DOI:
10.1039/D2MA01026G
(Paper)
Mater. Adv., 2023,
4, 1011-1020
Optical and X-ray attenuation properties of hafnium oxide nanoparticles surface functionalized with fucoidan: toward the early diagnosis of atherothrombotic diseases†
Received
10th November 2022
, Accepted 14th January 2023
First published on 18th January 2023
Abstract
Atherothrombosis is the main cause of death worldwide. An early diagnosis of this pathology is necessary to avoid serious clinical events. In this study, we report hafnium oxide nanoparticles (HfO2 NPs) designed as a CT contrast agent for an emerging imaging technology named spectral photon counting computed tomography (SPCCT). SPCCT allows the specific detection and quantification of the contrast agent concentration, according to its K-edge. HfO2 NPs were surface functionalized with fucoidan, a sulfated polysaccharide that has high affinity for P-selectin considered as a glycoprotein biomarker in atherothrombosis, and citrate ions as a control ligand. The physicochemical characterization of fucoidan coated HfO2 NPs (HfO2@fucoidan NPs) was finely studied, and the influence of the coating agent on the UV absorption and photoluminescence properties was evidenced. The in vitro flow adhesion assay showed the specific binding of HfO2@fucoidan NPs to activated platelets under arterial flow. These NPs showed excellent biocompatibility on the HUVEC cell line and the enhancement of cell viability. Phantoms performed with SPCCT show that the value of the attenuation rate (A. R.) of HfO2@fucoidan NPs (27 HU ml mg−1) is greater (×1.7) than that of HfO2@citrate NPs (17 HU ml mg−1). A good correlation between the measured concentration and the references was obtained. These synthesized HfO2@fucoidan NPs appear as a new candidate for X-ray imaging for the molecular-scale diagnosis of atherothrombosis.
Introduction
Atherothrombotic events represent the main cause of mortality and morbidity in the world,1,2 involving both the arterial and the venous part of the circulation: myocardial infarction, ischemic stroke, aneurysms, peripheral arterial disease, atrial thrombus and embolisms, phlebothrombosis and pulmonary embolisms. Many thrombotic events could be diagnosed before dramatic complications occur. By using an adequate molecular targeting strategy (e.g., molecules that bind specifically to thrombi), thrombotic sites could be diagnosed early, thereby representing a crucial indicator in the management of thrombosis through preventive therapies. However, the presence of thrombi does not immediately lead to the total obstruction of the blood vessels. Instead, non-occluding parietal thrombus often occurs.3 However, not all pathological sites are at risk of catastrophic events. Adequately and efficiently diagnosed at an early stage, non-occluding thrombi could represent an appropriate indicator for thrombotic diseases. Hence, new diagnostics could be based on visualizing thrombus at the diseased vascular wall rather than just assessing risk factors.4,5 Detecting at an early stage the preliminary events of thrombosis would represent a significant advance in cardiovascular medicine. Platelet activation and aggregation is one of the main “molecular signatures” of thrombotic diseases, and represents a major link between inflammation, thrombosis and atherogenesis. P-selectin, expressed at the surface of platelets and endothelial cells upon activation, belongs to a family of glycoproteins whose carbohydrate moieties are known to play a critical role in the physio-pathological processes such as cell–cell interactions, cell growth, lymphocyte trafficking, thrombosis or inflammation.6,7 By linking innate immunity with coagulation in the cardiovascular system, P-selectin represents an important molecular target for imaging and therapeutic approaches to intravascular clots in vivo.8,9 Therefore, numerous efforts have been devoted to the development of contrast agents for the imaging of P-selectin.10–12 These agents were either P-selectin antibodies or synthetic mimics of sialyl Lewis X (SLeX), the natural ligand of P-selectin.13,14 Although effective, the cost of synthesis of these agents, including raw materials, and the use of antibodies limit the potential translation to clinical use. Several sulfated polysaccharides have also been described to bind to P-selectin. Among them, fucoidan is a polysulfated L-fucose mainly extracted from brown seaweed endowed with biological activities closely connected to its chemical composition, to the distribution of the sulfate groups along the polyfucose backbone and to its molecular weight.15 Fucoidan is a naturally occurring mimic of SLeX16,17 and exhibits in vitro nanomolar affinity for immobilized P-selectin as well as the lowest nonspecific binding.18 99mTc-radiolabeled fucoidan as well as fucoidan coated on ultrasmall superparamagnetic iron oxide nanoparticles (NPs) enabled the detection of vascular thrombi by scintigraphy and MRI, respectively, in rat models of thrombosis and heart ischemia.19,20
Hafnium oxide (HfO2) nanoparticles (NPs) are considered for a large variety of applications including optoelectronic, photocatalysis, scintillator, UV photosensor and biomedical applications.21–26 In nanomedicine, HfO2 NPs are mainly used as radiosensitizers in cancer radiotherapy27–30 (NBTXR3, Nanobiotix, phase II–III clinical trial) as contrast agents for diagnostic X-ray imaging,26,31–33 biosensors,26 or radioluminescent scintillators.34
In this work, we will explore the potentiality of HfO2 NPs as computed tomography (CT) contrast agents for the molecular imaging of atherothrombosis with K-edge imaging enabled by spectral photon counting CT (SPCCT) imaging. SPCCT is an emerging CT modality in the clinical field that can detect specifically and quantify accurately a large variety of atoms with high Z atomic numbers (including 72Hf) by using the K-edge technique.35–43 In addition, this modality allows a significant improvement of the spatial resolution in comparison to standard CT, for improving the depiction of small structures such as calcification, ulceration or thrombus encountered in different atherosclerotic processes.44–48
In a previous paper,49 we optimized the synthesis of HfO2 NPs through a sol–gel method associated with a microwave via the benzyl alcohol route, with direct extraction and stabilization in water without any post-modification treatment. The monoclinic m-HfO2 NPs, with an ellipsoidal shape (average major and minor axes of 8 and 4 nm, respectively), show strong absorption in the UV range and photoluminescence properties related to the presence of oxygen vacancies. Herein, the NP surface was functionalized using an electrostatic approach for the direct and one-step complexation with fucoidan (HfO2@fucoidan) or with citrate ions (control NPs, HfO2@citrate). The efficiency of the surface functionalization was carefully characterized using various physicochemical techniques (Raman and infrared spectroscopy, thermogravimetry analysis (TGA) and dynamic light scattering (DLS)). Interestingly, the UV-vis absorption spectrum of HfO2@fucoidan presents a high extinction coefficient in the UV range compared to the absorption spectrum of the non-functionalized and control NPs. In addition, exaltation (by a factor 8) of the photoluminescence (PL) emission spectrum was observed for the HfO2@fucoidan NPs. Such an effect was related to an increase of oxygen vacancy concentration after fucoidan surface functionalization. After incubation with human umbilical vein endothelial cells (HUVECs), transmission electron microscopy (TEM) images show better internalization of control NPs compared to HfO2@fucoidan NPs. Concomitantly 50% cell inhibition was observed after incubation with 42 mM citrate NPs whereas for fucoidan-NPs cell viability remains at 100%. The in vitro flow adhesion assay under arterial blood flow conditions shows the specificity of the interaction between activated platelets and HfO2@fucoidan NPs. SPCCT Phantom imaging indicated a higher value of the attenuation rate (A. R.) for HfO2@fucoidan NPs (factor 1.7) compared to HfO2@citrate NPs at 120 kVp. To our knowledge, no studies report the effect of surface functionalization of HfO2 NPs on CT attenuation using an SPCCT system. Such an effect was correlated with different mass attenuation coefficients for HfO2@fucoidan NPs and HfO2@citrate NPs related to different mass fractions of citrate and fucoidan.
Experimental
Materials
The reagents for nanoparticle synthesis were hafnium(IV) chloride (HfCl4, 99.9%) from Alfa Aesar and benzyl alcohol (99%) from Acros Organics. The reagents were stocked in a glovebox (MB-Easylab V2.0 Braun) under a controlled atmosphere (O2 < 0.5 ppm, H2O < 0.5ppm). The NP water phase transfer was performed using dichloromethane (99%, Fisher Scientific). The pH was adjusted using hydrochloric acid (1 M) or sodium hydroxide (1 M) from Carlo Erba.
Low molecular weight fucoidan (batch #ASPHY12399) from the brown seaweed Ascophyllum nodosum was purchased from Algues & Mer (Ile d’Ouessant, France). Fucose and sulfate contents were 43 and 26, respectively (% w/w), with Mn = 4100 g mol−1 and Mw = 10
800 g mol−1. Citric acid and rhodamine 123 were purchased from Sigma-Aldrich and Fisher Scientific, respectively. 1-Ethyl-3-(3-dimethylaminopropyl)carbodiimide (EDC), N-hydroxysuccinimide (NHS), and N ethyl diisopropylamine were purchased from Fisher Scientific.
HfO2-fucoidan-NP synthesis and surface functionalization
Non-coated NPs were synthesized by the reaction of hafnium(IV) chloride (140 mM with benzyl alcohol (10 ml) at 200 °C for 3 h under microwave irradiation, on a Monowave 300 from Anton Paar (Anton Paar GmbH, Graz, Austria) according to a procedure already described.49 To coat the NPs with fucoidan, HfO2 NPs dispersed in water at pH = 2 ([HfO2] ≈ 100 mM) were mixed with various amounts of fucoidan dissolved in water (pH = 7) under stirring for 2 hours, corresponding to a ratio R between 0 and 25, with R = 1000 ([fucoidan]/[HfO2]). The HfO2@fucoidan NPs were washed three times by ultracentrifugation (Amicon 100 kDa, Merck Millipore) and suspended in water. The pH was adjusted to 7.4 by the addition of NaOH (1 M).
The HfO2 NPs were also surface functionalized with citrate ions and used as control NPs. HfO2 NPs were dispersed in water at pH = 2 ([HfO2] ≈ 100 mM) and mixed for 2 hours under magnetic stirring with citric acid dissolved in water (pH = 3) with a mass ratio of 2 between the ligand and the NPs. After the reaction, the pH was adjusted to 7.4 by the addition of NaOH (1 M) and the NPs-citrate was washed three times by ultracentrifugation (Amicon 100 kDa, Merck Millipore) and suspended in water (pH = 7.4).
The NPs were also labelled with rhodamine 123. The carboxylate functions at the outer surface of HfO2@fucoidan and HfO2@citrate NPs ([Hf] = 50 mM) were activated using 1-ethyl-3-(3-dimethylaminopropyl)carbodiimide (EDC) and N-hydroxysuccinimide (NHS), [EDC] = [NHS] = 13 mM) at pH = 4, stirred for 2 h at 37 °C. The second step was the linkage of the amine function of Rhodamine-123 with the activated carboxylic acid functions on the NPs. The pH of the NP samples was adjusted to pH = 9 with N ethyl diisopropylamine. The Rhodamine-123 was dissolved in water at pH = 9 (adjusted with N-ethyl diisopropylamine) and then added to the samples and stirred for 24 h, under ambient temperature. The modified particles were washed 8 times by ultracentrifugation (Vivaspin 50 kDa) with deionized water. The NPs were redispersed in water at physiological pH.
Physicochemical characterization
The Hf concentration was determined by the freeze drying process as described in a previous work49 and by total reflection X-ray fluorescence spectrometry (TXRF, S2 PICOFOX, Brucker). For TXRF, calibration curves were first obtained using HfO2@fucoidan or HfO2@citrate solution (concentration of the stock solution determined after the freeze drying process), Fig. S1 (ESI†). TXRF data were collected over 300 s with a molybdenum tube excitation source operating at 50 kV/700 μA. Elemental concentrations were calculated using gallium standard solution (1 g L−1, Kraft GmbH) for each sample through the PICOFOX™ software included in the equipment.
The hydrodynamic size and zeta potential of the NPs were investigated by dynamic laser light scattering (DLS) at a fixed attenuator of 6 and a center position of 4.65 mm, using a Nano-ZS (Red Badge) ZEN 3600 device (Malvern Instruments, Malvern, UK). TEM images were obtained using an FEI Tecnai 12 (Philips), and the samples were prepared by depositing 10 μL of NP suspension on carbon-coated copper grids placed on a filter paper. The median diameter is deduced from TEM data measurements, simulating the diameter distribution with a log-normal function. The grafting of the fucoidan to the surface of the NPs was studied by Fourier transform infrared (FTIR) analysis, Raman analysis and thermogravimetric analysis (TGA). The FTIR spectra were recorded on a Bruker Tensor 27 FTIR spectrometer using the KBr pellet technique. The Raman measurements were performed on an Xplora spectrometer (Horiba Scientific-France) with a 660 nm excitation wavelength (diode laser). The sample as a solution was deposited on a microscopy glass and evaporated at 80 °C for 24 h. The spectra were recorded using a macro-objective with a magnification of 100× (NA = 1.2). To obtain a high resolution, a grating of 1800 T mm−1 was used. The laser power used was 1 mW. Quantification of the number of fucoidan and citrate coated per NP was evaluated by TGA using a LABSYS Evo TG-DTA-DSC 16000 device from Setaram Instrumentation. The UV-visible and fluorescence properties of the NPs were studied using a V-630 UV-VIS Spectrophotometer (Jasco) and a Spex FluoroMax spectrofluorometer equipped with a Hamamatsu 928 photomultiplier (HORIBA Jobin Yvon, Villeneuve D’Ascq, France), respectively.
Cell culture and cytotoxicity assay
The cytotoxicity of the HfO2@fucoidan and HfO2@citrate were evaluated on Human Umbilical Vein Endothelial cells (HUVECs) using the resazurin test. The cells were grown in DMEM supplemented with 10% (v/v) fetal bovine serum, 4 mmol L-glutamine, 100 U ml−1 penicillin, and 100 μg ml−1 streptomycin and kept in an incubator at 37 °C in a humidified atmosphere of 5%. The cells were seeded at 10
000 cells per well in a 96-well plate. After 24 h of incubation, the cell culture medium was removed and replaced by 200 μl of NP solution (in NaCl 0.9%) at various concentrations ranging from 0 (positive control) to 42 mM. The cells were incubated for another 24 h. To measure the cell metabolic activity, the medium was removed and replaced with 100 μL of Resazurin solution (10%), and the plates were covered in foil and incubated for 2 h. The fluorescence signal of Resazurin was monitored using 540 nm excitation and 590 nm emission wavelengths on an Infinite® 200 PRO microplate reader (TECAN Group Ltd, Mannedorf, Switzerland). The obtained fluorescence (Fl) values were blank corrected, and the relative cell viability was expressed as (FlNPs/Flcontrol) × 100%, where Flcontrol was obtained in the absence of the NPs. The experiment was performed in hexaplicate.
Transmission electron microscopy (TEM) of NP-loaded HUVEC cells
For TEM imaging, 2 × 106 HUVEC cells were cultured in a 6-well plate. After 24 h of incubation, the cell culture medium is removed and replaced with 1 ml of HfO2@fucoidane or HfO2@citrate (2 mM, 12 mM and 32 mM) for another 24 h of incubation. The cells were then washed with PBS and fixed for 1 h with 2% paraformaldehyde (PFA), and 1% glutaraldehyde diluted in PBS 1× and water. After 3 washes with PBS, the cells were maintained at 4 °C. For the observation with TEM, the samples were included in Epon and ultra-sectioned. The sections were deposited onto copper grids for observation with a TECNAI 12 electron microscope.
Flow microchamber experiments
An in vitro flow adhesion assay was performed to assess the binding affinity of HfO2@fucoidan for P-selectin expressed on activated blood platelets50,51 Micro-channels of Vena8 Fluoro + chambers (width: 0.04 cm, height: 0.01 cm, and length: 2.8 cm; Cellix Ltd, Dublin, Ireland) were coated overnight with 100 μg ml−1 collagen and then rinsed with NaCl 0.9%. Human whole blood was perfused under an arterial flow for 5 min (shear stress 67.5 dyne cm−2) leading to the formation of aggregated and activated blood platelets deposited on the micro-channels covered by collagen. The Rh123 labelled HfO2@fucoidan and HfO2@citrate (negative control) were injected under an arterial flow for 5 min. The binding and accumulation of the NPs were observed in real time by fluorescence microscopy (Axio Observer, Carl Zeiss Microscopy, Oberkochen, Germany). Fluorescence images were taken along each micro-channel after washing with NaCl 0.9%.
Statistical analysis
The results are expressed as the mean ± SEM of at least three independent experiments. Statistical analysis was performed using an ANOVA test. Differences were considered significant when P < 0.05.
NP phantom preparation for spectral photon-counting computed tomography (SPCCT)
The SPCCT system (Philips research and development; Haifa, ISRAEL) is a large field-of-view (50 cm in-plane) clinical prototype CT equipped with energy-sensitive photon-counting detectors (PCDs). Pixel pitch is of 275 × 275 μm 2 at the isocenter, bonded to Philips’ proprietary ChromAIX2 application-specific integrated circuit, relying on the direct conversion high band gap semiconductor of cadmium zinc telluride. Z-coverage is 17.6 mm and the minimal rotation time of the gantry is 0.33 s. Each channel offers pulse-height discrimination with five controllable energy thresholds set as 30, 51, 64, 72 and 85 keV in order to allow the energy-based discrimination of Hafnium.52 Further technical details are provided in previous studies.38,53 A custom-made polyoxymethylene cylindrical phantom with a diameter of 10 cm and 12 holes with 1 cm diameter was used. The samples were loaded into the phantom using 1.5 ml polypropylene centrifuge tubes. Two sets of 12 tubes were prepared, each containing HfO2@fucoidan or HfO2@citrate NPs diluted in glucose (5%) with various concentrations. The concentrations (mg ml−1) of the NPs depended on the corresponding attenuation at 120 kVp. The target attenuation for each solution was chosen within the clinical standard for CT angiography applications, i.e. 280 Hounsfield units (HU) approximately. Hence, the NP concentration was in the range of 0–15 mg ml−1.
Five axial scans were performed using a conventional X-ray tube with a voltage of 120 kVp and a current of 100 mA. Conventional images scaled in Hounsfield units and spectral images scaled in mg ml−1 of materials were reconstructed using 1 mm slice thickness, a field-of-view of 220 mm in-plane and a matrix of 512 mm. Spectral images consisted of reconstructing three materials, i.e., 2 non-K-edge materials (water and iodine) and a K-edge Hafnium image. It is noted that a water image approximates the water content in the tissue even in the presence of contrast agents.
Results and discussion
HfO2@fucoidan NP synthesis and physicochemical characterization
Ellipsoidal HfO2-NPs with average major (2a) and minor (2b) axes of 8 and 4 nm (Fig. 1A) were synthesized using microwave non-aqueous sol–gel synthesis in a two-step process, following a procedure described previously.49 NPs were surface functionalized with fucoidan, a negatively charged polysaccharide, or with citrate, one of the most used coating agents, using an electrostatic approach for the direct and one-step complexation54 and taking care of the avidity effect of carboxylate (fucoidan, citrate) and sulfate (fucoidan) groups for the oxide surface.55 To our knowledge, we reported herein a simple process for the direct and one step complexation of the HfO2 Np surface. As shown recently,56 water soluble HfO2 NPs were obtained after ligand exchange from apolar NPs (dispersed in toluene) using at least 3 precipitation–redispersion cycles (starting from dispersion in toluene, and then re-dispersion in ethanol, and later on in methanol and finally in water). To saturate the HfO2 NP surface with fucoidan, increasing amounts of polymer (R = 1000 × [fucoidan]/[Hf]) were added to HfO2 NPs dispersed in acidic water (pH = 2, [Hf] = 100 mM). Under these conditions, hydroxyl ions onto the HfO2 surface led to a positive surface charge, increasing the electrostatic interaction and chelating bridging with the polymer after mixing. After surface functionalization, the NPs were filtered to remove the unreacted chemical species. Surface coating was evidenced by FT-IR spectra, (Fig. 1B). In the 1500–500 cm−1 region, the IR spectrum of fucoidan57 was characterized by three peaks observed at around 1257 cm−1 (O
S
O stretching), 1035 cm−1 (C–O–C stretching) and 844 cm−1 (C–O–S bending). The IR spectrum of the NPs present bands at 530, 555, 650, 680, and 750 cm−1 in good agreement with IR phonon modes predicted for the monoclinic HfO2 phase.58 After complexation with the NP surface, the IR fucoidan bands were also displayed on the spectra of HfO2@fucoidan NPs in addition to the Hf–O characteristic vibration bands. Fig. 1C shows the evolution of the IR surface band ratio A1257/A750versus R. The signal rose with the increase of the molar ratio up to R ∼ 14, to reach a plateau. This indicated an increase in the number of fucoidan molecules on the surface of the HfO2 NPs with an R ratio and saturation at R = 14. Considering the surface band ratio A1257/A750 at this saturated ratio, before and after ultrafiltration, the average number of fucoidan molecules per NP was estimated to be ∼70 fucoidan per NP (Fig. S2, ESI†).
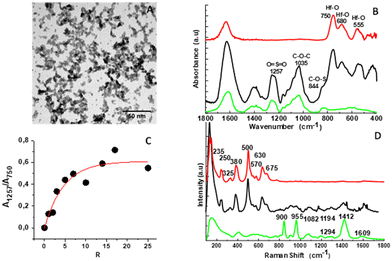 |
| Fig. 1 Physicochemical characterization of HfO2@fucoidan NPs: TEM image (A), FTIR spectra (B) and evolution of the FTIR surface bands A1257/A750versus R (C), and Raman spectra (D) of HfO2 NPs (red curve), fucoidan (green curve) and HfO2@fucoidan NPs (black curve). | |
The coating was also evidenced by Raman spectroscopy and thermogravimetric analysis (TGA). Comparison of the Raman spectra of coated NPs with those of the native polysaccharide57 and bare HfO2 NPs evidenced the organic layer around the NP, Fig. 1D. The TGA thermogram (Fig. S3, ESI†) exhibits a two-step decomposition: (i) the removal of water up to 200 °C that was estimated to be 3.25% (w/w) and (ii) the consecutive full degradation of the organic layer at around 900 °C. The overall weight loss (9.75% (w/w) for HfO2@fucoidan NPs allowed the estimation of an average quantity of molecules per NP approximated to 65 in good accordance with the IR result.
The Raman and FTIR spectra of control NPs (HfO2@citrate NPs) show the characteristic citrate and Hf–O bands, indicating an effective citrate surface complexation (Fig. S4, ESI†). The TGA thermogram (Fig. S5, ESI†) indicates an average of 800 citrate per NP. Considering an average number of 1440 hafnium onto the NP surface, this indicates that, as observed with iron oxide NPs,59 two carboxylate functions per molecule are involved in two hafnium surface complexation and that the surface is fully saturated by citrate ions.
After coating, the NPs exhibit a hydrodynamic diameter at physiological pH (in intensity) of 58 nm (PdI = 0.2) and 33 nm (PdI = 0.4), and a negative charge surface of −27 mV and −33 mV for HfO2@fucoidan NPs and HfO2@citrate-NPs, respectively (Fig. S6, ESI† for pH stability). The NPs were also dispersed in glucose (5%) or in NaCl (0.9%), Fig. S7 (ESI†). A slight increase of the hydrodynamic diameter in the two media and after 1 day was observed for HfO2@fucoidan NPs whereas the size does not change for HfO2@citrate-NPs (Fig. S7, ESI†). In addition, better stability with time (measurement of the hydrodynamic diameter after 10 days) is observed for both NPs in glucose compared to NaCl medium (Fig. S7, ESI†).
Influence of surface functionalization on the optical properties
Fig. 2 presents the UV-vis absorption and photoluminescence PL emission (PLem) spectra of the HfO2 (pH = 2, [HfO2] = 1 mM), HfO2@citrate and HfO2@fucoidan NPs (PH = 7.4, [HfO2] = 1 mM). The UV-visible spectra of HfO2 and HfO2@citrate were similar whereas the HfO2@fucoidan sample presents a high extinction coefficient in the UV range, Fig. 2A. Considering an average of ∼70 fucoidan per NP, a concentration of HfO2 equal to 1 mM corresponds to about 6 μM fucoidan. Fig. 2A inset shows the absorption spectrum of fucoidan at a concentration of 6 μM. Clearly, the higher molar attenuation coefficient is not related to the UV absorption of fucoidan. This increase of UV absorption was observed in the case of HfO2 films with reduced oxygen content and was attributed to the higher concentration of oxygen vacancies.60,61
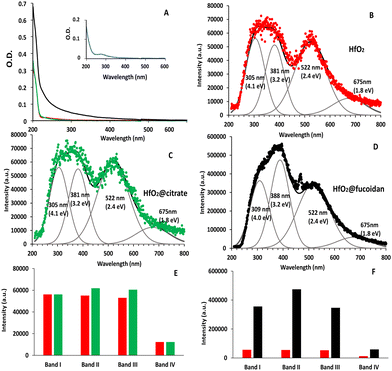 |
| Fig. 2 (A) UV-Visible absorption spectrum of HfO2 (red curve [HfO2] = 1 mM, pH = 2), HfO2@fucoidan (black curve) and HfO2@citrate (green curve) NPs ([HfO2] = 1 mM, pH = 7). Inset: UV-Visible absorption spectra of fucoidan in water ([fucoidan] = 6 μM, pH = 7). (B–D) PL emission and deconvolution of the PLem spectrum using a Gaussian fit (grey curve) for HfO2 (red curve, B), HfO2@citrate (green curve, C) and HfO2@fucoidan (black curve, D) NPs ([Hf] = 1 mM, λex = 200 nm. (E and F) comparison of the evolution of the PL intensity bands between (E) HfO2 (red bars) and HfO2@citrate (green bars) NPs and (F) HfO2 (red bars) and HfO2@fucoidan (black NPs). | |
Polysaccharides are used as a reducing agent as well as a surface stabilizing template for the NPs.62 One explanation63 could be that more Hf3+ species are formed by the reduction function of fucoidan compared to sodium citrate. This higher ratio of Hf3+/Hf4+ for HfO2@fucoidan compared to HfO2@citrate results in the creation of more surface oxygen vacancies to maintain charge balance.
After excitation at 200 nm wavelength, the PLem spectra of HfO2 and HfO2@citrate were similar and present two emission bands centered at 325 (3.8 eV, blue transition) and 522 nm (2.4 eV, middle red transition) Fig. 2B and C. This PLem profile was attributed, in a previous paper,49 to the oxygen vacancies inducing defect levels located deep in the band gap. The PLem spectra for nude NPs were deconvolved with 4 Gaussian fits centered at 305 nm (4.1 eV, band I), 381 nm (3.2 eV, band II), 522 nm (2.4 eV, band III) and 675 nm (1.8 eV, band IV). The emission peaks at 381 nm and 522 nm were assigned to VO3 with recombination to the valence band and to the defect level, respectively, and the band at 675 nm to the presence of interstitial oxygen defects. The PL component centered at 305 nm was either assigned to the vibronic transition of the excited OH˙* radical (hydroxyl ions at the surface of the NPs) or to the self-trapped exciton.64–66 Considering that the latter band was already observed after fucoidan or citrate surface functionalization, this favors the hypothesis of the self-trapped exciton. For HfO2 the intensity of the bands at 305 nm, 381 nm and 522 nm were quite similar and the contribution of the 675 nm band is lower (≈80% lower compared to bands I and III) (Fig. 2B and E). After surface functionalization with citrate, a slight increase of the intensity of bands II and III was observed whereas the intensity of the other bands (I and IV) does not change compared to HfO2 (Fig. 2E). An exaltation of the emission (by a factor 8) was observed for HfO2@fucoidan NPs (Fig. 2F) and the contribution of the band II increased. The best deconvolution of the PLem spectrum was obtained with 4 Gaussian fits centered at 309 nm (4.0 eV, band I), 388 nm (3.2 eV, band II), 522 nm (2.4 eV, band III) and 675 nm (1.8 eV, band IV), Fig. 2D. Hence the positions of bands I and II are slightly red shifted compared to those of HfO2 and HfO2@citrate NPs. In addition, the intensity of bands I and III remains quite similar whereas band II increases by a factor of ≈31%. Finally, the contribution of band IV remains ≈80% lower compared to bands I and III as observed for HfO2 and HfO2@citrate NPs.
The proportions of each defect were estimated thanks to the area of each Gaussian function,67Table 1. Briefly, the amount of self-trapped exciton (band 1) and interstitial oxygen defects (band IV) is found to be constant in the three samples with mean percentages of 25% and 9%, respectively. The proportion of band II (VO3 with recombination to the valence band) is quite similar (24%) for nude and citrate coated NPs and increases for HfO2@fucoidan NPs (31%). Finally, the proportion of band III (VO3 with recombination to the defect level) is again quite similar (42%) for nude and citrate coated NPs and slightly decreases for HfO2@fucoidan NPs (38%). These results suggest that band II is related to surface oxygen vacancies.
Table 1 Results of the multi-Gaussian models of fluorescence emission spectra. The percentage of each contribution is estimated by the area at each wavelength over the total area
% |
Band I (305 nm) |
Band II (381 nm) |
Band III (521 nm) |
Band IV (675 nm) |
HfO2 |
25 |
25 |
41 |
9 |
HfO2@citrate |
24 |
24 |
43 |
9 |
HfO2@fucoidan |
24 |
31 |
38 |
7 |
The efficiency of a PL emission is contributed to both by radiative and non-radiative recombination processes. Radiative recombination, related to oxygen vacancy concentration, increases the PL intensity while non-radiative recombination induces the opposite effect. The non-radiative transition is initiated by defects present on the surface.67 It is well known that the passivation of some surface defects by the capping ligands could affect wavelength shifts and variations of the intensities of the emission bands.68,69 Surface defects act as the centers of non-radiative recombination for electrons and holes and promoting their passivation can raise the probability of radiative transitions.
Comparing HfO2@citrate and HfO2@fucoidan versus HfO2 NPs, the large difference in emission intensity in favor of the HfO2@fucoidan NPs could be explained by an increase of surface oxygen vacancy concentration. This effect could be due to the more effective reductive effect of fucoidan compared to citrate and to a better complexation of the surface of the NP with the sulfate groups of fucoidan with respect to the carboxylate groups of citrate.70
Influence of surface functionalization on HUVEC cell internalization and cytotoxicity
We first studied the HfO2@fucoidan and HfO2@citrate NPs (dispersed in NaCl 0.9%) internalization on stimulated fetal bovine serum-(SVF) human umbilical vein endothelial cells (HUVECs) using TEM. The cells were incubated for 24 h with three extracellular Hf concentrations: 2 mM, 12 mM and 32 mM. TEM imaging of the cells containing both types of NPs, one day after their internalization is shown in Fig. 3. They evidence (i) the endosomal confinement of the NP, with no differences in the intracellular location observed depending on the coating, (ii) the NP cell internalization increases upon increasing the incubation concentration as observed by us and others, (iii) better internalization of HfO2@citrate NPs compared to HfO2@fucoidan NPs. This latter effect can be related to high aggregation of HfO2@citrate dispersed in NaCl 0.9% and DMEM or in NaCl 0.9%–DMEM− + SVF 10%, compared to HfO2@fucoidan NPs as shown in Fig. S8 (ESI†).
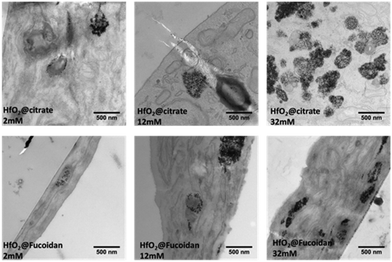 |
| Fig. 3 TEM images of the HUVEC cell line incubated with HfO2@citrate and HfO2@fucoidan at different extracellular concentrations (2 mM, 12 mM, 32 mM) for 24 h. | |
To evaluate the cytotoxicity of the NPs, the cell viability assay (Resazurin) was performed on the HUVEC cell line. After 24 h of NP incubation, as shown in Fig. 4, no toxicity was observed for HUVECs from 2 to 32 mM for both NPs, as compared to untreated cells. From 0 mM to 6 mM, there is no significant difference in cell viability with untreated cells. For HfO2@citrate, an increase of cell viability is observed at 8 mM, 12 mM and 24 mM with a significant and maximum enhancement observed at 12 mM (factor 1.8). For HfO2@fucoidan a maximum enhancement of the cell viability is observed at 12 mM and 32 mM (factor 2.6). For higher concentrations, this phenomenon disappears, and 50% cell inhibition was observed after incubation with 42 mM citrate NPs, whereas for fucoidan-NPs the cell viability remains 100%. This increase of cell viability with both NPs can be related to the interaction with cell membrane receptors and/or growth factors, thereby activating mitogenic signals or repressing growth-inhibitory signals. Beyond 8 mM and up to 32 mM, this effect seems to be stopped with citrate NPs and not with fucoidan NPs. In the latter case, it has been shown that fucoidan alone stimulated fetal bovine serum-induced HUVEC proliferation.71 The mechanism of vascular cell growth modulation is still unknown, but it was postulated that fucoidan potentiates the fibroblast growth factor-1 FGF-1 mitogenic activity by stabilizing the growth factor and inhibiting FGF-2 mitogenic activity. Both effects occurred in a concentration-dependent manner. Hence, considering that the cell internalization of HfO2@citrate NPs seems to be higher compared to HfO2@fucoidan NPs (as observed in Fig. 3), the toxicity of HfO2@fucoidan NPs will appear at higher extracellular concentrations and is counterbalanced by the fucoidan stimulation effect.
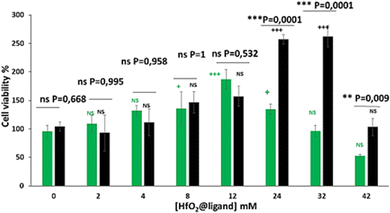 |
| Fig. 4 HUVEC cell viability after 24 h of incubation of various extracellular concentrations of HfO2@citrate (green bars) and HfO2@fucoidan (black bars) NPs. | |
Binding of HfO2-fucoidan NPs on P-selectin in vitro
To evaluate the ability of HfO2@fucoidan NPs to target P-selectin under arterial blood flow conditions, it was necessary to label the NPs with a fluorophore to visualize and quantify in real-time the NP adhesion under fluorescence microscopy. The amine function of Rhodamine 123 (Rh123) was covalently linked to carboxylate functions at the outside NP surface using conventional carbodiimine chemistry, using the same conditions for both NPs (see the Experimental section). The dye labelling was more efficient (5-fold) for HfO2@fucoidan NPs compared to HfO2@citrate NPs, so arterial blood flow experiments (Fig. 5) were performed at the iso-nanoparticle concentration ([Hf] = 25 mM) and iso-fluorescence intensity (HfO2@fucoidan NPs: [Hf] = 5 mM and HfO2@citrate NPs: [Hf] = 25 mM).
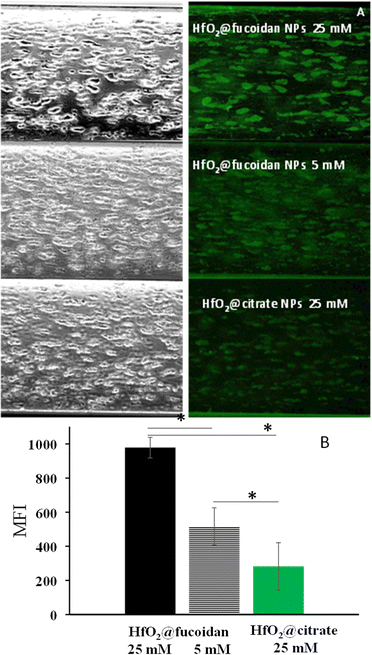 |
| Fig. 5 Nanoparticle adhesion over platelet aggregates. Platelets adhered onto the channel wall forming aggregates expressing P-selectin (left column) and Rh123 labelled HfO2@fucoidan NPs ([Hf] = 25 mM (top), 5 mM (middle)) and HfO2@citrate NPs ([Hf] = 25 mM) were then injected for 5 minutes (right column). Channels were washed with 0.9% NaCl. (B) Quantitative analysis of the mean fluorescence intensity of platelet aggregates (n = 3 for both NPs), *P < 0.05. | |
When passed at the arterial flow on the platelet aggregates, Rh123 labelled HfO2@fucoidan NPs bound to the aggregates and remained attached, whereas Rh123 labelled HfO2@citrate NPs showed almost no binding (Fig. 5A). Quantitative analysis after 5 minutes confirmed that the mean fluorescence intensity (MFI) of fluorescent NPs was significantly higher for HfO2@fucoidan NPs ([Hf] = 25 mM, MFI = 978 ± 61; ([Hf] = 5 mM, MFI = 515 ± 109) than for HfO2@citrate NPs ([Hf] = 25 mM, MFI = 282 ± 138) (Fig. 5B). In addition, by decreasing the average number of HfO2@fucoidan NPs (5-fold) it is clearly observed that HfO2@fucoidan NPs adhered significantly more onto activated platelets than control NPs.
In vitro phantom imaging
Fig. 6 shows the spectral photon-counting images of conventional images (A and D), K-edge images of Hafnium (purple, B and E), and fusion between the K-edge and conventional images (C and F) for HfO2@citrate NPs (top row) and HfO2@fucoidan NPs (bottom row).
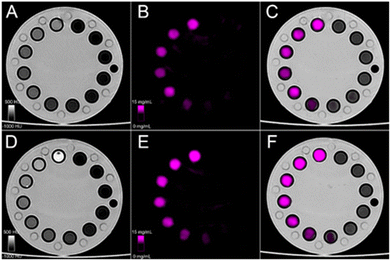 |
| Fig. 6 Spectral photon-counting images of conventional images (A and D), K-edge images of Hafnium (purple, B and E), and fusion between K-edge and conventional images (C and F) for HfO2@citrate NPs (top row) and HfO2@fucoidan (bottom row). | |
Conventional images provide an image of the phantom, the tubes as well as the attenuation values (Fig. 7A) while the K-edge image provides a specific and absolute quantification of the hafnium concentration found in the NPs (Fig. 7B). There was an excellent linear correlation with an R2 value very close to unity. The value of the attenuation rate (A. R.) of HfO2@fucoidan NPs (27 HU ml mg−1) is greater (×1.6) than that of HfO2@citrate NPs (17 HU ml mg−1). Such a decrease of A.R. value with HfO2@citrate NPs might be related to different mass attenuation coefficients for HfO2@fucoidan NPs and HfO2@citrate NPs. The photon mass attenuation coefficients are generally expressed as μ/ρ, where μ is the linear attenuation coefficient (in cm−1) and ρ is the material density (in g cm−3). The density of a composite made of HfO2 Nps surface functionalized with ligands (fucoidan and citrate) is ρc = ρligands·wligands + ρHfO2·wHfO2s where wligands and wHfO2 are the volume fractions of ligands and HfO2 NPs. Considering an average of 65 fucoidan molecules and 800 citrate molecules per NP, a large difference in the molecular weight of the two ligands, it can be considered that the density of the composite is different considering HfO2@fucoidan NPs and HfO2@citrate NPs. For HfO2@fucoidan NPs, the correlations between the measured and prepared concentrations, Fig. 7B, were strongly linear for all dilutions (R2 ≥ 0.98). The slope (0.52) was slightly underestimated for citrate. Compared to the commercial gadolinium based molecular agent, DOTAREM® (gadoterate meglumine; Guerbet, Villepinte, France), with an attenuation rate (A. R.) equal to 32.5 HU ml mg−1 (Fig. S9, ESI†), the attenuation rate of HfO2@fucoidan NPs is on the same order of magnitude (A. R. = 27 HU ml mg−1). In addition to specific targeting for the P-selectin biomarker, HfO2@fucoidan NPs appear to be an efficient X-ray contrast agent.
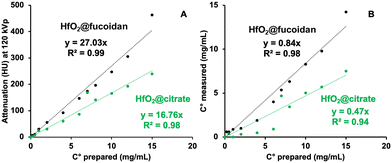 |
| Fig. 7 Left: Attenuation rates measured for HfO2@fucoidan (black) and HfO2@citrate (green) with an SPCCT system. Right: Comparison between the expected and measured concentrations with K-edge imaging for HfO2@fucoidan (black) and HfO2@citrate (green) | |
Conclusions
Atherothrombosis is the first cause of death worldwide. Early detection of atherothrombotic lesions remains a big medical challenge. In this work we have developed HfO2 NPs as computed tomography contrast agents for the detection of atherothrombosis. The nude HfO2 NPs were successfully functionalized with fucoidan or citrate and exhibited good colloidal stability in water or glucose. The fucoidan functionalized NPs allowed specific targeting of aggregated platelets under a dynamic flow test, and very good biocompatibility was demonstrated through an in vitro cytotoxicity assay, for high extracellular concentrations. The internalization inside the cells was confirmed with transmission electron microscopy and showed higher internalization of citrate NPs compared to fucoidan. Enhancement of the UV absorption and photoluminescence properties was observed with HfO2-fucoidan NPs and was related to an increase of oxygen vacancies due to the reductive effect of fucoidan. The proof of concept for an efficient X-ray attenuation was illustrated through in vitro phantom imaging, with spectral photon counted computed tomography. The better attenuation rate observed with the fucoidan functionalized NPs compared to citrate evidenced the impact of the ligand surface on X-ray attenuation. These results confirm the ability of fucoidan coated HfO2 NPs as potential new contrast agents for X-ray imaging and particularly for the detection of atherothrombosis.
Author contributions
Conceptualization and project administration: L. M. and P. O.; funding acquisition: P. O. and F. C.; investigation: Y. S., S. S.-M., R. A., and M. C.; data curation: Y. S. and S. S.-M., L. M.; formal analysis: Y. S., S. S.-M., F. G., and L. M.; resources: P. O., F. C., L. M., S. S.-M., and Y. L.; supervision: L. M. and P. O.; validation: Y. S., S. S.-M., R. A., and M. C.; visualization: Y. S. and L. M.; writing – original draft: Y. S. and L. M.
Conflicts of interest
There are no conflicts to declare.
Acknowledgements
The authors would like to acknowledge Mohammad Varasteh, PhD, CREATIS, UMR 5220, Univ Lyon, INSA Lyon, University Claude Bernard Lyon 1, Lyon, France, to perform the spectral photon-counting images of the commercial gadolinium based molecular agent: DOTAREM®, NanoMat platform of the Sorbonne Université Paris Nord for the use of UV-vis, Zetasizer Nano ZS, the ImagoSeine core facility of the Institut Jacques Monod (R. Le Borgne and C. Durieu), member of IBiSA and France-BioImaging (ANR-10-INBS-04) infrastructures for TEM imaging. We warmly thank ANR to have supported our project ANR-16-CE18-0031.
References
- D. L. Steen, I. Khan, K. Andrade, A. Koumas and R. P. Giugliano, J. Am. Heart Assoc., 2022, 11, e022198 CrossRef PubMed.
- J. Lin, Y. Chen, N. Jiang, Z. Li and S. Xu, Front. Cardiovasc. Med., 2022, 9, 868370 CrossRef CAS PubMed.
- K. Fujii, T. Seki, Y. Nakata, K. Atagi and T. Matsuyama, Surg. Case Rep., 2022, 8, 36 CrossRef PubMed.
- B. Guo, Z. Li, P. Tu, H. Tang and Y. Tu, Front. Cardiovasc. Med., 2021, 8, 692915 CrossRef CAS PubMed.
- J. Sanz and Z. A. Fayad, Nature, 2008, 451, 953–957 CrossRef CAS PubMed.
- K. Stark and S. Massberg, Nat. Rev. Cardiol., 2021, 18, 666–682 CrossRef PubMed.
- G. Baidildinova, M. Nagy, K. Jurk, P. S. Wild, H. ten Cate and P. E. J. van der Meijden, Front. Cardiovasc. Med., 2021, 8, 684920 CrossRef CAS PubMed.
- J. W. Semple and J. Freedman, Cell. Mol. Life Sci., 2010, 67, 499–511 CrossRef CAS PubMed.
- K. Pluta, K. Porébska, T. Urbanowicz, A. Gsecka, A. Olasinska-Wisniewska, R. TargoÅ„ski, A. KrasiÅ„ska, K. J. Filipiak, M. Jemielity and Z. KrasiÅ„ski, Biology, 2022, 11, 224 CrossRef CAS PubMed.
- D. Ganesh, P. Jain, C. D. Shanthamurthy, S. Toraskar and R. Kikkeri, Front. Chem., 2021, 9, 773027 CrossRef CAS PubMed.
- H. Liu, G. Pietersz, K. Peter and X. Wang, J. Nanobiotechnol., 2022, 20, 75 CrossRef CAS PubMed.
- X. Wang, M. Ziegler, J. D. McFadyen and K. Peter, Br. J. Pharmacol., 2021, 178, 4246–4269 CrossRef CAS PubMed.
- C. Mayer, D. S. Cooper, A. Redfern, X. Geng, J. Shi, M. L. van Zutphen-van Geffen, I. Kuan, K. Koeck, H. Kastrissios, K. Patel, M. Davis and P. Yue, Blood, 2021, 138, 977 CrossRef.
- R. P. McEver, K. L. Moore and R. D. Cummings, J. Biol. Chem., 1995, 270, 11025–11028 CrossRef CAS PubMed.
- K. Wang, X. Xu, Q. Wei, Q. Yang, J. Zhao, Y. Wang, X. Li, K. Ji and S. Song, Ther. Adv. Chronic Dis., 2022, 13, 20406223221076891 CAS.
- M. Kusaykin, I. Bakunina, V. Sova, S. Ermakova, T. Kuznetsova, N. Besednova, T. Zaporozhets and T. Zvyagintseva, Biotechnol. J., 2008, 3, 904–915 CrossRef CAS PubMed.
- B. Li, F. Lu, X. Wei and R. Zhao, Molecules, 2008, 13, 1671–1695 CrossRef CAS PubMed.
- L. Bachelet, I. Bertholon, D. Lavigne, R. Vassy, M. Jandrot-Perrus, F. D. R. Chaubet and D. Letourneur, Biochim. Biophys. Acta, Gen. Subj., 2009, 1790, 141–146 CrossRef CAS PubMed.
- F. Rouzet, L. Bachelet-Violette, J. M. Alsac, M. Suzuki, A. Meulemans, L. Louedec, A. Petiet, M. Jandrot-Perrus, F. Chaubet, J. B. Michel, D. Le Guludec and D. Letourneur, J. Nucl. Med., 2011, 52, 1433–1440 CrossRef CAS PubMed.
- M. Suzuki, L. Bachelet-Violette, F. Rouzet, A. Beilvert, G. Autret, M. Maire, C. Menager, L. Louedec, C. Choqueux, P. Saboural, O. Haddad, C. Chauvierre, F. Chaubet, J. B. Michel, J. M. Serfaty and D. Letourneur, Nanomedicine, 2014, 10, 73–87 CrossRef PubMed.
- W. J. Bae, M. Trikeriotis, J. Sha, E. L. Schwartz, R. Rodriguez, P. Zimmerman, E. P. Giannelis and C. K. Ober, J. Mater. Chem., 2010, 20, 5186–5189 RSC.
- R. Ambrosio, O. Arciniega, A. Carrillo, M. Moreno, A. Heredia and C. Martinez, Can. J. Phys., 2014, 92, 806–812 CrossRef CAS.
- J. Molina, A. L. Munoz, W. Calleja, P. Rosales and A. Torres, J. Mater. Sci., 2011, 47, 2248–2255 CrossRef.
- C. Liu, T. J. Hajagos, D. Kishpaugh, Y. Jin, W. Hu, Q. Chen and Q. Pei, Adv. Funct. Mater., 2015, 25, 4607–4616 CrossRef CAS.
- F. N. I. Sari, S. H. Lu and J. M. Ting, J. Am. Ceram. Soc., 2020, 103, 2252–2261 CrossRef CAS.
- T. L. McGinnity, O. Dominguez, T. E. Curtis, P. D. Nallathamby, A. J. Hoffman and R. K. Roeder, Nanoscale, 2016, 8, 13627–13637 RSC.
- S. Bonvalot, C. Le Pechoux, T. De Baere, G. Kantor, X. Buy, E. Stoeckle, P. Terrier, P. Sargos, J. M. Coindre, N. Lassau, R. Ait Sarkouh, M. Dimitriu, E. Borghi, L. Levy, E. Deutsch and J. C. Soria, Clin. Cancer Res., 2017, 23, 908–917 CrossRef CAS PubMed.
- S. Bonvalot, P. L. Rutkowski, J. Thariat, S. Carrère, A. Ducassou, M. P. Sunyach, P. Agoston, A. Hong, A. Mervoyer, M. Rastrelli, V. Moreno, R. K. Li, B. Tiangco, A. C. Herraez, A. Gronchi, L. Mangel, T. Sy-Ortin, P. Hohenberger, T. de Baère, A. Le Cesne, S. Helfre, E. Saada-Bouzid, A. Borkowska, R. Anghel, A. Co, M. Gebhart, G. Kantor, A. Montero, H. H. Loong, R. Vergés, L. Lapeire, S. Dema, G. Kacso, L. Austen, L. Moureau-Zabotto, V. Servois, E. Wardelmann, P. Terrier, A. J. Lazar, J. Bovée, C. Le Péchoux and Z. Papai, Lancet Oncol., 2019, 20, 1148–1159 CrossRef CAS PubMed.
- Y. Li, Y. Qi, H. Zhang, Z. Xia, T. Xie, W. Li, D. Zhong, H. Zhu and M. Zhou, Biomaterials, 2019, 226, 119538 CrossRef PubMed.
- L. Maggiorella, G. Barouch, C. Devaux, A. Pottier, E. Deutsch, J. Bourhis, E. Borghi and L. Levy, Future Oncol., 2012, 8, 1167–1181 CrossRef CAS PubMed.
-
R. K. Roeder, T. E. Curtis, P. D. Nallathamby, L. E. Irimata, T. L. McGinnity, L. E. Cole, T. Vargo-Gogola and K. D. Cowden Dahl, in Society of Photo-Optical Instrumentation Engineers (SPIE) Conference Series, 2017, vol. 10132, p. 101320X.
- F. Ostadhossein, S. K. Misra, I. Tripathi, V. Kravchuk, G. Vulugundam, D. LoBato, L. E. Selmic and D. Pan, Biomaterials, 2018, 181, 252–267 CrossRef CAS PubMed.
- F. Ostadhossein, I. Tripathi, L. Benig, D. LoBato, M. Moghiseh, C. Lowe, A. Raja, A. Butler, R. Panta, M. Anjomrouz, A. Chernoglazov and D. Pan, Adv. Funct. Mater., 2020, 30, 2070025 CrossRef.
- C. Liu, T. J. Hajagos, D. Kishpaugh, Y. Jin, W. Hu, Q. Chen and Q. Pei, Adv. Funct. Mater., 2015, 25, 4607–4616 CrossRef CAS.
- D. P. Cormode, S. Si-Mohamed, D. Bar-Ness, M. Sigovan, P. C. Naha, J. Balegamire, F. Lavenne, P. Coulon, E. Roessl, M. Bartels, M. Rokni, I. Blevis, L. Boussel and P. Douek, Sci. Rep., 2017, 7, 4784 CrossRef PubMed.
- N. Halttunen, F. Lerouge, F. Chaput, M. Vandamme, S. Karpati, S. Si-Mohamed, M. Sigovan, L. Boussel, E. Chereul, P. Douek and S. Parola, Sci. Rep., 2019, 9, 12090 CrossRef PubMed.
- I. Riederer, D. Bar-Ness, M. A. Kimm, S. Si-Mohamed, P. B. Noël, E. J. Rummeny, P. Douek and D. Pfeiffer, Sci. Rep., 2019, 9, 5268 CrossRef PubMed.
- S. Si-Mohamed, D. Bar-Ness, M. Sigovan, V. R. Tatard-Leitman, D. P. Cormode, P. C. Naha, P. Coulon, L. Rascle, E. Roessl, M. Rokni, A. Altman, Y. Yagil, L. Boussel and P. Douek, Eur. Radiol. Exp., 2018, 2, 34 CrossRef PubMed.
- S. Si-Mohamed, D. P. Cormode, D. Bar-Ness, M. Sigovan, P. C. Naha, J.-B. Langlois, L. Chalabreysse, P. Coulon, I. Blevis, E. Roessl, K. Erhard, L. Boussel and P. Douek, Nanoscale, 2017, 9, 18246–18257 RSC.
- S. Si-Mohamed, V. r Tatard-Leitman, A. Laugerette, M. Sigovan, D. Pfeiffer, E. J. Rummeny, P. Coulon, Y. Yagil, P. Douek, L. Boussel and P. B. Noel, Sci. Rep., 2019, 9, 8458 CrossRef PubMed.
- S. Si-Mohamed, A. Thivolet, P. E. Bonnot, D. Bar-Ness, V. Képénékian, D. P. Cormode, P. Douek and P. Rousset, Invest. Radiol., 2018, 53(10), 629–639 CrossRef PubMed.
- S. A. Si-Mohamed, J. Miailhes, P.-A. Rodesch, S. Boccalini, H. Lacombe, V. R. Leitman, V. Cottin, L. Boussel and P. Douek, J. Clin. Med., 2021, 10, 5757 CrossRef PubMed.
- S. A. Si-Mohamed, M. Sigovan, J. C. Hsu, V. Tatard-Leitman, L. Chalabreysse, P. C. Naha, T. Garrivier, R. Dessouky, M. Carnaru, L. Boussel, D. P. Cormode and P. C. Douek, Radiology, 2021, 300(1), 98–107 CrossRef PubMed.
- A. S.-M. Salim, B. Sara, L. Hugo, D. Adja, V. Mohammad, R. Pierre-Antoine, D. Riham, V. Marjorie, T.-L. Valérie, B. Thomas, C. Philippe, Y. Yoad, L. Elias, E. Klaus, R. Benjamin, B. Eric, R. Gilles, F. Gerard, B. Cyrille, B. Loic, G. Joel and C. D. Philippe, Radiology, 2022, 303, 303–313 CrossRef PubMed.
- N. R. van der Werf, P. A. Rodesch, S. Si-Mohamed, R. W. van Hamersvelt, M. J. W. Greuter, T. Leiner, L. Boussel, M. J. Willemink and P. Douek, Eur. Radiol., 2022, 32(5), 3447–3457 CrossRef CAS PubMed.
- N. R. van der Werf, S. Si-Mohamed, P. A. Rodesch, R. W. van Hamersvelt, M. J. W. Greuter, S. Boccalini, J. Greffier, T. Leiner, L. Boussel, M. J. Willemink and P. Douek, Eur. Radiol., 2022, 32(1), 152–162 CrossRef CAS PubMed.
- M. Sigovan, S. Si-Mohamed, D. Bar-Ness, J. Mitchell, J.-B. Langlois, P. Coulon, E. Roessl, I. Blevis, M. Rokni, G. Rioufol, P. Douek and L. Boussel, Sci. Rep., 2019, 9, 19850 CrossRef CAS PubMed.
- S. Boccalini, S. A. Si-Mohamed, H. Lacombe, A. Diaw, M. Varasteh, P. A. Rodesch, M. Villien, M. Sigovan, R. Dessouky, P. Coulon, Y. Yagil, E. Lahoud, K. Erhard, G. Rioufol, G. Finet, E. Bonnefoy-Cudraz, C. Bergerot, L. Boussel and P. C. Douek, Invest. Radiol., 2022, 57(4), 212–221 CrossRef CAS PubMed.
- Y. Sebti, T. Chauveau, M. Chalal, Y. Lalatonne, C. Lefebvre and L. Motte, Inorg. Chem., 2022, 61, 6508–6518 CrossRef CAS PubMed.
- M. Juenet, R. Aid-Launais, B. Li, A. Berger, J. Aerts, V. Ollivier, A. Nicoletti, D. Letourneur and C. Chauvierre, Biomaterials, 2018, 156, 204–216 CrossRef CAS PubMed.
- B. Li, M. Juenet, R. Aid-Launais, M. Maire, V. Ollivier, D. Letourneur and C. Chauvierre, Adv. Healthcare Mater., 2017, 6(4), 10 Search PubMed.
- E. Roessl and R. Proksa, Phys. Med. Biol., 2007, 52, 4679–4696 CrossRef CAS PubMed.
- S. Si-Mohamed, S. Boccalini, P.-A. Rodesch, R. Dessouky, E. Lahoud, T. Broussaud, M. Sigovan, D. Gamondes, P. Coulon, Y. Yagil, L. C. Boussel and P. Douek, Diagn. Interventional Imaging, 2021, 102, 305–312 CrossRef PubMed.
- F. Geinguenaud, I. s Souissi, R. m Fagard, Y. Lalatonne and L. Motte, J. Phys. Chem. B, 2014, 118, 1535–1544 CrossRef CAS PubMed.
- H. Nguyen, E. Tinet, T. Chauveau, F. Geinguenaud, Y. Lalatonne, A. Michel, R. Aid-Launais, C. Journe, C. Lefèbvre and T. Simon-Yarza, Molecules, 2019, 24, 962 CrossRef PubMed.
- L. Deblock, E. Goossens, R. Pokratath, K. De Buysser and J. De Roo, JACS Au, 2022, 2, 711–722 CrossRef CAS PubMed.
- N. Marinval, P. Saboural, O. Haddad, M. Maire, K. Bassand, F. Geinguenaud, N. Djaker, K. Ben Akrout, M. Lamy de la Chapelle, R. Robert, O. Oudar, E. Guyot, C. Laguillier-Morizot, A. Sutton, C. Chauvierre, F. Chaubet, N. Charnaux and H. Hlawaty, Mar. Drugs, 2016, 14, 185 CrossRef PubMed.
- B. Zhou, H. Shi, X. D. Zhang, Q. Su and Z. Y. Jiang, J. Phys. D: Appl. Phys., 2014, 47, 115502 CrossRef.
- A. Situm, M. A. Rahman, S. Goldberg and H. A. Al-Abadleh, Environ. Sci.: Nano, 2016, 3, 910–926 RSC.
- S. Papernov, M. D. Brunsman, J. B. Oliver, B. N. Hoffman, A. A. Kozlov, S. G. Demos, A. Shvydky, F. H. M. Cavalcante, L. Yang, C. S. Menoni, B. Roshanzadeh, S. T. P. Boyd, L. A. Emmert and W. Rudolph, Opt. Express, 2018, 26, 17608–17623 CrossRef CAS PubMed.
- M. Kong, B. Li, C. Guo, P. Zeng, M. Wei and W. He, Coatings, 2019, 9, 307 CrossRef CAS.
- M. Mahdavi, F. Namvar, M. B. Ahmad and R. Mohamad, Molecules, 2013, 18, 5954–5964 CrossRef PubMed.
- Y. Liu, Q. Lan, S. Sun and Q. Yang, RSC Adv., 2022, 12, 2928–2937 RSC.
- A. A. Rastorguev, V. I. Belyi, T. P. Smirnova, L. V. Yakovkina, M. V. Zamoryanskaya, V. A. Gritsenko and H. Wong, Phys. Rev. B: Condens. Matter Mater. Phys., 2007, 76, 235315 CrossRef.
- A. Y. Maslov and O. V. Proshina, Semiconductors, 2005, 39, 1076–1081 CrossRef CAS.
- S. A. Eliziário, L. S. Cavalcante, J. C. Sczancoski, P. S. Pizani, J. A. Varela, J. W. M. Espinosa and E. Longo, Nanoscale Res. Lett., 2009, 4, 1371 CrossRef PubMed.
- B. Choudhury and A. Choudhury, Phys. E, 2014, 56, 364–371 CrossRef CAS.
- A. R. Gheisi, C. Neygandhi, A. K. Sternig, E. Carrasco, H. Marbach, D. Thomele and O. Diwald, Phys. Chem. Chem. Phys., 2014, 16, 23922–23929 RSC.
- H.-M. Xiong, J. Mater. Chem., 2010, 20, 4251–4262 RSC.
- G. E. Brown, V. E. Henrich, W. H. Casey, D. L. Clark, C. Eggleston, A. Felmy, D. W. Goodman, M. Grätzel, G. Maciel, M. I. McCarthy, K. H. Nealson, D. A. Sverjensky, M. F. Toney and J. M. Zachara, Chem. Rev., 1999, 99, 77–174 CrossRef CAS PubMed.
- J.-L. Giraux, S. Matou, A. E. Bros, J. Tapon-Bretaudière, D. Letourneur and A.-M. Fischer, Eur. J. Cell Biol., 1998, 77, 352–359 CrossRef CAS PubMed.
Footnote |
† Electronic supplementary information (ESI) available: Representative TXRF spectrum of the HfO2 NP sample and the corresponding calibration curves of HfO2@fucoidan NPs and HfO2@citrate NPs; FTIR spectra of HfO2@fucoidan NPs at the saturated ratio R = 14 before and after ultrafiltration; TGA curves; FTIR and Raman spectra of HfO2 NPs, citrate and HfO2@citrate NPs; stability versus pH, in glucose 5%, NaCl 0.9%, DMEM and DMEM+ 10% FBS at various times, and the attenuation rate measured with a SPCCT system for the commercial gadolinium based molecular agent: DOTAREM®. See DOI: https://doi.org/10.1039/d2ma01026g |
|
This journal is © The Royal Society of Chemistry 2023 |