DOI:
10.1039/D2FO03263E
(Paper)
Food Funct., 2023,
14, 354-368
Bifidobacterium longum subsp. infantis FJSYZ1M3 ameliorates DSS-induced colitis by maintaining the intestinal barrier, regulating inflammatory cytokines, and modifying gut microbiota
Received
27th October 2022
, Accepted 7th December 2022
First published on 8th December 2022
Abstract
B. longum subsp. infantis is a subspecies of Bifidobacterium longum, and very few strains are shown to have immunomodulatory effects. In the present study, the improvement of dextran sulphate sodium (DSS)-induced colitis by four B. longum subsp. infantis strains was compared. The results showed that B. longum subsp. infantis FJSYZ1M3 could significantly decrease disease activity index (DAI), inhibit weight loss and colon shortening, and attenuate colon tissue damage in DSS-induced colitis mice. And B. longum subsp. infantis FJSYZ1M3 intervention improved the integrity of intestinal tight junctions, relieved mucus layer damage and inhibited epithelial cell apoptosis, thereby maintaining the intestinal barrier. Additionally, B. longum subsp. infantis FJSYZ1M3 significantly affected the levels of inflammatory cytokines IL-6, IL-1β, and IL-10 in the colon, thus relieving inflammation in colitis mice. Furthermore, B. longum subsp. infantis FJSYZ1M3 could ameliorate gut microbiota disturbance caused by DSS exposure and increase the level of butyric acid in cecal contents. In general, these findings suggested that B. longum subsp. infantis FJSYZ1M3 alleviated DSS-induced colitis by maintaining the intestinal barrier, regulating inflammatory cytokines, and modifying the gut microbiota.
Introduction
Ulcerative colitis (UC) is a type of inflammatory bowel disease, and the exact pathogenesis of UC is still unclear and might include mucosal immune dysregulation, genetic susceptibility, gut microbiota imbalance, and environmental factors.1 The current conventional treatment therapies are aminosalicylates, corticosteroids, and immunosuppressants, however, these medicines have problems such as side effects and relapse.2,3 Many previous studies have found that probiotics were able to maintain the intestinal mucosal barrier, improve immune system function, and rebalance the gut microbiota. And their important role in alleviating UC has been widely reported.4–8
B. longum subsp. infantis is a subspecies of B. longum, which is widely isolated from the intestine of breastfed infants.9 There are significant differences between B. longum subsp. longum and B. longum subsp. infantis in physiological characteristics (for instance, carbohydrate utilization) and genetic information.10 However, most of the commercial B. longum strains belong to B. longum subsp. longum currently, and there are relatively few studies on the health-associated benefits of B. longum subsp. infantis. A number of B. longum subsp. longum strains were reported to improve UC in animal models and/or clinical trials, such as B. longum subsp. longum BB536, 35624™, YS108R and CCM7952.11–14 By contrast, at present, B. longum subsp. infantis ATCC15697 was the only strain in B. longum subsp. infantis with the benefit to alleviate DSS-induced colitis. The intervention with this strain negatively regulated intestinal epithelial co-stimulatory molecules (including CD80 and CD40) and reduced the responses of IL-17A production by CD4+ T cells induced by intestinal epithelial cells.15 The effects of other B. longum subsp. infantis strains on UC need further investigation.
In our previous genomic analysis of B. longum subsp. infantis, it was found that there were significant differences in genetics among different strains,10 which indicated that their physiological functions might differ. Therefore, in the present study, four strains with relatively distant genetic relationship were selected to compare their effects on DSS-induced colitis. And the improvement effects were further analyzed in terms of intestinal barrier, inflammatory cytokines, and gut microbiota.
Materials and methods
Activation and cultivation of strains
B. longum subsp. infantis FJND2M2, FJSYZ1M3 (CCFM1210), FBJCP1M11, and FGZ17I1M1 were isolated and identified in fecal samples from healthy infants in our previous work,10 and deposited at Culture Collection of Food Microorganisms (CCFM), Jiangnan University. The strains were cultured anaerobically at 37 °C for 24 h in de Man, Rogosa, and Sharpe (MRS) broth medium with 0.05% L-cysteine hydrochloride. After sub-culturing three times, the cells were collected by centrifugation (8000g, 15 min), washed three times with sterile phosphate-buffered saline (PBS, pH = 7.4), re-suspended in 30% (v/v) glycerol solution, and then stored at −80 °C before use. The glycerol solution was removed and the cells were diluted to 1 × 1010 CFU mL−1 with 0.9% sodium chloride solution before gavage.
Design of animal experiment
The animal experiment protocol was approved by the Experimental Animal Management and Animal Welfare Ethics Committee of Jiangnan University (JN. No. 20210530c1280708[144]). All the animals were kept in the Experimental Animal Center of Jiangnan University in a standard environment (constant temperature of 20–26 °C, humidity of 40%–70%, and 12 h light/dark cycle). Six-week-old male C57BL/6N mice (Beijing Vital River Laboratory Animal Technology Co., Ltd, Beijing, China) were randomly divided into six groups (n = 8), including control group, DSS group, B. longum subsp. infantis FJND2M2 group, B. longum subsp. infantis FJSYZ1M3 group, B. longum subsp. infantis FBJCP1M11 group, and B. longum subsp. infantis FGZ17I1M1 group. During the trial, 0.2 mL of 0.9% sterile sodium chloride solution was provided to the mice in the control group and DSS group, and the mice in B. longum subsp. infantis intervention groups were orally gavaged with an equal volume of B. longum subsp. infantis (1 × 1010 CFU mL−1) for a total of 14 days. On days 1–7, the mice in all groups freely drank sterilized water. And on days 8–14, the mice drank 2.7% (w/v) sterile dextran sulphate sodium (DSS, MP Biomedical, LLC., Santa Ana, CA, USA) solution freely, except for the control group.
Assessment of colitis
During the DSS exposure, the body weight of mice was daily recorded, and the change of the body weight relative to the initial body weight was calculated. The change in disease activity index (DAI) over 7 days was measured in terms of criteria in previous studies.16,17 On the 15th day of the experiment, the mice were sacrificed. And then the colon tissues were photographed, and their length was recorded. The 4% (w/v) paraformaldehyde was used to fix the distal colon. And other tissues were snap-frozen in liquid nitrogen and stored at −80 °C. Embedding, sectioning, and Hematoxylin and Eosin (H&E) staining of distal colon tissue were performed by Wuhan Servicebio Technology Co., Ltd, Wuhan, China. Moreover, the Pannoramic MIDI digital slide scanner (3DHistech Co., Ltd, Budapest, Hungary) was used to scan the stained colon tissue, and the calculation of colon histological scores was based on existing approaches.17,18
Determination of myeloperoxidase
The Pierce™ BCA Protein Assay Kit (Thermo Fisher Scientific, Inc., Waltham, MA, USA) was used to measure the concentration of protein. The determination of myeloperoxidase (MPO) concentration in the colon was performed by commercial ELISA kits (Multisciences Biotech, Co., Ltd, Hangzhou, China).
Assessment of intestinal barrier damage
The concentration of intestinal tight junction proteins, MUC2 and Caspase-3 was detected by the commercial ELISA kits (Nanjing SenBeiJia Biological Technology Co., Ltd, Nanjing, China). Additionally, the immunofluorescence staining for intestinal tight junction proteins, alcian blue staining, periodic acid-Schiff (PAS) staining, and TUNEL fluorescent staining were completed by Wuhan Servicebio Technology. For immunofluorescence staining and TUNEL fluorescent staining, the tissue sections were incubated with antibody or TUNEL reaction solution and counterstained with 4′,6-diamidino-2-phenylindole (DAPI), and photographed with a fluorescence microscope. And the main experimental processes of alcian blue staining and periodic acid-Schiff (PAS) staining included section dewaxing, dye staining, and dehydration sealing.
Measurement of cytokines in the colon
The commercial ELISA kits (R&D Systems, Minneapolis, MN, USA) were used to measure the concentrations of IL-6, IL-1β, TNF-α, IL-10, and IL-17 in the colon. And commercial ELISA kits (Multisciences Biotech) were used to measure the levels of IL-4 and IFN-γ.
Determination of short-chain fatty acids (SCFAs)
The concentrations of acetic acid, propionic acid, and butyric acid in the cecal contents of mice were detected by the GC-MS. Briefly, the cecal contents were acidified with sulfuric acid, extracted with ether and dehydrated, and then analyzed by GC-MS. And the detailed methods followed the previous description.19
Analyses of gut microbiota
Fecal genomic DNA was extracted by the FastDNA Spin Kit (MP Biomedicals, LLC, Irvine, CA) amd the previously described methods were used to amplify the V3–V4 region of 16S rDNA, purify the PCR product, and sequence.20,21 The sequencing data was analyzed by the QIIME2 pipeline. And MicrobiomeAnalyst (https://www.microbiomeanalyst.ca) was used to analyze the diversity of gut microbiota.22 In addition, linear discriminant analysis effect size (LEfSe) analyses were performed by an online tool (https://huttenhower.sph.harvard.edu/galaxy).23 And SPSS26.0 was used to analyze the significant difference in the relative abundance of dominant microorganisms at the genus level.
Statistical analysis
Data analysis and plotting were done by GraphPad Prism8 and SPSS26.0. The one-way ANOVA using Tukey's test was used to analyze the significant difference. And the results were expressed as the mean ± standard error of the mean (mean ± SEM). A significant difference was indicated by a p-value < 0.05.
Results
B. longum subsp. infantis improved colitis symptoms
During the DSS exposure, the stool consistency, hematochezia, and weight loss of the mice were recorded daily. On the fifth day of the free drinking of DSS solution, the weight of the DSS group mice began to decrease, and the weight loss was 13.40% ± 0.85 on the seventh day (Fig. 1A). Compared with the DSS group, B. longum subsp. infantis FJSYZ1M3 intervention significantly alleviated the weight loss of mice (p < 0.05), which was only 6.94% ± 1.39 (Fig. 1A). B. longum subsp. infantis FBJCP1M11 and FGZ17I1M1 could alleviate the weight loss of mice to a certain extent, but without significance. B. longum subsp. infantis FJND2M2-treated mice began to lose body weight from the fourth day of DSS exposure and finally, the weight loss was 18.75% ± 2.75. The DAI of mice in the DSS group reached 9.25 ± 0.53 on the 7th day, while that in B. longum subsp. infantis FJSYZ1M3-treated mice were 6.12 ± 0.77, with a significant decrease of 33.84% (p < 0.05). However, the DAI of mice in B. longum subsp. infantis FJND2M2 group was higher than that in the DSS group (Fig. 1B). Furthermore, DSS exposure resulted in colon swelling and shortening (Fig. 1C). And compared with the DSS group, the colon length of mice in B. longum subsp. infantis FJSYZ1M3 group was significantly longer (p < 0.05, Fig. 1D).
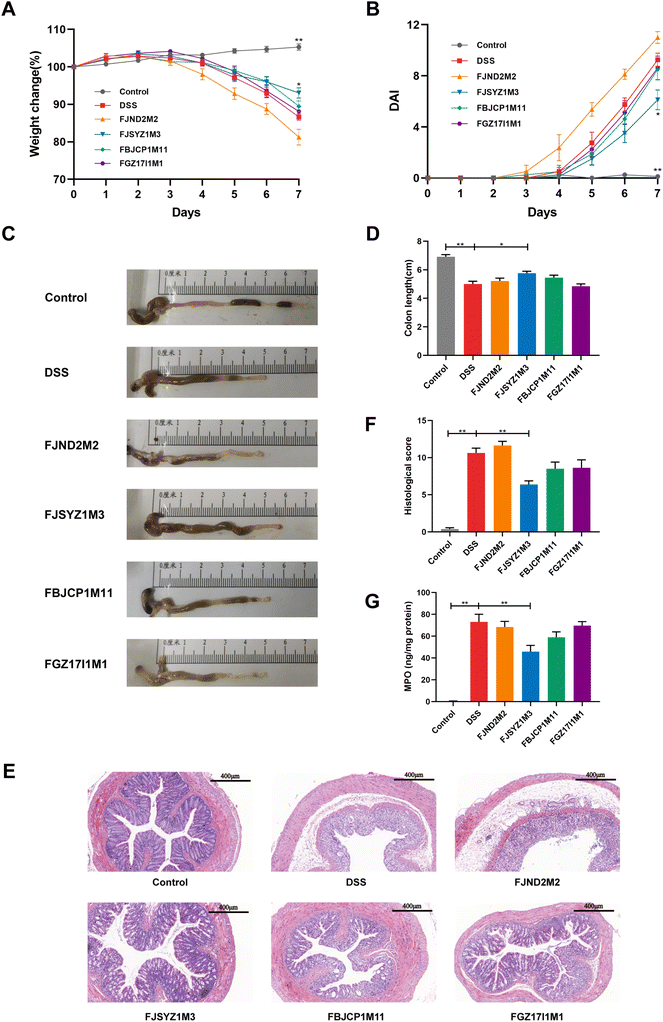 |
| Fig. 1 Improvement of colitis symptoms by B. longum subsp. infantis. (A) Body weight loss, (B) disease activity index, (C) macroscopic pictures of mice colons, (D) colon length, (E) the distal colons were stained with H&E, scale bars, 400 μm. (F) histological score of the colon, (G) myeloperoxidase concentration in the colon. * p < 0.05, ** p < 0.01 vs. DSS. Data show the mean ± SEM (n = 8). | |
H&E staining and histological score were used to determine the damage of colon tissue. DSS exposure led to severe colon tissue damage, such as the disappearance of crypt structure, intestinal epithelium destruction, submucosal edema, and inflammatory cell infiltration (Fig. 1E). Compared with the DSS group, there was more severe inflammation in the colon tissue of B. longum subsp. infantis FJND2M2-treated mice. The intervention of the other three strains could alleviate the tissue damage. And B. longum subsp. infantis FJSYZ1M3 showed a significant improvement effect, only 1/3 of the crypt structure disappeared, the epithelium was intact, and only a few inflammatory cells were infiltrated. Moreover, compared with the DSS group, B. longum subsp. infantis FJSYZ1M3-treated mice had a significantly lower histological score (p < 0.01), and B. longum subsp. infantis FBJCP1M11 and FGZ17I1M1 treatment also reduced the colonic histological score (Fig. 1F).
Additionally, DSS exposure led to a significantly increased MPO in the colon, while B. longum subsp. infantis intervention could reduce the concentration of MPO, with only B. longum subsp. infantis FJSYZ1M3 having a significant effect (p < 0.01, Fig. 1G).
B. longum subsp. infantis maintained the intestinal barrier
B. longum subsp. infantis FJSYZ1M3 intervention significantly increased the concentration of tight junction proteins (Occludin, Claudin-1, and ZO-1) in colon tissue compared with the DSS group (p < 0.05), while B. longum subsp. infantis FJND2M2 had no significant influence on the concentrations of Occludin, Claudin-1, nor ZO-1 (Fig. 2A–C). Compared with the DSS group, mice in B. longum subsp. infantis FBJCP1M11 and FGZ17I1M1 groups only showed significant differences in Claudin-1 (p < 0.05, Fig. 2C). Furthermore, the results of immunofluorescence analyses reflected the distribution of Occludin, Claudin-1, and ZO-1. The expression levels of the three tight junction proteins in the DSS group were significantly reduced compared with those in the control group, and the original structural distribution was destroyed. The tight junction structure damage in B. longum subsp. infantis FJND2M2 group was similar to that in the DSS group and even more serious. And other strains could alleviate the damage of tight junction integrity caused by DSS exposure to a certain extent and B. longum subsp. infantis FJSYZ1M3 treatment showed the most significant positive effect (Fig. 2B).
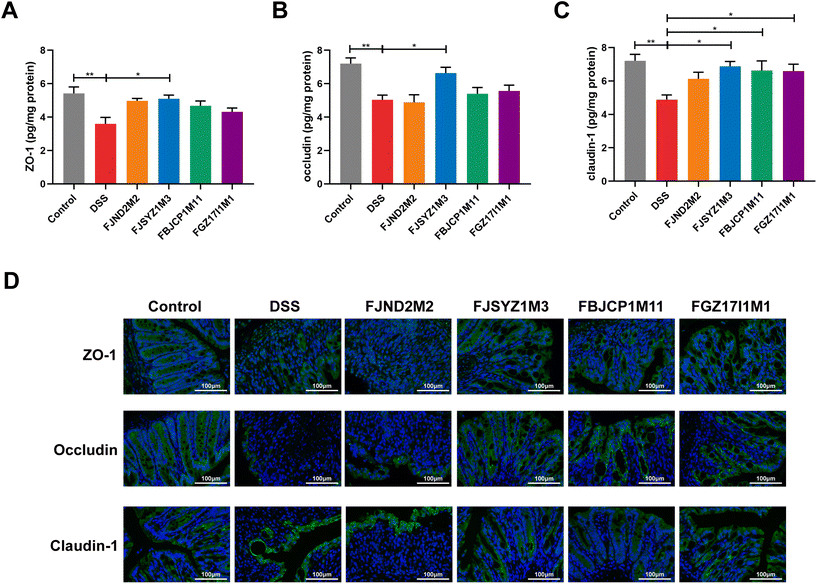 |
| Fig. 2 Improvement of tight junction in the colon by B. longum subsp. infantis. Quantification of tight junction proteins (A) ZO-1, (B) Occludin, (C) Claudin-1, (D) immunofluorescence of tight junction proteins, scale bars, 100 μm. * p < 0.05, ** p < 0.01 vs. DSS. Data show the mean ± SEM (n = 8). | |
The results of alcian blue staining and PAS staining indicated that DSS exposure resulted in significant decreases in the number of goblet cells in crypts and the level of mucin, and serious damage to the mucus layer. The intervention of B. longum subsp. infantis could up-regulate the level of mucin and reduce the mucus layer damage, except B. longum subsp. infantis FJND2M2 (Fig. 3A and B). The protein concentration of MUC2 was further determined, and the results suggested that compared with the DSS group, B. longum subsp. infantis FJSYZ1M3 intervention significantly increased the level of MUC2 (p < 0.05, Fig. 3C).
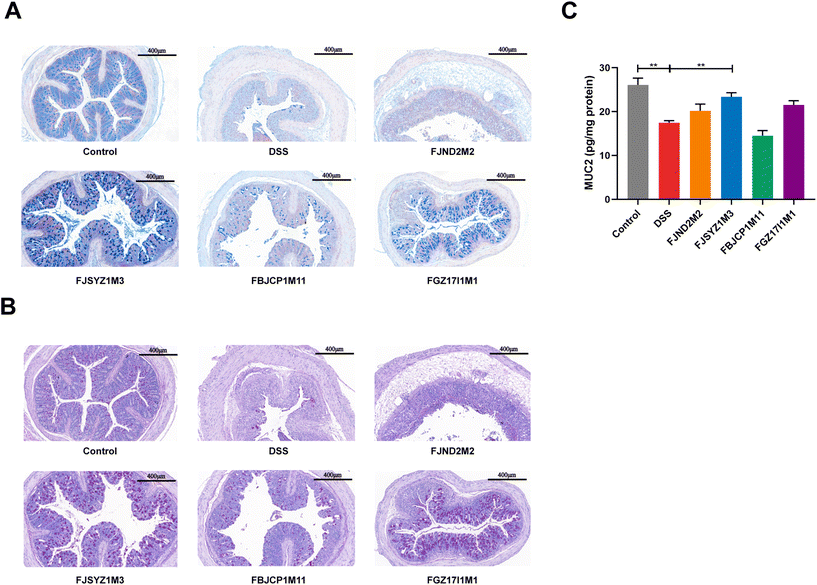 |
| Fig. 3 Effects on colonic mucus layer in mice of B. longum subsp. infantis. (A) Alcian blue staining, scale bars, 400 μm. (B) PAS staining, scale bars, 400 μm, (C) quantification of MUC2. ** p < 0.01 vs. DSS. Data show the mean ± SEM (n = 8). | |
TUNEL staining showed that the DSS challenge could significantly increase the number of apoptotic cells in mice colons, and the colon of B. longum subsp. infantis FBJCP1M11 treated and FJSYZ1M3 treated mice had fewer apoptotic cells (Fig. 4A). The concentration of Caspase3 in B. longum subsp. infantis FJND2M2 treated mice was higher compared with that in the DSS group, while other B. longum subsp. infantis treatments reduced the Caspase3 concentration (Fig. 4B).
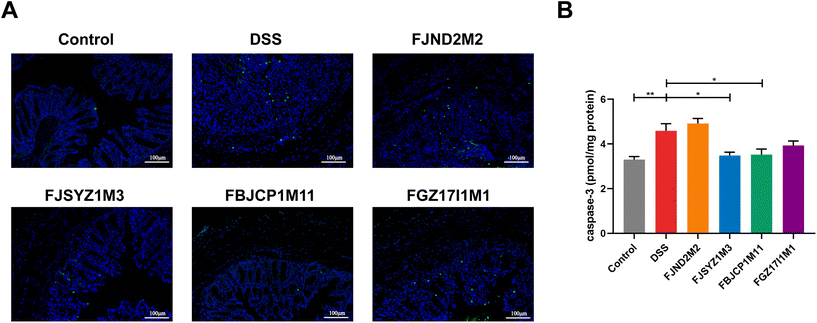 |
| Fig. 4 Effects on apoptosis of colonic epithelial cells in mice in B. longum subsp. infantis. (A) TUNEL analysis of colon, scale bars, 100 μm. (B) Quantification of Caspase3. ** p < 0.01 vs. DSS. Data show the mean ± SEM (n = 8). | |
B. longum subsp. infantis influenced the inflammatory cytokines
The protein concentration of inflammatory cytokines in colon tissues was determined to further reveal the effects of B. longum subsp. infantis on inflammation in colitis mice. And compared with the DSS group, B. longum subsp. infantis FJSYZ1M3 intervention significantly reduced the protein concentration of pro-inflammatory cytokines (IL-1β and IL-6) in mice and significantly up-regulated the level of the anti-inflammatory cytokine (IL-10), while it had no significant effect on IFN-γ, TNF-α, IL-17, nor IL-4 (p < 0.05, Fig. 5). B. longum subsp. infantis FBJCP1M11 and FGZ17I1M1 showed significant effects on the concentration of IL-10 or IL-6, respectively (p < 0.05, Fig. 5A and F), whereas B. longum subsp. infantis FJND2M2 had a little positive effect on the regulation of inflammatory cytokines.
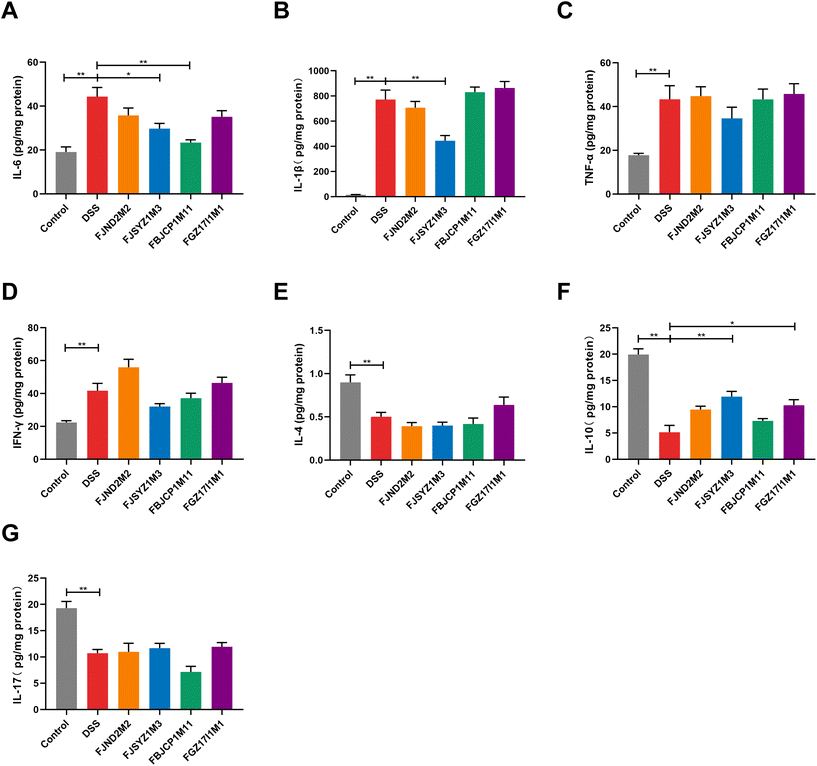 |
| Fig. 5 Effects of B. longum subsp. infantis on inflammatory cytokines in the colon. (A) IL-6, (B) IL-1β, (C) TNF-α, (D) IFN-γ, (E) IL-4, (F) IL-10, (G) IL-17. * p < 0.05, ** p < 0.01 vs. DSS. Data show the mean ± SEM (n = 8). | |
B. longum subsp. infantis regulated gut microbiota
The α-diversity reflects species richness and evenness of gut microbiota in mice, including the Chao1 index, Shannon index, and Simpson index. Chao1 index suggested that B. longum subsp. infantis FJSYZ1M3 treatment enlarged the species richness of gut microbiota in mice significantly (p < 0.05, Fig. 6A), and at the same time, the distribution among species showed more evenness (Fig. 6B and C), therefore, the α-diversity of gut microbiota increased. Moreover, the other strains could increase the α-diversity of gut microbiota to a certain extent, but it was not statistically significant.
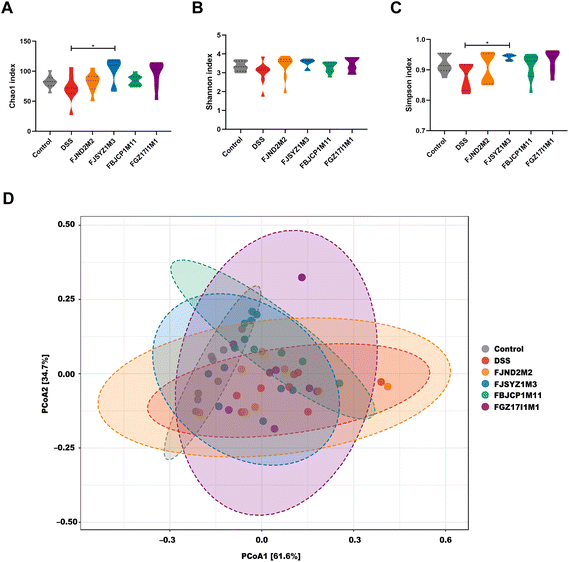 |
| Fig. 6 Effects of B. longum subsp. infantis on gut microbiota. (A) Chao1 index. (B) Shannon index. (C) Simpson index. (D) The principal coordinate analysis of β-diversity at the phylum level was based on weighted UniFrac distance. * p < 0.05, ** p < 0.01 vs. DSS. Data show the mean ± SEM (n = 8). | |
The principal coordinate analysis of gut microbiota based on weighted UniFrac distance revealed that gut microbiota profiles in mice within the same groups tended to cluster together, and DSS exposure led to the changes in gut microbiota profile (r2 = 0.283, p < 0.01, PERMANOVA test; Fig. 6D). The effects of four B. longum subsp. infantis strains on gut microbiota profiles were different. The gut microbiota profiles of B. longum subsp. infantis FJND2M2 group were similar to those in the DSS group, while gut microbiota profile in B. longum subsp. infantis FJSYZ1M3 group was closer to the control group (although there were deviations in individuals).
The phylum-level abundance of the gut microbiota in mice was analyzed. And the results suggested that the gut microbiota in the control group was dominated by Firmicutes and Bacteroidetes, with the abundance reaching 48.39% and 46.74%, respectively. The abundance of Firmicutes and Bacteroidetes decreased, and the abundance of Proteobacteria increased significantly because of the DSS challenge. And B. longum subsp. infantis interventions shifted the relative abundance in a direction more similar to the control group (Fig. 7A).
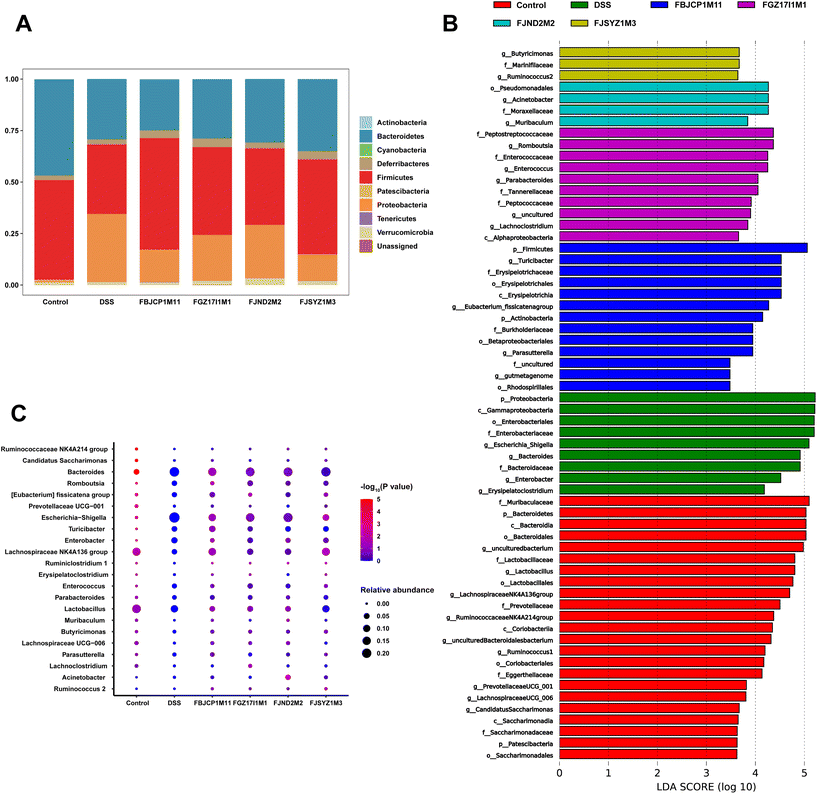 |
| Fig. 7 Effects of B. longum subsp. infantis on phylum-level abundance and dominant microorganisms. (A) Relative abundance of gut microbiota at the phylum level. (B) LDA distribution histogram, with a log LDA score above 3.0. (C) The difference in the relative abundance of gut microbiota at the genus level. | |
LEfSe analysis revealed dominant microbes in the gut microbiota of mice in the six groups (Fig. 7B). The dominant microbes in the DSS group were Escherichia–Shigella and Bacteroides, and Acinetobacter was the dominant microbes in B. longum subsp. infantis FJND2M2 group. And the dominant genus in B. longum subsp. infantis FJSYZ1M3, FBJCP1M11, and FGZ17I1M1 group were Butyricimonas, Turicibacter, and Romboutsia, respectively. And then these dominant microbes were further analyzed at the genus level. The results indicated that, compared with the control group, the relative abundances of Lactobacillus, Lachnospiraceae NK4A136 group, and Ruminococcaceae_NK4A214_group were significantly decreased (p < 0.05). And the relative abundances of Bacteroides, Enterobacter, Escherichia–Shigella, and Turicibacter in the DSS group increased significantly (p < 0.05, Fig. 7C). B. longum subsp. infantis treatment inhibited gut microbiota abundance changes in colitis mice to a certain extent. For example, compared with the DSS group, B. longum subsp. infantis FBJCP1M11 treatment decreased the relative abundance of Bacteroides (p < 0.05), and the relative abundance of Escherichia–Shigella in B. longum subsp. infantis FJSYZ1M3 group significantly decreased (p < 0.05). Furthermore, the relative abundance of Lachnospiraceae NK4A136 group in B. longum subsp. infantis FJSYZ1M3 group significantly increased (p < 0.05).
B. longum subsp. infantis regulated the concentration of SCFAs
The concentration of acetic acid, propionic acid, and butyric acid in cecal contents was measured (Fig. 8A–C). DSS exposure led to a decrease in cecal propionic acid and butyric acid (Fig. 8B and C). Compared with the DSS group, B. longum subsp. infantis FJSYZ1M3 and FBJCP1M11 treatments significantly increased the concentration of butyric acid in colitis mice (p < 0.05, Fig. 8C).
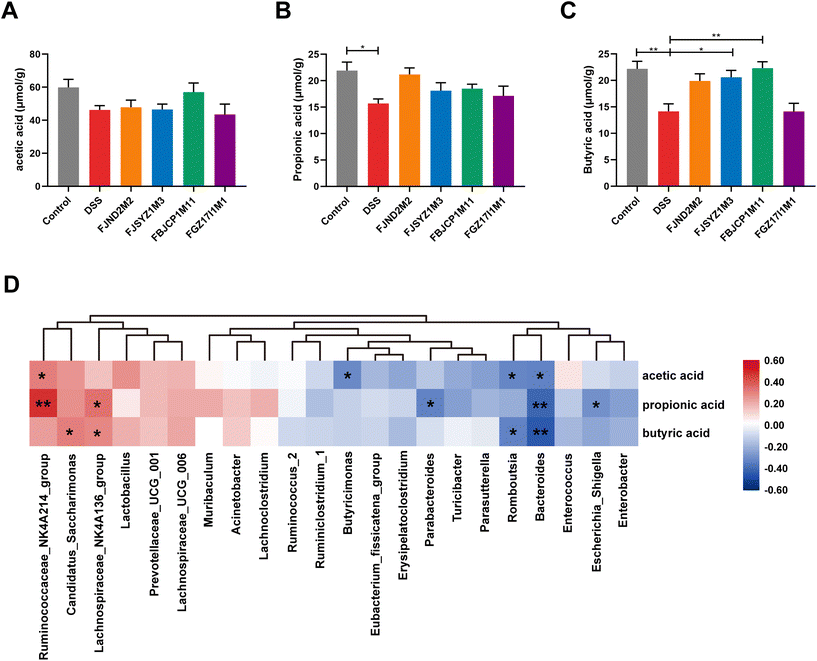 |
| Fig. 8 Effects of B. longum subsp. infantis on the concentrations of SCFAs. (A) Acetic acid. (B) Propionic acid. (C) Butyric acid. (D) Correlation between the concentrations of SCFAs and the dominant genus in gut microbiota. * p < 0.05, ** p < 0.01 vs. DSS. Data show the mean ± SEM (n = 8). | |
Pearson correlation analysis was conducted on the dominant genus of gut microbiota and the concentration of three SCFAs. The results indicated that the concentration of three SCFAs was significantly negatively correlated with the relative abundance of Bacteroides (p < 0.05), while the concentration of acetic acid and propionic acid was significantly positively correlated with the relative abundance of Ruminococcaceae_NK4A214_group (p < 0.05, Fig. 8D). The concentration of acetic acid positively correlated with the relative abundance of Lactobacillus, but not significantly (p = 0.06).
Correlation analyses of colitis indexes
To further illustrate the role of B. longum subsp. infantis in alleviating colitis, Pearson correlation of colitis symptoms, inflammatory cytokines, intestinal barrier, and dominant genus of gut microbiota was performed. Body weight, DAI, colon length, histological score, and the level of MPO were important indexes to reflect the severity of colitis symptoms. The results of correlation analyses showed that inflammatory cytokines were significantly associated with the improvement of colitis symptoms (p < 0.05 and |r2| > 0.6) (Fig. 9). Additionally, the concentration of tight junction protein (Occludin) significantly negatively correlated with histological score and the level of MPO, and the level of MUC2 significantly negatively correlated with the level of MPO (p < 0.05 and |r2| > 0.6). Interestingly, the relative abundance of Ruminococcaceae_NK4A214_group was significantly correlated with five indexes reflecting the severity of colitis (p < 0.05 and |r2| > 0.6) (Fig. 9).
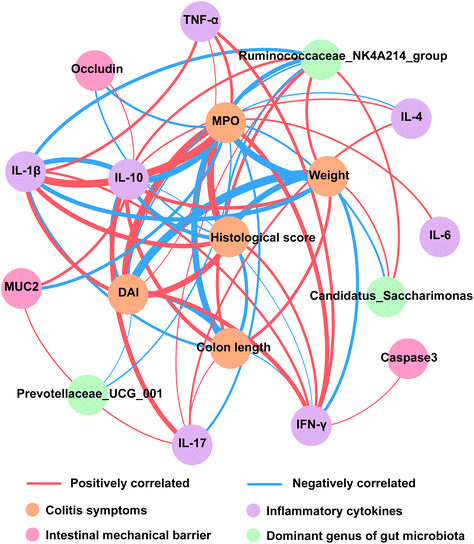 |
| Fig. 9 Correlation of colitis symptoms, inflammatory cytokines, intestinal barrier, and dominant gut microbiota. Nodes represented indicators with significant correlation (p < 0.05 and |r2| > 0.6), and other indicators were not shown. The edge thickness represented the weight. | |
Discussion
Body weight changes, DAI index, colon length, and colon histological score are important criteria for evaluating the severity of colitis. In addition, MPO is a peroxidase that abundantly expresses and secretes by neutrophils, and its changes reflect the neutrophil infiltration in the colon and the degree of tissue damage.24 In the present study, the results of those indicators suggested that the immunomodulatory effects of B. longum subsp. infantis were strain-dependant. And B. longum subsp. infantis FJSYZ1M3 treatment significantly improved the symptoms of DSS-induced colitis and B. longum subsp. infantis FBJCP1M11 and FGZ17I1M1 only had a little improvement in colitis, while B. longum subsp. infantis FJND2M2 aggravated the symptoms. In the previous study of B. longum subsp. infantis ATCC15697 alleviating colitis, DSS challenge resulted in significant destruction of epithelial cells with acinar loss and mucosal hypertrophy, and the strain had obvious effects on alleviating weight loss, improving DAI, maintaining colon length, and reducing tissue damage in mice.15 The previous studies on B. longum and other Bifidobacterium alleviating DSS-induced colitis suggested that bacterial interventions could reduce the histological score and alleviate the damage to colon tissue.25–27 Additionally, B. longum subsp. longum YS108R significantly alleviated the increase in MPO activity caused by DSS exposure.13 And B. longum subsp. longum HY8004, LC67, and CH57 were able to inhibit MPO activity, thereby alleviating 2,4,6-trinitrobenzene sulfonic acid (TNBS)-induced colitis in mice.28–30 These were consistent with the present study.
Tight junctions are important proteins that connect the adjacent intestinal epithelial cells to form an epithelial barrier. The proteins and molecules that constitute tight junctions mainly include three transmembrane proteins, namely Occludin, Claudins, and junctional adhesion molecules (JAM), and closed small circle proteins (ZOs) that connect transmembrane proteins and the cytoskeleton.31 Hence, the effects of B. longum subsp. infantis on intestinal tight junction integrity was assessed by analyzing the protein concentration and distribution of three tight junction proteins. The results revealed that B. longum subsp. infantis FJSYZ1M3 could significantly up-regulate the level of intestinal tight junction proteins, and improve the intestinal tight junction integrity. B. longum FGDLZ8M1 could significantly restore the relative mRNA expressions of Occludin, ZO-1, and Claudin-1 in the gastrointestinal tract of colitis mice in the previous report.26 And B. longum DD98 could increase the expression of tight junction protein in DSS-induced colitis, including ZO-1 and Occludin.32 Moreover, the ameliorating effects of other Bifidobacterium strains on colitis showed to be related to their restoration of tight junction integrity, including B. breve, B. pseudocatenulatum, and B. bifidum.21,27,33,34
The mucus layer covering the surface of the intestinal epithelium is one of the key components of intestinal barrier integrity, and MUC2 is the main component of the intestinal mucus layer. Mucus layer damage is one of the hallmarks of UC, including decreased MUC2 secretion, thinning of the mucus layer, and altered mucus composition.35,36B. longum subsp. infantis FJSYZ1M3 significantly increased the concentration of MUC2 and alleviated the damage to the mucus layer caused by DSS exposure in this study. The colitis mice which were given B. longum subsp. longum YS108R maintained the MUC2 gene expression at a level similar to the control group in the previous study.13 In addition, in the study of Bifidobacterium alleviating colitis, it was also found that B. animalis subsp. lactis A6 was able to improve the mucus layer destruction and decrease the number of crypt goblet cells caused by DSS exposure.25
Intestinal epithelial cell apoptosis is one of the reasons for intestinal barrier destruction.37 Caspase3 is a key enzyme in the process of apoptosis and belongs to the effector caspase.38 The intervention of B. longum subsp. infantis generally inhibited epithelial cell apoptosis in colitis mice in the present study. In a previous report, it was found that two B. breve strains could significantly reduce the activity of Caspase3 in colitis mice.21 Furthermore, B. pseudocatenulatum was shown to reduce Caspase3 activity and inhibit epithelial cell apoptosis.33 Therefore, B. longum subsp. infantis FJSYZ1M3 could maintain the intestinal barrier by improving intestinal tight junction integrity, inhibiting epithelial cell apoptosis, and relieving mucus layer damage.
The intestinal barrier disruption permitted more pathogenic microbiota to cross the barrier and stimulated immune cells to produce various inflammatory cytokines, thereby contributing to the intestinal inflammatory response in patients with colitis.1,39,40 Therefore, inflammatory cytokines in the colon after B. longum subsp. infantis interventions were determined. And the results revealed that B. longum subsp. infantis FJSYZ1M3 significantly influenced the protein concentration of inflammatory cytokines IL-6, IL-1β, and IL-10. And B. longum subsp. longum could also regulate the concentration of inflammatory cytokines in colitis mice according to previous studies. For example, B. longum CH57 significantly decreased the concentration of IL-17, TNF-α, and IL-1β, while up-regulated the expression of IL-10, although colitis was induced by TNBS.29 The results in other studies of Bifidobacterium were similar, such as B. adolescentis IF1-03, which could significantly increase the levels of IL-10 in the colon while reducing the expression of TNF-α, IL-6, and IL-1β.41 Additionally, the results of correlation analysis indicated that regulating the level of inflammatory cytokines was a vital reason for B. longum subsp. infantis to alleviate colitis.
The gut microbiota in patients with UC was characterized by reducing microbial diversity, particularly Proteobacteria (especially Enterobacteriaceae) and Firmicutes.42 DSS exposure could cause gut microbiota disturbance in mice, hence, the influence of B. longum subsp. infantis intervention on gut microbiota was further evaluated. The results of gut microbiota diversity analysis suggested that B. longum subsp. infantis FJSYZ1M3 intervention alleviated the disturbance of gut microbiota caused by DSS exposure in the present study. It has been shown that supplementation with B. longum Bif10 could improve the Shannon index of gut microbiota in mice with colitis and make the profile of gut microbiota develop in a direction close to that of the control group.43
The result of the present study revealed that B. longum subsp. infantis FJSYZ1M3 treatment could improve the abundance of gut microbiota closer to that in the control group. It has been found that the relative abundance of Firmicutes decreased because of DSS exposure,17,21 which was consistent with this study, whereas the relative abundance changes of Bacteroidetes varied.13,20,21 In UC patients, a significantly higher relative abundance of Proteobacteria was shown in the severe phase compared to the moderate and mild phases of inflammation.44 In the study of B. bifidum ATCC29521 improving colitis, there was an increase in the relative abundance of Firmicutes and a decrease in the relative abundance of Proteobacteria after the bifidobacterial intervention.45 And B. breve CCFM683 intervention could also inhibit the relative abundance decrease of Bacteroidetes and the relative abundance increase of Proteobacteria after the DSS challenge,20 which was consistent with the present study.
Previously LEfSe analysis demonstrated that mice with colitis exhibited increased relative abundances of Escherichia–Shigella, suggesting that the number of these pathogens belonging to Proteobacteria was significantly suppressed in mice supplied with B. bifidum supplements,45 which showed a result consistent with this study. Escherichia–Shigella had been implicated in the development of gut microbiota imbalance and inflammation as harmful bacteria in multiple studies.46–49 In addition, in the study of B. breve CCFM683 alleviating colitis, it was found that B. breve intervention could inhibit the increased abundance of Turicibacter induced by DSS exposure,20 and the intervention of other B. breve was also shown to reduce the relative abundance of Bacteroides.21 In another similar study, it was found that B. animalis subsp. lactis XLTG11 increased the relative abundance of Lachnospiraceae NK4A136 group in colitis mice to a certain extent, but the change was not significant.50 In a comprehensive analysis of target bacteria during the progress of colitis, Turicibacter was suggested to be positively correlated with inflammation status, while an increased relative abundance of Lachnospiraceae was able to reduce inflammation.51 Furthermore, the results of correlation analysis showed that Ruminococcaceae_NK4A214_group had a positive effect on the improvement of colitis. Therefore, restoring gut microbiota balance was another important reason for B. longum subsp. infantis to relieve colitis.
SCFAs played a vital role in IBD pathogenesis, and the levels of acetic acid, propionic acid, and butyric acid were reduced in stool samples from patients with UC.52 It has shown that butyric acid could regulate various cytokines production and could also promote epithelial barrier function.53–55 In this study, B. longum subsp. infantis FJSYZ1M3 and FBJCP1M11 significantly increased butyric acid in colitis mice, which might also affect the expression of inflammatory cytokines and tight junctions at the same time. It was found that B. longum FGDLZ8M1 could significantly increase acetic acid and total SCFAs in colitis mice in the previous study.26 In addition, the Pearson correlation analysis of gut microbiota and SCFAs in a DSS-induced colitis model showed that the levels of acetic acid and propionic acid were significantly positively correlated with the relative abundance of Lactobacillus,56 which was consistent with the results of the present study. In another Pearson correlation analysis, it was also found that the levels of SCFAs negatively correlated with the abundance of Bacteroides or Escherichia_Shigella.57 Moreover, the analysis of fecal samples from healthy adults showed that the production of SCFAs positively correlated with the relative abundance of Ruminocochaceae, while the relative abundance of Bacteroides negatively correlated with the level of SCFAs.58 These results indicated that changes in the relative abundance of gut microbiota could influence the levels of SCFAs, and B. longum subsp. infantis could relieve colitis by up-regulating the levels of SCFAs.
In summary, B. longum subsp. infantis FJSYZ1M3 could significantly alleviate the symptoms of colitis, and the patterns of alleviating colitis mainly included reducing the damage of the intestinal barrier, regulating the inflammatory cytokines, up-regulating butyric acid, and relieving gut microbiota disturbance.
Author contributions
Mingjie Li: investigation, formal analysis, visualization, and writing-original draft preparation; Jiuhong Ding: conceptualization and methodology, writing-review, and editing; Catherine Stanton and R. Paul Ross: conceptualization, writing-review and editing; Jianxin Zhao: resources and validation; Bo Yang: conceptualization, supervision, writing-original draft preparation, writing-review, and editing; Wei Chen: funding acquisition and supervision.
Conflicts of interest
All authors declared no conflict of interest.
Acknowledgements
This research was supported by the National Natural Science Foundation of China (No. 32021005, 31820103010, 31771953), 111 Project (BP0719028), and the Collaborative Innovation Center of Food Safety and Quality Control in Jiangsu Province.
References
- T. Kobayashi, B. Siegmund, C. Le Berre, S. C. Wei, M. Ferrante, B. Shen, C. N. Bernstein, S. Danese, L. Peyrin-Biroulet and T. Hibi, Ulcerative colitis, Nat. Rev. Dis. Primers, 2020, 6, 74 CrossRef PubMed.
- R. Ungaro, S. Mehandru, P. B. Allen, L. Peyrin-Biroulet and J. F. Colombel, Ulcerative colitis, Lancet, 2017, 389, 1756–1770 CrossRef PubMed.
- K. Hazel and A. O'Connor, Emerging treatments for inflammatory bowel disease, Ther. Adv. Chronic Dis., 2020, 11, 2040622319899297 CAS.
- Z. H. Shen, C. X. Zhu, Y. S. Quan, Z. Y. Yang, S. Wu, W. W. Luo, B. Tan and X. Y. Wang, Relationship between intestinal microbiota and ulcerative colitis: Mechanisms and clinical application of probiotics and fecal microbiota transplantation, World J. Gastroenterol., 2018, 24, 5–14 CrossRef CAS PubMed.
- Y. J. Jang, W.-K. Kim, D. H. Han, K. Lee and G. Ko,
Lactobacillus fermentum species ameliorate dextran sulfate sodium-induced colitis by regulating the immune response and altering gut microbiota, Gut Microbes, 2019, 10, 696–711 CrossRef CAS PubMed.
- C. Hidalgo-Cantabrana, F. Algieri, A. Rodriguez-Nogales, T. Vezza, P. Martinez-Camblor, A. Margolles, P. Ruas-Madiedo and J. Galvez, Effect of a ropy exopolysaccharide-producing Bifidobacterium animalis subsp lactis strain orally administered on DSS-induced colitis mice model, Front. Microbiol., 2016, 7, 782 Search PubMed.
- Y. Derwa, D. J. Gracie, P. J. Hamlin and A. C. Ford, Systematic review with meta-analysis: the efficacy of probiotics in inflammatory bowel disease, Aliment. Pharmacol. Ther., 2017, 46, 389–400 CrossRef CAS PubMed.
- L. Kaur, M. Gordon, P. A. Baines, Z. Iheozor-Ejiofor, V. Sinopoulou and A. K. Akobeng, Probiotics for induction of remission in ulcerative colitis, Cochrane Database Syst. Rev., 2020, 3, CD005573 Search PubMed.
- P. Mattarelli, C. Bonaparte, B. Pot and B. Biavati, Proposal to reclassify the three biotypes of Bifidobacterium longum as three subspecies: Bifidobacterium longum subsp. longum subsp. nov., Bifidobacterium longum subsp. infantis comb. nov. and Bifidobacterium longum subsp. suis comb. nov, Int. J. Syst. Evol. Microbiol., 2008, 58, 767–772 CrossRef CAS PubMed.
- M. Li, X. Zhou, C. Stanton, R. P. Ross, J. Zhao, H. Zhang, B. Yang and W. Chen, Comparative genomics analyses reveal the differences between B. longum subsp. infantis and B. longum subsp. longum in carbohydrate utilisation, CRISPR-Cas systems and bacteriocin operons, Microorganisms, 2021, 9, 1713 CrossRef CAS PubMed.
- D. Groeger, L. O'Mahony, E. F. Murphy, J. F. Bourke, T. G. Dinan, B. Kiely, F. Shanahan and E. M. Quigley, Bifidobacterium infantis, 35624 modulates host inflammatory processes beyond the gut, Gut Microbes, 2013, 4, 325–339 CrossRef PubMed.
- H. Tamaki, H. Nakase, S. Inoue, C. Kawanami, T. Itani, M. Ohana, T. Kusaka, S. Uose, H. Hisatsune, M. Tojo, T. Noda, S. Arasawa, M. Izuta, A. Kubo, C. Ogawa, T. Matsunaka and M. Shibatouge, Efficacy of probiotic treatment with Bifidobacterium longum, 536 for induction of remission in active ulcerative colitis: A randomized, double-blinded, placebo-controlled multicenter trial, Dig. Endosc., 2016, 28, 67–74 CrossRef PubMed.
- S. Yan, B. Yang, J. C. Zhao, J. X. Zhao, C. Stanton, R. P. Ross, H. Zhang and W. Chen, A ropy exopolysaccharide producing strain Bifidobacterium longum subsp. longum YS108R alleviates DSS-induced colitis by maintenance of the mucosal barrier and gut microbiota modulation, Food Funct., 2019, 10, 1595–1608 RSC.
- D. Srutkova, M. Schwarzer, T. Hudcovic, Z. Zakostelska, V. Drab, A. Spanova, B. Rittich, H. Kozakova and I. Schabussova, Bifidobacterium longum CCM 7952 promotes epithelial barrier function and prevents acute DSS-induced colitis in strictly strain-specific manner, PLoS One, 2015, 10, e0134050 CrossRef PubMed.
- E. Miyauchi, T. Ogita, J. Miyamoto, S. Kawamoto, H. Morita, H. Ohno, T. Suzuki and S. Tanabe, Bifidobacterium longum alleviates dextran sulfate sodium-induced colitis by suppressing IL-17A response: Involvement of intestinal epithelial costimulatory molecules, PLoS One, 2013, 8, e79735 CrossRef CAS PubMed.
- S. N. S. Murthy, H. S. Cooper, H. Shim, R. S. Shah, S. A. Ibrahim and D. J. Sedergran, Treatment of dextran sulfate sodium-induced murine colitis by intracolonic cyclosporing, Dig. Dis. Sci., 1993, 38, 1722–1734 CrossRef CAS PubMed.
- B. Yang, M. J. Li, S. Wang, R. P. Ross, C. Stanton, J. N. Zhao, H. Zhang and W. Chen, Lactobacillus ruminis alleviates DSS-induced colitis by inflammatory cytokines and gut microbiota modulation, Foods, 2021, 10, 1349 CrossRef PubMed.
- L. A. Dieleman, M. J. H. J. Palmen, H. Akol, E. Bloemena, A. S. Pena, S. G. M. Meuwissen and E. P. van Rees, Chronic experimental colitis induced by dextran sulphate sodium (DSS) is characterized by Th1 and Th2 cytokines, Clin. Exp. Immunol., 1998, 114, 385–391 CrossRef CAS PubMed.
- B. Y. Mao, D. Y. Li, C. Q. Ai, J. X. Zhao, H. Zhang and W. Chen, Lactulose differently modulates the composition of luminal and mucosal microbiota in C57BL/6J mice, J. Agric. Food Chem., 2016, 64, 6240–6247 CrossRef CAS PubMed.
- B. Yang, H. Q. Chen, H. Gao, J. T. Wang, C. Stanton, R. P. Ross, H. Zhang and W. Chen,
Bifidobacterium breve CCFM683 could ameliorate DSS-induced colitis in mice primarily via conjugated linoleic acid production and gut microbiota modulation, J. Funct. Foods, 2018, 49, 61–72 CrossRef CAS.
- Y. Chen, Y. Jin, C. Stanton, R. P. Ross, J. X. Zhao, H. Zhan, B. Yang and W. Chen, Alleviation effects of Bifidobacterium breve on DSS-induced colitis depends on intestinal tract barrier maintenance and gut microbiota modulation, Eur. J. Nutr., 2021, 60, 369–387 CrossRef CAS PubMed.
- J. Chong, P. Liu, G. Y. Zhou and J. G. Xia, Using MicrobiomeAnalyst for comprehensive statistical, functional, and meta-analysis of microbiome data, Nat. Protoc., 2020, 15, 799–821 CrossRef CAS PubMed.
- N. Segata, J. Izard, L. Waldron, D. Gevers, L. Miropolsky, W. S. Garrett and C. Huttenhower, Metagenomic biomarker discovery and explanation, Genome Biol., 2011, 12, R60 CrossRef PubMed.
- S. J. Klebanoff, Myeloperoxidase: friend and foe, J. Leukocyte Biol., 2005, 77, 598–625 CrossRef CAS PubMed.
- H. Wang, C. F. Fan, Z. E. Zhao, Z. Y. Zhai and Y. L. Hao, Anti-inflammatory effect of Bifidobacterium animalis subsp. lactis, A6 on DSS-induced colitis in mice, J. Appl. Microbiol., 2022, 133, 2063–2073 CrossRef CAS PubMed.
- C. C. Zhang, Y. Zhao, J. C. Jiang, L. L. Yu, F. W. Tian, J. X. Zhao, H. Zhang, W. Chen and Q. X. Zhai, Identification of the key characteristics of Bifidobacterium longum strains for the alleviation of ulcerative colitis dagger, Food Funct., 2021, 12, 3476–3492 RSC.
- Q. Y. Cui, X. Y. Tian, X. Liang, Z. Zhang, R. Wang, Y. Zhou, H. X. Yi, P. M. Gong, K. Lin, T. J. Liu and L. W. Zhang,
Bifidobacterium bifidum relieved DSS-induced
colitis in mice potentially by activating the aryl hydrocarbon receptor, Food Funct., 2022, 13, 5115–5123 RSC.
- S. E. Jang, J. J. Jeong, J. K. Kim, M. J. Han and D. H. Kim, Simultaneous amelioratation of colitis and liver injury in mice by Bifidobacterium longum LC67 and Lactobacillus plantarum LC27, Sci. Rep., 2018, 8, 7500 CrossRef PubMed.
- S. M. Lim, J. J. Jeong, S. E. Jang, M. J. Han and D. H. Kim, A mixture of the probiotic strains Bifidobacterium longum CH57 and Lactobacillus brevis CH23 ameliorates colitis in mice by inhibiting macrophage activation and restoring the Th17/Treg balance, J. Funct. Foods, 2016, 27, 295–309 CrossRef CAS.
- I. A. Lee, E. A. Bae, J. H. Lee, H. Lee, Y. T. Ahn, C. S. Huh and D. H. Kim,
Bifidobacterium longum HY8004 attenuates TNBS-induced colitis by inhibiting lipid peroxidation in mice, Inflammation Res., 2010, 59, 359–368 CrossRef CAS PubMed.
- T. Suzuki, Regulation of intestinal epithelial permeability by tight junctions, Cell. Mol. Life Sci., 2013, 70, 631–659 CrossRef CAS PubMed.
- Y. J. Hu, X. L. Jin, F. Gao, T. Lin, H. Zhu, X. Hou, Y. Yin, S. D. Kan and D. J. Chen, Selenium-enriched Bifidobacterium longum DD98 effectively ameliorates dextran sulfate sodium-induced ulcerative colitis in mice, Front. Microbiol., 2022, 13, 955112 CrossRef PubMed.
- Y. Chen, B. Yang, C. Stanton, R. P. Ross, J. X. Zhao, H. Zhang and W. Chen,
Bifidobacterium pseudocatenulatum ameliorates DSS-induced colitis by maintaining intestinal mechanical barrier, blocking proinflammatory cytokines, inhibiting TLR4/NF-kappaB signaling, and altering gut microbiota, J. Agric. Food Chem., 2021, 69, 1496–1512 CrossRef CAS PubMed.
- A. U. Din, A. Hassan, Y. Zhu, K. Zhang, Y. Wang, T. Li, Y. Wang and G. Wang, Inhibitory effect of Bifidobacterium bifidum ATCC 29521 on colitis and its mechanism, J. Nutr. Biochem., 2020, 79, 108353 CrossRef CAS PubMed.
- P. Paone and P. D. Cani, Mucus barrier, mucins and gut microbiota: the expected slimy partners?, Gut, 2020, 69, 2232–2243 CrossRef CAS PubMed.
- M. Van der Sluis, B. A. E. De Koning, A. De Bruijn, A. Velcich, J. P. P. Meijerink, J. B. Van Goudoever, H. A. Buller, J. Dekker, I. Van Seuningen, I. B. Renes and A. W. C. Einerhand, Muc2-deficient mice spontaneously develop colitis, indicating that Muc2 is critical for colonic protection, Gastroenterology, 2006, 131, 117–129 CrossRef CAS PubMed.
- C. Gunther, H. Neumann, M. F. Neurath and C. Becker, Apoptosis, necrosis and necroptosis: cell death regulation in the intestinal epithelium, Gut, 2013, 62, 1062–1071 CrossRef PubMed.
- E. K. Joseph and J. D. Levine, Caspase signalling in neuropathic and inflammatory pain in the rat, Eur. J. Neurosci., 2004, 20, 2896–2902 CrossRef PubMed.
- C. T. Capaldo, D. N. Powell and D. Kalman, Layered defense: How mucus and tight junctions seal the intestinal barrier, J. Mol. Med., 2017, 95, 927–934 CrossRef CAS PubMed.
- Y. Huang and Z. E. Chen, Inflammatory bowel disease related innate immunity and adaptive immunity, Am. J. Transl. Res., 2016, 8, 2490–2497 CAS.
- R. Yu, F. L. Zuo, H. Q. Ma and S. W. Chen, Exopolysaccharide-producing Bifidobacterium adolescentis strains with similar adhesion property induce differential regulation of inflammatory immune response in Treg/Th17 axis of DSS-colitis mice, Nutrients, 2019, 11, 782 CrossRef CAS PubMed.
- N. R. Shin, T. W. Whon and J. W. Bae, Proteobacteria: Microbial signature of dysbiosis in gut microbiota, Trends Biotechnol., 2015, 33, 496–503 CrossRef CAS PubMed.
- S. Singh, R. Bhatia, P. Khare, S. Sharma, S. Rajarammohan, M. Bishnoi, S. K. Bhadada, S. S. Sharma, J. Kaur and K. K. Kondepudi, Anti-inflammatory Bifidobacterium strains prevent dextran sodium sulfate induced colitis and associated gut microbial dysbiosis in mice, Sci. Rep., 2020, 10, 18597 CrossRef CAS PubMed.
- S. A. Walujkar, D. P. Dhotre, N. P. Marathe, P. S. Lawate, R. S. Bharadwaj and Y. S. Shouche, Characterization of bacterial community shift in human ulcerative colitis patients revealed by Illumina based 16S rRNA gene amplicon sequencing, Gut Pathog., 2014, 6, 22 CrossRef PubMed.
- Y. J. Weng, D. X. Jiang, J. Liang, S. C. Ye, W. K. Tan, C. Y. Yu and Y. Zhou, Effects of Pretreatment with Bifidobacterium bifidum using 16S ribosomal RNA gene sequencing in a mouse model of acute colitis induced by dextran sulfate sodium, Med. Sci. Monit., 2021, 27, e928478 CrossRef CAS PubMed.
- N. Castano-Rodriguez, A. P. Underwood, J. Merif, S. M. Riordan, W. D. Rawlinson, H. M. Mitchell and N. O. Kaakoush, Gut microbiome analysis identifies potential etiological factors in acute gastroenteritis, Infect. Immun., 2018, 86, e00060 CrossRef CAS PubMed.
- L. Peng, X. Y. Gao, L. Nie, J. Xie, T. Y. Dai, C. Y. Shi, L. Tao, Y. Wang, Y. Tian and J. Sheng, Astragalin attenuates dextran sulfate sodium (DSS)-induced acute experimental colitis by alleviating gut microbiota dysbiosis and inhibiting NF-kappaB activation in mice, Front. Immunol., 2020, 11, 2058 CrossRef CAS PubMed.
- Z. X. Zhang, L. Taylor, N. Shommu, S. Ghosh, R. Reimer, R. Panaccione, S. Kaur, J. E. Hyun, C. X. Cai, E. C. Deehan, N. Hotte, K. L. Madsen and M. Raman, A diversified dietary pattern is associated with a balanced gut microbial composition of Faecalibacterium and Escherichia/Shigella in patients with crohn's disease in remission, J. Crohns Colitis, 2020, 14, 1547–1557 CrossRef PubMed.
- F. Shen, R. D. Zheng, X. Q. Sun, W. J. Ding, X. Y. Wang and J. G. Fan, Gut microbiota dysbiosis in patients with non-alcoholic fatty liver disease, Hepatobiliary Pancreatic Dis. Int., 2017, 16, 375–381 CrossRef PubMed.
- N. N. Wang, S. Wang, B. F. Xu, F. Liu, G. C. Huo and B. L. Li, Alleviation effects of Bifidobacterium animalis subsp. lactis XLTG11 on dextran sulfate sodium-induced colitis in mice, Microorganisms, 2021, 9, 2093 CrossRef CAS PubMed.
- L. J. Shang, H. B. Liu, H. T. Yu, M. X. Chen, T. R. Yang, X. F. Zeng and S. Y. Qiao, Core altered microorganisms in colitis mouse model: A comprehensive time-point and fecal microbiota transplantation analysis, Antibiotics, 2021, 10, 643 CrossRef CAS PubMed.
- K. Machiels, M. Joossens, J. Sabino, V. De Preter, I. Arijs, V. Eeckhaut, V. Ballet, K. Claes, F. Van Immerseel, K. Verbeke, M. Ferrante, J. Verhaegen, P. Rutgeerts and S. Vermeire, A decrease of the butyrate-producing species Roseburia hominis and Faecalibacterium prausnitzii defines dysbiosis in patients with ulcerative colitis, Gut, 2014, 63, 1275–1283 CrossRef CAS PubMed.
- D. R. Donohoe, N. Garge, X. X. Zhang, W. Sun, T. M. O'Connell, M. K. Bunger and S. J. Bultman, The microbiome and butyrate regulate energy metabolism and autophagy in the mammalian colon, Cell Metab., 2011, 13, 517–526 CrossRef CAS PubMed.
- M. A. R. Vinolo, H. G. Rodrigues, R. T. Nachbar and R. Curi, Regulation of inflammation by short chain fatty acids, Nutrients, 2011, 3, 858–876 CrossRef CAS PubMed.
- D. P. Venegas, M. K. De la Fuente, G. Landskron, M. J. Gonzalez, R. Quera, G. Dijkstra, H. J. M. Harmsen, K. N. Faber and M. A. Hermoso, Short chain fatty acids (SCFAs)-mediated gut epithelial and immune regulation and its relevance for inflammatory bowel diseases, Front. Immunol., 2019, 10, 277 CrossRef CAS PubMed.
- C. C. Yang, Y. Du, D. Y. Ren, X. B. Yang and Y. Zhao, Gut microbiota-dependent catabolites of tryptophan play a predominant role in the protective effects of turmeric polysaccharides against DSS-induced ulcerative colitis, Food Funct., 2021, 12, 9793–9807 RSC.
- M. S. Zhou, B. Zhang, Z. L. Gao, R. P. Zheng, D. Marcellin, A. Saro, J. Pan, L. Chu, T. S. Wang and J. F. Huang, Altered diversity and composition of gut microbiota in patients with allergic rhinitis, Microb. Pathog., 2021, 161, 105272 CrossRef CAS PubMed.
- R. Yamamura, K. Nakamura, N. Kitada, T. Aizawa, Y. Shimizu, K. Nakamura, T. Ayabe, T. Kimura and A. Tamakoshi, Associations of gut microbiota, dietary intake, and serum short-chain fatty acids with fecal short-chain fatty acids, Biosci. Microbiota, Food Health, 2020, 39, 11–17 CrossRef CAS PubMed.
|
This journal is © The Royal Society of Chemistry 2023 |
Click here to see how this site uses Cookies. View our privacy policy here.