DOI:
10.1039/D2MA01068B
(Paper)
Mater. Adv., 2023,
4, 1916-1926
Development of cytocompatible protein-based hydrogels crosslinked using tetrakis(hydroxymethyl)phosphonium chloride
Received
5th December 2022
, Accepted 16th March 2023
First published on 20th March 2023
Abstract
Gelatin, a collagen derivative, possesses excellent properties such as high biocompatibility, high bioactivity, biodegradability, and low immunogenicity, making it an ideal candidate for developing hydrogels for biomedical applications. Gelatin hydrogels are often used in conjunction with other compounds and crosslinkers to improve their physicochemical properties. Tetrakis(hydroxymethyl)phosphonium chloride (THPC) is an amine-reactive crosslinker used previously for hydrogel preparation. However, its usage is limited due to its cytotoxicity at higher concentrations, mainly due to the formation of formaldehyde as a reaction intermediate. Herein, we report gelatin hydrogels crosslinked with THPC along with a novel thermal treatment method to improve the cytocompatibility of the developed hydrogels. Furthermore, LAPONITE® has been incorporated into the proposed hydrogels to further enhance its cytocompatibility along with physicochemical properties. The method of thermal treatment significantly improved the cell viability from ∼20% to ∼60% at a high THPC concentration of 8 mM which was further increased to ∼80% with LAPONITE® incorporation. Thermal treatment also increased the degradation time of hydrogels from ∼9 days to ∼18 days and LAPONITE® incorporation further increased it to ∼22 days at 8 mM THPC concentration. Based on the results, it can be concluded that the thermal treatment method can be an ideal choice for improving the cytocompatibility of THPC-based hydrogels and the synergistic effects of LAPONITE® and thermal treatment can make these hydrogels an ideal candidate for biomedical applications.
Introduction
Gelatin is derived by partially hydrolyzing collagen, which includes the breakage of polypeptide bonds and disrupting the cross-linkages present in between the polypeptide chains of the collagen protein.1 As an essential component of the extracellular matrix and a collagen derivative, it is highly biocompatible, and biodegradable.2 Additionally, the RGD (arginyl-glycyl-aspartic acid) sequences in the gelatin chains provide the cell attachment sites and promote cell adhesion. These properties make gelatin an ideal candidate to be used as hydrogels for numerous biomedical applications. However, lower mechanical strength, slow gel formation process due to longer gelation time, and instability at physiological temperature due to their thermoreversible nature limit the application of gelatin-based hydrogels.3 Various strategies have been reported to improve the mechanical properties and stability and to reduce the gelation time of gelatin-based hydrogels and chemical crosslinking is one of them. Multiple crosslinkers have been reported previously to achieve the desired properties in gelatin hydrogels including dextran dialdehyde,4 genipin,5 glutaraldehyde,6 and so forth. Tetrakis(hydroxymethyl)phosphonium chloride (THPC) is one such example which is a tetra-functional crosslinker due to the presence of four hydroxymethyl arms in its structure. It is a crystalline solid known to be highly soluble in water and remains stable in the air.7 The reaction mechanism of THPC involves a Mannich-type reaction yielding formaldehyde as an intermediate.8 THPC is known to reduce the gelation time, yielding mechanically robust hydrogels. THPC has also been reported to have reversible bonding, which is also a reason for using this as the crosslinker of choice.9
Some of the studies have reported the use of THPC as a crosslinker with protein-based hydrogels. In a study conducted by Chung et al., THPC was used as a covalent crosslinking agent to form hydrogel with recombinant elastin-like protein.8 They demonstrated the cytocompatibility and cardiomyocyte differentiation in the developed hydrogels and quantified the formaldehyde released by the hydrogels. The study made use of modular recombinant protein developed using protein engineering technique and the gels formed exhibited a longer gelation time (∼7 min to ∼27 min), and lower mechanical strength with storage modulus ranging between ∼250 Pa to ∼2200 Pa. In a similar study conducted by Kambe et al., elastin-like protein-based hydrogels were developed by crosslinking with THPC.10 The hydrogels supported murine-induced pluripotent stem cells' division and differentiation and showed good water retention capacity and rheological properties. However, this study also made use of recombinant elastin-like proteins to achieve desired properties. In a recent study conducted by Yalçın et al., keratose proteins were crosslinked using THPC to develop a novel biopolymer-based scaffold which was characterized using various techniques.11 The hydrogels exhibited an excellent cell proliferation rate but the concentration of THPC used was low (<∼6 mM) to avoid its cytotoxic effects. Thus, as evident from previous reports, THPC-based hydrogels exhibit cell viability and proliferation either at a low concentration of THPC or with additional structural modifications in the base proteins. However, if used in lower concentrations, the THPC-based hydrogels exhibit poor mechanical properties with increased gelation time. Furthermore, at higher concentrations, it can affect cellular functions by reacting with free amines present on the surface of the cells.8
Herein, we report gelatin-based hydrogels crosslinked with THPC with a novel thermal treatment method to improve the cytocompatibility of the hydrogels. Additionally, LAPONITE®, a synthetic nanosized clay has also been incorporated into the hydrogels to improve its cytocompatibility and other physicochemical properties.12 Nanoclays are found to have excellent adsorption capacity due to their high surface area and thus they are assigned to adsorb organic and polar molecules.13,14 In our study, we have employed LAPONITE® to entrap the formaldehyde released from the samples to improve the cytocompatibility along with improving the physicochemical properties of the gelatin–THPC hydrogels.12 With the increase in the THPC concentration, we were able to achieve tunable properties including reduced gelation time, improved mechanical properties, increased degradation time, and minimal swelling ratio. However, to overcome the cytotoxic effects of THPC, we introduced the thermal treatment method which allowed for the use of higher concentrations of THPC (8 mM and 16 mM) with significant cell viability (from ∼20% to ∼60% at 8 mM THPC concentration) by reducing the formaldehyde release from the samples. Thermal treatment also had a profound effect on the stability of the hydrogels shown by an increased degradation time (∼9 days to ∼18 days) along with an improved swelling ratio (∼160% to ∼200% after 24 hours) as compared to untreated samples at 8 mM THPC concentration. Furthermore, the LAPONITE® incorporation contributed towards improved cell viability (∼80% at 8 mM THPC concentration), faster gelation process, improved hydrogel stability (degradation time: ∼38 days at 16 mM THPC concentration), and reduced formaldehyde release from samples. Therefore, the thermal treatment method combined with LAPONITE® incorporation can serve as a feasible, low-cost, and simple solution to reduce the cytotoxic effects of THPC in thermostable protein-based hydrogels. Moreover, the gelatin–THPC hydrogels developed displayed excellent properties and thus can be employed in diverse biomedical applications.
Materials and methodology
Materials
Gelatin type A from porcine skin, 300 g Bloom (G1890), and THPC 80% in H2O (404861) were obtained from Sigma Aldrich. LAPONITE® RD (TCD121001) was acquired from Talas, Brooklyn, NY, USA.
Methods
Preparation of gelatin–THPC nanocomposite hydrogels.
Different compositions of hydrogels were prepared using gelatin, LAPONITE®, and varying concentrations of THPC as shown in Table 1. The concentration of gelatin and LAPONITE® was kept constant at 20% and 1%, respectively. LAPONITE® dispersion was formed in water held at 80 °C by using a magnetic stirrer hot plate at 1200 rpm. After complete dispersion of LAPONITE®, gelatin was added to the solution and was allowed to mix at 80 °C until a clear solution was formed. The solution was then pipetted into MCT (Micro centrifuge tube), and the required amount of THPC was added. The MCTs were then subjected to a vortex, forming a clear hydrogel. The hydrogel formation was confirmed by using the tube inversion method.
Table 1 Compositions of the gelatin–LAPONITE®–THPC and gelatin–THPC hydrogels
S. no |
Gelatin (w/v) (%) |
LAPONITE® (w/v) (%) |
THPC (mM) |
1 |
20 |
1 |
6 |
2 |
20 |
1 |
8 |
3 |
20 |
1 |
16 |
4 |
20 |
0 |
6 |
5 |
20 |
0 |
8 |
6 |
20 |
0 |
16 |
Preparation of gelatin–THPC hydrogels
Gelatin–THPC hydrogels without LAPONITE® and thermal treatment were used as controls. These hydrogels were prepared by adding gelatin to water at 80 °C on a magnetic stirrer hot plate. The solution was pipetted into an MCT, and THPC was added according to the required concentrations as shown in Table 1.
Thermal treatment
Thermal treatment was employed to assess its effect on the cell viability of the gelatin–THPC crosslinked hydrogels with or without LAPONITE® incorporation. The samples were allowed to gel before transferring them into a water bath kept at 80 °C, where samples were placed for 1 hour with an open lid of MCTs and the water bath.
Gelation time estimation
The gelation time of the formed hydrogels was estimated using the tube inversion method. The polymer solution and crosslinker were vortexed in a centrifuge tube, and the gelation time was noted by inverting the tube. The hydrogels were considered gelled when there was no flow of the gel after inverting the tube.
Compression test
This test was performed with a Ta·HD plus texture analyzer (Stable Micro Systems) following a previously reported protocol to evaluate the mechanical properties of the samples.15 The thermally treated samples were kept in room temperature to cool down so that the temperature remains same for all samples. Samples were prepared with dimensions of 9 mm in diameter and 13 mm in height. The stress–strain curve was plotted for each sample, and the elastic modulus was obtained by calculating the slope of the elastic region at 5% strain rate.
Fourier-transform Infrared spectroscopy (FTIR)
FTIR analysis was performed using Bruker, Alpha E in the range of 4000 to 600 cm−1 to analyze the characteristic peaks of the gelatin–THPC and gelatin–LAPONITE®–THPC hydrogels. The samples for FTIR analysis were prepared in MCTs and cut into small discs. The yielded samples were then used for FTIR analysis in attenuated total reflectance (ATR) mode. The results were analyzed using the OriginPro software (OriginPro version 9.0.0).
Swelling ratio
The swelling ratios of samples were determined by immersing the samples in PBS solution at room temperature and taking weights at varied time intervals. After each interval, the hydrogels were taken out and wiped with a tissue to remove the excessive water. After removing excess water from the surface of hydrogels, the samples were weighed and immersed back into the PBS solution. The process was repeated after regular time intervals. The swelling ratio of the samples was calculated using the equation given below: | 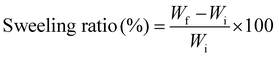 | (1) |
where, Wi and Wf are the initial and final weights of samples before and after immersing the samples in PBS solution.
Biodegradation
The biodegradation rate of the hydrogel samples was measured by immersing the samples in 0.5 mg mL−1 of lysozyme solution prepared in PBS and incubating the samples at 37 °C.16 The initial weights of the samples were noted down, followed by weighing the samples after 24 hours. The lysozyme solution of the samples was changed after every 48 hours to avoid contamination. The relative mass of hydrogels after every 24 hours was calculated using the equation depicted below:17 | 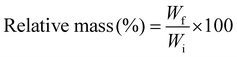 | (2) |
where, Wi is the initial weight of each sample before immersing them in lysozyme solution and Wf is the final weight of each sample measured after every 24 hours.
THPC release quantification assay
A standard curve was prepared to analyze the excess THPC released from hydrogels by plotting absorbance values corresponding to various THPC concentrations according to a previously reported protocol.18 Briefly, different concentrations of THPC were made to react with 2 mM of AgNO3, and the corresponding absorbance values of the AgCl precipitated were noted at 395 nm using the Duetta Absorbance and Fluorescence Spectrometer (HORIBA, Ltd). Silver nitrate is light-sensitive; thus, the entire experiment was performed in a dark room. Distilled water was taken as blank.
The weight of each sample was kept equal. These samples were then kept in distilled water and incubated at 37 °C in a CO2 incubator. The extracts were collected after 24 hours and analyzed for the presence of THPC by adding 2 mM of AgNO3. The absorbance values were obtained at 395 nm, which were compared with the standard curve to obtain the final concentration of released THPC in the samples.
Formaldehyde release quantification assay
Initially, a standard curve was prepared to quantify the formaldehyde released from the hydrogels by plotting absorbance values corresponding to various known values of formaldehyde concentrations according to a previous protocol.19 Samples were taken in equal weights and immersed in distilled followed by incubation in a CO2 incubator at 37 °C. The extracts were collected from the samples after 24 hours.
From these extracts, 100 μL of the extract was taken and added to a solution containing 3 mL of concentrated sulphuric acid and 100 μL of 0.1% carbazole prepared in absolute ethanol. The prepared samples were incubated for 1.5 hours at room temperature. The absorbance was measured at 649 nm using the Duetta Absorbance and Fluorescence Spectrometer (HORIBA, Ltd). For the blank sample, 100 μL of distilled water was added to 3 mL of concentrated sulphuric acid and 100 μL of 0.1% carbazole. The formaldehyde concentration in each hydrogel was then calculated using the standard curve.
Analysis of cytocompatibility
Live–dead assay using confocal microscopy.
The confocal microscopy was performed to check for live and dead cells seeded onto hydrogels using a confocal microscope with Model ID Confocal TCS SP8. Two sets of the sample were prepared; one set was thermally treated continuously for 1 hour at 80 °C, while the other set was kept without thermal treatment. The hydrogel samples were seeded with L929 cells and incubated in Dulbecco's Modified Eagle Media (DMEM) medium for 24 hours at 37 °C. Further, the samples were stained with fluorescein diacetate (FDA) and propidium iodide (PI) dyes and visualized under a confocal microscope to analyse the live and dead cells, respectively.
Quantification of cell viability using MTT assay
The cytocompatibility of the hydrogels was analysed using the extract based method or the MTT assay. The hydrogel samples were dipped in DMEM and incubated in a CO2 incubator at 37 °C for 24 hours. Simultaneously, wells for each sample were seeded with cells in a 96-well plate cultured for 24 hours with a concentration of 104 cells per well. After 24 hours, the cells were treated with the extracts of the hydrogels, and the cells were further incubated for 24 hours. The extracts from the hydrogels were used as treatment to assess the cytotoxicity of released formaldehyde from the samples. A control well was also used, which contained only the cells and DMEM. The MTT assay was performed to quantify the cell viability in the hydrogels by obtaining the absorbance values using a Biorad Eyemark microplate absorbance reader at 595 nm.
Statistical analysis
The results obtained for gelatin–LAPONITE®–THPC nanocomposites and gelatin–THPC hydrogels were compared using the two-way ANOVA test followed by Tukey's multiple comparisons test (GraphPad Prism), and results with P values *P < 0.0005 and **P < 0.0001 are statistically significant. All the data are represented as mean ± standard deviation (n = 3).
Results and discussion
Chemical crosslinking has been a promising alternative to develop natural protein-based hydrogels with enhanced physicochemical properties. The combination of chemical crosslinkers and natural proteins allows the achievement of improved mechanical strength, stability, and tunable properties along with biocompatibility and biodegradability due to the presence of natural proteins. In recent years, THPC has emerged as a potentially promising crosslinker due to its low cost, ease of availability, solubility in water and ability to enhance the properties of protein-based hydrogels. Nevertheless, the reaction of THPC with primary and secondary amines of proteins releases formaldehyde as a reaction intermediate. The cytotoxic effect induced due to the formaldehyde release restricts the use of high concentrations of THPC thus limiting its potential. Previous studies reported the use of low concentrations of THPC to reduce the levels of free formaldehyde present in the hydrogels thus enhancing the cytocompatibility. However, the use of lower concentrations of THPC compromises the mechanical strength and stability of hydrogels.
The aim of the present study was to introduce a facile method to enhance the cytocompatibility of THPC-based hydrogels at high THPC concentrations without compromising mechanical strength, stability, and other properties. The thermal treatment method was applied to hydrogels containing different concentrations of THPC. The hydrogels were exposed to a high temperature of 80 °C and the gels were heated in an open environment. We hypothesized that heating the hydrogels at higher temperatures will lead to evaporation of the excessive free formaldehyde or the residual formaldehyde present in the samples thus improving the cell viability. In previous studies, it has been reported that LAPONITE® has the ability to reduce formaldehyde released from the samples by entrapping the free formaldehyde.12 Furthermore, the incorporation of LAPONITE® into hydrogels has been known to enhance thermal stability, improve cell adhesion, increase the degradation time, and decrease the swelling ratio.20 Therefore, LAPONITE® has also been used in the developed hydrogels to improve their properties and cytocompatibility. The study focused on assessing the effects of thermal treatment, LAPONITE® incorporation, and a combination of both on the properties of gelatin–THPC hydrogels.
Gelation time estimation
Gelation time is an essential criterion based on which the applicability of hydrogels is assessed and the use of crosslinkers allows for tuning of gelation time based on the intended application. The gelation time of gelatin–THPC hydrogels was found to be affected by the concentration of THPC along with the addition of LAPONITE®. As evident from Fig. 1, a decrease in the gelation time was observed with increasing concentration of THPC. The highest gelation time was 2 min 30 s ± 5 s observed in the sample containing 6 mM THPC whereas the sample with 16 mM THPC exhibited the lowest value of 1 min 30 s ± 6 s. Our findings are consistent with previous findings of Chung et al., who showed that as long as the THPC concentrations are below the saturation point, with increasing concentrations of THPC, the gelation process gets faster due to the availability of increased reactive sites of THPC to the free amine-reactive groups of proteins.8
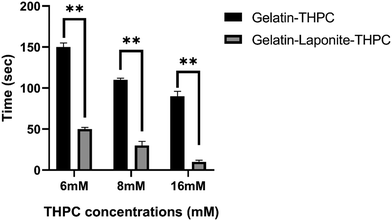 |
| Fig. 1 Graph showing gelation time of gelatin–THPC and gelatin–LAPONITE®–THPC hydrogels at different THPC concentrations. The data are analyzed using a two-way ANOVA test followed by Tukey's multiple comparisons test and P values with *P < 0.0005 and **P < 0.0001 are statistically significant. The data are represented as mean ± SD (n = 3). | |
The addition of LAPONITE® further reduced the gelation time and with the sample containing 16 mM THPC concentration, the gels were formed almost instantaneously. LAPONITE® has been reported to have electrostatic interactions and hydrogen bonding with gelatin, which helped to reduce the gelation time of these hydrogels considerably.21
Compression test
A compression study was done to assess the mechanical strength of the gelatin–THPC hydrogels and the impact of varying concentrations of THPC, thermal treatment, and LAPONITE® incorporation on their mechanical strength. Fig. 2 illustrates the elastic modulus of gelatin–THPC and gelatin–LAPONITE®–THPC hydrogels before and after thermal treatment. The elastic modulus was found to be increasing with increasing THPC concentration. The highest elastic modulus was observed in the 16 mM gelatin–THPC sample with a value of 54.45 ± 1.31 kPa. Thermal treatment led to a decrease in the elastic modulus of the gelatin–THPC hydrogels with an elastic modulus of 23.22 ± 1.2 kPa and 25.38 ± 1.8 kPa for samples with 6 mM and 16 mM THPC concentrations respectively. The elastic modulus of thermally treated samples was lesser than the untreated samples in the gelatin–THPC hydrogels due to the prolonged heating of gelatin at higher temperatures which causes disruption of the physical network of gelatin due to the transition from triple helical structure to random coil. Thus, heating leads to partial degradation of gelatin, its interaction with THPC reduces leading to lower crosslinking density thereby decreasing the elastic modulus of the hydrogels. On the contrary, the presence of an intact triple helical structure led to a higher elastic modulus in the case of untreated hydrogels. The results are also supported by a similar study by Van et al., who observed a lower elastic modulus in methacrylamide-modified gelatin hydrogels at temperatures above the melting point of gelatin.22 Moreover, a further decrease in elastic modulus was observed after incorporating LAPONITE® into gelatin–THPC hydrogels. The elastic modulus of the LAPONITE®-incorporated hydrogels (13.31 ± 2.1 kPa to 18.67 ± 2.31 kPa) was significantly lower than the gelatin–THPC hydrogels (23.22 kPa ± 1.3 to 54.45 ± 1.31 kPa). This decrease in elastic modulus can be attributed to the formation of agglomerates of the nanocomposites resulting in defects in the matrix and low interaction of nanocomposites to the polymer matrix.23–25 These results are further corroborated by the similar findings of Golafshan et al., who showed that the elastic modulus reduced for gelatin–polydopamine modified LAPONITE® hydrogels upon increased LAPONITE® concentration from 0.5 to 2% in PVA–alginate hydrogels.23
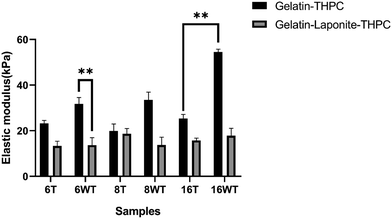 |
| Fig. 2 Result of compression test showing elastic modulus of thermally treated (T) and untreated (WT – without treatment) (A) gelatin–THPC samples and (B) gelatin–LAPONITE®–THPC samples. The data are analyzed using a two-way ANOVA test followed by Tukey's multiple comparisons test and P values with *P < 0.0005 and **P < 0.0001 are statistically significant. The data are represented as mean ± SD (n = 3). | |
Fourier transform infrared spectroscopy
FTIR was performed for both gelatin–THPC and gelatin–LAPONITE® hydrogels for different concentrations of THPC. Fig. 3 describes the FTIR graph of sample with 8 mM THPC concentration and the effect of thermal treatment and LAPONITE® incorporation on the sample. The peak at 1003 cm−1 corresponds to Si–O stretching in the LAPONITE® particles thus indicating that LAPONITE® was successfully incorporated in the samples.26 An increase in the band intensity between 3200–3400 cm−1 is due to the contribution of O–H band stretching in the LAPONITE® nanoparticles.27 The peak at 1557 cm−1 and 1636 cm−1 is due to the presence of carboxylate groups (O
C–O–) and amide I of gelatin, respectively.28,29 There was no change in the graph of the sample after thermal treatment indicating that the method does not lead to any changes in the chemical structure of hydrogels. In addition, the FTIR analysis showed no additional peaks after adding LAPONITE®, indicating only physical interaction between the LAPONITE® and the polymer matrix.27,30
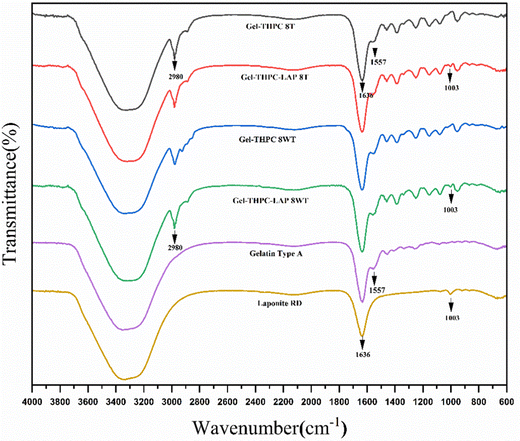 |
| Fig. 3 Comparison of FTIR graph of thermally treated (T) and untreated (WT – without treatment) gelatin–THPC and gelatin–LAPONITE®–THPC hydrogels. | |
Swelling ratio
The ability of protein-based hydrogels to imbibe higher amounts of water or liquid contents is one of the most essential properties which makes it ideal for biomedical applications. A higher swelling ratio is a desirable property in certain tissue engineering applications as it allows better diffusion and a high water content will ensure proper transport of nutrients and removal of toxic compound from the hydrogels thus improving the cell viability.31 However, there are also certain clinical conditions which requires hydrogels to be applied to confined areas like those involving brain and spinal cord injuries where hydrogels with minimal swelling ratio are required. Fig. 4 describes the swelling ratio of gelatin–THPC and gelatin–LAPONITE®–THPC in thermally treated and untreated samples with different THPC concentrations. The swelling studies were restricted to 24 hours as the increase in swelling was gradual towards the end of 24 hours and beyond it the degradation of samples was observed. The swelling ratio was observed to be decreasing as we increased the THPC concentration. The highest swelling ratio, 190.76 ± 6.22%, was observed corresponding to the lowest concentration of THPC in the gelatin–THPC hydrogels whereas with a concentration of 16 mM THPC, lowest swelling ratio of 115.11 ± 10.71% was observed after 24 hours. A high concentration of crosslinker will lead to higher crosslinking density making the samples rigid thus restricting the movement of polymeric chains and limiting the water uptake. Our observations were similar to the previous study by Yalçın et al., who reported a decrease in the swelling ratio from 69 ± 4 to 23 ± 3 with an increase in the keratose:THPC reactive groups ratio from 1
:
2 to 1
:
4 due to an increase in the crosslinking density of the hydrogels.11 Thermal treatment method was found to increase the swelling ratio of both gelatin–THPC and gelatin–LAPONITE®–THPC hydrogels. Among all the samples studied, maximum swelling ratio of 225.21 ± 11.25% was attained by the thermally treated gelatin–THPC hydrogel at 6 mM THPC concentration at the end of 24 hours. The prolonged heating of gelatin at higher temperatures (≥80 °C) leads to its partial degradation which reduces the crosslinking density of heat treated hydrogels resulting in increased swelling ratio. Moreover, water loss was observed in heat treated samples which might have contributed to an increase in swelling. On the contrary, the addition of LAPONITE® to samples led to a decrease in the swelling ratio as compared to the samples without the presence of LAPONITE®. At 6 mM THPC concentration, the swelling ratio of gelatin–LAPONITE®–THPC hydrogels was found to be 134.97 ± 7.42% which was lower than the pure gelatin–THPC hydrogel which attained a swelling ratio of 190.76 ± 6.22% at the end of 24 hours. This reduction in swelling ratio can be attributed to the interaction between LAPONITE® and gelatin thus reducing the water content of hydrogels. The swelling ratio of hydrogels exposed to both thermal treatment and LAPONITE® incorporation was 157.27 ± 5.84% which was significantly lower as compared to the swelling ratio of pure gelatin–THPC hydrogels with a value of at 6 mM THPC concentration after 24 hours. Thus, the combined effect of thermal treatment and LAPONITE® incorporation led to reduction in the swelling ratio and these samples can be utilized in applications requiring minimal swelling ratio.
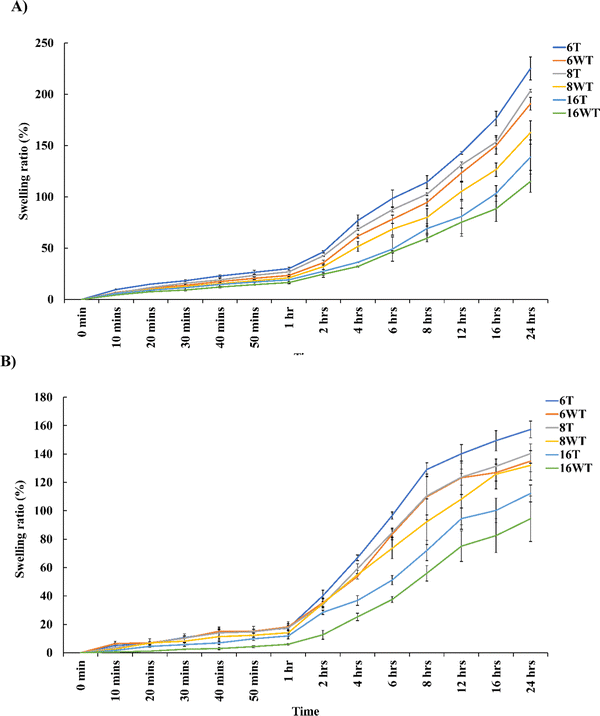 |
| Fig. 4 Swelling behaviour of thermally treated (T) and untreated (WT – without treatment) (A) gelatin–THPC samples and (B) gelatin–LAPONITE®–THPC samples. The data are analyzed using two-way ANOVA test followed by Tukey's multiple comparisons test and P values with *P < 0.0005 and **P < 0.0001 are statistically significant. The data are represented as mean ± SD (n = 3). | |
Biodegradation
A biodegradation study of these hydrogels was performed to assess their nature under pseudo-physiological conditions by keeping them in lyozyme solution at 37 °C. Fig. 5 shows the percentage decrease in the weight as a function of time in days for thermally treated and untreated gelatin–THPC and gelatin–LAPONITE®–THPC samples at various THPC concentrations. Initially, swelling of samples was observed in the lysozyme solution and thus the percentage of degradation was measured after each sample attained the maximum weight which was attained at different days by different samples. An increase in the degradation time was observed with increasing concentrations of THPC in the hydrogels. The sample containing 6 mM THPC degraded within 5 days which was significantly less as compared to the samples with 16 mM THPC whose complete degradation occurred after 22 days. Thermal treatment contributed towards the stability of gelatin–THPC hydrogels as a slower degradation was observed in thermally treated gelatin–THPC samples. During heating, the partial degradation of gelatin leads to lower crosslinking density which increases the swelling capacity of the hydrogels. Thus, heat treated samples demonstrated higher swelling and took longer duration to achieve maximum swelling as compared to untreated samples. The degradation was observed only after the samples attained their swelling equilibrium and thus heat-treated samples showed enhanced stability as compared to the untreated samples. Furthermore, LAPONITE® containing samples showed an enhanced stability due to the additional ionic interaction and hydrogen bonding besides the covalent crosslinking of gelatin–THPC.32 The presence of LAPONITE® also reduces the interaction between lysozyme and polymer matrix by blocking the attacking sites of lysozyme thereby increasing stability.33 The thermal treatment and LAPONITE® addition collectively imparted excellent stability to the hydrogels. The thermally treated gelatin–LAPONITE®–THPC sample with 16 mM THPC concentration was stable for the longest duration and degraded after 38 days.
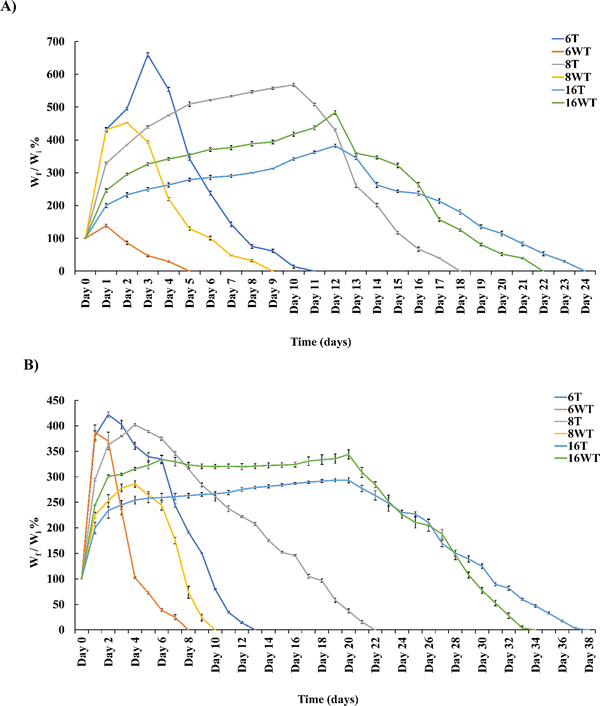 |
| Fig. 5 Percentage of degradation of thermally treated (T) and untreated (WT – without treatment) (A) gelatin–THPC samples and (B) gelatin–LAPONITE®–THPC samples. The data are analysed using two-way ANOVA test followed by Tukey's multiple comparisons test and P values with *P < 0.0005 and **P < 0.0001 are statistically significant. The data are represented as mean ± SD (n = 3). | |
THPC release quantification assay
THPC quantification assay was performed to determine the amount of unreacted THPC present in the samples. As the presence of free THPC can affect the cell viability by interacting with the primary amines present on the cell surface, it is crucial to determine the amount of unreacted THPC present in the samples. Fig. 6 describes the concentrations of unreacted THPC present in thermally treated and untreated gelatin–THPC and gelatin–LAPONITE®–THPC hydrogels. The concentration of unreacted THPC was observed in the range of 0.51 ± 0.08 mM to 1.18 ± 0.1 mM for all the samples. The variation in THPC concentration did not produce any significant changes in the amount of unreacted THPC thus showing that there is no oversaturation or undersaturation of the amine reactive sites and the reaction between THPC reactive sites and amine reactive sites took place efficiently in the present range of concentrations used. Moreover, thermal treatment also did not produce any significant change in the concentration of unreacted THPC in case of gelatin–THPC samples showing that the reaction between THPC and gelatin is not hindered by the thermal treatment process. However, addition of LAPONITE® showed a significant increase in the amount of unreacted THPC present in the samples. The concentration of unreacted THPC was found to be as high as 1.03 ± 0.06 mM and 1.18 ± 0.1 mM in the thermally treated samples with 8 mM and 16 mM THPC concentrations after addition of LAPONITE®. This can be attributed to the hindering of crosslinking in the nanocomposite hydrogels due to agglomerate formation and interaction of LAPONITE® to gelatin particles. In case of gelatin–LAPONITE®–THPC samples, thermal treatment also contributed significantly towards increasing concentration of unreacted THPC.
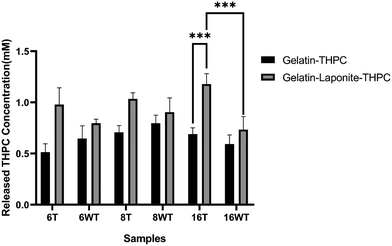 |
| Fig. 6 Concentration of released THPC in thermally treated (T) and untreated (WT – without treatment) (A) gelatin–THPC samples and (B) gelatin–LAPONITE®–THPC samples. The data are analysed using two-way ANOVA test followed by Tukey's multiple comparisons test and P values with *P < 0.0005 and **P < 0.0001 are statistically significant. The data are represented as mean ± SD (n = 3). | |
Formaldehyde release quantification assay
Formaldehyde released by the hydrogels was quantified using the carbazole assay. The residual formaldehyde concentration in gelatin–THPC and gelatin–LAPONITE®–THPC hydrogels before and after thermal treatment is represented in Fig. 7. As expected, with the increase in THPC concentration, the concentration of formaldehyde released from the samples increased. The amount of formaldehyde concentration released in gelatin–THPC hydrogel with 6 mM THPC concentration was found to be 2.12 ± 0.31 mM and for 16 mM THPC concentration, it was found to be 6.83 ± 0.09 mM, which was the highest amongst all the samples. The effect of thermal treatment was evident in all samples as a significant decrease in all samples was observed. For the sample with 6 mM THPC concentration, the residual formaldehyde was 1.46 ± 0.07 mM and for 16 mM THPC concentration, the formaldehyde released was 4.21 ± 0.28 mM. These concentrations were lesser than the samples without thermal treatment due to the evaporation of the residual formaldehyde. The addition of LAPONITE® also reduced the release of formaldehyde to a great extent with formaldehyde concentration of 0.54 ± 0.03 mM and 1.29 ± 0.12 mM in samples with 6 mM and 16 mM THPC concentrations respectively. The results were in line with earlier reports suggesting that LAPONITE® incorporation reduces the formaldehyde release drastically due to entrapment of free formaldehyde and its adsorption on LAPONITE®'s surface.12 The presence of LAPONITE® might have hindered the crosslinking of THPC leading to lower formaldehyde release. The lower crosslinking can also be confirmed by lower Young's modulus as observed in the compression study. The combined effect of thermal treatment and LAPONITE® addition yielded the best results and the lowest concentration of 0.04 ± 0.03 mM was in thermally treated gelatin–LAPONITE®–THPC samples with 6 mM THPC concentration.
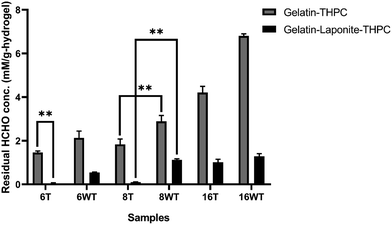 |
| Fig. 7 Concentration of released formaldehyde in thermally treated (T) and untreated (WT – without treatment) (A) gelatin–THPC samples and (B) gelatin–LAPONITE®–THPC samples. The data are analysed using two-way ANOVA test followed by Tukey's multiple comparisons test and P values with *P < 0.0005 and **P < 0.0001 are statistically significant. The data are represented as mean ± SD (n = 3). | |
Analysis of cytocompatibility
The primary objective of our present study was to enhance the cytocompatibility of the developed gelatin–THPC hydrogels. The cell viability was assessed qualitatively by utilizing a live–dead assay using confocal microscopy. The cells seeded on the hydrogels were stained using FDA-PI dyes and observed under a confocal microscope. It was observed that the cell viability was affected by three parameters – crosslinker concentration, thermal treatment, and LAPONITE® addition. The sample containing the highest concentration of THPC showed the least number of live cells whereas samples with lower THPC concentrations showed significant cell viability. We observed a lower concentration of free formaldehyde present in samples containing lower amounts of THPC and thus these samples showed significant viability. Fig. 8 shows the confocal images of gelatin–THPC and gelatin–LAPONITE®–THPC samples before and after thermal treatment with different concentrations of THPC. The thermal treatment also had a profound effect on the cytocompatibility of each sample. All samples showed a significant increase in cell viability post the thermal treatment process irrespective of the THPC concentration due to the possible evaporation of the free formaldehyde present in samples as observed by the lower formaldehyde concentrations present in the thermally treated samples. In addition to this, LAPONITE® also contributed significantly towards improving the cytocompatibility of the samples which can be attributed to the property of LAPONITE® to increase cell adhesion properties and its formaldehyde entrapping properties.12,34
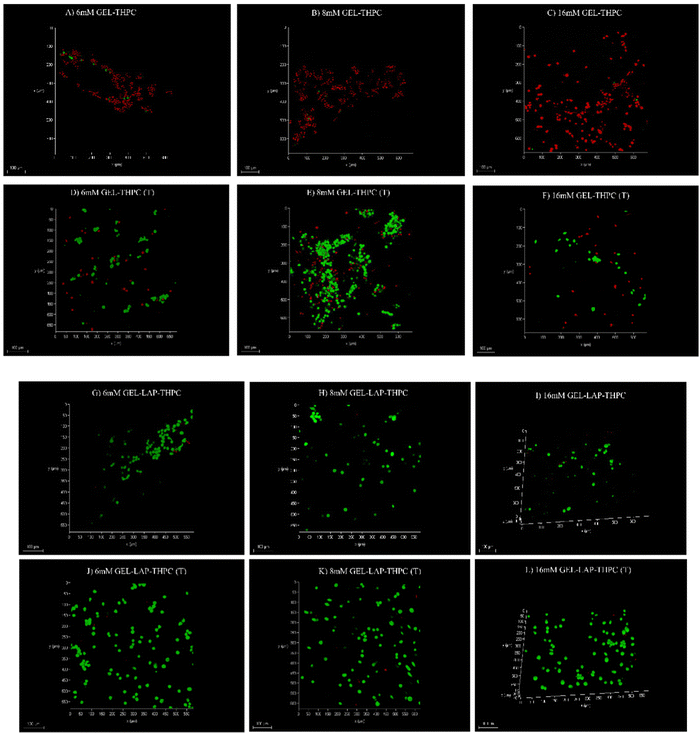 |
| Fig. 8 Confocal images representing live (green) and dead (red) cells in (i) gelatin–THPC (GEL–THPC) (A)–(C); (ii) gelatin–THPC after thermal treatment (GEL–THPC (T)) (D)–(F); (iii) gelatin–LAPONITE®–THPC (GEL–LAP–THPC) (G)–(I) and gelatin–LAPONITE®–THPC after thermal treatment (GEL–LAP–THPC) (J)–(L) samples at different THPC concentrations. Scale bar = 100 μm. | |
Cell viability was also quantified using the MTT assay and the results were in agreement with the confocal images. Fig. 9 shows the cell viability% for gelatin–THPC and gelatin–LAPONITE®–THPC before and after thermal treatment for various concentrations of THPC. The highest cell viability, 82 ± 4%, was observed in the thermally treated gelatin–LAPONITE® sample containing 8 mM THPC whereas it was around 65 ± 3% when the gelatin–THPC gels were exposed to only thermal treatment and 45 ± 2% in case of only LAPONITE® addition.
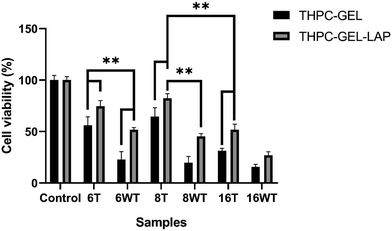 |
| Fig. 9 Cell viability (%) of thermally treated and non-thermally treated (A) gelatin–THPC and (B) gelatin–LAPONITE®–THPC hydrogels. The data are analysed using two-way ANOVA test followed by Tukey's multiple comparisons test and P values with *P < 0.0005 and **P < 0.0001 are statistically significant. The data are represented as mean ± SD (n = 3). | |
Thus, it can be confirmed that the synergistic effects of both thermal treatment and LAPONITE® incorporation is giving the best results and must be used together to achieve the highest cytocompatibility. A significant number of live cells were also observed in the sample containing highest concentration, 16 mM of THPC with a cell viability of 52 ± 5% when the samples were exposed to both thermal treatment and LAPONITE® addition, thus showing the utility of our strategy. However, a comparatively lesser cell viability was observed in samples with 6 mM THPC concentration, as the samples were not stable and structural disintegration was observed when they were kept in DMEM medium at 37 °C which might have caused excessive THPC release in extracts leading to cytotoxic effects. Nevertheless, the proposed methods can be used for stable samples to improve cell viability thereby yielding biocompatible THPC crosslinked hydrogels.
Conclusion
Thermal treatment significantly affected the cell viability of both nanocomposite and gelatin–THPC hydrogels. Additionally, LAPONITE®-incorporated gelatin–THPC nanocomposite hydrogels showed enhanced cytocompatibility than gelatin–THPC hydrogels. We found that 8 mM THPC concentration hydrogels were the most cytocompatible samples with or without the incorporation of LAPONITE®. These hydrogels have great potential, and thermal treatment is a novel process that can be implemented in hydrogels crosslinked with THPC or having excess formaldehyde concentration. These can be used as wound healing patches and may also be used to deliver drugs after encapsulation due to their tunable properties.
Author contributions
The manuscript was written through contributions of all authors. All authors have given approval to the final version of the manuscript.
Conflicts of interest
There are no conflicts to declare.
Acknowledgements
This work was funded by a research grant from the Science and Engineering Research Board (SERB), Department of Science and Technology, Human Resource Development, Government of India (SERB/F/10151/2018-2019). Science and Engineering Research Board (SERB), Department of Science and Technology, Human Resource Development, Government of India (SERB/F/10151/2018-2019).
References
- D. Liu, M. Nikoo, G. Boran, P. Zhou and J. M. Regenstein, Annu. Rev. Food Sci. Technol., 2015, 6, 527–557 CrossRef CAS PubMed.
- J. Il Kang and K. M. Park, J. Mater. Chem. B, 2021, 9, 1503–1520 RSC.
- J. Alipal, N. A. S. Mohd Pu’ad, T. C. Lee, N. H. M. Nayan, N. Sahari, H. Basri, M. I. Idris and H. Z. Abdullah, Mater. Today Proc., 2021, 42, 240–250 CrossRef CAS.
- E. Schacht, B. Bogdanov, A. Van Den Bulcke and N. De Rooze, React. Funct. Polym., 1997, 33, 109–116 CrossRef CAS.
- G. Thakur, A. Mitra, D. Rousseau, A. Basak, S. Sarkar and K. Pal, J. Mater. Sci.: Mater. Med., 2010, 22, 115–123 CrossRef PubMed.
- S. Farris, J. Song and Q. Huang, J. Agric. Food Chem., 2010, 58, 998–1003 CrossRef CAS PubMed.
- D. V. Moiseev and B. R. James, Phosphorus, Sulfur Silicon Relat. Elem., 2019, 195, 263–279 CrossRef.
- C. Chung, K. J. Lampe and S. C. Heilshorn, Biomacromolecules, 2012, 13, 3912–3916 CrossRef CAS PubMed.
- Z. Liu, Z. Tang, L. Zhu, S. Lu, F. Chen, C. Tang, H. Sun, J. Yang, G. Qin and Q. Chen, Int. J. Biol. Macromol., 2019, 141, 108–116 CrossRef CAS PubMed.
- Y. Kambe, T. Tokushige, A. Mahara, Y. Iwasaki and T. Yamaoka, Polym. J., 2018, 51, 97–105 CrossRef.
- D. Yalçın and A. Top, Iran. Polym. J., 2022, 31, 1057–1067 CrossRef.
- J. Shi, C. Wang, L. Hu, Y. Xiao and W. Lin, ACS Sustainable Chem. Eng., 2019, 7, 1195–1201 CrossRef CAS.
- J. P. Bellat, I. Bezverkhyy, G. Weber, S. Royer, R. Averlant, J. M. Giraudon and J. F. Lamonier, J. Hazard. Mater., 2015, 300, 711–717 CrossRef CAS PubMed.
- H. Y. Zhu and G. Q. Lu, Langmuir, 2001, 17, 588–594 CrossRef CAS.
- G. K. Wasupalli and D. Verma, Mater. Sci. Eng., C, 2020, 107, 110343 CrossRef CAS PubMed.
- G. K. Wasupalli and D. Verma, Carbohydr. Polym., 2022, 294, 119769 CrossRef CAS PubMed.
- P. Jain, H. C. Hung, B. Li, J. Ma, D. Dong, X. Lin, A. Sinclair, P. Zhang, M. B. O’Kelly, L. Niu and S. Jiang, Langmuir, 2019, 35, 1864–1871 CrossRef CAS PubMed.
- C. Brocker, H. Kim, D. Smith and S. Barua, Nanomater., 2017, 7, 127 CrossRef PubMed.
- K. A. Taylor, Appl. Biochem. Biotechnol., 1997, 68, 81–93 CrossRef CAS.
- D. López-Angulo, A. M. Q. B. Bittante, C. G. Luciano, G. Ayala-Valencia, C. H. C. Flaker, M. Djabourov and P. José do Amaral Sobral, Food Biosci., 2020, 35, 100596 CrossRef.
- Z. Cimen, S. Babadag, S. Odabas, S. Altuntas, G. Demirel and G. B. Demirel, ACS Appl. Polym. Mater., 2021, 3, 3504–3518 CrossRef CAS.
- A. I. Van Den Bulcke, B. Bogdanov, N. De Rooze, E. H. Schacht, M. Cornelissen and H. Berghmans, Biomacromolecules, 2000, 1, 31–38 CrossRef CAS PubMed.
- N. Golafshan, R. Rezahasani, M. Tarkesh Esfahani, M. Kharaziha and S. N. Khorasani, Carbohydr. Polym., 2017, 176, 392–401 CrossRef CAS PubMed.
- X. Ma, Y. Zare and K. Y. Rhee, Nanoscale Res. Lett., 2017, 12, 1–7 CrossRef CAS PubMed.
- N. Rajabi, M. Kharaziha, R. Emadi, A. Zarrabi, H. Mokhtari and S. Salehi, J. Colloid Interface Sci., 2020, 564, 155–169 CrossRef CAS PubMed.
- S. S. Gharaie, S. M. H. Dabiri and M. Akbari, Polymers, 2018, 10, 1317–1331 CrossRef PubMed.
- C. Li, C. Mu, W. Lin and T. Ngai, ACS Appl. Mater. Interfaces, 2015, 7, 18732–18741 CrossRef CAS PubMed.
- S. Mitra, A. Ghanbari-Siahkali, P. Kingshott, S. Hvilsted and K. Almdal, Mater. Chem. Phys., 2006, 98, 248–255 CrossRef CAS.
- S. Suresh, S. Karthikeyan and K. Jayamoorthy, J. Sci.: Adv. Mater. Devices, 2016, 1, 343–350 Search PubMed.
- G. A. Valencia, C. G. Luciano, R. V. Lourenço, A. M. Q. B. Bittante and P. J. do Amaral Sobral, Food Packag. Shelf Life, 2019, 19, 24–30 CrossRef.
- N. Bodenberger, D. Kubiczek, I. Abrosimova, A. Scharm, F. Kipper, P. Walther and F. Rosenau, Biotechnol. Rep., 2016, 12, 6–12 CrossRef PubMed.
- L. Tao, L. Zhonglong, X. Ming, Y. Zezheng, L. Zhiyuan, Z. Xiaojun and W. Jinwu, RSC Adv., 2017, 7, 54100–54110 RSC.
- J. Li, Z. Tian, H. Yang, L. Duan and Y. Liu, J. Appl. Polym. Sci., 2023, 140, e53366 CAS.
- V. de A. M. Gonzaga, A. L. Poli, J. S. Gabriel, D. Y. Tezuka, T. A. Valdes, A. Leitão, C. F. Rodero, T. M. Bauab, M. Chorilli and C. C. Schmitt, J. Biomed. Mater. Res., Part B, 2020, 108, 1388–1397 CrossRef PubMed.
Footnote |
† These authors contributed equally. |
|
This journal is © The Royal Society of Chemistry 2023 |
Click here to see how this site uses Cookies. View our privacy policy here.