DOI:
10.1039/D2MH01034H
(Communication)
Mater. Horiz., 2023,
10, 136-148
Cytoskeleton-inspired hydrogel ionotronics for tactile perception and electroluminescent display in complex mechanical environments†
Received
19th August 2022
, Accepted 24th October 2022
First published on 26th October 2022
Abstract
The emerging applications of hydrogel ionotronics (HIs) in devices and machines require them to maintain their robustness under complex mechanical environments. Nevertheless, existing HIs still suffer from various mechanical limitations, such as the lack of balance between softness, strength, toughness, and fatigue fracture under cyclic loads. Inspired by the structure of the cytoskeleton, this study develops a sustainable HI supported by a double filamentous network. This cytoskeleton-like structure can enhance the strength of the HI by 26 times and its toughness by 3 times. It also enables HI to tolerate extreme mechanical stimuli, such as severe deformation, long-term cyclic loading, and high-frequency shearing and shocking. The advantages of these structurally- and mechanically-optimized HI devices in tactile perception and electroluminescent display, i.e., two practical applications where complex mechanical stimuli need to be sustained, are demonstrated. The findings reported in this study can inspire the design of human skin-like robust and anti-fatigue-fracture HI devices for long-term stable use.
New concepts
Inspired by the structure of the cytoskeleton, this study develops hydrogel ionotronics (HIs) supported by a double filamentous network that consists of bacterial nanocellulose and silk fibroin. This cytoskeleton-like structure can enhance the strength of the HIs by 26 times and its toughness by 3 times. It enables HIs to tolerate extreme mechanical stimuli, such as severe deformation, long-term cyclic loading, and high-frequency shearing and shocking. The advantages of these structurally- and mechanically-optimized HI devices is illustrated in tactile perception and electroluminescent display. To realize tactile perception, a HI-based triboelectric nanogenerator is designed to sense and recognize objects in a similar way to the human finger touch. By combining triboelectric sensing information, machine learning, and Internet of Things techniques, the HI-based triboelectric nanogenerator can accurately recognize different materials among a diverse set of irregular and sharp objects, and further deliver them to the designated location. The classification accuracy is as high as 87.7% in 600 sorting tests. HI electrodes are also integrated with an emissive layer to achieve electroluminescent display, which can be stretched, kneaded, and folded and work normally in both hydrous and freezing environments. These results provide confidence for the future application of HIs in human-machine interfaces, soft robots, and ultra-flexible displays.
|
Introduction
Hydrogel ionotronics (HIs), which consist of polymer networks, water, and ions, have been attracting considerable attention by virtue of their versatility in electrical, mechanical, and biofunctional engineering.1 More specifically, due to their similarities to biological tissues in terms of mechanical and electrical features, HIs have been proposed as a potential bridge between living matter and electronic devices, and have been applied in the development of bioelectronic interfaces,2–6 artificial skins,7–10 artificial nerve fiber,11 and soft robotics.12–15 In these applications, HIs are required to maintain their physical integrity throughout their life span. In some cases, e.g., when used for artificial skins and soft robotics, HIs are required to carry significant mechanical loads and/or undergo substantial deformation.16,17 These loads and deformations can be either static or dynamic as well as continuous and instantaneous.18–20 Due to these complex mechanical environments, the mechanical strength, toughness, and fatigue resistance of HIs need to be enhanced in order to ensure the reliability and stability of the device for long-term use. Nevertheless, achieving such mechanical balance remains a significant challenge for HIs. For example, stretchable HIs usually possess low toughness; that is, the material may rupture under low stretch conditions when containing a crack.21–24 In addition, existing tough HIs still suffer from fatigue fracture under multiple cycles of mechanical loading.1,25,26 Such mechanical limitations significantly hinder the long-term application of HIs in devices and machines.
In this regard, inspiration can be derived from cell mechanics. Many eukaryotic cells, such as muscle cells and vascular endothelial cells, possess exceptional mechanical properties and can survive under millions of cycles of mechanical loads in their life span.27,28 Their ability to resist deformation and physical forces depends on their cytoskeleton, an interconnected network of biopolymers including microtubules (MTs), actin filaments (F-actin), and intermediate filaments (IFs).29 Due to the large persistence length of MTs (∼5 mm) and highly crosslinked configuration of F-actin, the networks consisting of MTs and F-actin are stiff, strong, and tough, enabling the cells to resist disintegration and regulate their shape and migration at small deformations.29 On the other hand, cytoplasmic IF networks play an important role in maintaining the mechanical integrity and resilience of cells under large deformations, since both MTs and F-actin yield or disassemble under moderate strain levels (20 and 60%, respectively).30 IF networks are random, stretchable, and hyperplastic structures, which work synergistically with other cytoplasmic components and effectively disperse local deformation in the cytoplasm. Consequently, they lead to a significant increase in the mechanical energy input required to damage the cytoplasm, substantially enhancing the strength, stretchability, resilience, and toughness of the cells.31,32 Moreover, hyperplastic IF networks can dramatically decelerate both the poroelastic and viscoelastic relaxation processes in the cytoplasm, which can enhance its mechanical damping capability and thus protect organelles against mechanical damage.33
With this inspiration, this study proposes a double network structure composed of bacterial cellulose nanofibrils (BCNFs) as the stiff and strong framework and flexible silk fibroin as the percolating phase to address the problems related to the stretchability, strength, stiffness, and toughness of HIs. This double network structure integrates fiber-reinforcement at the mesoscale and hybrid crosslinkers at the nanoscale, consistently providing high fracture energy under various environments and different loading conditions. For instance, the fracture energy of the proposed cytoskeleton-inspired HI (CIHI) is hundreds of times higher than that of single-network hydrogels.34–36 These mechanical advances have enabled CIHIs to be applied in various complex mechanical environments, e.g., severe deformation, long-term cyclic loading, and high-frequency shocking. The proposed CIHI was further used in two applications, i.e., a triboelectric nanogenerator and a hyperflexible electroluminescent skin, which can represent the mechanical complexity in which ionotronic devices are used. In particular, a triboelectric nanogenerator is required to tolerate millions of high-frequency pressure cycles in one day. On the contrary, the hyperflexible display must withstand long-term static and dynamic tensile stresses and maintain a normal display even in the presence of localized damage. The results confirm that, compared to previously reported HIs, the proposed structurally and mechanically optimized CIHI was able to significantly extend the lifetimes of both devices and resolve issues associated with flaw sensitivity, degradation under cyclic loading, fatigue damage, and fatigue fracture. These encouraging results provide confidence for the future application of CIHIs in human-machine interfaces, soft robots, and ultra-flexible displays.
Results and discussion
CIHI design and fabrication
Although HI can be prepared by combining various materials, structural support and metal ions are often required. Among them, the structural support provides the mechanical and physiological properties, while the metal ions primarily provide the ionic and electric conductivity.37 Therefore, in order to enhance the mechanical tolerance and balance the mechanical strength and toughness of HI, the structural design was first conducted to mimic the double network structure of the cytoskeleton. As it can be seen in Fig. 1A, BCNFs were chosen to mimic the structural frame that consists of F-actin and MTs, since both have high similarity in each structural hierarchy. For example, at the single nanofibril scale, the diameter and contour length of BCNF and F-actin are comparable, both exhibit semiflexible features, and have lengths of 1.3 μm and 1.2 μm, respectively (Fig. S1A, ESI†). In addition, similar to F-actin in the cytoskeleton, BCNFs are highly interwoven in the aerogel (Fig. S1B and C, ESI†). This structure promotes the assembly of highly organized and stiff structures, such as isotropic, bundled, and branched networks. Moreover, these networks exhibit similar fractal structures and dimensions to the network formed by F-actin and MTs (Fig. S1D, ESI†). Accordingly, similar to the F-actin in the cytoskeleton, these BCNFs can preserve the structural integrity of HIs. Besides, most crosslinks in the BCNF network are a physical entanglement among BCNFs. These crosslinks can be dis-entangled and re-entangled, and the BCNFs among crosslinks can be oriented and disoriented under external mechanical stimuli. Therefore, the BCNF network is stretchable and can sustain external loads.
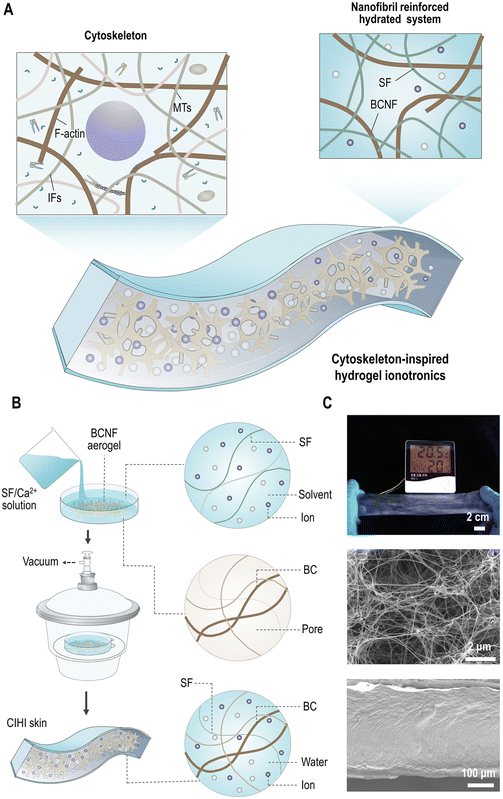 |
| Fig. 1 CIHI design and fabrication. (A) Schematic of the design principle of the CIHIs. (B) Schematic illustration of the two-step strategy used to fabricate CIHI. (C) Photograph and SEM images of the produced CIHI: (top) photograph demonstrating the transparency and conductivity of CIHI; (middle) cross-sectional SEM image of the BCNF aerogel; (bottom) cross-sectional SEM image of the CIHI. | |
Subsequently, silk fibroin (SF) was selected to mimic the flexible IF network of the cytoskeleton. As a fibrous protein, SF has an inherent molecular network structure which is composed of heavy chains (391.6 kDa), light chains (27.7 kDa), and glycoprotein P25 (30 kDa).38 In this molecular network, the heavy and light chains are crosslinked through a covalent disulfide linkage. The P25 protein connects the heavy and light chains through hydrogen bonding.38 The SF molecular networks contain both permanent covalent crosslinks and relatively weak and reformable noncovalent crosslinks. Thus, sharing similar functions with IF networks, they can preserve the integrity of HI under extreme deformations. The stretchability of SF under hydrated state39 also allows HI to effectively disperse locally-induced mechanical stresses to larger regions within HI, enabling the dissipation of energy throughout the material.
In addition to mechanical reinforcement, the electric and ionic conductivity should be taken into consideration in the design of HIs. Ionic conductors can be solid electrolytes,40 ionic liquids,41,42 or salts.43,44 In this work, calcium chloride (CaCl2) was selected, since it can be well dispersed in the SF network due to that the formic acid (HCOOH or FA)/CaCl2 solution is a good solvent for SF.45 Furthermore, Ca2+ ions can be well immobilized on the silk protein through chelation. In addition, they have a strong capability to capture water molecules from the surrounding environment through coordination interactions; e.g., a Ca2+ ion can coordinate about 6–8 water molecules.46,47 Hence, the strong hygroscopicity of Ca2+ ions is very important for both the electrical conductivity and stretchability of HIs. For salt-based HIs, the conductivity depends on both hydrated proton and ion conduction.48 As a result, the conductivity of HI is positively correlated with the hydration degree of the metal ions. Moreover, the water molecules and hydrated metal ions are plasticizers of SF; thereby, they can enhance the stretchability of the HI. All in all, CIHI is a biomimetic system with structural support and electrical conduction provided by a filamentous double network and hydrated salt ions, respectively.
Fig. 1B demonstrates the two-step strategy followed to fabricate CIHI. First, BCNF aerogel samples with desired dimensions were fully immersed in SF/HCOOH/CaCl2 solution (weight ratio of 3/30/1). The detailed methods for the production of the BCNF aerogel and the SF/HCOOH/CaCl2 solution can be found in the Experimental section. Subsequently, the resultant system was transferred to a desiccator in order to perform vacuum-assisted solution permeation. In this process, the negative pressure caused by the vacuum drives the SF/HCOOH/CaCl2 solution to penetrate into the BCNF network, forming a mesoscopic dense and uniform structure. By this strategy, large-scaled and homogenous HIs can be produced. In addition to these intrinsic features, such as conductivity, stretchability, and conformability (Fig. S2, ESI†), the cytoskeleton-inspired double network structure endows CIHI with mechanical advances beyond those of HIs consisting of single-molecule networks. As it can be observed in Fig. 1C, CIHI is soft in appearance and feels like human skin while being mechanically robust and ductile. For example, a single piece of CIHI with a weight of 171 mg and length of 5 cm could hold a 1 kg weight without breaking (Fig. 2A). For comparison, most HIs with single-molecule networks, such as SF/CaCl2,44 chewed gum/NaCl,49 glutinous rice/NaCl,50 gluten/NaCl (K2CO3),51 and dopamine (sulfobetaine methacrylate),52 exhibit a gum-like viscous state. This means that a small tensile load can produce large deformation, which cannot be recovered when the tensile load is removed.
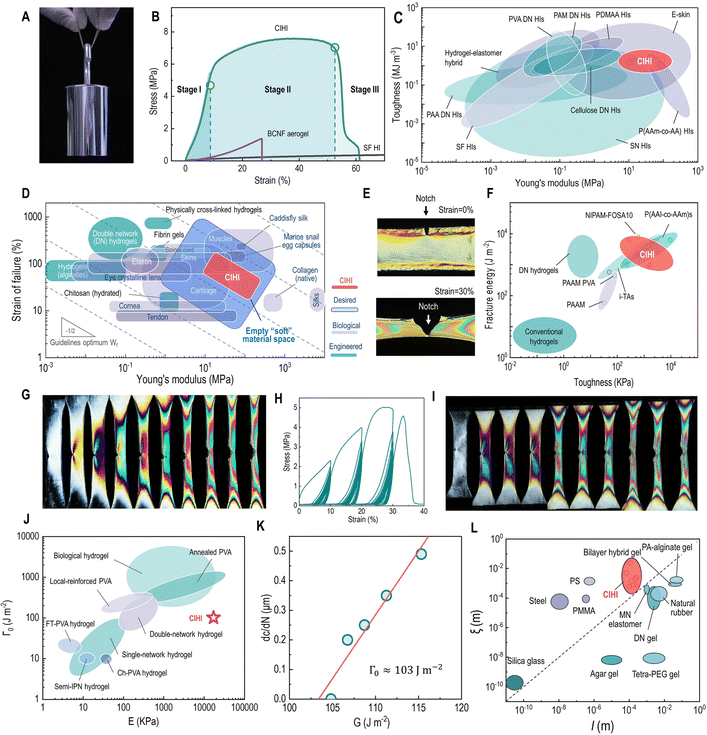 |
| Fig. 2 Mechanical properties of CIHI. (A) Photograph of a CIHI sample bearing a 1 kg weight without breaking. (B) Tensile stress–strain curves of SFHI (black), hydrated BCNF aerogel (purple), and CIHI (green). (C) Ashby plot of the Young's modulus vs. toughness of CIHI and other representative materials. (D) Comparison of the Young's modulus and failure strain of CIHI with those of other soft engineered and biological materials. The Ashby plot of representative materials has been adapted with permission.67 Copyright 2014, Royal Society of Chemistry. (E) Polarized images of a pre-notched CIHI at the strains of 0% and 30%. (F) Ashby plot of the fracture energy and toughness for CIHI and other tough hydrogel materials. (G) Polarized images of a pre-notched CIHI sample during tensile testing. (H) Stress–strain curve of the pre-notched sample obtained under three-strain-stage cyclic stretching. The maximum strains in these three stages were 10%, 20%, and 30%, respectively. (I) Polarized images obtained during the three-strain-stage cyclic stretching tests. (J) Ashby plot of the Young's modulus E and Γ0 for CIHI and other representative soft tough materials. The Ashby plot of representative materials was adapted with permission.71 Copyright CC BY 4.0. (K) Fatigue threshold Γ0 of CIHI. (L) Ashby plot of the length scale of l and ξ for CIHI and other representative soft tough materials. The Ashby plot of representative materials was adapted with permission.74 Copyright 2021, Annual Reviews. | |
Mechanical performance
Quasi-static tensile tests were then conducted to quantitatively assess the mechanical performance of CIHIs. As illustrated in Fig. 2B, the CIHIs exhibited a typical ductile stress–strain curve consisting of a pre-yield stage (from 0 to the yield point), a post-yield stage (from the yield point to the failure point), and a ductile fracture stage (from the failure point to the zero-stress point). The occurrence of these three stages is closely associated with the network structure in the CIHI. More specifically, the pre-yield phase is attributed to the glassy-like elastic response of the stiff BCNF network to the initial external load. At this initial stage, the tensile force exerted on CIHI is limited; thus, only the relatively rigid BCNF structural frame can sense these mechanical stimuli. In this case, the external force is not sufficient to cause noticeable topological changes to the rigid BCNF network. Therefore, at this stage, the structural and mechanical response is recoverable. In addition, a careful examination of the profiles of the stress–strain curves revealed that, at this stage, they exhibit a J-shape. This indicates that, initially, a small increase in the stress results into large deformation. However, as the strain increases, the material becomes stiffer and more difficult to extend. Human skin and other high-performance biomaterials, such as polyacrylic acid (PAA)/betaine,53 polyacrylamide (PAAm)/polyethylene fibers,54 alginate,55 alginate/poly(acrylamide-co-acrylic acid),56 and PVA,57,58 also feature J-shaped stress–strain curves. Moreover, J-shaped stress–strain curves are also typical of tough materials. This is because the first portion of the J-shaped curve corresponds to a large extension for low applied stress; thus, the Young's modulus in this region is very low.59
After yielding, the BCNF network structure can deform and re-orient to withstand the further increase of tensile loads. Simultaneously, as a viscoelastic body, significant viscoelastic deformation occurs in the SF network to dissipate the external stress and restrain or conform to the structural remodeling of the BCNF frame. The combined effects of the BCNF and SF networks drive the CIHI to enter the equilibrium stage. For single-network HIs, no matter elastic or viscoelastic, once the tensile strain reaches the failure point, i.e., the strain where macroscale defects are generated, the material undergoes catastrophic failure, and the tensile stress drops abruptly to zero. On the contrary, CIHI exhibits the representative gradual failure characteristics of ductile fracture (Fig. 2H and I). More specifically, even when macroscopic defects occur, the sample can still gradually carry the external loads and fails with increasing strain. The human skin and other soft tissues, as well as fiber-reinforced materials have also been reported to have similar fracture characteristics.60–63 The synergic effects of the (i) gradual fracture of the fibrous network, (ii) limited slippage and pull out of the filaments, and (iii) viscous resistance of the matrix are critical factors that contribute to this tough fracture behavior. This elegant ductile fracture mode is desirable for HI devices, since it can prevent the catastrophic functional failure of the device.
By comparing the mechanical properties of the CIHI and the BCNF aerogel, it was confirmed that the strengthening and toughening effects were induced by the synergy of the double network structure. According to Fig. 2B and Table S1 (ESI†), the failure strength (7 ± 2 MPa), Young's modulus (74 ± 33 MPa), and toughness (1.8 ± 0.8 MJ m−3) of the CIHI were 5-, 27-, and 11-fold higher than those of the BCNF aerogel, respectively. In addition, compared to other commonly used HIs, CIHI maintained an elegant balance between stiffness, strength, stretchability, and toughness. For instance, although single-network HIs, such as SF/CaCl2,44 glutinous rice/NaCl,50 chewed gum/NaCl,49 PAM/LiCl,64 and PAA/LiCl/Fe(NO3)3,65 can be stretched to 2–23 times their original length, their Young's modulus and yield strength are only about 3 × 10−4–4 MPa and 5 × 10−4–0.6 MPa, i.e., 2.4 × 105–20 and 8.0 × 103–10 times lower than those of CIHI, respectively. By contrast, the Young's modulus of P(AAm-co-AA) HIs66 can reach 0.29 GPa, while its toughness is only 9.7 kJ m−3; i.e., ∼180 times lower than that of CIHI (Fig. 2C). When the mechanical properties of CIHI are compared with those of other biological and engineered material systems (Fig. 2D), it can be found that its mechanical performance matches well with that of several soft tissues (e.g., skin, muscles, and cartilages) and biological materials (e.g., keratin, collagen, and actin).67 Consequently, the tissue-like mechanical feature of CIHI indicates its potential application in fields associated with biofunctional engineering, such as artificial skins, human-machine interfaces, and soft robots, especially since CIHI fills up nicely the empty space of “soft” engineered biomaterials.
Fracture toughness
The fracture toughness of the CIHI was quantitatively evaluated by the work of the rupture U (area under the engineering σ–ε curves in Fig. 2B) and the fracture energy obtained from tensile tests of single-edge notched specimens (Fig. S3, ESI†). The obtained values for the CIHI, i.e., U ∼ 1700 kPa and Γ ∼ 3300 J m−2, were both around 50 folds larger than those of single-network hydrogels and similar to those of existing tough hydrogels (Fig. 2F).68 The toughening induced by the double network structure was confirmed by the crack blunting of the CIHI. As it can be observed in Fig. 2G, when the pre-notched specimen was stretched, initially, a butterfly-shaped stress concentration pattern appeared at the crack tip. Nevertheless, this stress concentration pattern disappeared gradually as the stretching progressed, and the tensile and shear stresses were uniformly distributed over the entire specimen. This observation is in stark contrast to the rapid forward propagation of the butterfly crack tip observed in brittle materials (Fig. 2E).
The contribution of the stretch-induced structural remodeling of the BCNF network to the fracture toughness was clearly revealed in the high-resolution synchrotron small-angle X-ray scattering (SAXS) mapping (Fig. 3). Under low strain conditions, the BCNFs in the process zone and other zones exhibited almost the same orientation degree, i.e., Bψ = 60–70. In this case, the orientation can be attributed to the directional growth of ice crystals during the preparation of the BCNF aerogel. With the increase of the tensile strain, it was found that the orientation degree of the BCNFs in the near-crack-tip zone and the unnotched area increased progressively and was significantly higher than that in the left and right regions of the crack. This is mainly due to that the tensile stress is principally applied to the near-crack-tip zone and the unnotched area. During this process, the external stress causes the specimen to stretch longitudinally and shrink laterally, forcing the BCNF network to re-orient along the stretching direction. Such structural remodeling events can dissipate a large amount of fracture energy. Moreover, the number of filaments per unit area in the near-crack-tip zone increases, and the long axis of these filaments is perpendicular to the crack propagation direction. This configuration further increases the resistance to crack propagation and can even induce a longitudinal transfer of the crack tip. In addition, the presence of viscoelastic SF among BCNFs also increases the resistance to crack propagation, since under this condition, the fracture energy can be dissipated through restricted slippage. These synergistic effects result in a significant blunting of the initial crack during the tensile process.
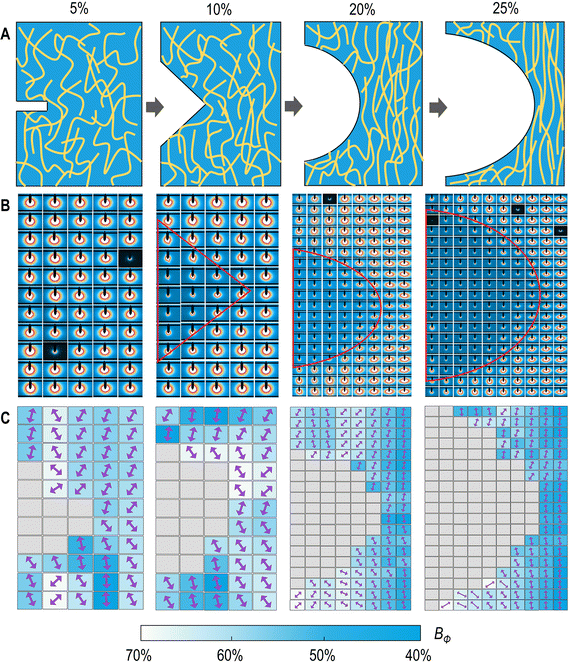 |
| Fig. 3 Structural evolution of a pre-notched CIHI sample during stretching as detected by synchrotron SAXS mapping. (A) Schematic illustration of the CIHI structure evolution around crack under different tensile strain levels. (B) SAXS mapping around the crack of pre-notched CIHI under different tensile strain levels. The red line indicates the location of the crack edge. The longitudinal mapping spatial resolution at the strains of 5% and 10% is 100 μm, and at the strains of 20% and 25% is 200 μm. The horizontal mapping spatial resolution for all strains is 200 μm. The weak patterns in (B) correspond to the signal of air, and the patterns with sharp split peaks correspond to the signals of the interface between air and sample. (C) Heatmap of the orientation distribution (B∅) of CIHI around the crack under different tensile strain levels. The arrows in each pixel indicate the orientation direction of the filamentous and molecular networks. In general, the smaller the B∅, the higher the orientation degree. The near-white pixels indicate that no apparent orientation distributions were found. The arrows inside those pixels cannot directly reflect the orientation directions of the contained filamentous and molecular networks. | |
The fracture behavior of highly deformable soft materials can be compared using two length scale parameters; that is, the elasto-adhesive length l and the crack-tip load-transfer length ξ (Fig. S4, ESI†).69 More specifically, l represents the typical distance from a crack tip, below which the deformation is dominated by elastic nonlinearity at the onset of crack initiation. It can be calculated by:
where
Γ is the fracture energy and
E is the Young's modulus. On the other hand,
ξ represents the size of the regions around the crack tip where the stress or strain concentration is wiped out,
70 and it can be calculated by:
where
W* is the critical energy per unit volume for material failure. The detailed methods for calculating
l and
ξ can be found in the ESI.
† By comparing these two length scales with the crack size
c, different soft materials can be classified according to their mechanical fracture properties. For instance,
l ≪
ξ ≪
c indicates stiff brittle materials;
l ≪
ξ ∼
c indicates stiff ductile materials;
ξ ≪
l ≪
c indicates brittle materials such as brittle hydrogels; and
l ≫
ξ ∼
c indicates soft ductile materials. Through
eqn (1) and (2), the
l and
ξ values of CIHI were calculated to be (1.7 ± 0.7) × 10
−4 m and (7 ± 4) × 10
−3 m, respectively. These values stand on the soft tough areas that are comparable with soft tough materials such as natural rubbers, double-network gels, and bilayer hybrid gels, indicating that CIHI can sustain a large strain at failure (
Fig. 2L).
The fatigue fracture behavior of CIHI also evaluated through cyclic tensile loading-unloading tests,71 which were performed with unnotched and single-edge pre-notched samples. During cyclic loading, a triangular loading profile was applied (Fig. S5, ESI†). The maximum stretch was kept constant at λmax, while the minimum stretch was kept at 1. The fatigue threshold of CIHI evaluated using the single-edge pre-notched approach was found to be 103 J m−2 (Fig. 2K), a value 2–5 folds higher than that of single-network hydrogels and comparable to that of several soft anti-fatigue-fracture materials,68,72 such as double-network hydrogels25,73 and locally reinforced PVA (Fig. 2J).71 The calculation details for the fatigue threshold can be found in the ESI.†
CIHI for tactile perception
The mechanical advances in terms of skin-matching features and fracture resistance enable the CIHI to be applied in various complex mechanical stimuli, such as cyclic stretch, fold, and tap. Two prototypes were prepared using the CIHI. More specifically, the CIHI was assembled into a triboelectric nanogenerator (TENG) for object recognition (Fig. 4A) and an electroluminescent skin (ES) for hyperflexible display. The detailed methods followed to assembly these devices can be found in the methods section. The two devices represent the mechanical complexity in which HI devices are used. For example, the TENG is required to tolerate millions of high-frequency pressure cycles within one day. On the other hand, the hyperflexible electroluminescent display is required to withstand long-term static and dynamic tensile stresses, and maintain a normal display even in the presence of localized damage. As it can be observed in Fig. 4B and Fig. S6 (ESI†), the CIHI-TENG maintained its structural integrity and triboelectric stability after 100
000 flapping-type contact-separation tests. Deconvolution results disclose that the content of β-sheet in silk portion was increased from the initial 1.7% to 4.5% (Fig. S7, ESI†) after long-term operations. More remarkably, the triboelectric performance of the CIHI-TENG was also maintained when the CIHI-TENG was tested under several extreme conditions, such as extreme deformation and high-frequency shocking (Fig. 4C and Fig. S8, ESI†). Furthermore, the triboelectric signals generated by the CIHI-TENG when in contact with different objects are specific (Fig. 4D and E); hence, when the CIHI-TENG was integrated on a humanoid robot hand, through machine learning and the Internet of Things (IoT) techniques, it was able to accurately recognize different materials among a diverse set of spherical objects and further deliver them to their designated location. In particular, the CIHI-TENG exhibited an excellent classification accuracy of 93.9% in 600 tests, where the task was to recognize five types of spherical objects (Fig. 4F and Fig. S9 and Movie 1, ESI†). Such a humanoid robot hand has application potential in garbage sorting, where it may need to recognize various sharp objects, such as ceramics, glass, and DVD shards. To this end, these sharp objects were also used to assess its intelligent sorting performance. Even with these more challenging objects, the classification accuracy of the humanoid robot hand was as high as 87.7% in 600 sorting tests (Fig. 4G and Fig. S10 and Movie 2, ESI†), and the CIHI-TENG remained intact. This proved the effectiveness, high accuracy, and durability of the CIHI-TENG for identification and classification applications.
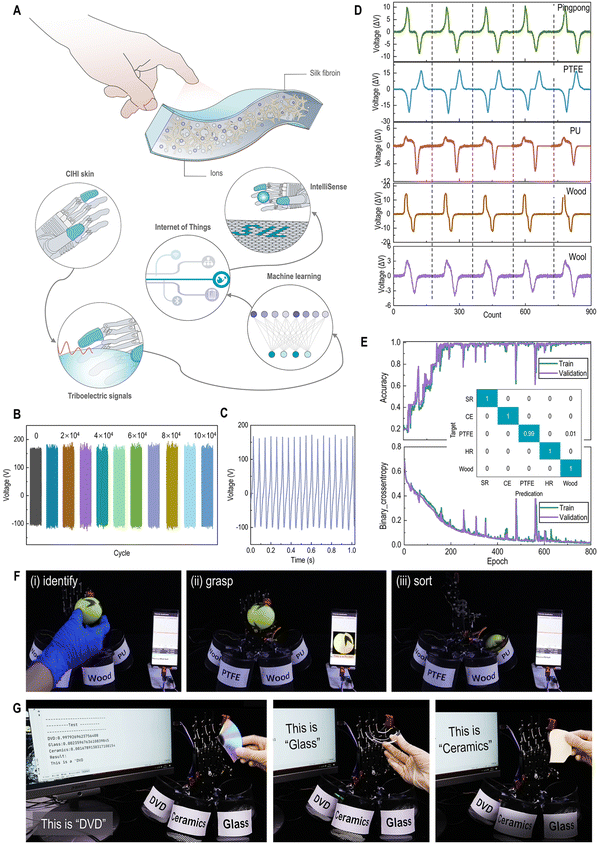 |
| Fig. 4 CIHI for tactile perception. (A) Schematic illustration of the design principle of the CIHI used for object recognition. (B) Triboelectric stability of the CIHI-TENG for 100 000 contact-separation cycles. (C) Output voltage profile of the CIHI-TENG generated under a contact-separation frequency of 16.7 Hz. (D) Voltage variation of a 1 nF capacitor charged by the CIHI-TENG when in contact with different types of ball objects. (E) Training history of the signal classification model. The inset is the confusion matrix of the single-attempt test in actual use. (F) Photographs of the intelligent classification manipulator successfully recognizing a PU ball and delivering it into the corresponding basket. (G) Photographs of the intelligent classification manipulator successfully recognizing sharp pieces of DVD, glass, and ceramics. | |
CIHI for electroluminescent display
The structural design of the CIHI for the electroluminescent display is illustrated in Fig. 5. In this configuration, the CIHI and ecoflex-30/phosphor served as the stretchable electrodes and emissive layers, respectively, and were assembled into a sandwich structure. Subsequently, the upper and lower surfaces of the sandwich structure were encapsulated with transparent very-high-bond (VHB) tape to obtain the final electroluminescent display. As summarized in Table S1 (ESI†), the mechanical stiffness and stretchability of the encapsulation material and the electroluminescent layer were similar to those of the CIHI. Therefore, the obtained CIHI-ES had a flexibility similar to that of CIHI and could be stretched, kneaded, and folded. In addition, it was also conformable to various uneven surfaces, such as the human finger (Fig. S11, ESI†). Moreover, since both the CIHI and the EL layer were frost-resistant and sealed by the hydrophobic tape, the CIHI-ES could still emit light normally in both hydrous and freezing environments (Fig. 6A and B). A series of extreme mechanical stimulation experiments confirmed that the CIHI-ES retained the mechanical advantages of CIHI. For instance, the CIHI-ES was able to normally display during cyclic stretching even in the presence of pre-notches (Fig. 6C), and no obvious crack propagation was observed during stretching for 50 cycles at a strain of 50%. Additionally, neither needling nor rubbing had a substantial effect on the display (Fig. 6D and E and Movies S3 and S4, ESI†). The needling experiment also revealed the self-healing ability of the CIHI-ES. As demonstrated in Movie S3 (ESI†), when the needle pierced the CIHI-ES, the display was interrupted due to a short circuit. However, once the needle was pulled out, the CIHI-ES resumed immediately, even at the area punctured by the needle. Since the brightness of the CIHI-ES display can be adjusted by the excitation voltage (or electric field) which is proportional to the loudness level (Fig. 6F and G), it is easy to produce dynamic displays that change brightness in sync with the rhythm of the music (Fig. 6H and Movie S5, ESI†). Such demonstrations illustrate the great potential of the CIHI-ES in situational interactive displays.
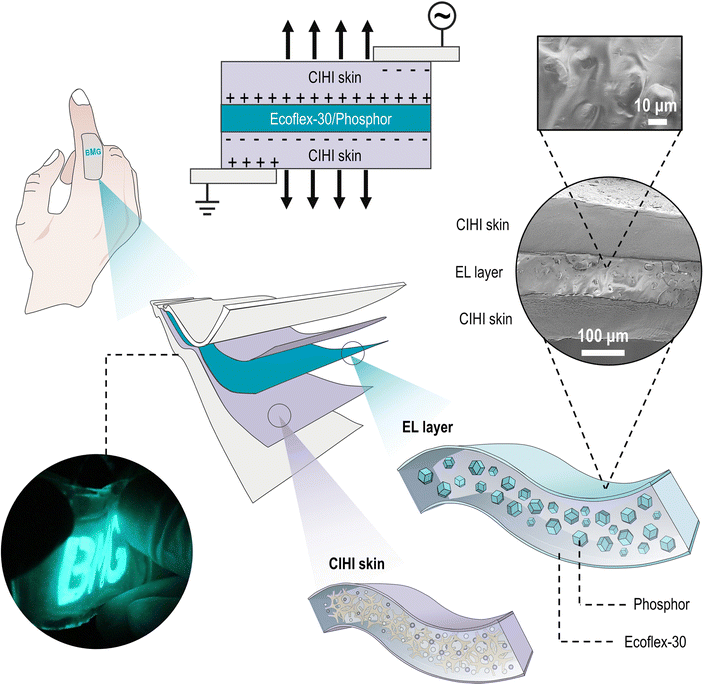 |
| Fig. 5 Working principle, SEM image, and photograph of the CIHI-ES. | |
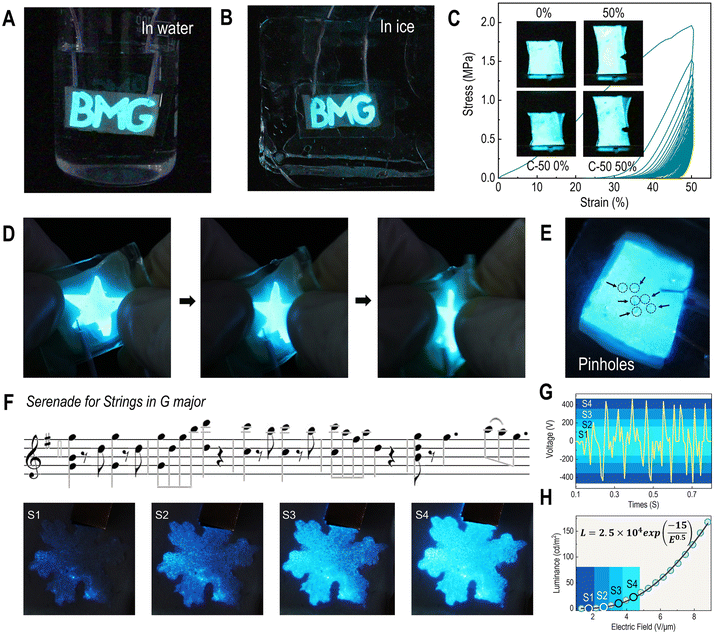 |
| Fig. 6 CIHI for electroluminescent display. (A and B) Photographs illustrating that the CIHI-ES can be illuminated in both water and ice, respectively. (C) Cyclic stress-strain curve of the pre-notched CIHI-ES. The inset photographs depict the CIHI-ES at the 1st and 50th cycle under a tensile strain of 0% and 50%, respectively. (D) Photographs illustrating that the CIHI-ES kept illuminating under rubbing. (E) Photographs illustrating that the CIHI-ES kept illuminating after multiple punctures. The black arrows and circles indicate the pinhole positions. (F) Photographs of CIHI-ES with different brightness levels corresponding to different sound levels (S1–S4) in the broadcasting of music “Serenade for Strings in G major”. (G) Correspondence between sound level and voltage applied to CIHI-ES. (H) Relationship between the brightness of the CIHI-ES and the excitation voltage. | |
Conclusions
In summary, this study introduced a biomimetic strategy to develop HIs for overcoming the mechanical limitations related to the lack of balance between softness, strength, toughness, and fatigue fracture under cyclic loads. The proposed biomimetic HI has a cytoskeleton-inspired double filamentous network structure, which has been confirmed to be critical for enhancing the strength, stretchability, resilience, and toughness of cells. Benefiting from this unique structure, strong and tough CIHIs can be obtained with a fracture strength 26 times higher than that of single-network HIs. In addition, the produced CIHIs can tolerate a variety of extreme mechanical stimuli, such as severe deformation, long-term cyclic loading, and high-frequency shearing and shocking. Such structural and mechanical advantages enable CIHIs to successfully overcome the structural instability of HI devices in tactile perception and electroluminescent display applications, where complex mechanical stimuli need to be sustained. Combining the mechanical advantages of CIHIs with the inherent properties of HIs, such as antifreeze, flame retardant, and self-healable, to fabricate devices that can be used under various extreme environments is of particular interest. This is due to that the primary applications of HIs, e.g., as human-machine interfaces and electronic skins, require them to withstand various harsh conditions, such as ultra-low or ultra-high temperatures, high humidity, and extreme dryness. The resolution of these challenges can undoubtedly facilitate the practical application of HIs in such emerging fields.
Experimental section
BCNF aerogel preparation
BCNF papers (purchased from Guilin Qihong Technology Co. Ltd., China) with a size of 32 cm × 26 cm × 0.03 cm were first boiled in 2 wt% NaOH aqueous solution for 2 h to remove acetobacter xylinum and other impurities. Then, the BCNF papers were washed using deionized (DI) water until their pH was close to 7. Thereafter, the purified and highly swollen BCNF papers were placed into liquid nitrogen for freezing. Finally, the BCNF aerogel was obtained by the freeze-drying of the frozen BCNF papers at −47 °C for 2–3 days.
SF/CaCl2/FA solution preparation
First, the sericin of Bombyx mori silkworm cocoons was removed through degumming processing. More specifically, 20 g cocoons were added into 4 L DI water with 20 g NaHCO3 and boiled for 30 min; this process was repeated one more time to thoroughly remove the sericin. Second, the degummed silk fibers were washed with DI water and then dried in a blast oven at 60 °C overnight. Third, 3 g of degummed silk fibers were dissolved in 30 g CaCl2/FA solution (1/30 wt/wt), followed by vigorous stirring at room temperature to obtain the SF/CaCl2/FA solution. Finally, the insoluble impurities were removed by gauze filtration.
CIHI and silk fibroin ionotronics (SFI) preparation
A 5 cm × 5 cm BCNF aerogel sample was immersed into 30 g of the SF/CaCl2/FA solution. Subsequently, the resultant solution was transferred into a sealable container for vacuum processing at room temperature for 5 min. The negative pressure enabled the SF/CaCl2/FA solution to gradually penetrate into the BCNF aerogel and obtain the BCNF/SF ionogel. Thereafter, the ionogel was removed from the container and placed in a laboratory fume hood at room temperature to evaporate the FA. After the FA was thoroughly removed, CIHI was obtained. The thorough removal of FA was confirmed by Fourier transform infrared spectroscopy (Fig. S12, ESI†). As regards the SFI, its preparation protocol has been well established.44 In brief, 30 g of the SF/CaCl2/FA solution prepared in the previous step were directly cast onto a polystyrene Petri dish with a diameter of 150 mm, allowing FA to evaporate thoroughly at room temperature. Since FA is evaporable at room temperature, all steps were conducted in a chemical fume hood with the necessary precautions.
CIHI for intelligent sorting
The adhesion strength of CIHI to Cu electrode and Acrylic elastomer was 2.6 and 3.3 MPa, respectively (Fig. S13, ESI†), providing a stable connection during long time usage. CIHI was bonded with a dielectric Acrylic elastomer-PE film to obtain a single electrode (CIHI-TENG). Acrylic elastomer-PE film (Shenzhen Nade Adhesive Technology Co., Ltd) can also seal the CIIS to avoid its direct contact with open air. CIHI-TENG was further glued on the index finger of a raspberry Pi manipulator for object identification. This was realized by detecting and analyzing the triboelectric signals generated by the contact and separation processes between CIHI-TENG and different objects. The signal was detected by a single-chip microcomputer, which was used to collect the triboelectric signals and transmit them to a Python program on a computer or phone application through a serial port. Signal analysis was performed using a signal-classification machine learning (ML) model, which was compiled with TensorFlow (2.3.1) and Python (3.7.4). The Keras Sequential model comprised 11
447 trainable parameters and 360 nontrainable parameters. The model received signals composed of 180 sampling points and output a 5-digit code representing each material. More details about the acquisition of 180 data points can be found in Supplementary Note 2 in the ESI.† A batch normalization layer was used at the beginning of the model to standardize the input signal, followed by two dense layers and three long short-term memory (LSTM) layers. To avoid overfitting, several dropout layers were added as well. During the training process, Adam was chosen as the optimizer with its default parameters, and binary cross-entropy was chosen as the loss function. This ML model was compiled into a Python program. Thus, when the Python program received the triboelectric signals generated from the CIHI, it further extracted these signals and input them into the ML model for learning and prediction based on the established training set; thereby, the material of the objects (ping-pong, PU, PTFE, rubber, wool, wood, DVD, ceramics, glass) in contact with the CIHI could be identified. Finally, the predicted information was fed back to the Raspberry Pi manipulator to perform the subsequent classification tasks.
CIHI electroluminescent skin (CIHI-ES) assembly
The electroluminescent (EL) layer was fabricated by mixing EL phosphor powder (ZnS
:
Cu) with Ecoflex 00–30 (50 wt% phosphor). Subsequently, spin-coating was conducted at 1000 rpm for 60 s to apply the mixture on a plastic substrate, which was followed by curing at room temperature for 1 h. To strengthen the interface bonding with CIHI, both surfaces of the EL layer were treated with plasma cleaning at 10 W for 40 s. The EL layer and two CIHI electrodes were assembled into a sandwich structure, which was encapsulated with transparent VHB tape (Shanghai Haotie Electronic Technology Co., Ltd) to avoid the impact of the environment and obtain the final EL display. A copper wire was inserted into each electrode and connected to an external power source to enable light emission.
Tensile, toughness, and fatigue tests
All tensile tests were performed on an Instron 5966 tensile testing machine. Unnotched dumbbell-shaped samples (50 mm × 4 mm × 0.6 mm) were stretched to failure. The fracture energy of CIHI was obtained from a pure shear test using dumbbell-shaped samples with an initial notch of 0.1 mm. During the experiment, the temperature was kept constant at 25 °C, and the nominal displacement rate was 10 mm min−1. Fatigue tests were performed mainly using the dumbbell-shaped samples (50 mm × 4 mm × 0.6 mm) with an initial notch of 0.1 mm at a nominal displacement rate of 50 mm min−1. A polarizing optical system (Fig. S14, ESI†) was applied to observe the stress distribution during the fatigue tests. During these tests, the temperature was kept constant at 25 °C, and the nominal displacement rate was 200 mm min−1.
Material characterization
The microstructure of the materials was characterized using a scanning electron microscope (SEM; JEOL JSM-7800F, Japan). Samples sprayed with platinum were observed at an accelerating voltage of 1 kV. Small-angle X-ray scattering (SAXS) experiments were conducted at the Shanghai Synchrotron Radiation Facility on a BL19U2 beamline with a wavelength of 1.03 Å. The beam size was set to 0.3 mm (horizontal) × 0.2 mm (vertical). The samples (50 mm × 4 mm × 0.6 mm) with a 1 mm-deep notch were stretched to 5%, 10%, 20%, and 25% and the orientation around the crack was scanned line-by-line by the SAXS technique. The humidity of the testing environment was 75% RH. The triboelectric performance was assessed by using 3 × 3 cm2 CIHI-TENG samples with thickness of 1 mm. Structure diagram of the assembled CIHI-TENG is illustrated in Fig. S6A (ESI†). A CIHI-ES sample (5.4 cm × 5.1 cm × 0.98 mm) with a notch of 1 mm was mounted on the machine, and two electrodes were connected to an alternating current (AC) source (Vrms = 157 V; 2.7 kHz). Fatigue measurements were performed at 15 °C and 44% RH, at a displacement rate of 50 mm min−1 and elongation of 50% for 50 cycles.
Author contributions
S. L. supervised and guided the project; C. D., Y. W., Y. S., C. Y., Z. L., and L. C. performed the experiments and analyzed the data; H. Y., S. L., Z. S. and J. L. commented on the manuscript; S. L., J. R., and W. C. discussed the results and commented on the manuscript. All authors have approved the final version of the manuscript.
Conflicts of interest
The authors declare no competing interests.
Acknowledgements
This work was supported by the National Natural Science Foundation of China (no. 51973116, 21935002, 52003156); the starting grant of ShanghaiTech University; Double First-Class Initiative Fund of ShanghaiTech University; and the Research Funds of Institute of Zhejiang University-Quzhou. Materials were tested at Analytical Instrumentation Center (#SPST-AIC10112914), and the Center for High-resolution Electron Microscopy (CℏEM), SPST, ShanghaiTech University. The authors also thank the staff members from BL01B and BL19U2 beamline of National Facility for Protein Science in Shanghai (NFPS) at Shanghai Synchrotron Radiation Facility, BL06B, BL16B1 and BL13HB1 beamline of Shanghai Synchrotron Radiation Facility, BL01B beamline of National Synchrotron Radiation Laboratory in Hefei (NSRL) at University of Science and Technology of China, for assistance during data collection.
References
- C. Yang and Z. Suo, Nat. Rev. Mater., 2018, 3, 125–142 CrossRef CAS.
- M. Amjadi, K. U. Kyung, I. Park and M. Sitti, Adv. Funct. Mater., 2016, 26, 1678–1698 CrossRef CAS.
- D.-H. Kim, R. Ghaffari, N. Lu and J. A. Rogers, Annu. Rev. Biomed. Eng., 2012, 14, 113–128 CrossRef CAS.
- H. Yuk, B. Lu and X. Zhao, Chem. Soc. Rev., 2019, 48, 1642–1667 RSC.
- P. Cai, X. Zhang, M. Wang, Y.-L. Wu and X. Chen, ACS Nano, 2018, 12, 5078–5084 CrossRef CAS PubMed.
- X. Qu, Z. Liu, P. Tan, C. Wang, Y. Liu, H. Feng, D. Luo, Z. Li and Z. L. Wang, Sci. Adv., 2022, 8, eabq2521 CrossRef CAS.
- N. Gogurla, B. Roy and S. Kim, Nano Energy, 2020, 77, 105242 CrossRef CAS.
- B. Xue, H. Sheng, Y. Li, L. Li, W. Di, Z. Xu, L. Ma, X. Wang, H. Jiang and M. Qin, Natl. Sci. Rev., 2021, 9, nwab147 CrossRef PubMed.
- Q. Zhou, J. Lyu, G. Wang, M. Robertson, Z. Qiang, B. Sun, C. Ye and M. Zhu, Adv. Funct. Mater., 2021, 31, 2104536 CrossRef CAS.
- R. Fu, L. Tu, Y. Zhou, L. Fan, F. Zhang, Z. Wang, J. Xing, D. Chen, C. Deng and G. Tan, Chem. Mater., 2019, 31, 9850–9860 CrossRef CAS.
- C. Wang, Y. Liu, X. Qu, B. Shi, Q. Zheng, X. Lin, S. Chao, C. Wang, J. Zhou and Y. Sun, Adv. Mater., 2022, 34, 2270122 CrossRef.
- Y. Zhao, C.-Y. Lo, L. Ruan, C.-H. Pi, C. Kim, Y. Alsaid, I. Frenkel, R. Rico, T.-C. Tsao and X. He, Sci. Rob., 2021, 6, eabd5483 CrossRef PubMed.
- X. Zhang, P. Xue, X. Yang, C. Valenzuela, Y. Chen, P. Lv, Z. Wang, L. Wang and X. Xu, ACS Appl. Mater. Interfaces, 2022, 14, 11834–11841 CrossRef CAS.
- H. Yuk, S. Lin, C. Ma, M. Takaffoli, N. X. Fang and X. Zhao, Nat. Commun., 2017, 8, 1–12 CrossRef PubMed.
- H. Na, Y.-W. Kang, C. S. Park, S. Jung, H.-Y. Kim and J.-Y. Sun, Science, 2022, 376, 301–307 CrossRef CAS PubMed.
- J. Y. Sun, C. Keplinger, G. M. Whitesides and Z. Suo, Adv. Mater., 2014, 26, 7608–7614 CrossRef CAS.
- H. W. Kim, E. Kim, J. Oh, H. Lee and U. Jeong, Adv. Sci., 2022, 9, 2200687 CrossRef CAS.
- H. Huang, L. Han, X. Fu, Y. Wang, Z. Yang, L. Pan and M. Xu, Adv. Electron. Mater., 2020, 6, 2000239 CrossRef CAS.
- Z. Shen, X. Zhu, C. Majidi and G. Gu, Adv. Mater., 2021, 33, 2102069 CrossRef CAS.
- B. Ying, R. Z. Chen, R. Zuo, J. Li and X. Liu, Adv. Funct. Mater., 2021, 31, 2104665 CrossRef CAS.
- Z. Lei, J. Huang and P. Wu, Adv. Funct. Mater., 2019, 30, 1908018 CrossRef.
- J. Huang and P. Wu, Adv. Sci., 2022, 9, 2200108 CrossRef.
- Z. Lei, Q. Wang, S. Sun, W. Zhu and P. Wu, Adv. Mater., 2017, 29, 1700321 CrossRef.
- H. Zhang, W. Niu and S. Zhang, ACS Appl. Mater. Interfaces, 2019, 11, 24639–24647 CrossRef CAS.
- R. Bai, Q. Yang, J. Tang, X. P. Morelle, J. Vlassak and Z. Suo, Extreme Mech. Lett., 2017, 15, 91–96 CrossRef.
- R. Bai, J. Yang, X. P. Morelle, C. Yang and Z. Suo, ACS Macro Lett., 2018, 7, 312–317 CrossRef CAS.
- F. Burla, Y. Mulla, B. E. Vos, A. Aufderhorst-Roberts and G. H. Koenderink, Nat. Rev. Phys., 2019, 1, 249–263 CrossRef.
- B. R. Freedman, N. D. Bade, C. N. Riggin, S. Zhang, P. G. Haines, K. L. Ong and P. A. Janmey, Biochim. Biophys. Acta, Mol. Cell Res., 2015, 1853, 3153–3164 CrossRef CAS.
- D. A. Fletcher and R. D. Mullins, Nature, 2010, 463, 485–492 CrossRef CAS.
- P. A. Janmey, U. Euteneuer, P. Traub and M. Schliwa, J. Cell Biol., 1991, 113, 155–160 CrossRef CAS PubMed.
- E. W. Flitney, E. R. Kuczmarski, S. A. Adam and R. D. Goldman, FASEB J., 2009, 23, 2110–2119 CrossRef CAS PubMed.
- M.-Y. Tsai, S. Wang, J. M. Heidinger, D. K. Shumaker, S. A. Adam, R. D. Goldman and Y. Zheng, Science, 2006, 311, 1887–1893 CrossRef CAS.
- J. Hu, Y. Li, Y. Hao, T. Zheng, S. K. Gupta, G. A. Parada, H. Wu, S. Lin, S. Wang and X. Zhao, Proc. Natl. Acad. Sci. U. S. A., 2019, 116, 17175–17180 CrossRef CAS.
- G. Bao, R. Huo, Z. Ma, M. Strong, A. Valiei, S. Jiang, S. Liu, L. Mongeau and J. Li, ACS Appl. Mater. Interfaces, 2021, 13, 37849–37861 CrossRef CAS.
- J. Zarzycki, J. Non-Cryst. Solids, 1988, 100, 359–363 CrossRef CAS.
- T. Baumberger and O. Ronsin, Biomacromolecules, 2010, 11, 1571–1578 CrossRef CAS PubMed.
-
J. Leger, M. Berggren and S. Carter, Iontronics: Ionic carriers in organic electronic materials and devices, CRC Press, 2016 Search PubMed.
- F. Takei, Y. Kikuchi, A. Kikuchi, S. Mizuno and K. Shimura, J. Cell Biol., 1987, 105, 175–180 CrossRef CAS PubMed.
- T. Shu, Z. Lv, C. T. Chen, G. X. Gu, J. Ren, L. Cao, Y. Pei, S. Ling and D. L. Kaplan, Small, 2021, 17, e2102660 CrossRef PubMed.
- Z. Zhang and L. F. Nazar, Nat. Rev. Mater., 2022, 7, 389–405 CrossRef.
- Y. M. Kim and H. C. Moon, Adv. Funct. Mater., 2020, 30, 1907290 CrossRef CAS.
- W. Li, L. Li, S. Zheng, Z. Liu, X. Zou, Z. Sun, J. Guo and F. Yan, Adv. Mater., 2022, 34, 2203049 CrossRef CAS PubMed.
- J.-Y. Sun, X. Zhao, W. R. Illeperuma, O. Chaudhuri, K. H. Oh, D. J. Mooney, J. J. Vlassak and Z. Suo, Nature, 2012, 489, 133–136 CrossRef CAS.
- Q. Liu, S. Yang, J. Ren and S. Ling, ACS Mater. Lett., 2020, 2, 712–720 CrossRef CAS.
- S. Ling, Q. Zhang, D. L. Kaplan, F. Omenetto, M. J. Buehler and Z. Qin, Lab Chip, 2016, 16, 2459–2466 RSC.
- N. C. Strynadka and M. N. James, Annu. Rev. Biochem., 1989, 58, 951–999 CrossRef CAS.
- A. K. Katz, J. P. Glusker, S. A. Beebe and C. W. Bock, J. Am. Chem. Soc., 1996, 118, 5752–5763 CrossRef CAS.
- S. Yang, Q. Liu, J. Ren and S. Ling, Giant, 2021, 5, 100044 CrossRef CAS.
- B. Cheng and P. Wu, ACS Appl. Mater. Interfaces, 2021, 13, 6731–6738 CrossRef CAS PubMed.
- S. Tian, Y. Xing, Y. Long, H. Guo, S. Xu, Y. Ma, C. Wen, Q. Li, X. Liu and L. Zhang, ACS Appl. Mater. Interfaces, 2022, 14, 5122–5133 CrossRef CAS.
- Z. Lei, J. Huang and P. Wu, Adv. Funct. Mater., 2020, 30, 1908018 CrossRef CAS.
- C. Zhang, Y. Zhou, H. Han, H. Zheng, W. Xu and Z. Wang, ACS Nano, 2021, 15, 1785–1794 CrossRef CAS.
- W. Zhang, B. Wu, S. Sun and P. Wu, Nat. Commun., 2021, 12, 1–12 CrossRef PubMed.
- F.-L. Yi, F.-L. Guo, Y.-Q. Li, D.-Y. Wang, P. Huang and S.-Y. Fu, ACS Appl. Mater. Interfaces, 2021, 13, 32084–32093 CrossRef CAS PubMed.
- Z. Chen, T. Zhang, C.-T. Chen, S. Yang, Z. Lv, L. Cao, J. Ren, Z. Shao, L. Jiang and S. Ling, Mater. Horiz., 2022, 9, 1735–1749 RSC.
- X. Li, H. Wang, D. Li, S. Long, G. Zhang and Z. Wu, ACS Appl. Mater. Interfaces, 2018, 10, 31198–31207 CrossRef CAS.
- S. Lin, J. Liu, X. Liu and X. Zhao, Proc. Natl. Acad. Sci. U. S. A., 2019, 116, 10244–10249 CrossRef CAS.
- J. Luo, S. Li, J. Xu, M. Chai, L. Gao, C. Yang and X. Shi, Adv. Funct. Mater., 2021, 31, 2104139 CrossRef CAS.
-
V. A. Adedayo, Publication name, 2020, 7.
- C. Storm, J. J. Pastore, F. C. MacKintosh, T. C. Lubensky and P. A. Janmey, Nature, 2005, 435, 191–194 CrossRef CAS.
- M. Vatankhah-Varnosfaderani, A. N. Keith, Y. Cong, H. Liang, M. Rosenthal, M. Sztucki, C. Clair, S. Magonov, D. A. Ivanov and A. V. Dobrynin, Science, 2018, 359, 1509–1513 CrossRef CAS.
- M. Wang, R. Li, X. Feng, C. Dang, F. Dai, X. Yin, M. He, D. Liu and H. Qi, ACS Appl. Mater. Interfaces, 2020, 12, 27545–27554 CrossRef CAS.
- D. Zhang, J. Jian, Y. Xie, S. Gao, Z. Ling, C. Lai, J. Wang, C. Wang, F. Chu and M.-J. Dumont, Chem. Eng. J., 2022, 427, 130921 CrossRef CAS.
- C. Larson, B. Peele, S. Li, S. Robinson, M. Totaro, L. Beccai, B. Mazzolai and R. Shepherd, Science, 2016, 351, 1071–1074 CrossRef CAS PubMed.
- Y. Liu, P. Wang, X. Su, L. Xu, Z. Tian, H. Wang, G. Ji and J. Huang, Adv. Mater., 2022, 34, 2108820 CrossRef CAS PubMed.
- M. Wang, P. Zhang, M. Shamsi, J. L. Thelen, W. Qian, V. K. Truong, J. Ma, J. Hu and M. D. Dickey, Nat. Mater., 2022, 21, 359–365 CrossRef CAS.
- A. Miserez, J. C. Weaver and O. Chaudhuri, J. Mater. Chem. B, 2015, 3, 13–24 RSC.
- J. Tang, J. Li, J. J. Vlassak and Z. Suo, Extreme Mech. Lett., 2017, 10, 24–31 CrossRef.
- C. Creton and M. Ciccotti, Rep. Prog. Phys., 2016, 79, 046601 CrossRef.
- Z. P. Bažant, Int. J. Fract., 1997, 83, 19–40 CrossRef.
- S. Lin, X. Liu, J. Liu, H. Yuk, H.-C. Loh, G. A. Parada, C. Settens, J. Song, A. Masic and G. H. McKinley, Sci. Adv., 2019, 5, eaau8528 CrossRef.
- E. Zhang, R. Bai, X. P. Morelle and Z. Suo, Soft Matter, 2018, 14, 3563–3571 RSC.
- W. Zhang, X. Liu, J. Wang, J. Tang, J. Hu, T. Lu and Z. Suo, Eng. Fract. Mech., 2018, 187, 74–93 CrossRef.
- R. Long, C.-Y. Hui, J. P. Gong and E. Bouchbinder, Annu. Rev. Condens. Matter Phys., 2021, 12, 71–94 CrossRef.
|
This journal is © The Royal Society of Chemistry 2023 |