DOI:
10.1039/D2FO01476A
(Paper)
Food Funct., 2023,
14, 369-387
Okanin from Coreopsis tinctoria Nutt. alleviates cognitive impairment in bilateral common carotid artery occlusion mice by regulating the miR-7/NLRP3 axis in microglia†
Received
30th May 2022
, Accepted 13th November 2022
First published on 25th November 2022
Abstract
Cognitive impairment is the main clinical feature following stroke, and microglia-mediated inflammatory response is a major contributor to it. Coreopsis tinctoria Nutt., an edible chrysanthemum, is commonly used as a functional ingredient in healthcare beverages and food. Okanin, the main active ingredient of Coreopsis tinctoria Nutt. flower, inhibits microglial activation. However, the role of okanin in cognitive impairment following ischemic stroke is still unknown. In this study, we investigated the effect of okanin on ischemic stroke and its underlying mechanism both in vivo and in vitro. Okanin was found to attenuate cognitive impairment in bilateral common carotid artery occlusion (BCCAO) mice, inhibit neuronal loss and microglial activation, decrease NOD-like receptor family pyrin domain containing 3 (NLRP3) inflammasome activation, and increase miR-7 expression. Okanin suppressed NLRP3 inflammasome activation in oxygen-glucose deprivation (OGD) and lipopolysaccharide (LPS)-stimulated microglia by increasing miR-7 expression and inhibited microglia-induced neuronal injury. This study provides new insights into the role of okanin in ischemic stroke and shows that the miR-7/NLRP3 axis plays an important role in mediating the beneficial effects of okanin on cerebral ischemia. These findings suggest that okanin has great potential as a functional food for stroke recovery.
1. Introduction
Ischemic stroke is one of the most common cerebrovascular diseases with a high mortality rate, and a reduction in cerebral blood flow is a major cause of ischemic stroke.1,2 Although there has been a steady decline in ischemic stroke-associated mortality over the past few decades, many survivors develop cognitive impairment after the stroke.3,4 Post-stroke cognitive impairment is a complex pathogenetic event, and an inflammatory response is a major contributor to it.5 Neuroinflammation is a long-lasting response following stroke onset and is thought to be a key factor in brain damage.6 Several studies indicate that inhibition of neuroinflammation might be a potential therapeutic strategy for the alleviation of post-stroke cognitive impairment.7,8
Microglia, the resident immune cells in the brain, are one of the most important cell types that contribute to neuroinflammation.9 Microglia are activated and secrete cytokines causing neuronal death, following brain ischemia. Inflammasomes are highly expressed in the microglia and can trigger the immune system to react to ischemic injury. Increasing evidence has highlighted the crucial role of the NLRP3 inflammasome in the microglia-mediated inflammatory response.10,11
MicroRNAs (miRNAs), which are small noncoding RNAs, participate in various physiological and pathological processes.12 It has been reported that miR-7 is enriched in the brain.13 It can modulate microglial activation by directly targeting the 3′-UTR of NLRP3 mRNA and is involved in the post-transcriptional regulation of NLRP3 expression.14 Previous studies have demonstrated that miR-7 overexpression can alleviate brain ischemic injury.15,16 Thus the interference of miR-7 expression may be a promising approach to inhibit neuroinflammation following ischemic stroke.
Functional food-based interventions are gaining considerable attention in disease recovery and health maintenance owing to their high safety and diverse biological properties. Edible chrysanthemum possesses compounds with various bioactivities and is commonly used as a functional ingredient in healthcare beverages and food.17Coreopsis tinctoria Nutt. is a kind of edible chrysanthemum, which originated in North America, and is currently distributed worldwide.18 The Coreopsis tinctoria Nutt. flower exhibits multiple properties, including anti-hyperlipidemic, cardioprotective, neuroprotective, and glycemic regulation effects, and is widely used as a tea-like beverage owing to its unique flavor and potential health benefits.19 In a previous study, we found that compounds extracted from the Coreopsis tinctoria Nutt. flower possess anti-neuroinflammatory properties, among which okanin is the major effective constituent.20 However, the effect of okanin on ischemic stroke is still unknown. We hypothesized that the anti-inflammatory effect of okanin may promote cognitive function recovery following ischemic stroke. Hence, the current study is designed to investigate the function of okanin on BCCAO mice in vivo and OGD and LPS-stimulated microglia in vitro and explore whether the beneficial effect is related to the miR-7/NLRP3 signaling pathway.
2. Materials and methods
2.1 Animals
The animal handling procedures and experimental protocols were in accordance with the previous description.21 Briefly, the animals were free to obtain food and water and maintained under standard conditions of a 12 h light/12 h dark cycle. Twenty-four Swiss-Kunming mice (18–22 g, male) were used to establish the BCCAO model. All twenty-four mice (eight mice per experimental group) were used for behavioral tests. After that, fifteen of these mice (five mice per experimental group) were used for immunofluorescence assay and the other nine mice (three mice per experimental group) were used for western blot and quantitative real-time PCR assay.
2.2 Mouse BCCAO model
BCCAO surgery was used to induce cerebral ischemia. During surgery, the animals were anesthetized with 2.5% tribromoethanol (Avertine®; Sigma-Aldrich, St Louis, MO) intraperitoneally according to previous reports.22–26 The BCCAO surgery was performed as previously reported.27 Briefly, common carotid arteries were exposed and separated carefully and then clamped for 30 min with aneurysm clips. The mice in the BCCAO group and the BCCAO + Okanin group underwent the BCCAO surgery. The mice in the sham group underwent the same surgical interventions but without ligation.
2.3 Okanin preparation and grouping
Okanin was extracted from Coreopsis tinctoria Nutt. as previously reported.20 Briefly, ethanol extracts that were obtained from air-dried Coreopsis tinctoria Nutt. flowers were partitioned with equivoluminal petroleum ether. Then, petroleum ether extracts were passed through a flash silica gel column and eluted with petroleum ether/ethyl acetate (1
:
1). This fraction was subjected to polyamide column chromatography and eluted with 20% MeOH/H2O to obtain okanin (purity > 98%). The structure of okanin is shown in Fig. 1A. Okanin was suspended with 1.0% sodium carboxy methyl cellulose for in vivo study. The sham group, the BCCAO group and the BCCAO + Okanin group were included in the animal studies and the experimental mice were divided into these different groups randomly. The animals in the BCCAO + Okanin group were given okanin at a dose of 10 mg per kg per day by gavage from the first day to the thirteenth day. The experimental design schedule is shown in Fig. 1B.
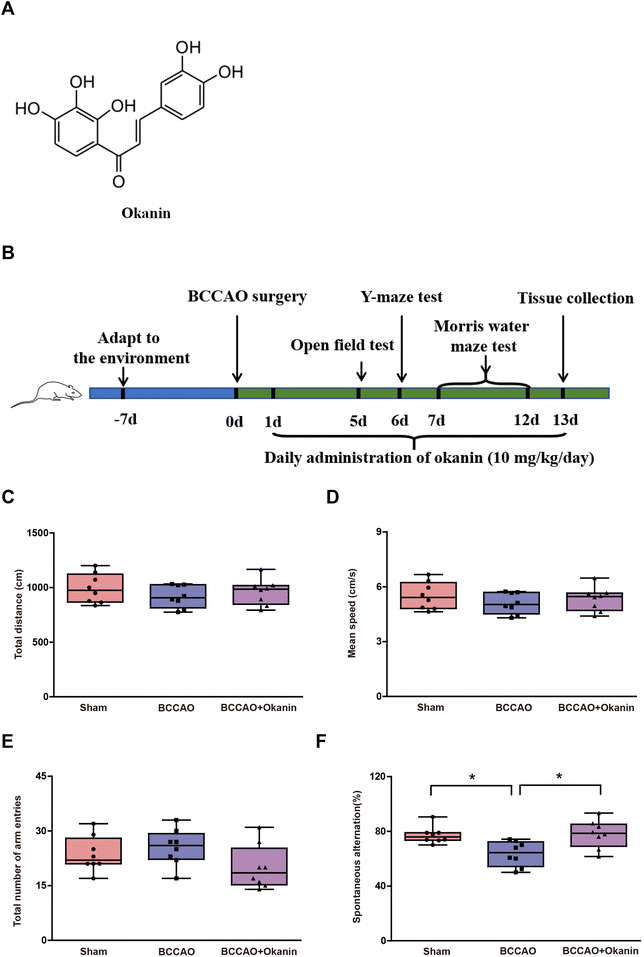 |
| Fig. 1 Okanin improves working memory in BCCAO mice. (A) The chemical structure of okanin. (B) The experimental design schedule where 10 mg per kg per day okanin was given to BCCAO mice by gavage. On the fifth day, an open field test was conducted. (C) Total distance and (D) mean speed were measured. On the sixth day, a Y-maze test was conducted. (E) Arm entry numbers and (F) the spontaneous alternation (%) were measured. Data are presented as box and whisker plots (n = 8 animals per group). One-way ANOVA with Bonferroni's post hoc test for statistical analyses. *p < 0.05 vs. the indicated group. | |
2.4 Behavioral tests
The locomotor activity of the mice was assessed on the fifth day after the BCCAO surgery by an open field test as previously reported.28 The working memory of the mice was evaluated on the sixth day after BCCAO surgery by a Y-maze test as previously reported.29 Spatial learning and the memory of the mice were evaluated from the seventh day to the twelfth day after the BCCAO surgery by a Morris water maze test as previously reported.29
2.5 Quantitative real-time PCR
MiR-7 expression was tested by a stem-loop real-time PCR system. Briefly, TRIzol reagent (Invitrogen, Carlsbad, CA, USA) and a TaqMan MicroRNA reverse transcription kit (Ambion, Carlsbad, CA, USA) were used for in vivo and in vitro RNA extraction and reverse transcription. U6 was used to normalize miR-7 expression. The loop-RT primer used for miR-7 was GTCGTATCCAGTGCAG GGTCCGAGGTATTCGCACTGGATACGACACAAA and the loop-RT primer for U6 was GTCGTATCCAGTGCAGGGTCCGAGGTATTCGCACTGGATACGACAAAAAT. The miR-7 forward primer used was AACCGGTGGAAGACTAGTGATT and the U6 forward primer was GAAGATTTAGCATGGCCCCTGC. The universal reverse primer used was CAGTGCAGG GTCCGAGGT.
2.6 Immunofluorescence
The animals were anesthetized and tissue collection was based on our previous study.30 Tissues for immunofluorescence assay were fixed in 4% paraformaldehyde and equilibrated in 20% and 30% sucrose. After cutting them into 20 μm-thick sections with a freezing microtome, goat serum was used to block the sections for 1 h. Iba-1 Rabbit monoclonal antibody (commercial numbers: ab178846) (Abcam, Cambridge, MA, USA) (1
:
500), NeuN Rabbit monoclonal antibody (commercial numbers: ab177487) (Abcam, Cambridge, MA, USA) (1
:
500) and IL-1β Mouse monoclonal antibody (commercial numbers: 12242) (CST, Boston, MA, USA) (1
:
400) were used to incubate the brain sections overnight at 4 °C. Then, the brain sections were incubated with Alexa Fluor 594- or Alexa Fluor 488-conjugated secondary antibodies for 2 h at room temperature. The images were acquired by using a fluorescence microscope. The fluorescence intensity was analyzed by Image J software.
2.7 Western blot
The protein of cerebral tissues or cell protein was collected as previously reported.30 The BCA kit (Beyotime, Shanghai, China) was used to detect the protein concentration and equal amounts of protein from each sample were used for immunoblotting analysis. After separation by SDS-polyacrylamide gel electrophoresis, the samples were transferred to a polyvinylidene fluoride membrane. NLRP3 Rabbit monoclonal antibody (commercial numbers: 15101) (CST, Boston, MA, USA) (1
:
1000), Caspase-1 Rabbit monoclonal antibody (commercial numbers: 24232) (CST, Boston, MA, USA) (1
:
1000), Cleaved Caspase-1 Rabbit monoclonal antibody (commercial numbers: 89332) (CST, Boston, MA, USA) (1
:
1000) and β-actin Mouse monoclonal antibody (commercial numbers: 3700) (CST, Boston, MA, USA) (1
:
1000) were used to incubate the membranes overnight at 4 °C after blocking with 5% skimmed milk. Then, horseradish peroxidase-conjugated secondary antibodies were used. Quantity One software (Bio-Rad) was used to test the band intensity.
2.8 Cell culture, OGD and LPS procedures, conditioned medium collection and miR-7 inhibitor transfection
The cells were kept at 37 °C in humidified 5% CO2. The DMEM medium used for culturing BV2 and SH-SY5Y cells contained 10% FBS, 100 U ml−1 penicillin and 100 μg ml−1 streptomycin.
Primary microglia were obtained from 1–2 day old mice, and primary neurons were obtained from 16–17 day old embryos of mice according to a previous study.31 For microglia, briefly, the meninges were removed, and the cerebral tissues were dissociated and digested. The cells were collected and cultured in DMEM containing FBS and penicillin/streptomycin. After 14–15 days, mixed glial cells were shaken and microglia were separated from the mixed glial cells. For neurons, briefly, the meninges were removed and cerebral tissues were collected and minced using 0.25% trypsin. DMEM/F12 containing FBS was used to terminate digestion and a pipette was used to perform dissociation. The cells were counted, pre-coated using poly-L-lysine and kept in a neurobasal medium containing B27, penicillin, and streptomycin.
For the OGD model, the cells were kept in 95% N2 and 5% CO2 and cultured in glucose-free DMEM for 4 h. Then the medium was refreshed with a normal culture medium or a normal culture medium containing different concentrations (1 μM, 5 μM, and 20 μM) of okanin for 24 h.
For the LPS model, BV2 cells were pre-treated for 2 h with different concentrations (1 μM, 5 μM, and 20 μM) of okanin and then co-treated with LPS (100 ng ml−1) for 24 h.
After OGD treatment, the culture medium of BV2 cells was collected as the conditioned medium and the collection procedure was that according to our previous study.32 The conditioned medium was used to culture SH-SY5Y cells or primary neurons and cell viability was measured by MTT assay.
An miR-7 inhibitor or negative control was mixed with Lipofectamine 2000 (Invitrogen, Carlsbad, CA, USA) according to the manufacturer's instructions. For in vitro experiments, BV2 cells were transfected with an miR-7 inhibitor or negative control, followed by OGD or LPS stimulation.
2.9 Enzyme-linked immunosorbent assay (ELISA)
The supernatant of cells was collected and the level of IL-1β was determined with an ELISA kit (NOVUS, Shanghai, China) according to the manufacturer's instructions.
2.10 Statistical analysis
The results of the comparison of the two groups were analyzed using the unpaired t-test. The results for the comparison of multiple factors were analyzed using one-way ANOVA or two-way ANOVA followed by Bonferroni's post hoc test. The correlation was analyzed using Spearman's rank correlation. The data of the behavioral tests are presented as a box and whisker plot. The data of other tests are presented as means ± SD. Data analyses were performed by using SPSS software and p < 0.05 was considered of statistical significance.
3. Results
3.1 Okanin improves working memory in BCCAO mice
To investigate the effects of okanin on locomotor activity, an open field test was performed. The total distance and mean speed were not observed as significant differences as shown in Fig. 1C and D among the sham group, the BCCAO group and the BCCAO + Okanin group. These results suggested that the BCCAO surgery or okanin administration did not affect the normal locomotor activity of mice. Normal locomotor activity was required for further cognitive function tests.33 To investigate the effects of okanin on working memory, a Y-maze test was performed. Fig. 1E shows that among the sham group, the BCCAO group and the BCCAO + Okanin group, the total number of arm entries did not show a significant difference and these data further confirmed that the locomotor activity of mice was not affected. Fig. 1F showed that compared with the sham mice, spontaneous alternation decreased in BCCAO mice, while okanin treatment increased spontaneous alternation. These results suggested that okanin improved working memory to alleviate cognitive impairment after BCCAO surgery.
3.2 Okanin improves spatial learning and memory in BCCAO mice
To investigate the effects of okanin on the abilities of spatial learning and memory, we performed a Morris water maze test. Fig. 2A and D show that among the sham group, the BCCAO group and the BCCAO + Okanin group, swimming speed did not show a significant difference during the navigation test and the probe test. Fig. 2B and C shows that among the sham group, the BCCAO group and the BCCAO + Okanin group, escape latency and distance did not show a significant difference from Day 7 to Day 9 in the navigation test. However, compared with sham mice, escape latency and distance increased in BCCAO mice, while okanin treatment decreased the escape latency and distance on Day 10 and Day 11. In the probe test, Fig. 2E shows that compared with sham mice, the target quadrant swimming time decreased in BCCAO mice, which was increased by okanin treatment. Fig. 2F shows that compared with the sham mice, the platform crossing numbers decreased in BCCAO mice, while okanin treatment increased the numbers. Representative tracks of movements among the different groups are shown in Fig. 2G. The results suggested that okanin enhanced the ability of spatial learning and memory to alleviate cognitive impairment after BCCAO surgery.
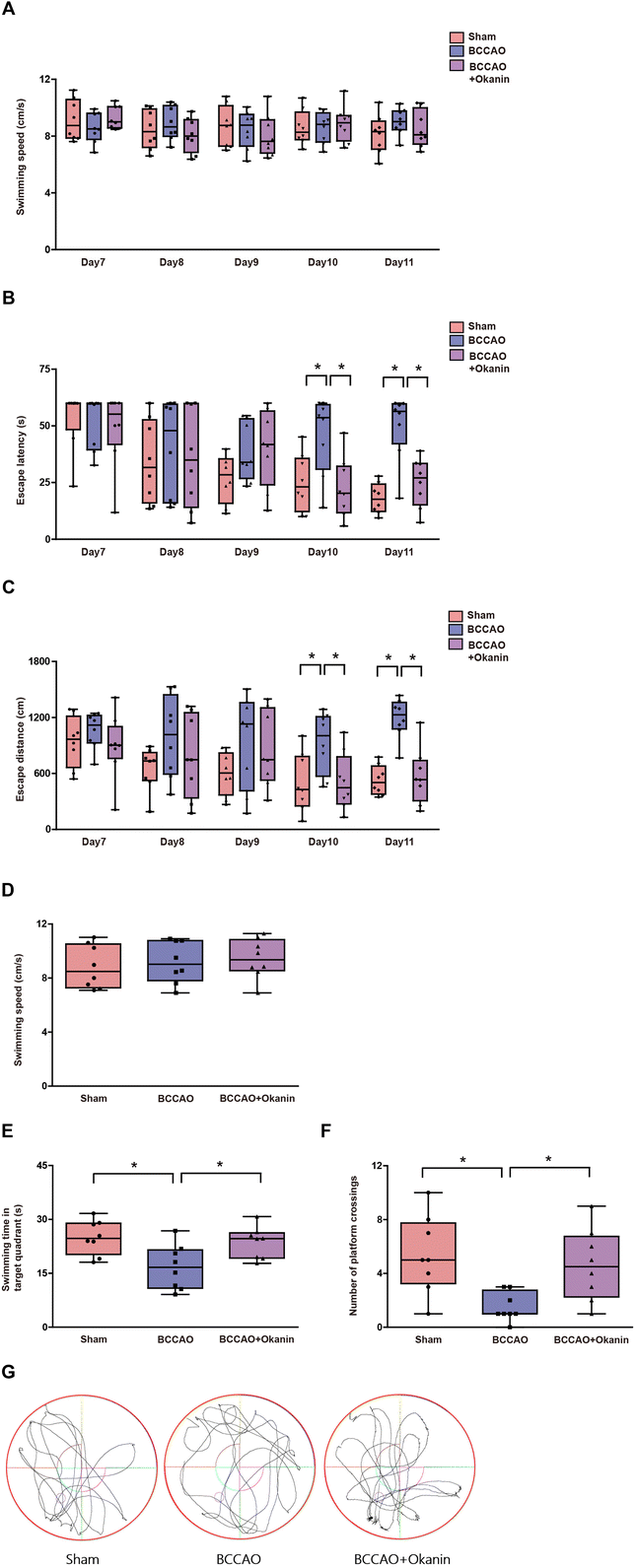 |
| Fig. 2 Okanin improves spatial learning and memory in BCCAO mice where 10 mg per kg per day okanin was given to BCCAO mice by gavage. From the seventh day to the eleventh day, (A) swimming speed and (B) and (C) escape latency and distance were measured in a Morris water maze navigation test. On the twelfth day, (D) swimming speed, (E) target quadrant swimming time, and (F) platform crossing numbers were measured in a Morris water maze probe test. (G) The representative tracks of the movements are shown. Data are presented as box and whisker plots (n = 8 animals per group). Two-way ANOVA with Bonferroni's post hoc test for (A)–(C) statistical analyses. One-way ANOVA with Bonferroni's post hoc test for (D)–(F) statistical analyses. *p < 0.05 vs. the indicated group. | |
3.3 Okanin inhibits neuronal loss and microglial activation in BCCAO mice
To investigate the neuroprotective and anti-inflammation effects of okanin, we examined neuronal loss and microglial activation in BCCAO mice. Fig. 3A shows that compared with the sham mice, the NeuN positive cells of hippocampal CA1 decreased in BCCAO mice, which was increased by okanin treatment. Fig. 3B shows that compared with the sham mice, the NeuN positive cells of hippocampal CA3 decreased in BCCAO mice, which was increased by okanin treatment. Moreover, Fig. 3C and D show that in hippocampal CA1 and CA3, compared with the sham mice, Iba-1 positive cells were increased in BCCAO mice, while okanin treatment decreased the Iba-1 positive cells. The correlation between NeuN and Iba-1 positive cells was investigated by Spearman rank correlation. Fig. 3E and F show that the positive cells of NeuN were negatively correlated with Iba-1 in hippocampal CA1 and CA3. These results suggested that okanin exerted neuroprotective and anti-inflammation effects after BCCAO surgery.
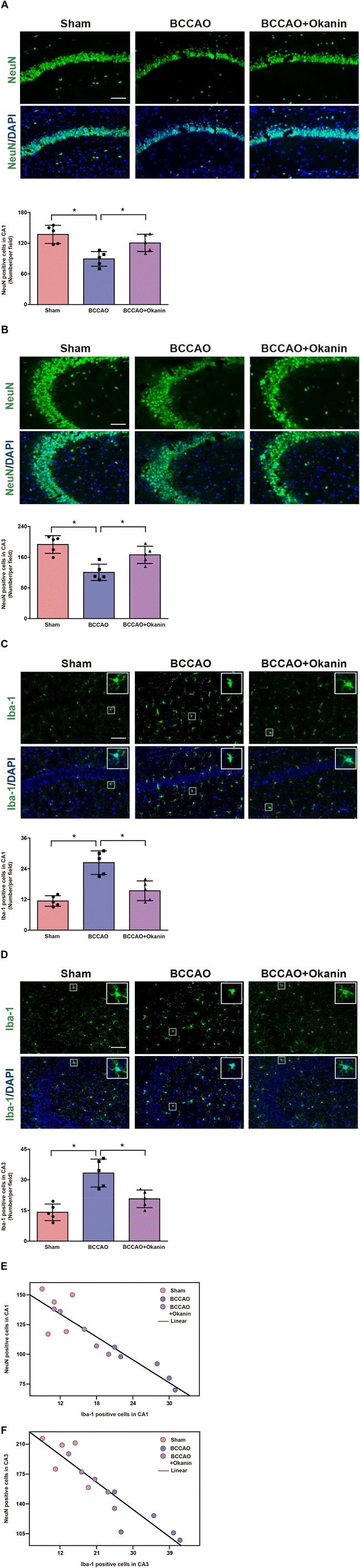 |
| Fig. 3 Okanin inhibits neuronal loss and microglial activation in BCCAO mice where 10 mg per kg per day okanin was given to BCCAO mice by gavage. (A) and (B) Representative photomicrographs of NeuN immunofluorescence in mouse hippocampal CA1 and CA3, and the statistical analysis. (C) and (D) Representative photomicrographs of Iba-1 immunofluorescence in mouse hippocampal CA1 and CA3, and the statistical analysis. (E) and (F) The correlation between NeuN and Iba-1 positive cells (CA1: r2 = 0.82, p < 0.05; CA3: r2 = 0.84, p < 0.05. Scale bar = 200 μm). Data are presented as means±SD (n = 5 animals per group). One-way ANOVA with Bonferroni's post hoc test for (A)–(D) statistical analyses. Spearman's correlation for (E) and (F) statistical analyses. *p < 0.05 vs. the indicated group. | |
3.4 Okanin regulates the miR-7/NLRP3 axis in BCCAO mice
To further investigate the possible anti-inflammation mechanism of okanin, we examined the miR-7/NLRP3 axis in BCCAO mice. As shown in Fig. 4A, the BCCAO mice showed a lower miR-7 expression, while okanin treatment increased miR-7 expression. As shown in Fig. 4B, the BCCAO mice showed elevated NLRP3 expression, which was decreased by okanin treatment. As shown in Fig. 4C and D, the BCCAO mice showed increased IL-1β positive cells, which was also decreased by okanin treatment. These results suggested that okanin exerted an anti-inflammation effect by regulating the miR-7 and NLRP3 signaling pathways after BCCAO surgery.
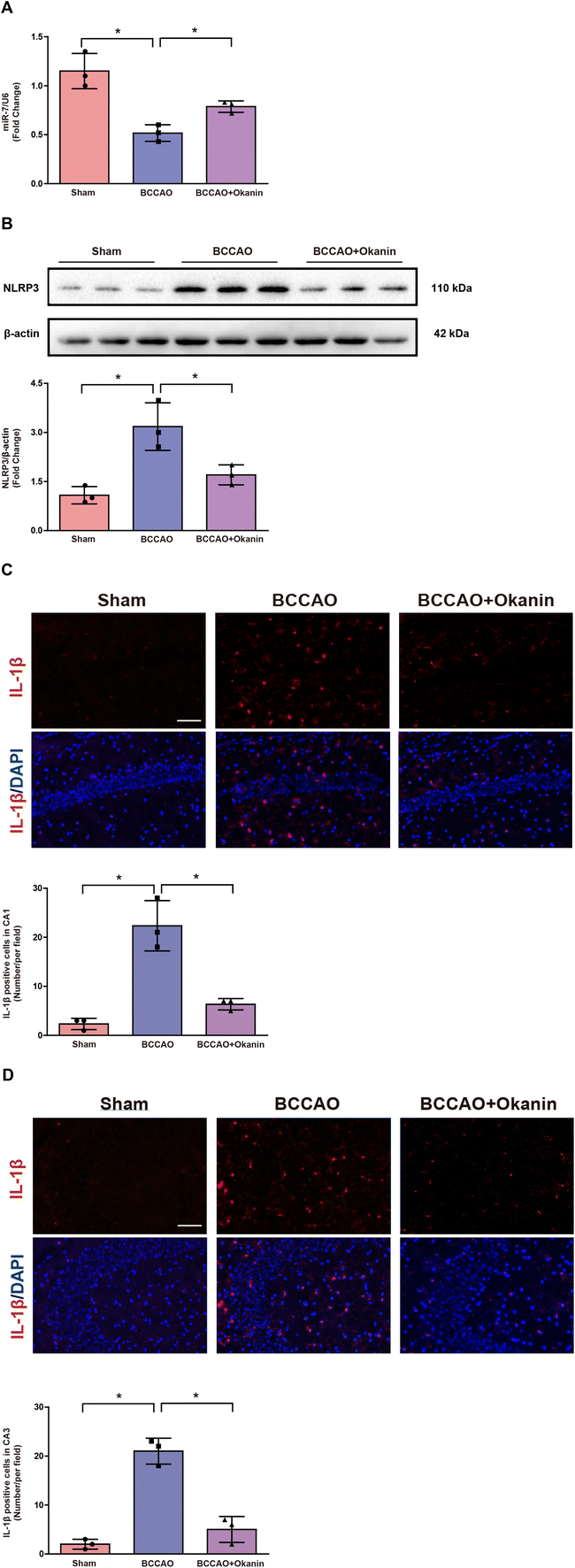 |
| Fig. 4 Okanin regulates the miR-7/NLRP3 axis in BCCAO mice where 10 mg per kg per day okanin was given to BCCAO mice by gavage. (A) Quantitative real-time PCR analysis of miR-7. (B) Immunoanalysis and quantification of NLRP3. (C) and (D) Representative photomicrographs of IL-1β immunofluorescence in mouse hippocampal CA1 and CA3, and statistical analysis. Scale bar = 200 μm. Data are presented as means±SD (n = 3 animals per group). One-way ANOVA with Bonferroni's post hoc test for statistical analyses. *p < 0.05 vs. the indicated group. | |
3.5 Okanin increases miR-7 expression and inhibits the NLRP3 signaling pathway in vitro
To explore the mechanism underlying the inhibitory effect of okanin on microglial activation, OGD- and LPS-induced cell models were used in in vitro experiments. As shown in Fig. 5A and B, okanin regulated miR-7 and NLRP3 expression in OGD-induced primary microglia. These results were consistent with the in vivo results. As shown in Fig. 5C–G, miR-7 expression decreased, and NLRP3 expression, C-Caspase-1 level and IL-1β production increased in OGD-induced BV2 cells compared with control cells, which were altered by okanin treatment in a concentration-dependent manner. Similarly, Fig. 5J–N show that compared with control cells, miR-7 expression decreased and NLRP3 expression, C-Caspase-1 level and IL-1β production increased in LPS-induced BV2 cells, while okanin treatment reversed the changes in a concentration-dependent manner. Moreover, we collected the conditioned medium of BV2 cells to culture neuronal cells. Fig. 5H shows that the SH-SY5Y cell viability decreased after treatment with OGD-induced BV2 cell-conditioned medium, while the conditioned medium collected from BV2 cells co-treated with okanin increased SH-SY5Y cell viability. Similarly, Fig. 5I shows that the viability of primary neurons decreased after treatment with OGD-induced BV2 cell-conditioned medium, while the conditioned medium collected from BV2 cells co-treated with okanin increased the viability of primary neurons. These results suggested that okanin regulated the miR-7/NLRP3 axis in microglia and its neuroprotective effect largely depended on the inhibitory effect on microglial activation.
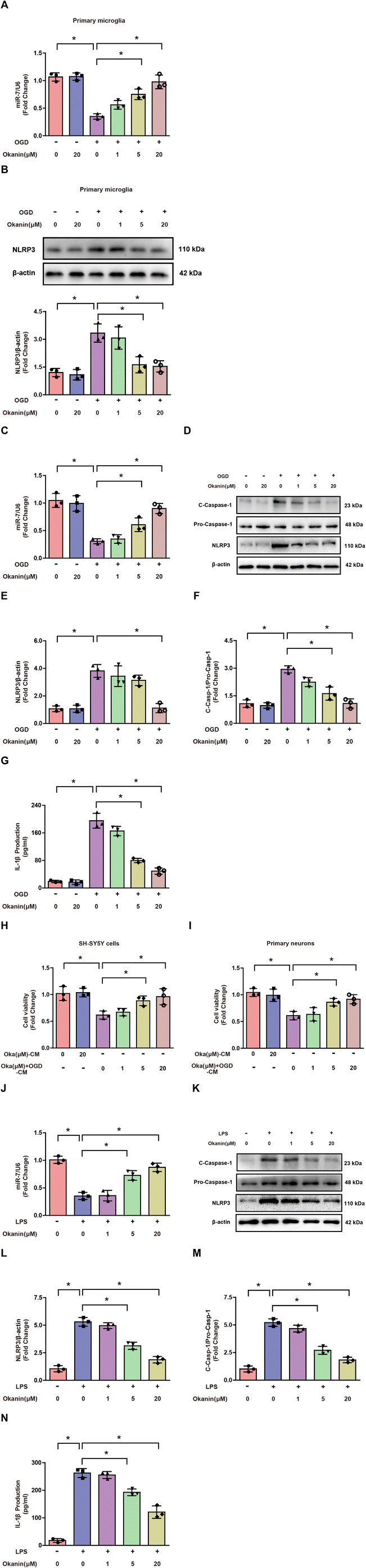 |
| Fig. 5 Okanin increases miR-7 expression and inhibits the NLRP3 signaling pathway in vitro. Okanin (1 μM, 5 μM, and 20 μM) was used to incubate ODG- or LPS-induced microglia. (A) and (B) Quantitative real-time PCR analysis of miR-7 and immunoanalysis and quantification of NLRP3 in OGD-induced primary microglia co-treated with okanin. (C) and (J) Quantitative real-time PCR analysis of miR-7 in OGD- or LPS-induced BV2 cells co-treated with okanin. (D)–(F) and (K)–(M) Immunoanalysis and quantification of NLRP3 and C-Caspase-1 in OGD- or LPS-induced BV2 cells co-treated with okanin. (G) and (N) ELISA assay analysis of IL-1β production in OGD- or LPS-induced BV2 cells co-treated with okanin. (H) MTT assay analysis of the viability of SH-SY5Y cells cultured in medium. (I) MTT assay analysis of the viability of primary neurons cultured in medium. Oka (μM)-CM: conditioned medium collected from okanin-treated BV2 cells. Oka (μM) + OGD-CM: conditioned medium collected from BV2 cells treated with OGD alone or OGD and okanin. Data are presented as means±SD (n = 3 independent cell batches). One-way ANOVA with Bonferroni's post hoc test for statistical analyses. *p < 0.05 vs. the indicated group. | |
3.6 MiR-7 is involved in the effect of okanin on the NLRP3 signaling pathway
In order to confirm the role of miR-7 in the effect of okanin on the NLRP3 signaling pathway, an miR-7 inhibitor was used for interfering with miR-7 expression. It is reported that miR-7 directly targets NLRP3 expression.14 To verify the transfection efficiency, BV2 cells were transfected with an miR-7 inhibitor or negative control. Compared to the negative control group, miR-7 inhibitor transfection increased the protein levels of NLRP3 in BV2 cells as shown in Fig. 6A. However, it is unclear whether miR-7 is involved in the effect of okanin on the NLRP3 signaling pathway. Hence, BV2 cells were transfected with miR-7 inhibitor or negative control, followed by OGD or LPS stimulation. Fig. 6B–D shows that the miR-7 inhibitor abolished the effect of okanin on NLRP3 expression and C-Caspase-1 level in OGD-induced BV2 cells. Similarly, as shown in Fig. 6G–I, in LPS-induced BV2 cells, okanin decreased NLRP3 expression and the C-Caspase-1 level, which was abolished by the miR-7 inhibitor. Moreover, Fig. 6E shows that the miR-7 inhibitor abolished the effect of the conditioned medium containing okanin on SH-SY5Y cells. Similarly, Fig. 6F shows that the conditioned medium containing okanin increased the viability of primary neurons, which was abolished by the miR-7 inhibitor. These results suggested that okanin inhibited the NLRP3 signaling pathway by regulating miR-7, and miR-7 plays a critical role in the anti-inflammatory and neuroprotective effects of okanin.
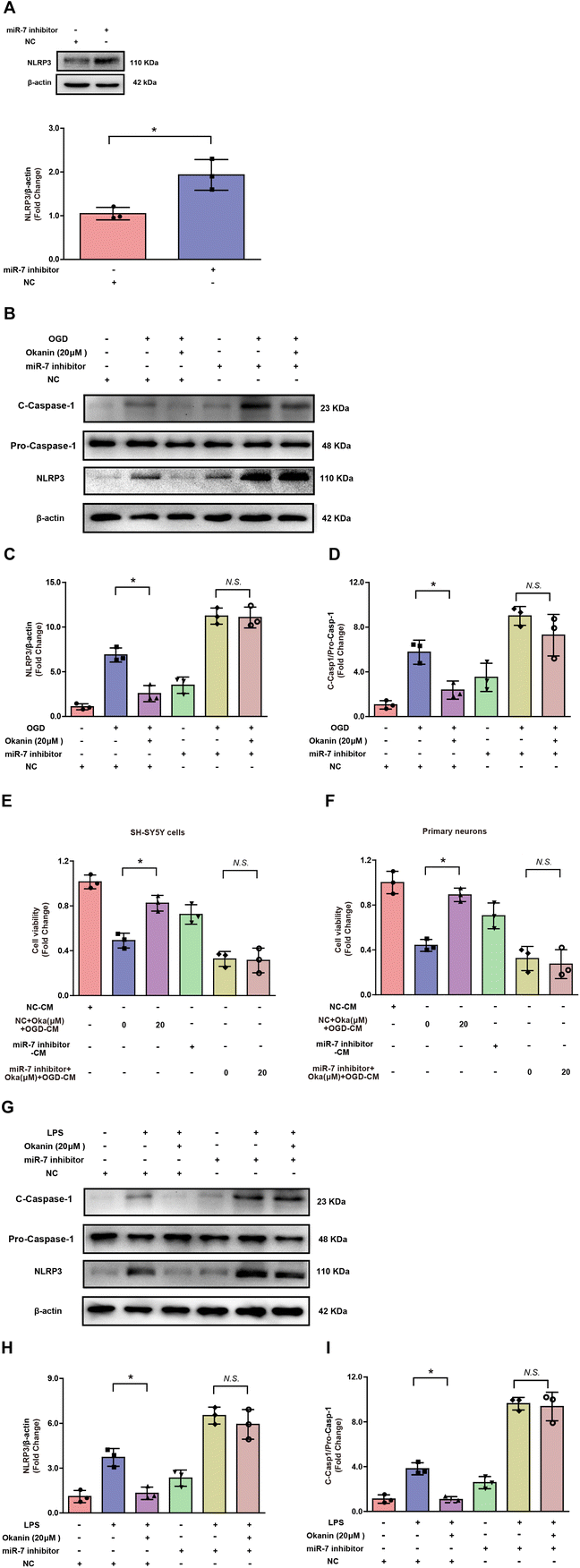 |
| Fig. 6 MiR-7 is involved in the effect of okanin on the NLRP3 signaling pathway. Okanin (20 μM) was used to incubate ODG- or LPS-induced BV2 cells. (A) Immunoanalysis and quantification of NLRP3 in BV2 cells transfected with miR-7 inhibitor or negative control (NC). (B)–(D and (G)–(I) After miR-7 inhibitor or NC transfection, immunoanalysis and quantification of NLRP3 and C-Caspase-1 in OGD- or LPS-induced BV2 cells co-treated with okanin. (E) MTT assay analysis of the viability of SH-SY5Y cells cultured in the conditioned medium. (F) MTT assay analysis of the viability of primary neurons cultured in the conditioned medium. NC-CM: conditioned medium collected from NC-transfected BV2 cells. NC + Oka (μM) + OGD-CM: conditioned medium collected from NC-transfected BV2 cells treated with OGD alone or OGD and okanin. MiR-7 inhibitor-CM: conditioned medium collected from miR-7 inhibitor-transfected BV2 cells. MiR-7 inhibitor + Oka (μM) + OGD-CM: conditioned medium collected from miR-7 inhibitor-transfected BV2 cells treated with OGD alone or OGD and okanin. Data are presented as means±SD (n = 3 independent cell batches). Unpaired t-test for (A) statistical analyses and two-way ANOVA with Bonferroni's post hoc test for (B)–(I) statistical analyses. *p < 0.05 vs. the indicated group. N.S., not significant. | |
4. Discussion
In the present study, we demonstrated, for the first time, that okanin has therapeutic roles in BCCAO mice and in OGD- and LPS-induced microglial cells as shown in Fig. 7. Moreover, the interference of miR-7 expression counteracted the inhibitory effect of okanin on the activation of the NLRP3 inflammasome, suggesting that miR-7 is involved in the anti-inflammatory and neuroprotective roles of okanin.
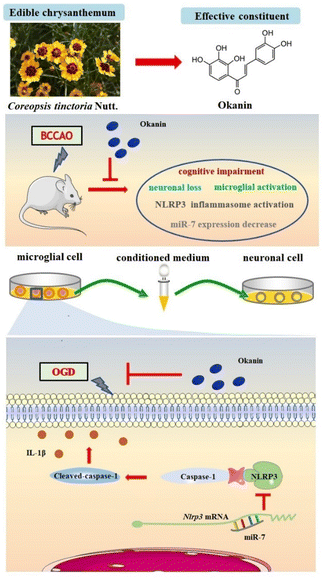 |
| Fig. 7 Schematic depiction of the proposed mechanism of okanin. Okanin from Coreopsis tinctoria Nutt. attenuated cognitive impairment in BCCAO mice, inhibited neuronal loss and microglial activation, decreased NLRP3 inflammasome activation and increased miR-7 expression. Okanin suppressed NLRP3 inflammasome activation in OGD- and LPS-stimulated microglia by increasing miR-7 expression, and inhibited microglia-induced neuronal injury. | |
Long-lasting neuroinflammation following ischemia persistently affects brain function.34 Cognitive decline is also common in patients with ischemic stroke, and the inflammatory response plays an important role in this development.35 Finding effective anti-inflammatory strategies may be instrumental for the rehabilitation of patients with ischemic stroke. Our previous studies showed that okanin, the active ingredient of Coreopsis tinctoria Nutt., could inhibit neuroinflammation effectively.20 In this study, we further investigated the role of okanin in the ischemic stroke model. Our results showed that the mice exhibited learning and memory function impairment after BCCAO surgery, which is consistent with the results of other studies.36,37 Okanin effectively improved the cognitive function as shown by the Y-maze and Morris water maze experiments. Neuronal loss is a major cause of functional cognitive deficits.38 The results showed that okanin significantly increased NeuN positive cells, indicating that okanin attenuated neuronal loss in BCCAO mice. These data suggest that okanin protects neurons and promotes the recovery of neurological functions.
Microglial activation is a prominent feature of neuroinflammation following ischemic stroke.39 The release of proinflammatory mediators by activated microglia is a major contributor to neuronal damage and death and also has a deleterious effect on cognitive function. In our study, we found that okanin significantly decreased the number of Iba-1 positive cells, indicating that okanin inhibited microglial activation in BCCAO mice. An increasing number of findings provide direct evidence to support the relationship between microglial activation and neuronal loss in injured brain regions.40,41 In this study, we analyzed the correlation between the positive cells of Iba-1 and NeuN, and our results showed a negative linear relationship between the two factors, indicating the important role of microglial activation in neuronal loss. Our results suggest that the therapeutic effects of okanin on ischemic stroke largely depend on its inhibitory effect on neuroinflammation.
The pivotal role of the NLRP3 inflammasome in the pathogenesis of ischemic stroke has been confirmed by several studies.42,43 The NLRP3 inflammasome, a multi-protein complex, consists of NLRP3, the adaptor protein ASC, and the precursor Caspase-1. NLRP3 inflammasome activation is accompanied by the cleavage of precursor Caspase-1, which further triggers IL-1β maturation. Enhanced expression of the NLRP3 inflammasome has been observed in ischemic stroke patients and animal models, and inhibition of the NLRP3 inflammasome has been considered to be a promising therapeutic approach.44 Our results showed that NLRP3 and IL-1β expressions increased in BCCAO mice, and okanin could significantly reverse this phenomenon. Microglia are the main sites of functional NLRP3 inflammasome formation following brain ischemia.45 Our in vitro study showed that okanin decreased the expressions of NLRP3 and C-Caspase-1, and IL-1β production in BV2 cells under OGD. It has been reported that the generation and release of IL-1β by microglia damage the surrounding neurons.14 Hence, we collected a microglia-conditioned medium to treat the neuronal cells. We found that okanin protected the OGD-damaged neuronal cells by regulating microglial activation. LPS has been reported to activate the NLRP3 inflammasome and trigger neuroinflammation. Inflammatory responses induced by microglial activation following ischemic stroke could be simulated in vitro by stimulating BV2 cells with LPS.46,47 In our study, we further confirmed the effect of okanin on NLRP3 inflammasome in LPS-induced BV2 cells. Okanin showed similar effects on both in vitro models. These data suggest that okanin suppresses NLRP3 inflammasome activation in vivo and in vitro, and exerts a neuroprotective effect in a concentration-dependent manner on NLRP3 inflammasome inhibition.
MiR-7 plays an essential role in ischemic stroke pathogenesis, and a reduction in miR-7 levels was observed following brain ischemia. It has been reported that miR-7 modulates neuroinflammation and protects neurons from inflammatory damage.14 In our study, we found that miR-7 expression decreased in BCCAO mice, and in LPS- and OGD-induced microglial cells, okanin significantly enhanced the levels of miR-7 in vivo and in vitro. NLRP3 is the target of miR-7, and the 3′-UTR of NLRP3 mRNA contains miR-7 binding sites. Importantly, the miR-7-induced downregulation of NLRP3 inhibits microglia-mediated neuroinflammation. Our in vitro data showed that treatment with an miR-7 inhibitor increased the NLRP3 levels. Moreover, in OGD- and LPS-induced BV2 cells, the miR-7 inhibitor counteracted the effect of okanin on the expressions of NLRP3 and C-Caspase-1. The data suggest that miR-7 is involved in the inhibitory effect of okanin on NLRP3 inflammasome.
Currently, drugs for the treatment of ischemic stroke are still limited. The anti-inflammation strategy has been regarded as a promising therapy. Our findings provide direct evidence that supports the function of okanin against ischemic stroke owing to the inhibition of neuroinflammation. In the present study, we mainly focused on the pharmaceutical effect and potential mechanisms of okanin. However, it is necessary to elucidate its pharmacokinetic characteristics in vivo including its specificity, safety, and the concentration of okanin that crosses the blood–brain barrier to reach the lesion. Moreover, there remains a limitation in this study. Although we found the effective dose and concentration of okanin for inhibiting microglial activation in vivo and in vitro, we could not exactly explain the relationship between the in vivo dose and the in vitro concentration of okanin, and how this relationship relates to its bioavailability at present. This may be due to the complexity of okanin absorption and distribution in vivo and the cross-talk between neurovascular units including neurons, microglia, astrocytes and endothelial cells during cerebral ischemia. These aspects need to be investigated further in future research.
5. Conclusion
The present study provides new insights into the role of okanin in ischemic stroke. Okanin improved working memory, enhanced spatial learning and memory in BCCAO mice, attenuated neuronal loss, inhibited microglial activation, suppressed NLRP3 inflammasome activation and increased miR-7 expression. Okanin suppressed NLRP3 inflammasome activation and inhibited microglia-induced neuronal injury in vitro by increasing miR-7 expression. Our results show that the miR-7/NLRP3 axis plays an important role in mediating the beneficial effects of okanin on cerebral ischemia and okanin has great potential as a functional food for stroke recovery.
Ethical statement
All animal-use procedures were in accordance with the Regulations of Experimental Animal Administration issued by the State Committee of Science and Technology of the People's Republic of China. The experiment was carried out with the approval of the Institutional Animal Ethical Care Committee of Shenyang Pharmaceutical University.
Author contributions
Yue Hou and Ning Li designed the research and revised the manuscript. Yan Mi, Qingqi Meng, Libin Xu and Yeshu Liu carried out the in vitro experiments and performed data analysis. Jikai Xu, Ruijia Shi, Yan Mi and Jingyu Liu carried out the in vivo experiments and performed data analyses. Di Zhou, Tingting Guo and Wei Li conducted the preparation of okanin. Yan Mi and Wei Li conducted the literature search and wrote the manuscript.
Conflicts of interest
All authors declare no conflicts of interest.
Acknowledgements
This work was financially supported by the National Natural Science Foundation of China (82174076, U1903122, and 81872768), the Fundamental Research Funds for the Central Universities (N2220002 and N2224004-1-01), the 111 Project (B16009), the Project of the State Key Laboratory for Chemistry and Molecular Engineering of Medicinal Resources (CMEMR2018-B01), the Liaoning Revitalization Talents Program (XLYC1807118) and the Shenyang Young Scientific and Technological Innovators Program (RC200408).
References
- C. Iadecola,
et al., Immune responses to stroke: mechanisms, modulation, and therapeutic potential, J. Clin. Invest., 2020, 130(6), 2777–2788 CrossRef CAS PubMed.
- G. A. Roth,
et al., Global Burden of Cardiovascular Diseases and Risk Factors, 1990–2019: Update From the GBD 2019 Study, J. Am. Coll. Cardiol., 2020, 76(25), 2982–3021 CrossRef PubMed.
- Y. Bejot,
et al., Influence of Preexisting Cognitive Impairment on Clinical Severity of Ischemic Stroke: The Dijon Stroke Registry, Stroke, 2020, 51(6), 1667–1673 CrossRef CAS PubMed.
- H. Kim,
et al., AIM2 inflammasome contributes to brain injury and chronic post-stroke cognitive impairment in mice, Brain, Behav., Immun., 2020, 87, 765–776 CrossRef CAS PubMed.
- A. Low,
et al., Inflammation and cerebral small vessel disease: A systematic review, Ageing Res. Rev., 2019, 53, 100916 CrossRef CAS PubMed.
- K. Shi,
et al., Global brain inflammation in stroke, Lancet Neurol., 2019, 18(11), 1058–1066 CrossRef PubMed.
- L. Jackson,
et al., Microglia knockdown reduces inflammation and preserves cognition in diabetic animals after experimental stroke, J. Neuroinflammation, 2020, 17(1), 137 CrossRef CAS PubMed.
- L. Jackson,
et al., Delayed Administration of Angiotensin II Type 2 Receptor (AT2R) Agonist Compound 21 Prevents the Development of Post-stroke Cognitive Impairment in Diabetes Through the Modulation of Microglia Polarization, Transl. Stroke Res., 2020, 11(4), 762–775 CrossRef CAS PubMed.
- S. Voet,
et al., Microglia in Central Nervous System Inflammation and Multiple Sclerosis Pathology, Trends Mol. Med., 2019, 25(2), 112–123 CrossRef CAS PubMed.
- J. Houtman,
et al., Beclin1-driven autophagy modulates the inflammatory response of microglia via NLRP3, EMBO J., 2019, 38(4), e99430 CrossRef PubMed.
- E. Lee,
et al., MPTP-driven NLRP3 inflammasome activation in microglia plays a central role in dopaminergic neurodegeneration, Cell Death Differ., 2019, 26(2), 213–228 CrossRef CAS PubMed.
- S. M. Hammond, An overview of microRNAs, Adv. Drug Delivery Rev., 2015, 87, 3–14 CrossRef.
- N. R. Choudhury,
et al., Tissue-specific control of brain-enriched miR-7 biogenesis, Genes Dev., 2013, 27(1), 24–38 CrossRef CAS PubMed.
- Y. Zhou,
et al., MicroRNA-7 targets Nod-like receptor protein 3 inflammasome to modulate neuroinflammation in the pathogenesis of Parkinson's disease, Mol. Neurodegener., 2016, 11, 28 CrossRef PubMed.
- Y. F. Dong,
et al., Potential role of microRNA-7 in the anti-neuroinflammation effects of nicorandil in astrocytes induced by oxygen-glucose deprivation, J. Neuroinflammation, 2016, 13(1), 60 CrossRef PubMed.
- T. Kim,
et al., The microRNA miR-7a-5p ameliorates ischemic brain damage by repressing alpha-synuclein, Sci. Signaling, 2018, 11(560), eaat4285 CrossRef CAS.
- J. Zheng,
et al., An update on the health benefits promoted by edible flowers and involved mechanisms, Food Chem., 2021, 340, 127940 CrossRef CAS.
- A. Peng,
et al., Classification of edible chrysanthemums based on phenolic profiles and mechanisms underlying the protective effects of characteristic phenolics on oxidatively damaged erythrocyte, Food Res. Int., 2019, 123, 64–74 CrossRef CAS PubMed.
- W. Wang,
et al., New phenolic compounds from Coreopsis tinctoria Nutt. and their antioxidant and angiotensin i-converting enzyme inhibitory activities, J. Agric. Food Chem., 2015, 63(1), 200–207 CrossRef CAS PubMed.
- N. Li,
et al., Anti-neuroinflammatory and NQO1 inducing activity of natural phytochemicals from Coreopsis tinctoria, J. Funct. Foods, 2015, 17, 837–846 CrossRef CAS.
- Y. Hou,
et al., Pterostilbene attenuates lipopolysaccharide- induced learning and memory impairment possibly via inhibiting microglia activation and protecting neuronal injury in mice, Prog. Neuropsychopharmacol. Biol. Psychiatry., 2014, 54, 92–102 CrossRef CAS PubMed.
- Y. Wang,
et al., Guhong injection promotes post-stroke functional recovery via attenuating cortical inflammation and apoptosis in subacute stage of ischemic stroke, Phytomedicine, 2022, 99, 154034 CrossRef CAS PubMed.
- M. Mota,
et al., Neuroprotective epi-drugs quench the inflammatory response and microglial/macrophage activation in a mouse model of permanent brain ischemia, J. Neuroinflammation, 2020, 17(1), 361 CrossRef CAS PubMed.
- Z. Li,
et al., GDF11 inhibits cardiomyocyte pyroptosis and exerts cardioprotection in acute myocardial infarction mice by upregulation of transcription factor HOXA3, Cell Death Dis., 2020, 11(10), 917 CrossRef CAS PubMed.
- X. Zhang,
et al., Ischemia-induced upregulation of autophagy preludes dysfunctional lysosomal storage and associated synaptic impairments in neurons, Autophagy, 2021, 17(6), 1519–1542 CrossRef CAS PubMed.
- J. J. Park,
et al., Coadministration of endothelial
and smooth muscle cells derived from human induced pluripotent stem cells as a therapy for critical limb ischemia, Stem Cells Transl. Med., 2021, 10(3), 414–426 CrossRef CAS PubMed.
- W. Zhang,
et al., Kellerin alleviates cognitive impairment in mice after ischemic stroke by multiple mechanisms, Phytother. Res., 2020, 34(9), 2258–2274 CrossRef CAS PubMed.
- J. Xu,
et al., Triad3A-Dependent TLR4 Ubiquitination and Degradation Contributes to the Anti-Inflammatory Effects of Pterostilbene on Vascular Dementia, J. Agric. Food Chem., 2022 Search PubMed , Online ahead of print.
- J. Xu,
et al., Pterostilbene Alleviates Aβ1–42-Induced Cognitive Dysfunction via Inhibition of Oxidative Stress by Activating Nrf2 Signaling Pathway, Mol. Nutr. Food Res., 2021, 65(2), e2000711 CrossRef CAS.
- J. Liu,
et al., Pterostilbene alleviates cerebral ischemia and reperfusion injury in rats by modulating microglial activation, Food Funct., 2020, 11(6), 5432–5445 RSC.
- Z. Zhang,
et al., The novel estrogenic receptor GPR30 alleviates ischemic injury by inhibiting TLR4-mediated microglial inflammation, J. Neuroinflammation, 2018, 15(1), 206 CrossRef PubMed.
- Y. Mi,
et al., Kellerin from Ferula sinkiangensis exerts neuroprotective effects after focal cerebral ischemia in rats by inhibiting microglia-mediated inflammatory responses, J. Ethnopharmacol., 2021, 269, 113718 CrossRef CAS PubMed.
- I. D. Pena,
et al., Effects of ginseol k-g3, an Rg3-enriched fraction, on scopolamine-induced memory impairment and learning deficit in mice, J. Ginseng Res., 2014, 38(1), 1–7 CrossRef.
- K. L. Lambertsen,
et al., Post-stroke inflammation-target or tool for therapy, Acta Neuropathol., 2019, 137(5), 693–714 CrossRef PubMed.
- A. Alawieh,
et al., Targeted complement inhibition salvages stressed neurons and inhibits neuroinflammation after stroke in mice, Sci. Transl. Med., 2018, 10(441), eaao6459 CrossRef PubMed.
- M. Ma,
et al., DPP-4 inhibition with linagliptin ameliorates cognitive impairment and brain atrophy induced by transient cerebral ischemia in type 2 diabetic mice, Cardiovasc. Diabetol., 2015, 14, 54 CrossRef PubMed.
- E. J. Yang,
et al., Neuroprotective Effects of Electroacupuncture on an Animal Model of Bilateral Common Carotid Artery Occlusion, Mol. Neurobiol., 2016, 53(10), 7228–7236 CrossRef CAS PubMed.
- E. E. Congdon and E. M. Sigurdsson, Tau-targeting therapies for Alzheimer disease, Nat. Rev. Neurol., 2018, 14(7), 399–415 CrossRef CAS.
- D. Li,
et al., Upregulation of Microglial ZEB1 Ameliorates Brain Damage after Acute Ischemic Stroke, Cell Rep., 2018, 22(13), 3574–3586 CrossRef CAS PubMed.
- E. E. Spangenberg,
et al., Eliminating microglia in Alzheimer's mice prevents neuronal loss without modulating amyloid-beta pathology, Brain, 2016, 139(Pt 4), 1265–1281 CrossRef PubMed.
- D. K. Wilton,
et al., Neuron-Glia Signaling in Synapse Elimination, Annu. Rev. Neurosci., 2019, 42, 107–127 CrossRef CAS PubMed.
- E. Lemarchand,
et al., Extent of Ischemic Brain Injury After Thrombotic Stroke Is Independent of the NLRP3 (NACHT, LRR and PYD Domains-Containing Protein 3) Inflammasome, Stroke, 2019, 50(5), 1232–1239 CrossRef CAS PubMed.
- P. Xu,
et al., Microglial TREM-1 receptor mediates neuroinflammatory injury via interaction with SYK in experimental ischemic stroke, Cell Death Dis., 2019, 10(8), 555 CrossRef PubMed.
- P. Hong,
et al., NLRP3 inflammasome as a potential treatment in ischemic stroke concomitant with diabetes, J. Neuroinflammation, 2019, 16(1), 121 CrossRef PubMed.
- Z. Gong,
et al., Mitochondrial dysfunction induces NLRP3 inflammasome activation during cerebral ischemia/reperfusion injury, J. Neuroinflammation, 2018, 15(1), 242 CrossRef PubMed.
- Y. Li,
et al., Propane-2-sulfonic acid octadec-9-enyl-amide, a novel PPARalpha/gamma dual agonist, protects against ischemia-induced brain damage in mice by inhibiting inflammatory responses, Brain, Behav., Immun., 2017, 66, 289–301 CrossRef CAS PubMed.
- X. Wang,
et al., miRNA-3473b contributes to neuroinflammation following cerebral ischemia, Cell Death Dis., 2018, 9(1), 11 CrossRef PubMed.
|
This journal is © The Royal Society of Chemistry 2023 |
Click here to see how this site uses Cookies. View our privacy policy here.