DOI:
10.1039/D3MA00037K
(Paper)
Mater. Adv., 2023,
4, 1927-1934
6H-[1,2,5]Thiadiazolo[3,4-e]thieno[3,2-b]indole-flanked para-azaquinodimethane based aromatic-quinoidal polymer semiconductors with high molecular weights synthesized via direct arylation polycondensation†
Received
18th January 2023
, Accepted 15th March 2023
First published on 17th March 2023
Abstract
The 6H-[1,2,5]thiadiazolo[3,4-e]thieno[3,2-b]indole-flanked para-azaquinodimethane (p-AQM)-based polymer semiconductors PQ-1 and PQ-2 are rationally designed through a π-extended aromatic-quinoidal design strategy. They are synthesized by the direct arylation polycondensation (DArP) method. Their physicochemical properties are investigated. Both polymers show high number-average molecular weights of over 120 kDa. PQ-1 exhibits a narrower band gap compared to its thiophene-flanked p-AQM polymer counterpart. PQ-2, with more aromatic thiophene units in the conjugated main chains, displays a broader band gap compared to PQ-1. Both PQ-1 and PQ-2 possess high-lying highest occupied molecular orbital energy levels (about −5.0 eV). They exhibit typical p-type semiconductor characteristics according to the results of characterization of organic field-effect transistors. This work presents an alternative π-extended aromatic-quinoidal design strategy and a convenient DArP method to build new p-AQM polymer semiconductors.
1. Introduction
Polymer semiconductors have received significant attention because they possess broad application prospects in organic electronics, such as organic field effect transistors (OFETs).1–5 In addition to that of conventional aromatic conjugated polymers, rapid progress in the development of conjugated polymers with quinoidal structures has been noted in recent years.6–9 The interest in the field is being increased because of the possibility of creating conjugated systems that combine the unique properties of aromatic and quinoidal moieties.10–14 The introduction of quinoidal units into the polymer backbones can effectively tune the physicochemical properties of the resulting polymers.15–17
Recently, Liu and co-workers have developed a promising para-azaquinodimethane (p-AQM) building block, which features an N-heteroaromatic quinoidal skeleton and has the ability to stabilize the quinoidal character of the conjugated system.18 The reported thiophene-flanked p-AQMs, which possess a coplanar structure and rigid conformation with limited rotational freedom due to non-covalent intramolecular S⋯N interactions, have generated significant interest in functional polymeric materials.18,19 The thiophene-flanked p-AQMs also exhibit distinct advantages of facile synthesis, ease of insertion into polymer backbones, and flexible band gap engineering.20,21 The fused heteroaromatic conjugated materials have been widely proven to be promising semiconducting small molecules22–28 and polymers.29–33 As a linked unit in the conjugated polymer, replacing the single thiophene ring with the fused heteroaromatic ring endows the resulting polymer with tunable physicochemical and semiconducting properties.34–36 For example, introducing the benzo[c][1,2,5]thiadiazole unit into a thiophene-flanked p-AQM polymer backbone offers improved backbone rigidity, stronger intermolecular interactions, and higher crystallinity, endowing the resulting polymer with high hole transport mobility.37 Therefore, the introduction of the fused heteroaromatic rings in p-AQMs may further expand the molecular design strategies of p-AQM-based conjugated polymers. However, the utilization of fused heteroaromatic ring-flanked p-AQMs for building conjugated polymers remains unexplored.
Another significant consideration for building p-AQM-based polymers is the choice of polymerization method. This kind of conjugated polymer has been still prepared by traditional Stille or Suzuki polycondensations (Fig. 1). The requisite organotin or organoboron monomers represent the limitations of reactions in terms of atom economy and green synthesis. It is encouraging that direct arylation polymerization has become a promising method for preparing conjugated polymers.38–47 However, it is still challenging to achieve solution-processed conjugated polymers with high molecular weights (Mn > 100 kDa).38–50
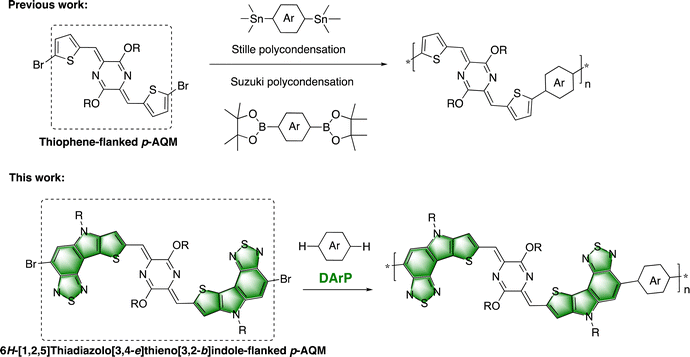 |
| Fig. 1 Design strategies for p-AQM conjugated polymers. | |
In this work, we present an alternative molecular design and synthetic strategy of p-AQM conjugated polymers (Fig. 1). A new fused heteroaromatic ring-flanked p-AQM monomer has been synthesized based on the 6H-[1,2,5]thiadiazolo[3,4-e]thieno[3,2-b]indole building block. This unique monomer has been further introduced into the backbones of p-AQM polymers through the convenient DArP method with thiophene-based units as the comonomers. The physicochemical properties and charge transport properties of the resulting polymers (PQ-1 and PQ-2) have been investigated.
2. Results and discussion
Design, theory calculations, and synthesis
In terms of bromo-6H-[1,2,5]thiadiazolo[3,4-e]thieno[3,2-b]indole (TTI), it is an asymmetric conjugated building unit constructed through the N-bridging strategy of a thiophene and benzo[c][1,2,5]thiadiazole. The TTI-flanked p-AQM is the target monomer M. The long, branched decyl tetradecyl side chains were employed on the periphery of M to support the solubility of its polymers. The density functional theory (DFT) calculation (B3LYP-D/6-31G(d) level) of M was carried out and the resulting optimized conformation is shown in Fig. 2. The dihedral angle between TT1 and p-AQM was 0.2°, indicating the planarity of the conjugated backbone. The synthetic route of the monomer M is shown in Scheme 1. Compound 1 was prepared according to the reported procedure.51 The Stille coupling reaction of compound 1 and tributyl(thiophen-2-yl)stannane provided compound 2 with a yield of 87%. The intermediate 3 was then prepared via Cadogan cyclization and following N-alkylation reactions in a total yield of 43%. The Vilsmeier–Haack reaction of intermediate 3 yielded intermediate 4 with a yield of 88%. Monomer M was obtained by Knoevenagel condensation between 1,4-diacetyl-2,5-diketopiperazine and intermediate 4 and subsequent alkylation in the presence of K2CO3 in a total yield of 48%. It should be noted that the O-alkylated product was gained rather than the N-alkylated one because a tautomerization occurs upon deprotonation of the amide.18–21 The structure of monomer M was assigned by nuclear magnetic resonance, high-resolution mass spectrometry, and infrared spectroscopy (see ESI†).
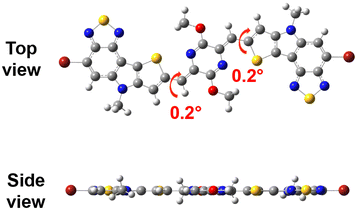 |
| Fig. 2 Optimized molecular geometry of M. Alkyl chains were replaced with methyl groups for simplification. | |
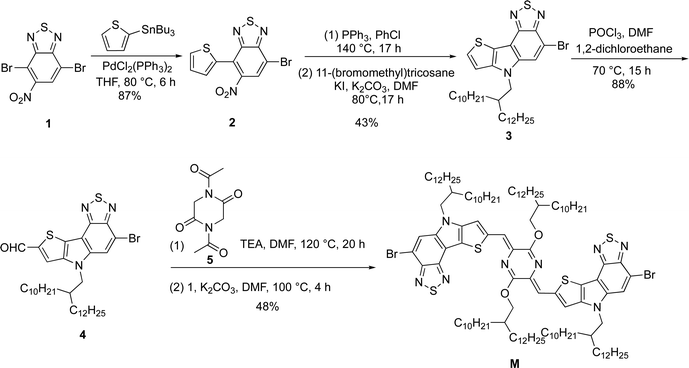 |
| Scheme 1 Synthesis of the monomer M. | |
With monomer M in hand, the 2,2′-bithiophene and 2,5-dithiophen-2-ylthieno[3,2-b]thiophene units were employed as comonomers to build aromatic-quinoidal conjugated polymers (PQ-1 and PQ-2) using DArP methods, respectively. The optimized conformation of the repeating units of target polymers PQ-1 and PQ-2 are shown in Fig. 3. The calculated results showed that they possessed similar calculated orbital electron distribution and energy levels. The model molecules with 2,2′-bithiophene and 2,5-di(thiophen-2-yl)thieno[3,2-b]thiophene bridge units were also calculated. The results showed that they owned relatively good conjugated backbone planarity (Fig. S19, ESI†). Synthetic routes to the polymers are shown in Scheme 2. The detailed synthetic procedures and characterizations are depicted in the ESI.†PQ-1 and PQ-2 were synthesized using Pd-catalyzed DArP of the monomer M and the corresponding comonomers 2,2′-bithiophene and 2,5-di(thiophen-2-yl)thieno[3,2-b]thiophene, respectively. The yield of PQ-1 can reach 96%, while that of PQ-2 was only 44%. The polymerization reaction of M and 2,5-dithiophen-2-ylthieno[3,2-b]thiophene produced an insoluble solid (yield: 46%), meaning that the synthetic yield for PQ-2 is low. It is possible that the 2,5-di(thiophen-2-yl)thieno[3,2-b]thiophene has more beta-protons, and the polymerization to give PQ-2 may have produced more branched structures with partial crosslinking. The polymer PQ-1 displayed good solubilities in tetrahydrofuran, toluene, chloroform and chlorobenzene, and the concentration of the polymer could reach 20 mg mL−1. PQ-2 could only be dissolved in chlorinated solvents, such as chloroform and chlorobenzene, and the concentration of the polymer was less than 10 mg mL−1. The thermogravimetric analysis showed that the temperature of 5% thermal weight loss of PQ-1 and PQ-2 was above 380 °C, indicating good thermal stability (Fig. S15, ESI†). Similar to some thiophene-flanked p-AQM-based polymers,20PQ-1 and PQ-2 showed no obvious phase-transition peaks in the range of 50 to 300 °C. The molecular weights of both polymers were evaluated through high-temperature GPC working at 150 °C. The number-average molecular weights (Mn) of PQ-1 and PQ-2 were calculated to be 155.7 and 128.3 kDa with a dispersity of 2.74 and 2.43, respectively (Table 1 and Fig. S13 and S14, ESI†). To the best of our knowledge, they have the highest molecular weight of this class of p-AQM conjugated polymers.
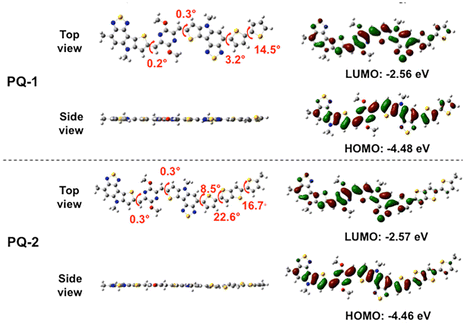 |
| Fig. 3 Optimized geometry, electron cloud distribution, and molecular orbital energy levels of repeating units of PQ-1 and PQ-2. Alkyl chains were replaced with methyl groups for simplification. | |
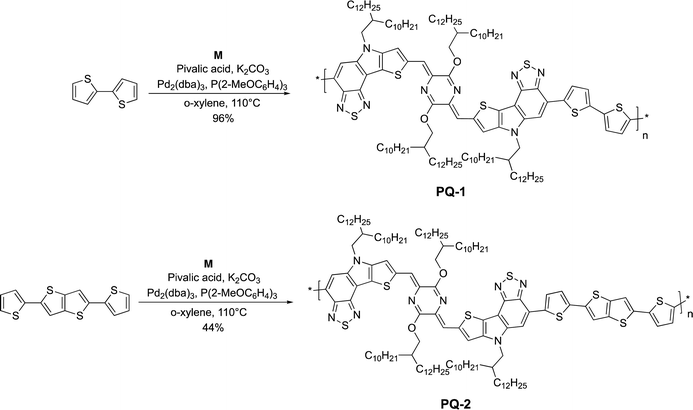 |
| Scheme 2 Synthesis of the polymers PQ-1 and PQ-2. | |
Table 1 Molecular weights and polydispersity index (Đ) of PQ-1 and PQ-2
Polymer |
M
n (kDa) |
M
w (kDa) |
Đ
|
PQ-1
|
155.7 |
426.5 |
2.74 |
PQ-2
|
128.3 |
311.7 |
2.43 |
Optical and electrochemical properties
The optical properties of M, PQ-1, and PQ-2 were investigated by UV-vis absorption spectroscopy (Fig. 4). M was compared to the known thiophene-flanked p-AQM monomer M-ref18 (see Fig. S17, ESI†). The UV-vis absorption spectra of M-ref are depicted in Fig. S17 (ESI†). The M-ref in a dilute chloroform solution showed an absorption maximum at 452 nm, which could be attributed to a π–π* transition. Unlike M-ref, M in the dilute chloroform solution showed an absorption spectrum with two well-resolved peaks at 564 and 610 nm, which could be attributed to the extension of the π-conjugated system, and intramolecular charge transfer caused by brominated 6H-[1,2,5]thiadiazolo[3,4-e]thieno[3,2-b]indole moieties located at the two ends of molecule M. The molar extinction coefficient for the primary absorption of M was four times that of M-ref (Fig. 3a and Fig. S18 (ESI†) and Table 2). In the thin film, the maximum absorption value of M-ref showed little difference from that of M-ref in the dilute chloroform solution, but a broader absorption spectrum. However, M showed a distinct blue-shift in maximum absorption (∼536 nm) compared to its solution, which could be attributed to H-aggregations.52,53 Compared with M, M-based polymers PQ-1 and PQ-2 possessed significantly red-shifted adsorption spectra. Both polymer solutions had high molar extinction coefficients in the visible-light region (Fig. 3a). Both polymer thin films showed broader absorption characteristics as compared with those of their corresponding solutions, indicating stronger interchain interaction in the solid state. The maximum absorption values of the PQ-1 and PQ-2 thin films were 768 and 672 nm, respectively. The optical band gaps of the thin films estimated from the absorption onsets for PQ-1 and PQ-2 were 1.45 and 1.54 eV, respectively. Compared to the PQ-2 thin film, the PQ-1 thin film had more red-shifted absorption and a narrower band gap. The thiophene-flanked p-AQM and 2,2′-bithiophene-based polymer PQ-ref18 (see Fig. S17, ESI†) was chosen as the reference polymer. Compared to PQ-ref, the 6H-[1,2,5]thiadiazolo[3,4-e]thieno[3,2-b]indole-flanked and p-AQM and 2,2′-bithiophene-based polymer PQ-1 showed a narrower band gap (Table 2).
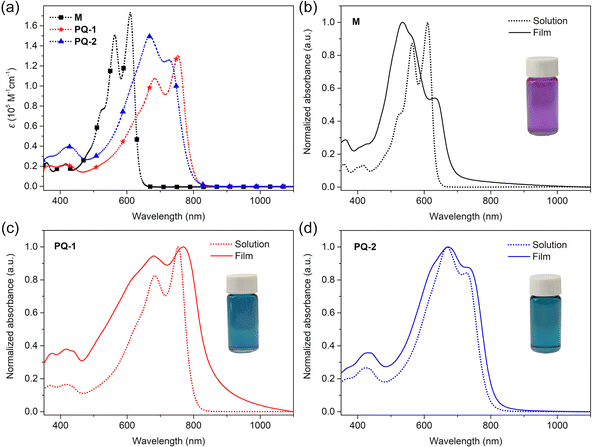 |
| Fig. 4 (a) UV-vis absorption spectra of the chlorobenzene solutions of M, PQ-1 and PQ-2; (b–d) Normalized UV-vis absorption spectra of the chlorobenzene solutions and thin films of M, PQ-1 and PQ-2. | |
Table 2 Optical properties of the monomers and polymers
Material |
ε [105 M−1cm−1] |
λ
solutionmax
[nm] |
λ
solutiononset [nm] |
λ
filmmax [nm] |
λ
filmonset [nm] |
E
optg
[eV] |
Measured in chlorobenzene.
Calculated by the equation: Eoptg = 1240/λfilmonset (eV).
|
M
|
1.7 |
564, 610 |
635 |
536, 632 |
685 |
1.81 |
M-ref
|
0.4 |
452 |
492 |
450 |
498 |
2.49 |
PQ-1
|
1.5 |
684, 752 |
793 |
680, 768 |
858 |
1.45 |
PQ-2
|
1.3 |
668, 728 |
787 |
672, 736 |
805 |
1.54 |
PQ-ref
18
|
0.85 |
731 |
805 |
731 |
810 |
1.53 |
The electrochemical energy levels of M, M-ref, PQ-1, and PQ-2 thin films were evaluated using cyclic voltammetry. The cyclic voltammogram is presented in Fig. 5a. The highest occupied molecular orbital energy levels (EHOMO) and lowest unoccupied molecular orbital energy levels (ELUMO) of each sample were estimated from their respective onset oxidations (Eox) and onset reductions (Ered), respectively. The data are shown in Fig. 5b and Table 3. M had a similar ELUMO compared to M-ref but slightly lower EHOMO (−5.19 vs. −5.14 eV). PQ-1 and PQ-2 possessed similar EHOMO values, slightly higher than the reference polymer PQ-ref18 (see Fig. S17, ESI†). The results were consistent with the theoretical calculation. The electrochemical energy levels of PQ-1 and PQ-2 indicated that both polymers could be potential hole transport materials.
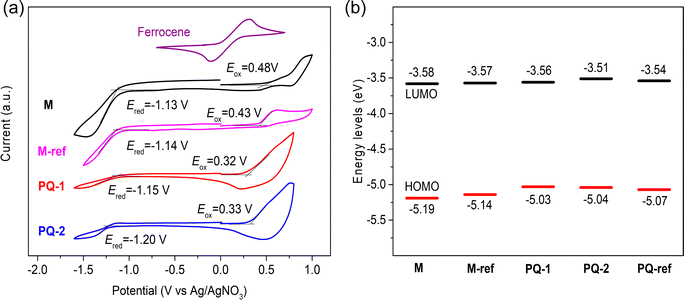 |
| Fig. 5 (a) Cyclic voltammogram of monomers and polymers and (b) energy level diagram of the monomers and polymers. | |
Table 3 Electrochemical properties of M, M-ref, PQ-1, PQ-2 and PQ-ref
Material |
E
red [V] |
E
LUMO
[eV] |
E
ox [V] |
E
HOMO
[eV] |
E
LUMO = −(Ered − EFc/Fc+1/2 + 4.8) eV, EFc/Fc+1/2 = 0.09 V.
E
HOMO = −(Eox − EFc/Fc+1/2 + 4.8) eV.
|
M
|
−1.13 |
−3.58 |
0.48 |
−5.19 |
M-ref
|
−1.14 |
−3.57 |
0.43 |
−5.14 |
PQ-1
|
−1.15 |
−3.56 |
0.32 |
−5.03 |
PQ-2
|
−1.20 |
−3.51 |
0.33 |
−5.04 |
PQ-ref
18
|
- |
−3.54 |
0.27 |
−5.07 |
Charge transport properties
The OFETs with a bottom-gate top-contact device configuration were fabricated to investigate the charge-transport properties of PQ-1 and PQ-2. Fig. 6a–d show the transfer characteristics and corresponding |IDS|1/2-VG curves of the six separated OFET devices for PQ-1 and PQ-2 in one batch, respectively, which reflects the relatively good uniformity and repeatability based on PQ-2. The representative output characteristics of OFETs based on PQ-1 and PQ-2 are shown in Fig. 6e and f, respectively. Both polymers showed p-type transport with a hole mobility of ∼10−3 cm2 V−1 s−1 and current on/off ratio (Ion/Ioff) >103 (Table 4), comparable to the hole mobility of the as-cast PQ-ref film reported in the literature.18 The as-cast films of both polymers showed only (100) diffraction peaks in their X-ray diffraction (XRD) spectra, indicating that they possessed low crystallinity to some extent (Fig. 7).
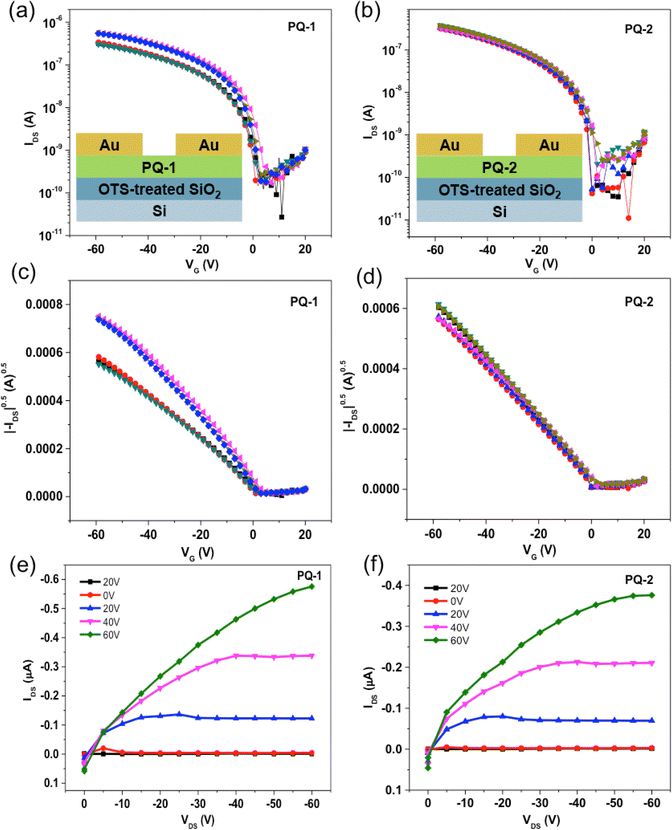 |
| Fig. 6 (a) Transfer, (c) |IDS|1/2-VG, and (e) output curves of OFETs based on PQ-1; (b) transfer, (d) |IDS|1/2-VG and (f) output curves of OFETs based on PQ-2. | |
Table 4 OFET performance of PQ-1 and PQ-2a
Polymer |
μ
h [cm2 V−1 s−1] |
I
on/Ioff |
V
T [V] |
Average performance parameters of six devices.
|
PQ-1 (at cast) |
2.0 × 10−3 |
2.9 × 103 |
2.8 |
PQ-2 (at cast) |
1.3 × 10−3 |
1.0 × 103 |
1.1 |
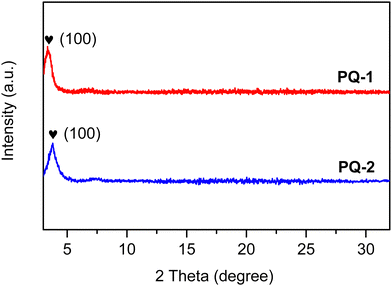 |
| Fig. 7 X-ray diffraction patterns of PQ-1 and PQ-2. | |
Conclusions
In summary, we have designed and synthesized two new 6H-[1,2,5]thiadiazolo[3,4-e]thieno[3,2-b]indole-flanked p-AQM based aromatic-quinoidal polymer semiconductors PQ-1 and PQ-2. To the best of our knowledge, these are the first p-AQM polymers synthesized by the convenient DArP method. Both polymers had high molecular weights (Mn > 120 kDa) and low band gaps (Eg < 1.55 eV). Compared to the reference polymer PQ-ref, PQ-1 with the same electron donor unit had more red-shifted absorption. Similarly to PQ-ref, PQ-1 and PQ-2 possessed a high EHOMO (∼−5.0 eV) and displayed typical p-type transport properties. We expect that the design strategy to flank fused heteroaromatic rings and the DArP method possess excellent potential to develop new π-extended conjugated unit-based polymer semiconductors.
Conflicts of interest
The authors declare no competing financial interest.
Acknowledgements
This work was financially supported by the National Natural Science Foundation of China (No. 21704015, U1905215, and 52072076), the Natural Science Foundation of Fujian Province (2021J01596), and the Natural Science Foundation Project of CQ CSTC (cstc2020jcyj-msxmX0488).
Notes and references
- X. Guo and A. Facchetti, Nat. Mater., 2020, 19, 922–928 CrossRef CAS PubMed.
- F. Huang, Z. S. Bo, Y. H. Geng, X. H. Wang, L. X. Wang, Y. G. Ma, J. H. Hou, W. P. Hu, J. Pei, H. L. Dong, S. Wang, Z. Li, Z. G. Shuai, Y. F. Li and Y. Cao, Acta Polym. Sin., 2019, 50, 988–1046 CAS.
- S. Holliday, J. E. Donaghey and I. McCulloch, Chem. Mater., 2014, 26, 647–663 CrossRef CAS.
- W. V. Wang, Y. Zhang, X.-Y. Li, Z.-Z. Chen, Z.-H. Wu, L. Zhang, Z.-W. Lin and H.-L. Zhang, InfoMat, 2021, 3, 814–822 CrossRef CAS.
- Y. Yang, Z. Liu, G. Zhang, X. Zhang and D. Zhang, Adv. Mater., 2019, 31, 1903104 CrossRef CAS PubMed.
- X. Zhao, H. Cai, Y. Deng, Y. Jiang, Z. Wang, Y. Shi, Y. Han and Y. Geng, Macromolecules, 2021, 54, 3498–3506 CrossRef CAS.
- T. Mikie, M. Hayakawa, K. Okamoto, K. Iguchi, S. Yashiro, T. Koganezawa, M. Sumiya, H. Ishii, S. Yamaguchi, A. Fukazawa and I. Osaka, Chem. Mater., 2021, 33, 8183–8193 CrossRef CAS.
- C. L. Anderson, N. Dai, S. J. Teat, B. He, S. Wang and Y. Liu, Angew. Chem., Int. Ed., 2019, 58, 17978–17985 CrossRef CAS PubMed.
- J. Huang and G. Yu, Mater. Chem. Front., 2021, 5, 76–96 RSC.
- T. Du, Y. Liu, C. Wang, Y. Deng and Y. Geng, Macromolecules, 2022, 55, 5975–5984 CrossRef CAS.
- P. Deng, L. Liu, S. Ren, H. Li and Q. Zhang, Chem. Commun., 2012, 48, 6960–6962 RSC.
- Y. Deng, B. Sun, Y. He, J. Quinn, C. Guo and Y. Li, Angew. Chem., Int. Ed., 2016, 55, 3459–3462 CrossRef CAS PubMed.
- C. Dong, Bi Meng, J. Liu and L. Wang, J. Mater. Chem. C, 2022, 10, 2718–2723 RSC.
- S. Dong and Z. Li, J. Mater. Chem. C, 2022, 10, 2431–2449 RSC.
- T. Mikie and I. Osaka, J. Mater. Chem. C, 2020, 8, 14262–14288 RSC.
- X. Ji and L. Fang, Polym. Chem., 2021, 12, 1347–1361 RSC.
- M. Yang, T. Du, X. Zhao, X. Huang, L. Pan, S. Pang, H. Tang, Z. Peng, L. Ye, Y. Deng, M. Sun, C. Duan, F. Huang and Y. Cao, Sci. China: Chem., 2021, 64, 1219–1227 CrossRef CAS.
- X. Liu, B. He, C. L. Anderson, J. Kang, T. Chen, J. Chen, S. Feng, L. Zhang, M. A. Kolaczkowski, S. J. Teat, M. A. Brady, C. Zhu, L.-W. Wang, J. Chen and Y. Liu, J. Am. Chem.
Soc., 2017, 139, 8355–8363 CrossRef CAS PubMed.
- X. Liu, B. He, A. Garzón-Ruiz, A. Navarro, T. L. Chen, M. A. Kolaczkowski, S. Feng, L. Zhang, C. A. Anderson, J. Chen and Y. Liu, Adv. Funct. Mater., 2018, 28, 1801874 CrossRef.
- H. Liang, C. Liu, Z. Zhang, X. Liu, Q. Zhou, G. Zheng, X. Gong, L. Xie, C. Yang, L. Zhang, B. He, J. Chen and Y. Liu, Adv. Funct. Mater., 2022, 32, 2201903 CrossRef CAS.
- B. Dyaga, S. Mayarambakam, O. A. Ibraikulov, N. Zimmermann, S. Fall, O. Boyron, T. Heiser, N. Leclerc, N. Berton and B. Schmaltz, Mater. Adv., 2022, 3, 6853–6861 RSC.
- C. Chen, C.-Z. Du and X.-Y. Wang, Adv. Sci., 2022, 9, 2200707 CrossRef CAS PubMed.
- H. Xin, B. Hou and X. Gao, Acc. Chem. Res., 2021, 54, 1737–1753 CrossRef CAS PubMed.
- N. Liang, D. Meng and Z. Wang, Acc. Chem. Res., 2021, 54, 961–975 CrossRef CAS PubMed.
- Y. Ma, D. Cai, S. Wan, P. Wang, J. Wang and Q. Zheng, Angew. Chem., Int. Ed., 2020, 59, 21627–21633 CrossRef CAS PubMed.
- J. Guo, Y. Yang, C. Dou and Y. Wang, J. Am. Chem. Soc., 2021, 143, 18272–18279 CrossRef CAS PubMed.
- H. Jiang, S. Zhu, Z. Cui, Z. Li, Y. Liang, J. Zhu, P. Hu, H.-L. Zhang and W. Hu, Chem. Soc. Rev., 2022, 51, 3071–3122 RSC.
- W. Xia, Y. Huang, P. Deng and Y. Yu, Dyes Pigm., 2022, 208, 110825 CrossRef CAS.
- Z.-F. Yao, Y.-Q. Zheng, J.-H. Dou, Y. Lu, Y. Ding, L. Ding, J.-Y. Wang and J. Pei, Adv. Mater., 2021, 33, 2006794 CrossRef CAS PubMed.
- B. Liu, J. Li, W. Zeng, W. Yang, H. Yan, D.-C. Li, Y. Zhou, X. Gao and Q. Zhang, Chem. Mater., 2021, 33, 580–588 CrossRef CAS.
- X. Tao, Y. Liu, L. Du, Y. Yan, Z. Wu, Y. Zhao, Y. Guo, H. Chen and Y. Liu, J. Mater. Chem. C, 2021, 9, 15083–15094 RSC.
- D.-W. Liu, Y. Zhang, X.-Y. Li, Q. Xiao, W.-J. Sun, X. Shao and H.-L. Zhang, J. Mater. Chem. C, 2021, 9, 6560–6567 RSC.
- R. C. L. Gott-Betts, A. A. Burney-Allen, D. L. Wheeler and M. Jeffries-EL, Mater. Adv., 2022, 3, 4831–4838 RSC.
- J. Ji, X. Wu, P. Deng, D. Zhou, D. Lai, H. Zhan and H. Chen, J. Mater. Chem. C, 2019, 7, 10860–10867 RSC.
- F. Gagnon, V. Tremblay, A. Soldera, M. U. Ocheje, S. Rondeau-Gagné, M. Leclerc and J.-F. Morin, Mater. Adv., 2022, 3, 599–603 RSC.
- A. C. B. Rodrigues, A. Eckert, J. Pina, U. Scherf and J. S. Seixas de Melo, Mater. Adv., 2021, 2, 3736–3743 RSC.
- C. Liu, X. Liu, G. Zheng, X. Gong, C. Yang, H. Liu, L. Zhang, C. L. Anderson, B. He, L. Xie, R. Zheng, H. Liang, Q. Zhou, Z. Zhang, J. Chen and Y. Liu, J. Mater. Chem. A, 2021, 9, 23497–23505 RSC.
- T. Bura, J. T. Blaskovits and M. Leclerc, J. Am. Chem. Soc., 2016, 138, 10056–10071 CrossRef CAS PubMed.
- A. L. Mayhugh, P. Yadav and C. K. Luscombe, J. Am. Chem. Soc., 2022, 144, 6123–6135 CrossRef CAS PubMed.
- H. Aoki, H. Saito, Y. Shimoyama, J. Kuwabara, T. Yasuda and T. Kanbara, ACS Macro Lett., 2018, 7, 90–94 CrossRef CAS PubMed.
- R. M. Pankow and B. C. Thompson, Polym. Chem., 2020, 11, 630–640 RSC.
- Z. Ni, H. Wang, H. Dong, Y. Dang, Q. Zhao, X. Zhang and W. Hu, Nat. Chem., 2019, 11, 271–277 CrossRef CAS PubMed.
- Q. Wang, S. V. Lenjani, O. Dolynchuk, A. D. Scaccabarozzi, H. Komber, Y. Guo, F. Guenther, S. Gemming, R. Magerle, M. Caironi and M. Sommer, Chem. Mater., 2021, 33, 668–677 CrossRef CAS.
- Y. Liu, K. Xian, X. Zhang, M. Gao, Y. Shi, K. Zhou, Y. Deng, J. Hou, Y. Geng and L. Ye, Macromolecules, 2022, 55, 3078–3086 CrossRef CAS.
- L. Giraud, S. Grelier, E. Grau, G. Hadziioannou, C. Brochon, H. Cramail and E. Cloutet, J. Mater. Chem. C, 2020, 8, 9792–9810 RSC.
- N. R. Kakde, H. J. Bharathkumar, B. A. Wavhal, A. Nikam, S. Patil, S. R. Dash, K. Vanka, K. Krishnamoorthy, A. Kulkarni and S. K. Asha, J. Mater. Chem. C, 2022, 10, 13025–13039 RSC.
- M. Mooney, A. Nyayachavadi and S. Rondeau-Gagné, J. Mater. Chem. C, 2020, 8, 14645–14664 RSC.
- M. Wakioka, S. Ishiki and F. Ozawa, Macromolecules, 2015, 48, 8382–8388 CrossRef CAS.
- J. Kuwabara and T. Kanbara, Bull. Chem. Soc. Jpn., 2019, 92, 152–161 CrossRef CAS.
- M. Leclerc, S. Brassard and S. Beaupre, Polym. J., 2020, 52, 13–20 CrossRef CAS.
- G. Qian, X. Wang, S. Wang, Y. Zheng, S. Wang, W. Zhu and Y. Wang, Chem. – Eur. J., 2019, 25, 15401–15410 CrossRef CAS PubMed.
- R. S. Ashraf, A. J. Kronemeijer, D. I. James, H. Sirringhaus and I. McCulloch, Chem. Commun., 2012, 48, 3939–3941 RSC.
- F. Wang, Y. Dai, W. Wang, H. Lu, L. Qiu, Y. Ding and G. Zhang, Chem. Mater., 2018, 30, 5451–5459 CrossRef CAS.
|
This journal is © The Royal Society of Chemistry 2023 |