFM (50 μg Fe per mL) in the presence or absence of a magnet. After co-incubation for 12 h, the cell nucleus and cytoskeletal networks were stained by DAPI or Alexa 488-phalloidin, respectively. The fluorescence of cell uptake was observed by a confocal laser scanning microscope (Carl Zeiss Jena, Germany, LSM 880 with Airyscan). Also, the quantitative fluorescent intensity of InP was measured by flow cytometry (BD Accuri C6 Plus). To evaluate the in vitro FMFM-induced magnetic heating cell killing effect, 4T1 cancerous cells were exposed to FMFM at different concentrations (50, 100, and 200 μg Fe per mL). In the absence or presence of the magnet, the cells were further cultured for 12 h. Then, the cells were exposed to AMF (H = 25 kA m−1) for 10 min. Finally, the cell viability was measured by a standard CCK-8 test method. Meanwhile, the visual observation (IX81, Olympus) of live and dead cells was carried out by calcein AM and propidium iodide (PI) staining.
In vivo magnetic hyperthermia therapy
All the 4T1 tumor bearing mice were randomly divided into 5 groups (n = 5): (1) PBS group, (2) PBS + AMF group, (3) FMFM group, (4) FMFM + AMF group, (5) FMFM + AMF + Magnet group. The groups (1) and (2) were treated with the intravenously injected 100 μL PBS. The mice of groups of (3), (4), and (5) were intravenously injected with FMFM (10 mg kg−1), and the mice of group (5) were fixed with a magnet in the tumor region. After 24 h, the mice of groups (2), (4), and (5) were exposed to AMF (H = 25 kA m−1, 15 min). After these treatments, the body weight and tumor volume of the mice were measured and recorded every two days. After 14 days, all the mice were sacrificed and the collected tumor tissue was weighted. All the collected organs and tumor were stained with Hematoxylin & eosin. To investigate the intratumoral delivery efficiency, the 4T1 breast cancer bearing mice were randomly divided into two groups (n = 5), and all mice were intravenously injected with FMFM (10 mg kg−1) and one group was treated with magnetic targeting. After 24 h, the tumor tissues were collected and treated with HNO3. The concentration of Fe was measured by inductively-coupled plasma mass spectrometry (ICP-MS).
All animal experiments were performed in accordance with the recommendations in the Guide for the Care and Use of Laboratory Animals of the National Institutes of Health, which were approved by the Institutional Animal Care and Use Committee (IACUC) of Hefei University of Technology and Anhui Medical University (LLSC20150134).
Author contributions
Y.J.X., J.X.W. and L.D conceived the idea and designed the experiments. Y.H.S., Y.Q.Z and K.J. supervised the research. Y.H.S. and Y.Q.Z. performed the experiments and analyzed the data. K.J., L.D., X.Y., D.L., S.C., H.Y.X., X.Z.Y. and Y.L. helped to analyze the data and provided valuable advice. Y.H.S., Y.Q.Z., K.J., Y.J.X., L.D. and J.X.W. co-wrote the manuscript. All authors discussed the results and commented on the manuscript.
Conflicts of interest
The authors declare no conflicts of interest.
Acknowledgements
This work was financially supported by the National Natural Science Foundation of China (No. 22105195, 52103160, 51702309, 51572067, 21501039), the Fundamental Research Funds for the Central Universities (No. WK9110000062, WK2060000033, JZ2021HGQB0279), the Anhui Provincial Natural Science Foundation (2208085MH259), China Postdoctoral Science Foundation (BH2060000173), and National Key Research and Development Program of China (Grant 2018YFE0202201), the Science and Technology Project of Guangzhou (No. 202102020441, 202102010025), Guangzhou Key Laboratory of Molecular Imaging and Clinical Translational Medicine (202201020376), Open Project of Guangdong Provincial Key Laboratory of Biomedical Engineering (KLBEMGD202103), and National Engineering Research Center for Tissue Restoration and Reconstruction (NERC-TRR202103), Guangdong Basic and Applied Basic Research Foundation (2022A1515010074), Young Talent Support Project of Guangzhou Association for Science and Technology (QT20220101146).
References
- N. Zhao, L. Yan, X. Zhao, X. Chen, A. Li, D. Zheng, X. Zhou, X. Dai and F. J. Xu, Versatile types of organic/inorganic nanohybrids: From strategic design to biomedical applications, Chem. Rev., 2019, 119, 1666–1762 CrossRef CAS.
- L. Jing, K. Ding, S. V. Kershaw, I. M. Kempson, A. L. Rogach and M. Gao, Magnetically engineered semiconductor quantum dots as multimodal imaging probes, Adv. Mater., 2014, 26, 6367–6386 CrossRef CAS PubMed.
- O. Chen, L. Riedemann, F. Etoc, H. Herrmann, M. Coppey, M. Barch, C. T. Farrar, J. Zhao, O. T. Bruns, H. Wei, P. Guo, J. Cui, R. Jensen, Y. Chen, D. K. Harris, J. M. Cordero, Z. Wang, A. Jasanoff, D. Fukumura, R. Reimer, M. Dahan, R. K. Jain and M. G. Bawendi, Magneto-fluorescent core-shell supernanoparticles, Nat. Commun., 2014, 5, 5093 CrossRef CAS PubMed.
- D. Kim, K. Shin, S. G. Kwon and T. Hyeon, Synthesis and biomedical applications of multifunctional nanoparticles, Adv. Mater., 2018, 30, 1802309 CrossRef.
- C. Y. Wen, H. Y. Xie, Z. L. Zhang, L. L. Wu, J. Hu, M. Tang, M. Wu and D. W. Pang, Fluorescent/magnetic micro/nano-spheres based on quantum dots and/or magnetic nanoparticles: preparation, properties, and their applications in cancer studies, Nanoscale, 2016, 8, 12406–12429 RSC.
- A. Tufani, A. Qureshi and J. H. Niazi, Iron oxide nanoparticles based magnetic luminescent quantum dots (MQDs) synthesis and biomedical/biological applications: A review, Mater. Sci. Eng., C, 2021, 118, 111545 CrossRef CAS.
- F. Yang, A. Skripka, M. S. Tabatabaei, S. H. Hong, F. Ren, A. Benayas, J. K. Oh, S. Martel, X. Liu, F. Vetrone and D. Ma, Multifunctional self-assembled supernanoparticles for deep-tissue bimodal imaging and amplified dual-mode heating treatment, ACS Nano, 2019, 13, 408–420 CrossRef CAS PubMed.
- G. Song, X. Zheng, Y. Wang, X. Xia, S. Chu and J. Rao, A Magneto-optical nanoplatform for multimodality imaging of tumors in mice, ACS Nano, 2019, 13, 7750–7758 CrossRef CAS PubMed.
- D. H. Ortgies, F. J. Teran, U. Rocha, L. de la Cueva, G. Salas, D. Cabrera, A. S. Vanetsev, M. Rähn, V. Sammelselg, Y. V. Orlovskii and D. Jaque, Optomagnetic nanoplatforms for in situ controlled hyperthermia, Adv. Funct. Mater., 2018, 28, 1704434 CrossRef.
- Y. Lu, Y. J. Xu, G. B. Zhang, D. S. Ling, M. Q. Wang, Y. Zhou, Y. D. Wu, T. Wu, M. J. Hackett, B. H. Kim, H. Chang, J. Kim, X. T. Hu, L. Dong, N. Lee, F. Y. Li, J. C. He, L. Zhang, H. Q. Wen, B. Yang, S. H. Choi, T. Hyeon and D. H. Zou, Iron oxide nanoclusters for T1 magnetic resonance imaging of non-human primates, Nat. Biomed. Eng., 2017, 1, 637–643 CrossRef CAS PubMed.
- Q. J. Bi, X. Song, A. Hu, T. Y. Luo, R. R. Jin, H. Ai and Y. Nie, Magnetofection: Magic magnetic nanoparticles for efficient gene delivery, Chin. Chem. Lett., 2020, 31, 3041–3046 CrossRef CAS.
- X. Liu, B. Yan, Y. Li, X. Ma, W. Jiao, K. Shi, T. Zhang, S. Chen, Y. He, X. J. Liang and H. Fan, Graphene oxide-grafted magnetic nanorings mediated magnetothermodynamic therapy favoring reactive oxygen species-related immune response for enhanced antitumor efficacy, ACS Nano, 2020, 14, 1936–1950 CrossRef CAS.
- Y. Song, L. A. Shi, H. Xing, K. Jiang, J. Ge, L. Dong, Y. Lu and S. H. Yu, A Magneto-heated ferrimagnetic sponge for continuous recovery of viscous crude oil, Adv. Mater., 2021, 33, 2100074 CrossRef CAS PubMed.
- W. Ying, Y. Zhang, W. Gao, X. Cai, G. Wang, X. Wu, L. Chen, Z. Meng, Y. Zheng, B. Hu and X. Lin, Hollow magnetic nanocatalysts drive starvation-chemodynamic-hyperthermia synergistic therapy for tumor, ACS Nano, 2020, 14, 9662–9674 CrossRef CAS.
- Y. Zhang, X. Wang, C. Chu, Z. Zhou, B. Chen, X. Pang, G. Lin, H. Lin, Y. Guo, E. Ren, P. Lv, Y. Shi, Q. Zheng, X. Yan, X. Chen and G. Liu, Genetically engineered magnetic nanocages for cancer magneto-catalytic theranostics, Nat. Commun., 2020, 11, 5421 CrossRef PubMed.
- J. Pan, Y. Xu, Q. Wu, P. Hu and J. Shi, Mild magnetic hyperthermia-activated innate immunity for liver cancer therapy, J. Am. Chem. Soc., 2021, 143, 8116–8128 CrossRef CAS PubMed.
- N. Yang, F. Gong, L. Cheng, H. Lei, W. Li, Z. Sun, C. Ni, Z. Wang and Z. Liu, Biodegradable magnesium alloy with eddy thermal effect for effective and accurate magnetic hyperthermia ablation of tumors, Natl. Sci. Rev., 2021, 8, nwaa122 CrossRef CAS.
- J. H. Lee, J. T. Jang, J. S. Choi, S. H. Moon, S. H. Noh, J. W. Kim, J. G. Kim, I. S. Kim, K. I. Park and J. Cheon, Exchange-coupled magnetic nanoparticles for efficient heat induction, Nat. Nanotechnol., 2011, 6, 418–422 CrossRef CAS PubMed.
- H. Gavilan, S. K. Avugadda, T. Fernandez-Cabada, N. Soni, M. Cassani, B. T. Mai, R. Chantrell and T. Pellegrino, Magnetic nanoparticles and clusters for magnetic hyperthermia: Optimizing their heat performance and developing combinatorial therapies to tackle cancer, Chem. Soc. Rev., 2021, 50, 11614–11667 RSC.
- N. Gu, Z. Zhang and Y. Li, Adaptive iron-based magnetic nanomaterials of high performance for biomedical applications, Nano Res., 2021, 15, 1–17 CrossRef.
- N. Lee, D. Yoo, D. Ling, M. H. Cho, T. Hyeon and J. Cheon, Iron oxide based nanoparticles for multimodal imaging and magnetoresponsive therapy, Chem. Rev., 2015, 115, 10637–10689 CrossRef CAS PubMed.
- S. K. Pahari, S. Olszakier, I. Kahn and L. Amirav, Magneto-fluorescent yolk-shell nanoparticles, Chem. Mater., 2018, 30, 775–780 CrossRef CAS.
- F. Part, C. Zaba, O. Bixner, T. A. Grünewald, H. Michor, S. Küpcü, M. Debreczeny, E. De Vito Francesco, A. Lassenberger, S. Schrittwieser, S. Hann, H. Lichtenegger and E.-K. Ehmoser, Doping method determines para- or superparamagnetic properties of photostable and surface-modifiable quantum dots for multimodal bioimaging, Chem. Mater., 2018, 30, 4233–4241 CrossRef.
- J. H. Park, G. von Maltzahn, E. Ruoslahti, S. N. Bhatia and M. J. Sailor, Micellar
hybrid nanoparticles for simultaneous magnetofluorescent imaging and drug delivery, Angew. Chem., Int. Ed., 2008, 47, 7284–7288 CrossRef CAS.
- J. Wang, Y. Liu, Y. Ma, C. Sun, W. Tao, Y. Wang, X. Yang and J. Wang, NIR-activated supersensitive drug release using nanoparticles with a flow core, Adv. Funct. Mater., 2016, 26, 7516–7525 CrossRef CAS.
- Y. H. Song, D. D. Li, Y. Lu, K. Jiang, Y. Yang, Y. J. Xu, L. Dong, X. Yan, D. S. Ling, X. Z. Yang and S. H. Yu, Ferrimagnetic mPEG-b-PHEP copolymer micelles loaded with iron oxide nanocubes and emodin for enhanced magnetic hyperthermia-chemotherapy, Natl. Sci. Rev., 2020, 7, 723–736 CrossRef CAS.
- Y. Zhu, Y. Song, Z. Cao, L. Dong, Y. Lu, X. Yang and J. Wang, Magnetically actuated active deep tumor penetration of deformable large nanocarriers for enhanced cancer therapy, Adv. Funct. Mater., 2021, 31, 2103655 CrossRef CAS.
- K. Kim, D. Yoo, H. Choi, S. Tamang, J. H. Ko, S. Kim, Y. H. Kim and S. Jeong, Halide-amine co-passivated indium phosphide colloidal quantum dots in tetrahedral shape, Angew. Chem., Int. Ed., 2016, 55, 3714–3718 CrossRef CAS PubMed.
- A. Tiwari, N. C. Verma, A. Singh, C. K. Nandi and J. K. Randhawa, Carbon coated core-shell multifunctional fluorescent SPIONs, Nanoscale, 2018, 10, 10389–10394 RSC.
- E. Oh, R. Liu, A. Nel, K. B. Gemill, M. Bilal, Y. Cohen and I. L. Medintz, Meta-analysis of cellular toxicity for cadmium-containing quantum dots, Nat. Nanotechnol., 2016, 11, 479–486 CrossRef CAS PubMed.
- K. Y. Qian, Y. Song, X. Yan, L. Dong, J. Xue, Y. Xu, B. Wang, B. Cao, Q. Hou, W. Peng, J. Hu, K. Jiang, S. Chen, H. Wang and Y. Lu, Injectable ferrimagnetic silk fibroin hydrogel for magnetic hyperthermia ablation of deep tumor, Biomaterials, 2020, 259, 120299 CrossRef CAS.
- D. Kim, N. Lee, M. Park, B. H. Kim, K. An and T. Hyeon, Synthesis of uniform ferrimagnetic magnetite nanocubes, J. Am. Chem. Soc., 2009, 131, 454–455 CrossRef CAS PubMed.
- M. Jeon, M. V. Halbert, Z. R. Stephen and M. Zhang, Iron oxide nanoparticles as T1 contrast agents for magnetic resonance imaging: Fundamentals, challenges, applications, and prospectives, Adv. Mater., 2021, 33, 1906539 CrossRef CAS.
- Z. Zhou, L. Yang, J. Gao and X. Chen, Structure-relaxivity relationships of magnetic nanoparticles for magnetic resonance imaging, Adv. Mater., 2019, 31, 1804567 CrossRef.
- J. Ding, J. Chen, L. Gao, Z. Jiang, Y. Zhang, M. Li, Q. Xiao, S. S. Lee and X. Chen, Engineered nanomedicines with enhanced tumor penetration, Nano Today, 2019, 29, 100800 CrossRef CAS.
- W. Li, J. Tang, D. Lee, T. R. Tice, S. P. Schwendeman and M. R. Prausnitz, Clinical translation of long-acting drug delivery formulations, Nat. Rev. Mater., 2022, 7(5), 406–420 CrossRef.
- B. Sanz, M. P. Calatayud, T. E. Torres, M. L. Fanarraga, M. R. Ibarra and G. F. Goya, Biomaterials, 2017, 114, 62–70 CrossRef CAS PubMed.
- S. Healy, A. F. Bakuzis, P. W. Goodwill, A. Attaluri, J. W. M. Bulte and R. Ivkov, Wiley Interdiscip. Rev.: Nanomed.
Nanobiotechnol., 2022, 14, e1779 Search PubMed.
- H. F. Rodrigues, G. Capistrano and A. F. Bakuzis, Int. J. Hyperthermia, 2020, 37, 76–99 CrossRef CAS PubMed.
Footnotes |
† Electronic supplementary information (ESI) available. See DOI: https://doi.org/10.1039/d2nr02059a |
‡ Y. H. S., Y. Q. Z and K. J. contributed equally to this work. |
|
This journal is © The Royal Society of Chemistry 2023 |
Click here to see how this site uses Cookies. View our privacy policy here.